System integration of magnetic medical microrobots: from design to control
- 1State Key Laboratory of Robotics, Shenyang Institute of Automation, Chinese Academy of Sciences, Shenyang, China
- 2Institutes for Robotics and Intelligent Manufacturing, Chinese Academy of Sciences, Shenyang, China
- 3University of Chinese Academy of Sciences, Beijing, China
- 4College of Information and Electrical Engineering, Shenyang Agricultural University, Shenyang, China
Magnetic microrobots are ideal for medical applications owing to their deep tissue penetration, precise control, and flexible movement. After decades of development, various magnetic microrobots have been used to achieve medical functions such as targeted delivery, cell manipulation, and minimally invasive surgery. This review introduces the research status and latest progress in the design and control systems of magnetic medical microrobots from a system integration perspective and summarizes the advantages and limitations of the research to provide a reference for developers. Finally, the future development direction of magnetic medical microrobot design and control systems are discussed.
1 Introduction
Since the concept of medical microrobots was first proposed in Feynman’s lecture in 1959, research on microrobots has increased. In the research, it was discovered that magnetic microrobots offer superior benefits for medical applications, such as penetration of magnetic fields, and harmlessness to the body, and can remotely control microrobots. Several studies have investigated the properties and selection of materials (Soto et al., 2022), others have focused on developing structures and manufacturing methods (Rajabasadi et al., 2021; Wang Z. B. et al., 2022d; Wang Z. H. et al., 2022e), and others aspire to study motion control (Yang and Li, 2020; Wang X. et al., 2022c; Jiang et al., 2022). Most of the studies focus on one part of the magnetic medical microrobots, but there are few review articles on integrating the entire system, including how to design magnetic medical microbots with specific properties and how to precisely control them to accomplish a specific medical task.
The design and control systems of magnetic medical microrobots are interrelated. Therefore, we must combine the two parts from a system integration perspective. This review focuses on the elements of magnetic medical microrobot design and control system integration to provide a reference for researchers to design magnetic medical microrobots for successful application in vivo.
In Section 2 the materials are classified, the functions and characteristics of the various materials are discussed, and the three main structures of magnetic medical microrobots (microhelicals, microspheres, and microscaffolds), as well as their manufacturing technology, are introduced. In Section 3 we first classify and summarize the magnetic actuation modules and then introduce mainstream imaging methods. Next, we divide the control methods into three types: open-loop, closed-loop, and autonomous controls, and discuss the advantages and disadvantages of the three control methods. Finally, in the conclusion and outlook section, future directions for the development of magnetic medical microrobots are proposed.
2 Design system of magnetic medical microrobots
The first step in the design process of magnetic medical microrobots is selecting the mechanism of movement, material, structure, and manufacturing method. Currently, research on the mechanism of movement is abundant (Dreyfus et al., 2005; Field et al., 2019; Zhou et al., 2021; Wang Z. B. et al., 2022d), therefore, it will not be introduced in this review. This section introduces the latest progress in materials, the three structures, and manufacturing methods.
2.1 Material
According to their usefulness, the materials that constitute a magnetic microrobot, are mainly divided into three categories: functional materials, scaffold materials, and materials that not only serve as scaffolds but also have the required functions (Zhu et al., 2022).
Functional materials are materials that can respond to external field stimuli. Magnetic materials are representative of functional materials. Magnetic materials are divided into hard and soft magnetic materials. Hard magnetic materials with their high remanence and coercivity, offer efficient propulsion for microrobots in weak magnetic fields. However, most hard magnetic materials have poor biocompatibility, such as Ni/Co/NdFeB (Bernasconi et al., 2018; Li et al., 2018; Goudu et al., 2020). A few hard magnetic materials have good biocompatibility, such as FePt (Bozuyuk et al., 2022; Dogan et al., 2022). Soft magnetic materials, such as Fe and superparamagnetic iron oxide nanoparticles (SPIONs) (i.e., Fe3O4, γ-Fe2O3) (Park et al., 2019; Noh et al., 2022), often have good biocompatibility and biodegradability. However, they cannot provide an effective propulsion force in a weak magnetic field. Magnetic microrobots are often generated by the integration of magnetic materials, which are functional materials, into three-dimensional structures composed of scaffold materials. The integration methods include (a) a magnetic layer on the surface of microrobots (Mayorga-Martinez et al., 2022), (b) nanoparticles in the form of a scaffold (Giltinan et al., 2021), and (c) hybridization in polymers such as hydrogels.
