- 1Applied Biomechanics Laboratory, Department of Integrative Physiology, University of Colorado Boulder, Boulder, CO, United States
- 2Applied Biomechanics Laboratory, Eastern Colorado Healthcare System, Department of Veterans Affairs, Denver, CO, United States
People with a transtibial amputation using passive-elastic prostheses exhibit reduced prosthetic ankle power and push-off work compared to non-amputees and compensate by increasing their affected leg (AL) hip joint work and unaffected leg (UL) ankle, knee, and hip joint and leg work during level-ground walking. Use of a powered ankle–foot prosthesis normalizes step-to-step transition work during level-ground walking over a range of speeds for people with a transtibial amputation, but the effects on joint work during level-ground, uphill, and downhill walking have not been assessed. We investigated how use of passive-elastic and powered ankle–foot prostheses affect leg joint biomechanics during level-ground and sloped walking. 10 people with a unilateral transtibial amputation walked at 1.25 m/s on a dual-belt force-measuring treadmill at 0°, ±3°, ±6°, and ±9° using their own passive-elastic and a powered prosthesis (BiOM T2, BionX Medical Technologies, Inc., Bedford, MA, USA) while we measured kinematic and kinetic data. We calculated AL and UL prosthetic, ankle, knee, hip, and individual leg positive, negative, and net work. Use of a powered compared to passive-elastic ankle–foot prosthesis resulted in greater AL prosthetic and individual leg net work on uphill and downhill slopes. Over a stride, AL prosthetic positive work was 23–30% greater (p < 0.05) during walking on uphill slopes of +6°, and +9°, prosthetic net work was up to 10 times greater (more positive) (p ≤ 0.005) on all uphill and downhill slopes and individual leg net work was 146 and 82% more positive (p < 0.05) at uphill slopes of +6° and +9°, respectively, with use of the powered compared to passive-elastic prosthesis. Greater prosthetic positive and net work through use of a powered ankle–foot prosthesis during uphill and downhill walking improves mechanical work symmetry between the legs, which could decrease metabolic cost and improve functional mobility in people with a transtibial amputation.
Introduction
Typically, people with a transtibial amputation are prescribed a passive-elastic energy storage and return (ESAR) prosthesis that is made of carbon fiber and functions like a spring with no ability to generate power anew or to articulate. When people with a unilateral transtibial amputation use such passive-elastic prostheses, they have 10–30% higher metabolic demands to walk at the same speeds as non-amputees (Torburn et al., 1995; Waters and Mulroy, 1999; Hsu et al., 2006) and compensate for the lack of prosthetic push-off work with increased unaffected leg (UL) and decreased affected leg (AL) step-to-step transition work (Herr and Grabowski, 2012; Adamczyk and Kuo, 2015; Russell Esposito et al., 2016). People with a transtibial amputation using an ESAR prosthesis also exhibit slower preferred walking velocities (Herr and Grabowski, 2012; Russell Esposito et al., 2014), increased sagittal plane angular momentum (Pickle et al., 2016), and increased knee joint adduction moments in their UL (Grabowski and D’Andrea, 2013) compared to non-amputees. In addition, when walking on level ground using passive-elastic prostheses, people with a transtibial amputation exhibit an increase in knee flexion in their AL compared to their UL at heel-strike and activate their AL biceps femoris more than their UL biceps femoris, suggesting that greater work is absorbed at the knee (Isakov et al., 2000). The increased AL knee flexion has been attributed to the shape of the prosthetic socket that is designed to increase patellar tendon loading for patellar tendon bearing sockets (Isakov et al., 2000). However, when people with a transtibial amputation walked on level ground with a conventional solid-ankle cushioned heel prosthesis, there was almost no positive or negative sagittal plane knee power during the first half of the stance phase (Winter and Sienko, 1988). The advent of ESAR prosthetic feet has resulted in no changes to knee sagittal plane range of motion compared to use of older, conventional solid-ankle cushioned heel prostheses (Postema et al., 1997) though it is not yet known how more advanced prostheses affect knee sagittal plane moments and powers. Normative knee moments and powers could improve symmetry between legs and mechanical energy transfer across the AL knee joint of people with transtibial amputations.
Previous modeling and experimental studies have found that people with a unilateral transtibial amputation walking 0.6–1.6 m/s over level ground while using a passive-elastic prosthesis compensate for reduced ankle push-off work with increased UL and AL hip positive work (Zmitrewicz et al., 2006; Silverman et al., 2008; Adamczyk and Kuo, 2015). But, in an often-cited study regarding compensatory strategies adopted by people with a transtibial amputation during walking on level ground, subjects used prostheses (solid-ankle cushioned heel) that were not designed to restore push-off energy to the wearer (Winter and Sienko, 1988). These studies also included some subjects who had a transtibial amputation due to vascular disease (Winter and Sienko, 1988; Silverman et al., 2008). People who undergo a transtibial amputation due to vascular disease, as opposed to a traumatic or congenital amputation, typically require even higher metabolic energy to walk at the same speeds as non-amputees on level ground and have a slower preferred walking speed (Torburn et al., 1995). Higher metabolic cost and a slower preferred walking speed could also be attributed to the redistribution of positive push-off work from the ankle to the hip, similar to the redistribution of joint work in elderly populations (Franz and Kram, 2013). Though many studies have shown a 10–30% higher metabolic cost for people with either a traumatic or dysvascular transtibial amputation using a passive-elastic prosthesis during level-ground walking compared to non-amputees walking at the same speed (Torburn et al., 1995; Waters and Mulroy, 1999; Hsu et al., 2006), a recent study of young subjects (average age 29 years) found that people with a traumatic transtibial amputation using a passive-elastic ESAR prosthesis during level-ground walking do not have an increased metabolic cost compared to non-amputees over a range of speeds (0.74–1.68 m/s) (Russell Esposito et al., 2014). Furthermore, the biomechanical effects of using a passive-elastic ESAR prosthesis on level-ground walking step-to-step transition work are inconclusive. Herr and Grabowski (2012) found that use of a passive-elastic ESAR prosthesis resulted in significantly higher leading leg negative and significantly lower trailing leg positive step-to-step transition work during level-ground walking over a range of speeds (0.75–1.75 m/s) (Herr and Grabowski, 2012). However, Russell Esposito et al. (2016) found that use of a passive-elastic ESAR prosthesis did not significantly affect leading leg step-to-step transition work during level-ground walking compared to non-amputees. Thus, the metabolic and biomechanical effects of walking on level ground while using a passive-elastic ESAR prosthesis are unclear.
A commercially available powered ankle–foot prosthesis (BiOM) has been developed that contains a one degree of freedom ankle articulation (plantar- and dorsi-flexion) and generates battery-powered mechanical push-off work in late stance through series-elastic actuation. A state space controller, which is based on level-ground biological ankle work loops (moment vs. angle curve) during steady speed walking, is used to govern the response of the BiOM (Au et al., 2007) based on prosthetic ankle position (angle) from the encoder. To tune the response of the BiOM to the wearer, tuning parameters within the device are adjusted until the wearer’s net prosthetic ankle work is within 2 SDs of average non-amputee ankle work values (BionX Medical Technologies, Inc., 2016). Use of this powered ankle–foot prosthesis has normalized the metabolic costs and biomechanics (preferred walking speed, step-to-step transition work) during level-ground walking at speeds of 0.75–1.50 m/s for people with a transtibial amputation compared to non-amputees (Herr and Grabowski, 2012; Russell Esposito et al., 2016). However, to our knowledge, only three studies have investigated how use of a powered prosthesis affects the metabolic cost and biomechanics of uphill walking compared to use of a passive-elastic prosthesis. Use of the powered prosthesis normalized metabolic cost while walking on level ground and normalized trailing leg step-to-step transition work on both level ground and a 5° uphill ramp (Russell Esposito et al., 2016). Use of the powered compared to passive-elastic prosthesis reduced hamstring muscle activation on uphill slopes of +3°, +6°, and +9° (Pickle et al., 2017) and reduced the range of sagittal plane whole-body angular momentum on slopes of −10°, −5°, 0°, and +5° (Pickle et al., 2016). It remains unclear how use of a powered ankle–foot prosthesis affects leg joint work contributions during uphill and downhill walking over a range of slopes compared to use of a passive-elastic prosthesis. Use of a powered ankle–foot prosthesis that can restore leg joint biomechanics and work during uphill and downhill walking could normalize metabolic cost and improve the overall function of people with transtibial amputations.
Biomimetic mechatronic devices, such as prostheses, orthoses, and exoskeletons, have been designed to match non-amputee leg joint biomechanics in order to restore function in individuals with a physical impairment and augment function in unimpaired individuals (Zoss et al., 2005; Au et al., 2009; Cherelle et al., 2012; Collins et al., 2015). When non-amputees walk uphill at 10°, the hip and ankle work combine to provide 86 and 95% of leg positive and net leg work, respectively, and to walk downhill at −10° the muscles acting at the knee perform 58 and 81% of the negative and net leg work (DeVita et al., 2007). Furthermore, as non-amputees walk uphill at 21.3°, peak positive hip moment more than doubles, peak positive ankle moment increases 19%, and peak positive knee moment almost doubles, compared to walking on level ground (Lay et al., 2006). Conversely, when non-amputees walk downhill at −21.3°, peak positive hip moment remains constant, peak positive ankle moment decreases 44% and the magnitude of peak negative knee moment increases over fourfold compared to walking on level ground (Lay et al., 2006). It is not yet clear how people with a transtibial amputation using powered and passive-elastic prostheses adapt to walking on uphill and downhill slopes. It is likely that the design of future mechatronic devices such as powered ankle–foot prostheses should mimic healthy non-amputee gait, but the effects of current device designs for walking on various slopes for people with a leg amputation are unknown. This information would be useful for determining if prostheses can restore function to people with an amputation and/or the modifications needed to improve prosthetic design. Thus, we sought to determine the effects of using a passive and powered ankle–foot prosthesis on leg joint biomechanics of people with a transtibial amputation walking on level-ground and at a range of uphill and downhill slopes.
People with transtibial amputations using passive-elastic prostheses have increased UL and decreased AL step-to-step transition work compared to non-amputees, but have equivalent step-to-step transition work when using a powered ankle–foot prosthesis during level-ground and inclined walking. Thus, we hypothesized that UL total individual leg positive and net work would decrease, and AL total individual leg positive and net work would increase with use of the powered compared to passive-elastic prosthesis when walking at each uphill and downhill slope. Use of a passive-elastic prosthesis results in lower AL prosthetic ankle work, no change in AL knee range of motion, and increased UL and AL hip positive work compared to use of a powered ankle–foot prosthesis on level ground and on a 5° incline. Because a powered ankle–foot prosthesis provides push-off power and net positive work, we hypothesized that UL ankle and hip joint positive and net work would decrease, AL prosthetic ankle positive and net work would increase and hip joint positive and net work would decrease, and knee joint work would remain unchanged when walking at each slope while using a powered compared to passive-elastic prosthesis.
Materials and Methods
Subject Recruitment
Ten healthy adults with a traumatic unilateral transtibial amputation (6 M, 4 F, mean ± SD: age 42 ± 11 years, height 1.7 ± 0.08 m, and mass without a prosthesis 77.3 ± 14.8 kg) (Table 1) provided written informed consent according to the Declaration of Helsinki and US Department of Veterans Affairs institutional review board. Subjects self-reported that they were at a Medicare functional classification level of K3 or higher, and free of neurological, cardiovascular, and musculoskeletal disease other than that associated with a unilateral transtibial amputation.
Experimental Protocol
Tuning of the Powered Prosthesis
First, a certified prosthetist from BionX Medical Technologies aligned the powered prosthesis (BiOM T2, BionX Medical Technologies, Inc., Bedford, MA, USA) to each subject. We then placed reflective markers on subjects’ lower limbs according to a modified Helen Hayes marker set. We placed markers over joint centers and clusters of four markers over each segment. We also placed reflective markers on the AL at the approximate locations of the prosthetic foot 1st and 5th metatarsal heads, posterior calcaneus, and medial and lateral malleoli, matching the locations on the UL. We placed “malleoli” markers for the powered prosthesis on the encoder, which coincides with the center of rotation in the sagittal plane (Figure 1). Subjects then walked using the BiOM at 1.25 m/s on a dual-belt force-measuring treadmill (Bertec Corp., Columbus, OH, USA) for a series of 45-s trials at slopes of 0°, ±3°, ±6°, and ±9° while we simultaneously measured kinematics at 100 Hz (Vicon, Oxford, UK) and ground reaction forces (GRFs) at 1,000 Hz. We filtered GRFs using a fourth order recursive Butterworth filter with a 30 Hz cutoff and filtered kinematic data using a sixth-order recursive Butterworth filter with a 7 Hz cutoff in a custom Matlab (Mathworks, Natick, MA, USA) script. Perpendicular GRF data from each leg were used to determine ground contact with a 20 N threshold.