Scaffold materials are materials that are used to create the main body (three-dimensional structure) of a magnetic medical microrobot. Biocompatibility and biodegradability are the first factors considered in selecting the scaffold material. SiO2 is often used to manufacture the main body of spherical microrobots (Bozuyuk et al., 2021). Photoresists and photosensitive resins, such as SU-8, are also used to manufacture microrobots with three-dimensional structures; however, they are difficult to degrade in vivo. The new generation of microrobots is manufactured with polymers, such as poly (lactic-co-glycolic acid) (PLGA) (Kim et al., 2018), pentaerythritol triacrylate (PETA) (Lee et al., 2023) and poly (ethylene glycol) diacrylate (PEGDA) (Chen et al., 2023). Cells and microbes can also be used as scaffold materials (Alapan et al., 2018).
The third category of materials is the intersection of functional and scaffolding materials. For example, (Alcântara et al., 2019) fabricated fully iron magnetic microrobots, Iron is a magnetic material and constitutes the main body of this magnetic microrobot, which means that the magnetic material that serves as a scaffold material belongs to the third class of materials. The smart hydrogel also belongs to the third material. While serving as the body of a magnetic microrobot, it can also change shape or volume in response to external field stimuli to accomplish complex medical tasks (Fusco et al., 2015; Lee et al., 2021; Xin et al., 2021). Chitosan (CHI) (Maria-Hormigos et al., 2023) and poly (N-isopropyl acrylamide) (PNIPAM), are the main representatives.
We customized the magnetic microrobots by selecting materials that correspond to the desired performance requirements. For example, if a magnetic material is integrated into a three-dimensional structure fabricated from a smart hydrogel, we will obtain a magnetic soft microrobot with deformation capabilities.
2.2 Structure and manufacturing method
The manufacturing technology for microhelical structures is mature, and includes glancing angle deposition (GLAD) (Walker et al., 2015), template-assisted electroless deposition (TAED) (Zeeshan et al., 2013; Bernasconi et al., 2022), bio-templated methods (Yan et al., 2017), and direct laser writing (DLW) (Ceylan et al., 2019) (Figures 1A–D). Microhelical microrobots are propelled by rotating magnetic fields and can achieve three-dimensional motion in liquid environments, and (Xu et al., 2022) ensure the accuracy of 3D motion by compensating for angles.
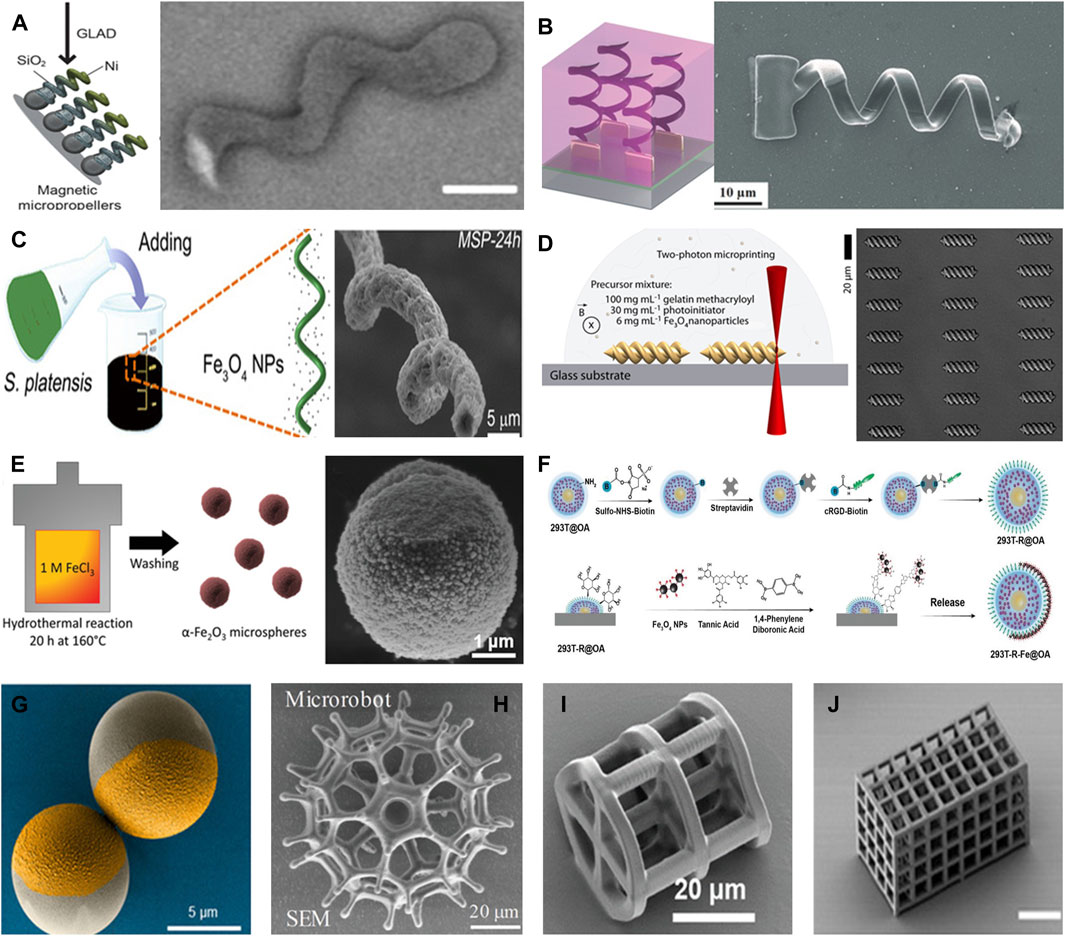
FIGURE 1. Structures and manufacturing technology. (A) GLAD technology. Adapted from Walker et al. (2015). Copyright 2015 The Authors under the CC-BY 4.0 license. (B) Template-assisted electroless deposition technology. Adapted from Zeeshan et al. (2013). Copyright 2013 John Wiley and Sons, Inc. (C) Bio-templated method technology. Adapted from Yan et al. (2017). Copyright 2017 AAAS. (D) DLW technology. Adapted from Ceylan et al. (2019). Copyright 2019 The Authors under the CC-BY 4.0 license. (E) α-Fe2O3-based microspheres. Adapted from Urso et al. (2021). Copyright 2021 John Wiley and Sons, Inc. (F) Cell-based microsphere. Adapted from Cong et al. (2022). Copyright 2022 John Wiley and Sons, Inc. (G) SiO2-based microspheres. Reprinted from Alapan et al. (2020). Copyright 2020 AAAS. (H) Porous spherical microrobot. Reprinted from Wei et al. (2020). Copyright 2020 John Wiley and Sons, Inc. (I) Porous cylindrical microrobot. Reprinted from Gyak et al. (2019). Copyright 2019 John Wiley and Sons, Inc. (J) Porous rectangular microrobot. Reprinted from Jeon et al. (2019). Copyright 2019 AAAS.
Because of their excellent motion ability and simple dynamics model (Wu et al., 2022), microsphere microrobots have been used by many researchers in the study of motion control and targeted delivery of microrobots. The motion model of the microsphere is similar to that of the vehicle, and the motion control method of the vehicle can also be applied to the microsphere. Researchers commonly use iron oxide (Urso et al., 2021), living cells (Cong et al., 2022), and SiO2 (Alapan et al., 2020) (Figures 1E–G) as the main bodies of microspheres. Microspheres can often be manufactured by hydrothermal reactions, emulsion methods, and drop casting methods, and magnetic materials are subsequently attached to the surface of the microsphere by magnetron sputtering to generate propulsion capability.
The structure of a microscaffold is complex; therefore, it is typically manufactured using DLW technology. (Wei et al., 2020). used DLW technology to create a porous spherical microrobot that could transport engineered stem cells to the desired location for targeted therapy (Figure 1H). Similarly, cylindrical (Gyak et al., 2019), rectangular (Jeon et al., 2019) (Figures 1I, J), and other porous scaffold structures have been manufactured.
Microhelices and microscaffolds are often used for targeted drug/cell transport owing to their strong loading capacities (Dong et al., 2020). However, the structure of the two is complex, and it is difficult to further reduce its size. The advantages of microspheres are that they are simple to manufacture and can be submicron in size to reach complex, narrow areas of deep tissue. Simultaneously, because the movement area is mainly the blood vessel wall, it is less affected by blood flow and thus has a strong movement ability.