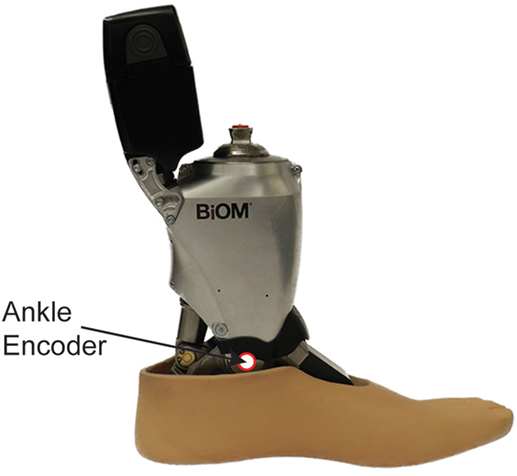
Figure 1. “Malleolus” marker placement on the encoder/center of rotation for the BiOM powered prosthesis.
To objectively tune the BiOM, we calculated prosthetic ankle angles, moments, powers, and net mechanical work normalized to body mass, including prosthetic mass using Visual 3D software (C-Motion, Germantown, MD, USA) after each 45-s trial and compared these data with averages from 20 non-amputees walking at the same speed and slopes (Jeffers et al., 2015). Similar to Ventura et al. (2011), we did not adjust the rigid segment model foot or shank in Visual 3D and used inertial properties inherent in the Visual 3D anatomical model due to the similar weight of the BiOM and an anatomical foot and shank (21.6 N). We then iteratively and systematically tuned the BiOM using a tablet with software provided by the manufacturer (BionX Medical Technologies, Bedford, MA, USA) until the lab-measured kinematic and kinetic data for AL prosthetic ankle range of motion, peak moment, peak power, and net work normalized to body mass matched the UL and non-amputee averages within 2 SDs at each slope (Figure 2). We manipulated tuning parameters including “Stiffness,” “Power Fast,” “Power Slow,” “Power Timing Fast,” “Power Timing Slow,” “Power Sensitivity,” “Stiffness Duration,” “Stance Dampening,” “Cadence Range,” and “Hard Stop Sensitivity.” We tuned the BiOM at each slope so that each subject replicated biological ankle biomechanics on that slope. We used the tuning parameters determined during up to two tuning sessions for each subject and each slope throughout the remainder of the experimental protocol.
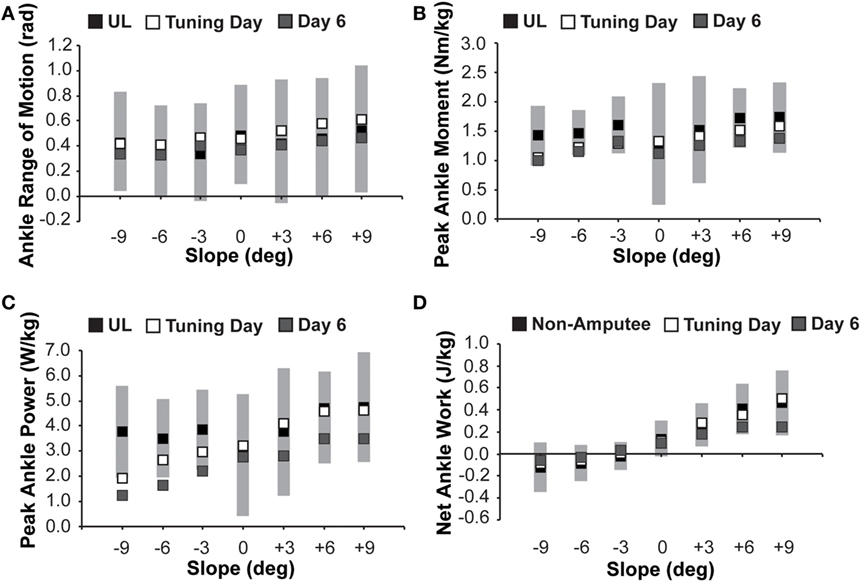
Figure 2. Prosthetic ankle (A) range of motion, (B) peak moment, (C) peak power, and (D) net work from the iterative BiOM tuning process for subjects walking at 1.25 m/s over a range of slopes. We tuned the BiOM to match average unaffected leg (UL) data (black) within 2 SDs (gray shaded area) for ankle (A) range of motion, (B) peak moment, and (C) peak power. We tuned the BiOM to match average data from non-amputees (black) within 2 SDs for ankle (D) net work. BiOM prosthetic ankle data (ensemble average) collected at the end of the tuning day (white) were numerically higher (closer to UL and non-amputee data) for most biomechanical variables at all slopes compared to the final day of the protocol (gray). We used data collected on Day 6 for analyses. In some cases, the symbols overlap.
Kinetic and Kinematic Data Collection
Subjects walked on the treadmill while using the powered prosthesis for approximately 10 h over five experimental sessions on separate days at the same speed and slopes prior to the session where we measured kinetic and kinematic data for analyses. All experimental sessions were separated by at least 22 h and no more than 2 weeks. The first two sessions were each 2–3 h long and the third through fifth sessions were each 1.5 h long. Then, during the sixth session (approximately 2.5 h long), we simultaneously measured kinematics at 100 Hz and GRFs at 1,000 Hz while subjects walked at 1.25 m/s on a dual-belt force-measuring treadmill (Bertec Corp., Columbus, OH, USA) at slopes of 0°, ±3°, ±6°, and ±9° using the powered prosthesis tuned for each slope and their own passive-elastic prosthesis. Each trial was approximately 1-min long and we randomized the trial order. We used the same marker set as described above and placed “malleoli” markers for the passive-elastic prosthesis on the medial and lateral edges of the carbon fiber prosthesis at the most dorsal point of the keel. We filtered GRFs using a fourth-order recursive Butterworth filter with a 30 Hz cutoff and filtered kinematic data using a sixth-order recursive Butterworth filter with a 7 Hz cutoff. Perpendicular GRF data from each leg were used to determine ground contact with a 20 N threshold. We calculated sagittal plane joint powers with Visual3D software (C-Motion, Germantown, MD, USA). Using a custom Matlab (MathWorks, Natick, MA, USA) script, we integrated joint power with respect to stride time to determine joint work over a stride (heel-strike to heel-strike of the same foot). We summed ankle, knee, and hip joint work over a stride to calculate leg work. We averaged at least five consecutive strides for each subject for each condition and calculated an ensemble average of all 10 subjects.
Statistical Analyses
Prior to choosing a statistical approach to determine the effects of speed, slope, and their interaction on individual leg and joint mechanics, we tested for linearity and normality of the data with RStudio statistical software (RStudio, Boston, MA, USA). We determined linearity by visually inspecting residuals and Q-Q plots in RStudio (Kim, 2015). Similarly, we determined normality by visually inspecting histograms in RStudio. The data were not linearly related but were normally distributed. Because our hypotheses are based on changes in leg or joint work at each slope and on the effects of using each prosthesis on leg and joint work, we used one-way repeated measures ANOVAs with prosthetic foot type (powered BiOM or passive-elastic ESAR) as the independent variable and leg or joint positive, negative or net work as the dependent variable with a significance level of 0.05 at each slope. We removed data outliers (total 260 of 3360 individual data points) from statistical analyses if they fell outside the first or third interquartile range (R Studio, Boston, MA, USA).
Results
During the tuning sessions, we were able to tune the powered prosthesis (BiOM) such that prosthetic ankle net work matched average non-amputee ankle net work within 2 SDs at all slopes (Figure 2). We were also able to match prosthetic ankle range of motion, peak moment, and peak power to the UL ankle averages within 2 SDs on all slopes (Figure 2). Similarly, on the final day of the protocol when we collected kinematic and kinetic data, the tuning established during the tuning sessions and used throughout the acclimation trials resulted in prosthetic ankle biomechanics that matched non-amputee average ankle net work within 2 SDs (Figure 2). Prosthetic ankle biomechanics also matched UL average ankle joint range of motion within 1 SD for all slopes, peak ankle moment within 2 SDs for all slopes, and peak ankle power within 2 SDs on level and all uphill slopes (Figure 2). While we matched the mechanics of the powered prosthesis to either the UL ankle or non-amputee ankle averages within 2 SDs on both the tuning and data collection days, when averaged across all slopes there was a numeric 20% decrease in ankle range of motion, 9% decrease in peak ankle moment, and 28% decrease in peak ankle power on the final day of the protocol compared to the tuning days. There was an average numeric 37% decrease in net ankle work done by the powered prosthesis on all slopes except at −3°, where the prosthetic ankle net work increased by almost fourfold from 0.007 to 0.033 J/kg on the final day of the protocol compared to the tuning days.
We found no effect of prosthetic foot type on UL positive, negative, or net work for all slopes (p > 0.05, Figure 3). We found no effect of prosthetic foot type on AL positive work (p > 0.05) or on AL negative work for all slopes (p < 0.001, Figure 3). There was an effect of prosthetic foot type on AL net work at uphill slopes of +6° and +9° (p < 0.05, Figure 3). AL net work was 146%, and 82% more positive on +6°, and +9° slopes, respectively, with use of the BiOM compared to ESAR prosthesis (Figure 3).
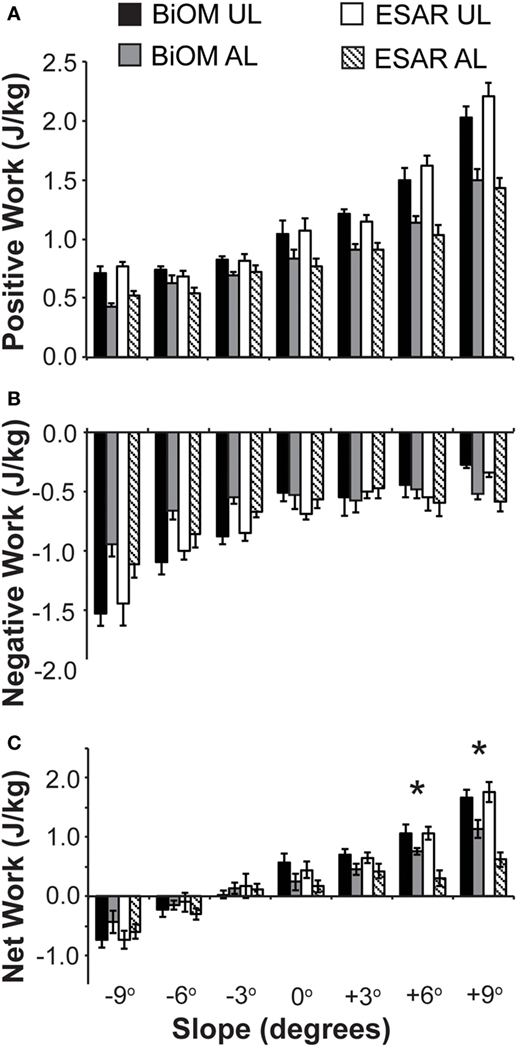
Figure 3. Unaffected leg (UL) and affected leg (AL) individual leg (A) positive, (B) negative, and (C) net work over an entire stride for subjects using the BiOM powered prosthesis (UL is black, AL is gray), and passive-elastic energy storage and return (ESAR) prosthesis (UL is white, AL is hashed) during walking at 1.25 m/s across a range of slopes. * indicates a significant difference in AL work between use of the BiOM powered and passive-elastic ESAR prosthesis.
We found no effect of prosthetic foot type on UL ankle or hip joint positive, negative, or net work for all slopes (p > 0.05, Figure 4). However, we did find an effect of prosthetic foot type on AL positive ankle work for uphill slopes of +6° and +9° (p < 0.05, Figure 4) and on AL net ankle work for all slopes (p ≤ 0.001, Figure 4). AL positive ankle work increased 89 and 55% at +6° and +9°, respectively, with use of the BiOM compared to ESAR prosthesis (Figure 4). In addition, at +3° there was a trend for AL positive ankle work to be 44% greater with use of the BiOM compared to ESAR prosthesis (p = 0.0575, Figure 4). AL net ankle work was greater (i.e., more positive) for all slopes with use of the BiOM compared to ESAR prosthesis. Specifically, at downhill slopes of −9°, −6°, and −3° AL net ankle work was 94, 109, and 155% more positive, respectively, with use of the BiOM compared to ESAR prosthesis (Figure 4). At 0°, +3°, +6°, and +9°, AL net ankle work increased 3.5-, 3.4-, 6.7-, and 9.4-fold, respectively, with use of the BiOM compared to ESAR prosthesis (Figure 4). In other words, the BiOM provided almost ten times as much net ankle work as an ESAR prosthesis at the steepest uphill slope of +9°. We also found a significant effect of prosthetic foot type on AL negative ankle work at −9°, −3°, +6°, and +9° (p < 0.05, Figure 4). Specifically, AL negative ankle work was 35–45% less negative with use of the BiOM compared to ESAR prosthesis (Figure 4). We did not find an effect of prosthetic foot type on AL hip joint positive, negative, or net work (p > 0.05, Figure 4). Similarly, we found no effect of prosthetic foot type on UL or AL knee joint positive, negative, or net work (p > 0.05, Figure 4).