In addition, there are numerous shapes and structures, such as half-moons (Liu et al., 2023), dumbbell shapes, and deformable structures. Researchers can select materials, structures, and manufacturing methods according to the needed performance, driving magnetic field, moving environment, and other aspects for manufacturing magnetic medical microrobots.
3 Control system of magnetic medical microrobots
After preparing magnetic medical microrobots, precise control of microrobots to complete various medical tasks has become a focus of attention. In this section, we divide the control system of the magnetic medical microrobot into three parts: magnetic actuation, imaging, and control modules, and we summarize and discuss the research progress of each module.
3.1 Magnetic actuation module
A magnetic actuation module that can output uniformly variable magnetic fields is the premise of accurately controlling the motion of the microrobot. Presently, the mainstream magnetic actuation modules are permanent magnet and electromagnetic systems.
The magnetic field generated by the permanent magnet system is stable and the price is low. Various permanent magnet systems have been designed over the years, such as the single permanent magnet system (Liao et al., 2016) (Figure 2A), permanent magnet systems that can generate a rotating magnetic field(Mahoney and Abbott, 2014), and multi-permanent magnet systems (Ryan and Diller, 2017; Ginoya et al., 2021). However, the magnetic field strength of a permanent magnet is proportional to its volume. This makes the drive system bulkier, leading to increased costs and reduced flexibility. Moreover, the magnetic field of a permanent magnet system cannot disappear immediately, which may lead to certain safety risks in clinical operations.
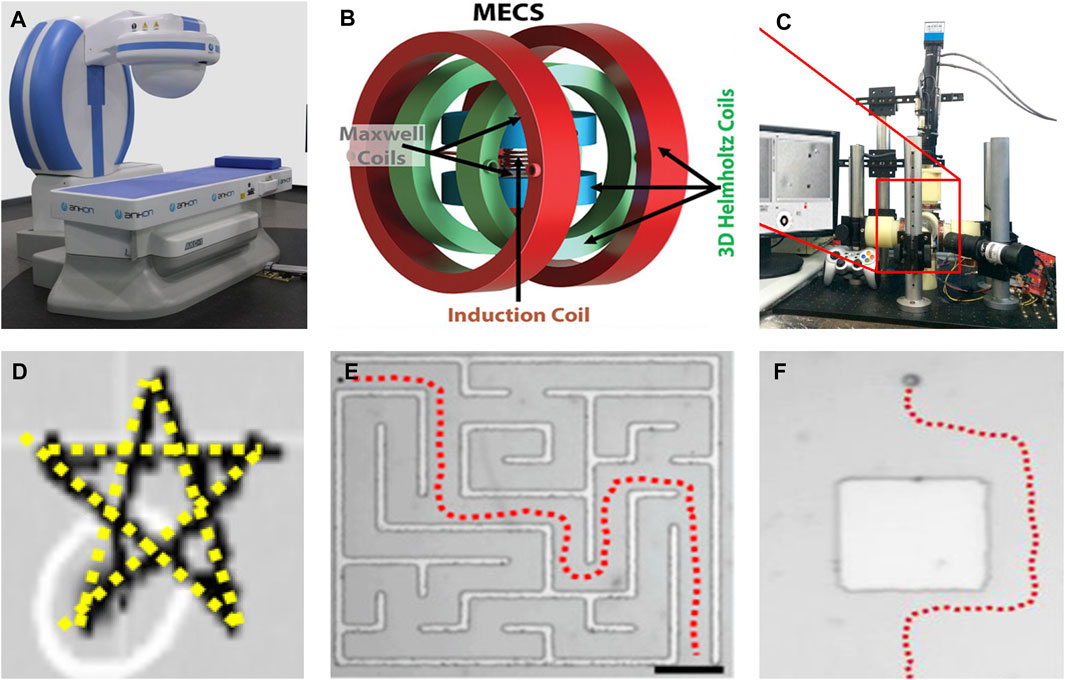
FIGURE 2. Magnetic actuation modules and control methods. (A) Single permanent magnet system. Reprinted from Liao et al. (2016). Copyright 2016 The Authors under the CC-BY 4.0 license. (B) Triaxial helmholtz coil. Reprinted from Ramos-Sebastian et al. (2022). Copyright 2022 The Authors under the CC-BY 4.0 license. (C) Five-coil electromagnetic system. Adapted from Wang et al. (2016). Copyright 2016 The Authors under the CC-BY 4.0 license. (D) PID control. Adapted from Tang et al. (2022). Copyright 2022 The Authors under the CC-BY 4.0 license. (E) The path planning algorithm Dijkstra. (F) Planning of obstacle avoidance paths. (E,F) reprinted from Li et al. (2017). Copyright 2017 American Chemical Society.
Electromagnetic systems generate the needed magnetic field by setting the input current value and waveform. Paired coils are a mainstream electromagnetic system, consisting of Helmholtz coils and Maxwell coils (Ramos-Sebastian et al., 2022) (Figure 2B), similar jobs include (Wang et al., 2022b; Xu et al., 2023). The disadvantage of paired coils is that the ratio of the coil diameter to the distance between the coils is unchanged. Increasing the coil diameter to expand the working space results in a larger device size, compromising energy efficiency and weakening the magnetic field strength. Distributed electromagnetic systems are another type of mainstream electromagnetic system. It installs cylindrical coils symmetrically around the workspace and inserts an iron core inside the coil to enhance the magnetic field. The number of coils in a distributed electromagnetic coil system is typically five (Chen et al., 2022), six (Wang et al., 2016) (Figure 2C), and eight (Kummer et al., 2010; Le et al., 2016). The more the number of coils, the more degrees of freedom the microrobot has (the number of coils is less than 8). When the number of coils reaches eight, all six degrees of freedom of motion of the magnetic microrobot are controllable. The disadvantage is that the working space of the electromagnetic system is certain and cannot be moved. However, (Yang Z. X. et al., 2020b; Du et al., 2022; Zhang et al., 2023), combined an electromagnetic system with a robotic arm to increase the working space. When an electromagnetic system works for a long time, it will produce a high temperature and thus may damage the system equipment. Therefore, the electromagnetic system usually needs to be equipped with an additional cooling system.
A permanent magnet system has a large working space; therefore, it is suitable for controlling magnetic medical microrobots with a wide range of motion. However, magnetic microrobots move with low precision and few degrees of freedom in a permanent magnet system. The electromagnetic system has numerous degrees of freedom; therefore, it can precisely control the robot to complete various complex movements; however, because the working space is fixed, the application is limited to the operation of local areas.
3.2 Imaging module
At present, most research experiments involve in vitro environments, and CCD cameras can be used to observe the size of microrobots at the millimeter and submillimeter levels (Xu et al., 2020). Microscopes and cameras are often combined to observe microrobots at micron or nanometer scales (Li et al., 2020).
When the motion scene of the microrobots is in the body, the camera cannot observe the position and state of the microrobots. Therefore, we need to adopt other imaging positioning technologies to locate the robot.
If the movement range of the microrobot is in shallow tissue, such as subcutaneous tissue, the optimal choice is fluorescent imaging (FI) (Yan et al., 2017) technology and ultrasonic (US) imaging technology (Wu et al., 2018; Wang et al., 2022a). The tissue penetration ability of the two technologies is limited; however, they are cost-effective and offer excellent real-time performance.
When a microrobot is in deep tissues, magnetic resonance imaging (MRI) technology with excellent tissue penetration ability is an excellent choice. (Tiryaki and Sitti, 2022). used MRI technology to locate the position of the microrobot in real-time in an in vitro pig kidney. MRI can also be used for postoperative imaging (Go et al., 2022). X-ray technology is also an excellent choice. (Nguyen et al., 2021). designed a microrobot loaded with doxorubicin (DOX) to autonomously navigate to the pathological position under X-ray imaging to complete drug release. However, the drawback of X-rays is that they produce strong ionizing radiation. Imaging cannot be performed over an extended period or in real time.
In addition to the imaging methods discussed above, imaging methods include magnetic (Son et al., 2016; Xu et al., 2021; Le et al., 2023) and radio frequency positioning methods (Bao et al., 2015; Hoang et al., 2023). The spatial position information of the microrobot was obtained by collecting and processing magnetic field information/high-frequency electromagnetic waves. However, the disadvantage of this method is that the relative position information between the microrobots and the organization cannot be obtained.