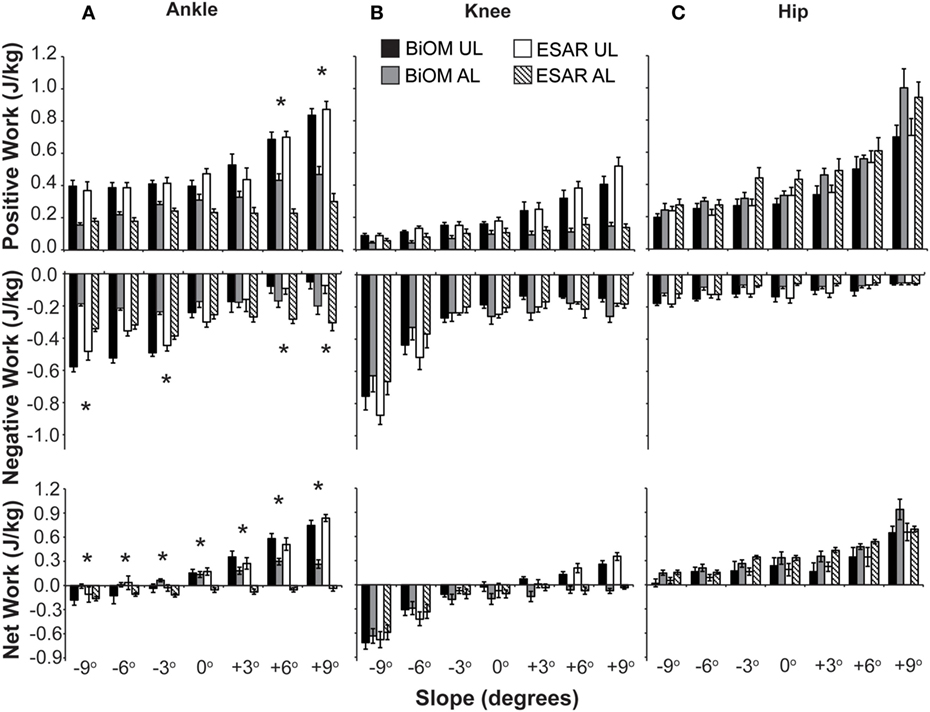
Figure 4. (A) Ankle, (B) knee, and (C) hip positive (top), negative (middle), and net (bottom) work over an entire stride for subjects using the BiOM powered prosthesis [unaffected leg (UL) is black, affected leg (AL) is gray], and passive-elastic energy storage and return (ESAR) prosthesis (UL is white and AL is hashed) during walking at 1.25 m/s across a range of slopes. * indicates a significant difference in AL work between use of the BiOM powered and passive-elastic ESAR prosthesis.
Discussion
In contrast to our hypothesis, which was based on results from level-ground and inclined walking, there were no changes in UL individual leg net work when subjects used the BiOM compared to their own ESAR prosthesis when walking on any slope. These results are in contrast with previous studies (Herr and Grabowski, 2012; Russell Esposito et al., 2016) that found leading (unaffected) leg step-to-step transition work was normalized with use of the BiOM powered ankle–foot prosthesis compared to an ESAR prosthesis on both level ground and a 5° incline. However, and in partial support of our hypothesis, AL individual leg net work was more positive (i.e., increased) at uphill slopes of +6° and +9° with use of the BiOM compared to ESAR prosthesis; but we found no effect of prosthetic foot type on individual leg total positive work. This increase in AL individual leg net work with use of the BiOM powered prosthesis compared to an ESAR prosthesis is due to an increase in AL prosthetic ankle positive work and AL prosthetic ankle net work, which is in partial support of our hypothesis. Our hypotheses were based on level-ground walking studies; however, the BiOM state space controller is based on a biological ankle work loop for level-ground walking and provides net positive prosthetic ankle work on all slopes. Thus, it is possible that the BiOM is not optimized for walking up and down slopes. It is also possible that at moderate uphill and all downhill slopes, the substantial increase in AL prosthetic ankle positive and net work with use of the BiOM was absorbed at the knee or within the socket–limb interface and, thus, did not translate into significantly higher AL individual leg net work. Or, it is possible that the increased prosthetic push-off work provided by the BiOM was not enough for the user to overcome the compensation strategy typically adopted by individuals with a transtibial amputation using passive-elastic prostheses (Silverman et al., 2008; Adamczyk and Kuo, 2015) or that our subjects did not utilize the compensation strategy at all – as evidenced by no change in hip work, though AL prosthetic ankle positive and net work increased with use of the BiOM. Specifically, and in contrast to our hypotheses, there were no differences in hip or knee joint positive or net work for either leg. By contrast, previous studies found an increase in both UL work and AL hip work during level-ground walking with a passive prosthesis compared to non-amputees (Winter and Sienko, 1988; Silverman et al., 2008; Adamczyk and Kuo, 2015). The type of prosthesis may affect compensation strategies, however, the type of ESAR prosthesis used by subjects in Silverman et al. and Adamczyk and Kuo is not clear (Silverman et al., 2008; Adamczyk and Kuo, 2015), and Winter and Sienko (1988) had subjects use solid-ankle cushioned heel prostheses. Furthermore, these studies included some subjects who underwent a leg amputation due to vascular disease (Winter and Sienko, 1988; Silverman et al., 2008). These people typically have a slower preferred walking speed than those who have a congenital or traumatic amputation (Torburn et al., 1995), which could have exacerbated any compensation strategy when walking at the same speed—faster than preferred speed—as people with a traumatic or congenital leg amputation using a passive-elastic prosthesis.
Another possible explanation for the increase in AL and prosthetic ankle net work, but no change in UL or AL hip work with use of the powered compared to a passive-elastic prosthesis, could be that the provided prosthetic ankle push-off work is similar to the work provided by the uni-articular soleus, rather than the bi-articular gastrocnemius. An ankle–foot prosthesis does not span the knee and thus is uni-articular. Neptune et al. used musculoskeletal modeling and predicted that horizontal trunk propulsion/acceleration is primarily provided by the soleus and rectus femoris during late stance in non-amputees walking on level ground (Neptune et al., 2008). However, in experimental studies of non-amputees that measured muscle activation of the plantar-flexors during level-ground walking, ankle push-off work was primarily due to medial gastrocnemius activation, while the soleus played a small role in providing push-off work (Gottschall and Kram, 2003; Franz and Kram, 2013). Therefore, it is possible that a powered ankle–foot prosthesis that can only replace the function of the uni-articular soleus is incapable of fully replicating biological ankle function during walking. Furthermore, musculoskeletal modeling studies have found that the use of powered or passive-elastic prostheses increases whole-body sagittal plane angular momentum compared to non-amputees and that neither device is capable of providing power to the trunk similar to the biological gastrocnemius (Pickle et al., 2016, 2017). Future studies should investigate the mechanical energy loss and transfer from the prosthetic ankle to the residual limb, and investigate the role of the socket–limb interface in this energy transfer. Future prosthetic designs may need to incorporate a connection that crosses the knee joint to improve the energy transfer to and from the prosthesis in order to potentially normalize biomechanics (Endo et al., 2009).