3.3 Control module
We divide the control methods into three categories (open-loop, closed-loop, and autonomous controls). The first type of control was open control. Open-loop control means that the operator manually controls the motion of microrobots. (Jeon et al., 2019).used an open-loop method to control the movement of porous microrobots loaded with stem cells in a mouse cerebral vascular model. Although the open-loop control mode is simple and convenient to operate, the error is large, and it is difficult to control the movement accurately.
The second type of control is closed-loop control. Currently, various control algorithms have been applied to magnetic medical microrobots, such as proportional integral derivative (PID) control (Tang et al., 2022) (Figure 2D), robust control, model predictive control (MPC) (Yang et al., 2021), sliding mode control (Mousavi et al., 2022), and backstepping control (Fruchard, 2023). The operator still must help the microrobot to identify the target and plan the trajectory, therefore, its control is not completely automatic and intelligent, and we also call the closed-loop control semi-autonomous control.
The third type of control is autonomous control. Autonomous control has a planning and decision-making component in addition to the sensing and control component, which enables magnetic microrobots to have autonomous trajectory planning, self-learning, and self-decision-making capabilities. It is difficult for microrobots that move only along a fixed trajectory to complete complex medical tasks; thus, an autonomous control method is needed for the next-generation of microrobots.
We often use global path planning algorithms to determine a collision-free optimal path for a magnetic microrobot to ensure that it can reach the target area quickly, as (Liu et al., 2020) used the informed optimal random exploring tree (Informed RRT*) algorithm to provide efficient and reliable obstacle avoidance paths for spiral microrobots. In subsequent work (Liu et al., 2021), the RRT*−Connect algorithm was used to plan a smooth and shortest path in space, similar to A-star (A*) (Fan et al., 2018) and PSO (Yang L. D. et al., 2020a). However, the global path planning algorithm is only applicable in static environments and cannot make decisions regarding dynamic obstacles. In complex and dynamic in vivo environments, hybrid global and local path planning is an optimal choice, (Li et al., 2017), used the Dijkstra algorithm to achieve global path planning (Figure 2E), while using analog logic methods to change the trajectory of magnetic microrobots when encountering dynamic obstacles, thus achieving dynamic obstacle avoidance (Figure 2F). Machine learning and deep learning have also been integrated into magnetic medical robots, empowering them with self-learning and enhanced environmental adaptability (Behrens and Ruder, 2022; Yang et al., 2022).
Among these three control methods, closed-loop control is currently the most suitable control metho. Although autonomous control offers the robot the ability to plan and make decisions, it can make faster and more accurate plans than the human brain. However, it overly relies on algorithms and is not as stable as the human brain. Hence, we contend that human involvement in the control system ensures safety.
4 Conclusion and outlooks
This review introduces the design and control systems of magnetic medical microrobots and divides the materials composed of magnetic medical microrobots into three categories. Here, we provide examples of the members and functions of the three materials. Fabrication methods for the three main structures of magnetic medical microrobots are also introduced. Subsequently, we analyze and discuss the control system using three modules. This study provides a reference for developers to design and control magnetic medical microrobots. Table 1 summarizes the characteristics of each module of a magnetic medical microrobot. Based on the needed performance and medical functions, we can select suitable materials, structural fabrication techniques, magnetic drive modules, imaging modules, and control methods. This allows for the comprehensive design of the robot and the construction of its control system.
Although microrobots have been tested in vitro, and several have been tested in animals, they are still not qualified for actual clinical application (Soto and Chrostowski, 2018). Future developments of magnetic medical microrobots include the following: 1) The materials of magnetic medical microrobots should meet the standards of practical biomedical applications and improve their biocompatibility and biodegradability. 2) Group magnetic medical microrobots can achieve various motion modes such as collaboration and modules, and perform more complex medical tasks, which is an important direction in the research and development of magnetic medical microrobots. (Wang et al., 2022b; Yu et al., 2022; Mayorga-Martinez et al., 2023). have been studying the motion of group magnetic medical microrobots. 3) Intelligent and multi-degree-of-freedom development of magnetic medical microrobots is necessary, with self-examination, learning ability, variability ability, and the ability to adapt to the changeable and complex environment by changing the shape or motion state. Through detailed research on the design and control systems of magnetic medical microrobots, we think that magnetic medical microrobots will become a daily presence, safeguarding our health.