We integrated sagittal plane joint power with respect to time to determine joint work. Joint power, and thus the net work done at the ankle, knee, and hip, was more positive when walking uphill and more negative when walking downhill when subjects used either prosthesis (Figure 4). Similar to non-amputees (Lay et al., 2006; DeVita et al., 2007), hip and ankle power became more positive with steeper uphill slopes (Figures S1 and S2 in Supplementary Material) and knee power became more negative with steeper downhill slopes (Figure S3 in Supplementary Material) when subjects with a transtibial amputation used either prosthesis. In support of our hypothesis, UL and AL sagittal plane knee joint work remained unchanged with use of the BiOM compared to an ESAR prosthesis. This result is in line with Postema et al. (1997) and Winter and Sienko (1988), who found no difference in sagittal plane AL knee range of motion or power during level-ground walking at a self-selected walking velocity with the use of an ESAR compared to conventional solid-ankle cushioned heel prosthesis. Furthermore, and similar to Winter and Sienko (1988), subjects exhibited little to no knee power in the first half of stance (Figure S3 in Supplementary Material), unlike non-amputees.
We iteratively tuned the BiOM prosthesis to match the average biological ankle sagittal plane range of motion, peak moment, peak power, and net work from 20 non-amputees at each slope (Jeffers et al., 2015). Though subjects used the same tuning parameters, prosthetic components, and alignment established in the tuning sessions for all experimental sessions, they likely modified the way that they walked while using the BiOM during the final experimental session compared to the tuning sessions (Figure 2). It is possible that after acclimation to walking while using the powered prosthesis, the tuning parameters should be further adjusted as the user modifies his or her gait. Previous studies that have analyzed the use of the BiOM prosthesis on level ground or up a 5° incline were completed in fewer experimental sessions (1–3 sessions in previous studies vs. 6 sessions for the present study), used over-ground measurements and relied on data collected from the BiOM’s on-board microprocessor (Herr and Grabowski, 2012; Russell Esposito et al., 2016), rather than from independent treadmill-based motion capture and GRF data. Furthermore, these previous studies used force plates mounted in a walkway to collect GRF data from only a few steps (Herr and Grabowski, 2012; Russell Esposito et al., 2016) and, thus, differences could exist between our data, which measured GRFs from multiple consecutive steps at a set speed, and these studies. The differences in tuning strategies and protocol length may also potentially explain the differences in our results compared to others. Based on our ankle joint mechanics data (Figures 2 and 4), subjects were able to match the BiOM prosthetic ankle net work to within 2 SDs of average biological ankle values during tuning and on the final day of our protocol, though prosthetic ankle net work numerically decreased by an average of 37% on all slopes except −3°. Thus, it is possible that tuning on the first day of a longer protocol and using the same tuning strategy throughout could affect prosthetic ankle mechanical power output, net work or range of motion. Future studies are needed to better understand the interaction of the user and the prosthesis during acclimation and the effects of different tuning strategies. Future studies may also be needed to measure the effects of matching the response of the BiOM to within 1 SD of the average of non-amputees.
Despite the differences in prosthetic ankle biomechanics between the tuning days and the final day of the protocol, we found a significant increase in AL prosthetic net work for all slopes and a significant increase in AL prosthetic positive work at uphill slopes of +6° and +9° with use of the BiOM compared to ESAR prosthesis. Based on previous studies of individuals with impaired or no ankle function (Winter and Sienko, 1988; Powers et al., 1994; Silverman et al., 2008; Collins and Kuo, 2010; Adamczyk and Kuo, 2015), a reduction in ankle push-off work is related to slower preferred walking speed, increased kinematic and kinetic asymmetry, increased metabolic energy expenditure, and increased AL hip positive work production. Thus, by increasing AL prosthetic ankle positive and net work, use of a powered ankle–foot prosthesis may increase preferred walking speed, improve kinematic and kinetic symmetry between legs, decrease metabolic demand, and decrease reliance on the muscular work performed by the AL hip joint and UL on uphill and downhill slopes for people with a transtibial amputation. The changes in leg joint mechanics when using a powered compared with an ESAR prosthesis during walking over a range of slopes may, therefore, result in improved functional mobility and, thus, quality of life, specifically when navigating uphill slopes (Burger and Marincek, 1997; Ehde et al., 2001; Ephraim et al., 2005).
Use of a powered prosthesis decreases frontal plane knee moments, which have been associated with knee osteoarthritis, in the UL during walking on level ground compared to use of an ESAR prosthesis (Morgenroth et al., 2011; Grabowski and D’Andrea, 2013). Furthermore, use of a powered prosthesis decreased sagittal plane angular momentum on a range of slopes compared to an ESAR prosthesis (Pickle et al., 2016). We calculated sagittal plane knee joint power but did not include contributions to or changes in frontal or transverse plane powers. It is possible that the reduction in frontal plane knee moments is still observed when using the powered prosthesis to walk uphill and downhill. In future studies, we intend to investigate frontal plane joint moments when people with a unilateral transtibial amputation walk uphill and downhill using passive-elastic and powered prostheses.
We normalized all values to each subjects’ mass with the respective prosthesis and there was an average 1.6 kg increase in body mass when wearing the BiOM compared to wearing their own ESAR prosthesis. While Mattes et al. (2000) found that adding mass to an ESAR prosthesis—until the total mass and inertia matched the intact limb, similar to the powered prosthesis (Herr and Grabowski, 2012)—resulted in greater gross metabolic power of approximately 21 W per 1 kg added, it is unclear how the combination of prosthetic ankle power and added distal mass relative to the residual limb change joint work contributions or individual leg work. Finally, while we attempted to match AL sagittal plane prosthetic ankle biomechanics to UL sagittal plane ankle biomechanics by adjusting tuning parameters in the BiOM powered prosthesis, prosthetic ankle range of motion, peak moment, peak power, and net work were 20–40% numerically lower on the final day of data collection than during the tuning sessions. Future studies are planned to investigate the effects of systematically varying powered ankle–foot prosthetic tuning parameters on the biomechanics of level-ground and sloped walking to determine the effects of tuning and appropriate acclimation times. Appropriate acclimation could influence the way current prostheses are tuned to an individual patient in the clinical setting and, thus, the efficacy of using a powered ankle–foot prosthesis during daily activities.
Conclusion
Previous studies of people with a transtibial amputation using passive-elastic and powered prostheses have primarily focused on level-ground walking. We found that with use of the BiOM powered compared to a passive-elastic ESAR prosthesis, AL sagittal plane prosthetic ankle positive work increased for uphill slopes of +6° and +9° and prosthetic ankle net work increased for all slopes (−9° to +9°). Similarly, with use of the BiOM powered compared to a passive-elastic prosthesis, AL net work increased for uphill slopes of +6° and +9°. There were no differences in AL knee or hip positive or net work nor did unaffected joint or leg work change when using the BiOM powered compared to a passive-elastic prosthesis. Greater prosthetic ankle positive and net work through use of a powered prosthesis could improve kinematic and kinetic symmetry between the legs of people with a transtibial amputation during walking on slopes and, thus, improve preferred walking speed, metabolic cost, functional mobility, and quality of life.
Ethics Statement
This study was carried out in accordance with the recommendations of Ethical Principles and Guidelines for the Protection of Human Subjects of Research, Colorado Multiple Institutional Review Board with written informed consent from all subjects. All subjects gave written informed consent in accordance with the Declaration of Helsinki. The protocol was approved by the Colorado Multiple Institutional Review Board.
Author Contributions
AG conceived of the experiment, and AG and JJ designed the experimental protocol. JJ recruited subjects, collected and analyzed data, and wrote custom code. AG analyzed data, reviewed, and edited the manuscript. All authors contributed to the writing of the manuscript.
Conflict of Interest Statement
The authors declare that the research was conducted in the absence of any commercial or financial relationships that could be construed as a potential conflict of interest.
Funding
This work was supported by a Career Development Award #A7972-W from the United States (U.S.) Department of Veterans Affairs Rehabilitation Research and Development Service. The contents do not represent the views of the U.S. Department of Veterans Affairs or the United States Government. NCT01784003.
Supplementary Material
The Supplementary Material for this article can be found online at http://www.frontiersin.org/articles/10.3389/frobt.2017.00072/full#supplementary-material.
Figure S1. Affected leg (AL) and unaffected leg (UL) hip power (ensemble average) during the stance phase for subjects using a BiOM powered prosthesis (black) and their own passive-elastic energy storage and return (ESAR) prosthesis (gray) during walking at 1.25 m/s on uphill slopes (A–C), level ground (D), and downhill slopes (E–G). Solid lines represent subjects’ UL data and dashed lines represent subjects’ AL data. This figure is for visualization of joint power over a stride, which we integrated to calculate joint work.
Figure S2. Affected leg (AL) prosthetic and unaffected leg (UL) ankle power (ensemble average) during the stance phase for subjects using a BiOM powered prosthesis (black) and their own passive-elastic energy storage and return (ESAR) prosthesis (gray) during walking at 1.25 m/s on uphill slopes (A–C), level ground (D), and downhill slopes (E–G). Solid lines represent subjects’ UL data and dashed lines represent subjects’ AL data. This figure is for visualization of joint power over a stride, which we integrated to calculate joint work.
Figure S3. Affected leg (AL) and unaffected leg (UL) knee power during the stance phase (ensemble average) for subjects using a BiOM powered prosthesis (black) and their own passive-elastic energy storage and return (ESAR) prosthesis (gray) during walking at 1.25 m/s on uphill slopes (A–C), level ground (D), and downhill slopes (E–G). Solid lines represent subjects’ UL data and dashed lines represent subjects’ AL data. This figure is for visualization of joint power over a stride, which we integrated to calculate joint work.
References
Adamczyk, P. G., and Kuo, A. D. (2015). Mechanisms of gait asymmetry due to push-off deficiency in unilateral amputees. IEEE Trans. Neural Syst. Rehabil. Eng. 23, 776–785. doi: 10.1109/TNSRE.2014.2356722
Au, S. K., Herr, H. M., Weber, J., and Martinez-Villalpando, E. C. (2007). “Powered ankle-foot prosthesis for the improvement of amputee ambulation,” in 29th Annual International Conference of the IEEE EMBS (Lyon, France: Cite Internationale).
Au, S. K., Weber, J., and Herr, H. (2009). Powered ankle-foot prosthesis improves walking metabolic economy. IEEE Trans. Robot. 25, 51–66. doi:10.1109/TRO.2008.2008747
BionX Medical Technologies, Inc. (2016). BiOM T2 Ankle Instructions for Use. Available at: http://www.bionxmed.com/biom-ankle-resources/
Burger, H., and Marincek, C. (1997). The life style of young persons after lower limb amputation caused by injury. Prosthet. Orthot. Int. 21, 35–39.
Cherelle, P., Arnout, M., Grosu, V., Vanderborght, B., and Lefeber, D. (2012). The AMP-Foot 2.0: Mimicking Intact Ankle Behavior with a Powered Transtibial Prosthesis. Brussel: Vrije Universiteit Brussel.
Collins, S. H., and Kuo, A. D. (2010). Recycling energy to restore impaired ankle function during human walking. PLoS ONE 5:e9307. doi:10.1371/journal.pone.0009307
Collins, S. H., Wiggin, M. B., and Sawicki, G. S. (2015). Reducing the energy cost of human walking using an unpowered exoskeleton. Nature 522, 212–215. doi:10.1038/nature14288
DeVita, P., Helseth, J., and Hortobagyi, T. (2007). Muscles do more positive than negative work in human locomotion. J. Exp. Biol. 210(Pt 19), 3361–3373. doi:10.1242/jeb.003970
Ehde, D. M., Smith, D. G., Czerniecki, J. M., Campbell, K. M., Malchow, D. M., and Robinson, R. L. R. (2001). Back pain as a secondary disability in persons with lower limb amputations. Arch. Phys. Med. Rehabil. 82, 731–734. doi:10.1053/apmr.2001.21962
Endo, K., Swart, E., and Herr, H. (2009). “An artificial gastrocnemius for a transtibial prosthesis,” in Engineering in Medicine and Biology Society. EBMC 2009. Annual International Conference of the IEEE (Minneapolis, MN).
Ephraim, P. L., Wegener, S. T., MacKenzie, E. J., Dillingham, T. R., and Pezzin, L. E. (2005). Phantom pain, residual limb pain, and back pain in amputees: results of a national survey. Arch. Phys. Med. Rehabil. 86, 1910–1919. doi:10.1016/j.apmr.2005.03.031
Franz, J. R., and Kram, R. (2013). Advanced age affects the individual leg mechanics of level, uphill, and downhill walking. J. Biomech. 46, 535–540. doi:10.1016/j.jbiomech.2012.09.032
Gottschall, J. S., and Kram, R. (2003). Energy cost and muscular activity required for propulsion during walking. J. Appl. Physiol. 94, 1766–1772. doi:10.1152/japplphysiol.00670.2002
Grabowski, A. M., and D’Andrea, S. (2013). Effects of a powered ankle-foot prosthesis on kinetic loading of the unaffected leg during level-ground walking. J. Neuroeng. Rehabil. 10, 49. doi:10.1186/1743-0003-10-49
Herr, H. M., and Grabowski, A. M. (2012). Bionic ankle-foot prosthesis normalizes walking gait for persons with leg amputation. Proc. Biol. Sci. 279, 457–464. doi:10.1098/rspb.2011.1194
Hsu, M. J., Nielsen, D. H., Lin-Chan, S. J., and Shurr, D. (2006). The effects of prosthetic foot design on physiologic measurements, self-selected walking velocity, and physical activity in people with transtibial amputation. Arch. Phys. Med. Rehabil. 87, 123–129. doi:10.1016/j.apmr.2005.07.310
Isakov, E., Keren, O., and Benjuya, N. (2000). Trans-tibial amputee gait: time-distance parameters and EMG activity. Prosthet. Orthot. Int. 24, 216–220. doi:10.1080/03093640008726550
Jeffers, J. R., Auyang, A. G., and Grabowski, A. M. (2015). The correlation between metabolic and individual leg mechanical power during walking at different slopes and velocities. J. Biomech. 48, 2919–2924. doi:10.1016/j.jbiomech.2015.04.023
Kim, B. (2015). Understanding Diagnostic Plots for Linear Regression Analysis. University of Virginia. Available at: http://data.library.virginia.edu/diagnostic-plots/
Lay, A. N., Hass, C. J., and Gregor, R. J. (2006). The effects of sloped surfaces on locomotion: a kinematic and kinetic analysis. J. Biomech. 39, 1621–1628. doi:10.1016/j.jbiomech.2005.05.005
Mattes, S. J., Martin, P. E., and Royer, T. D. (2000). Walking symmetry and energy cost in persons with unilateral transtibial amputations: matching prosthetic and intact limb inertial properties. Arch. Phys. Med. Rehabil. 81, 561–568. doi:10.1016/S0003-9993(00)90035-2
Morgenroth, D. C., Segal, A. D., Zelik, K. E., Czerniecki, J. M., Klute, G. K., Adamczyk, P. G., et al. (2011). The effect of prosthetic foot push-off on mechanical loading associated with knee osteoarthritis in lower extremity amputees. Gait Posture 34, 502–507. doi:10.1016/j.gaitpost.2011.07.001
Neptune, R. R., Sasaki, K., and Kautz, S. A. (2008). The effect of walking speed on muscle function and mechanical energetics. Gait Posture 28, 135–143. doi:10.1016/j.gaitpost.2007.11.004
Pickle, N. T., Grabowski, A. M., Jeffers, J. R., and Silverman, A. K. (2017). The functional roles of muscles, passive prostheses, and powered prostheses during sloped walking in people with a transtibial amputation. J. Biomech. Eng. 139, 111005–111011. doi:10.1115/1.4037938
Pickle, N. T., Wilken, J. M., Aldridge Whitehead, J. M., and Silverman, A. K. (2016). Whole-body angular momentum during sloped walking using passive and powered lower-limb prostheses. J. Biomech. 49, 3397–3406. doi:10.1016/j.jbiomech.2016.09.010
Postema, K., Hermens, H. J., De Vries, J., Koopman, H. F. J. M., and Eisma, W. H. (1997). Energy storage and release of prosthetic feet part 1: biomechanical analysis related to user benefits. Prosthet. Orthot. Int. 21, 17–27.
Powers, C. M., Torburn, L., Perry, J., and Ayyappa, E. (1994). Influence of prosthetic foot design on sound limb loading in adults with unilateral below-knee amputations. Arch. Phys. Med. Rehabil. 75, 825–829.
Russell Esposito, E., Aldridge Whitehead, J. M., and Wilken, J. M. (2016). Step-to-step transition work during level and inclined walking using passive and powered ankle-foot prostheses. Prosthet. Orthot. Int. 40, 311–319. doi:10.1177/0309364614564021
Russell Esposito, E., Rodriguez, K. M., Rabago, C. A., and Wilken, J. M. (2014). Does unilateral transtibial amputation lead to greater metabolic demand during walking? J. Rehabil. Res. Dev. 51, 1287–1296. doi:10.1682/JRRD.2014.06.0141
Silverman, A. K., Fey, N. P., Portillo, A., Walden, J. G., Bosker, G., and Neptune, R. R. (2008). Compensatory mechanisms in below-knee amputee gait in response to increasing steady-state walking speeds. Gait Posture 28, 602–609. doi:10.1016/j.gaitpost.2008.04.005
Torburn, L., Powers, C. M., Robert, G., and Perry, J. (1995). Energy expenditure during ambulation in dysvascular and traumatic below-knee amputees: a comparison of five prosthetic feet. J. Rehabil. Res. Dev. 32, 111–119.
Ventura, J. D., Klute, G. K., and Neptune, R. R. (2011). The effect of prosthetic ankle energy storage and return properties on muscle activity in below-knee amputee walking. Gait Posture 33, 220–226. doi:10.1016/j.gaitpost.2010.11.009
Waters, R. L., and Mulroy, S. (1999). The energy expenditure of normal and pathologic gait. Gait Posture 9, 207–231. doi:10.1016/S0966-6362(99)00009-0
Winter, D. A., and Sienko, S. E. (1988). Biomechanics of below-knee amputee gait. J. Biomech. 21, 361–367. doi:10.1016/0021-9290(88)90142-X
Zmitrewicz, R. J., Neptune, R. R., Walden, J. G., Rogers, W. E., and Bosker, G. W. (2006). The effect of foot and ankle prosthetic components on braking and propulsive impulses during transtibial amputee gait. Arch. Phys. Med. Rehabil. 87, 1334–1339. doi:10.1016/j.apmr.2006.06.013
Keywords: joint work, individual leg work, amputee, uphill walking, downhill walking
Citation: Jeffers JR and Grabowski AM (2017) Individual Leg and Joint Work during Sloped Walking for People with a Transtibial Amputation Using Passive and Powered Prostheses. Front. Robot. AI 4:72. doi: 10.3389/frobt.2017.00072
Received: 29 September 2017; Accepted: 11 December 2017;
Published: 22 December 2017
Edited by:
Monica A. Daley, Royal Veterinary College, United KingdomReviewed by:
Alexandra Voloshina, Stanford University, United StatesKiisa Nishikawa, Northern Arizona University, United States
Copyright: © 2017 Jeffers and Grabowski. This is an open-access article distributed under the terms of the Creative Commons Attribution License (CC BY). The use, distribution or reproduction in other forums is permitted, provided the original author(s) or licensor are credited and that the original publication in this journal is cited, in accordance with accepted academic practice. No use, distribution or reproduction is permitted which does not comply with these terms.
*Correspondence: Jana R. Jeffers, amFuYS5qZWZmZXJzQGNvbG9yYWRvLmVkdQ==