Author contributions
JZ: Writing–original draft. ML: Writing–review and editing. NL: Writing–review and editing. YZ: Writing–review and editing. JW: Writing–review and editing. NJ: Writing–review and editing.
Funding
The author(s) declare financial support was received for the research, authorship, and/or publication of this article. This work was supported by the National Natural Science Foundation of China (Grant Nos U23A20342, 62273331, 62127811, and 61821005), CAS Project for Young Scientists in Basic Research (Grant No. YSBR-036), and CAS/SAFEA International Partnership Program for Creative Research Teams.
Conflict of interest
The authors declare that the research was conducted in the absence of any commercial or financial relationships that could be construed as a potential conflict of interest.
Publisher’s note
All claims expressed in this article are solely those of the authors and do not necessarily represent those of their affiliated organizations, or those of the publisher, the editors and the reviewers. Any product that may be evaluated in this article, or claim that may be made by its manufacturer, is not guaranteed or endorsed by the publisher.
References
Alapan, Y., Bozuyuk, U., Erkoc, P., Karacakol, A. C., and Sitti, M. (2020). Multifunctional surface microrollers for targeted cargo delivery in physiological blood flow. Sci. Rob. 5, eaba5726. doi:10.1126/scirobotics.aba5726
Alapan, Y., Yasa, O., Schauer, O., Giltinan, J., Tabak, A. F., Sourjik, V., et al. (2018). Soft erythrocyte-based bacterial microswimmers for cargo delivery. Sci. Rob. 3, eaar4423. doi:10.1126/scirobotics.aar4423
Alcântara, C. C. J., Kim, S., Lee, S., Jang, B., Thakolkaran, P., Kim, J. Y., et al. (2019). 3D fabrication of fully iron magnetic microrobots. Small 15, 1805006. doi:10.1002/smll.201805006
Bao, G. Q., Pahlavan, K., and Mi, L. (2015). Hybrid localization of microrobotic endoscopic capsule inside small intestine by data fusion of vision and RF sensors. IEEE Sens. J. 15, 2669–2678. doi:10.1109/jsen.2014.2367495
Behrens, M. R., and Ruder, W. C. (2022). Smart magnetic microrobots learn to swim with deep reinforcement learning. Adv. Intell. Syst. 4, 2200023. doi:10.1002/aisy.202200023
Bernasconi, R., Cuneo, F., Carrara, E., Chatzipirpiridis, G., Hoop, M., Chen, X. Z., et al. (2018). Hard-magnetic cell microscaffolds from electroless coated 3D printed architectures. Mat. Horiz. 5, 699–707. doi:10.1039/c8mh00206a
Bernasconi, R., Prioglio, G., Angeli, M. C., Alcantara, C. C. J., Sevim, S., Pané, S., et al. (2022). Wet metallization of 3D printed microarchitectures: application to the manufacturing of bioinspired microswimmers. J. Manuf. Process 78, 11–21. doi:10.1016/j.jmapro.2022.03.057
Bozuyuk, U., Alapan, Y., Aghakhani, A., Yunusa, M., and Sitti, M. (2021). Shape anisotropy-governed locomotion of surface microrollers on vessel-like microtopographies against physiological flows. Proc. Natl. Acad. Sci. U.S.A. 118, e2022090118. doi:10.1073/pnas.2022090118
Bozuyuk, U., Suadiye, E., Aghakhani, A., Dogan, N. O., Lazovic, J., Tiryaki, M. E., et al. (2022). High-performance magnetic FePt (L10) surface microrollers towards medical imaging-guided endovascular delivery applications. Adv. Funct. Mat. 32, 2109741. doi:10.1002/adfm.202109741
Ceylan, H., Yasa, I. C., Yasa, O., Tabak, A. F., Giltinan, J., and Sitti, M. (2019). 3D-Printed biodegradable microswimmer for theranostic cargo delivery and release. Acs Nano 13, 3353–3362. doi:10.1021/acsnano.8b09233
Chen, J. F., Hu, H. W., and Wang, Y. (2023). Magnetic-driven 3D-printed biodegradable swimming microrobots. Smart Mat. Struct. 32, 085014. doi:10.1088/1361-665X/ace1ba
Chen, J. X., Lin, D. J., Zhou, Y. T., and Jiao, N. D. (2022). Detachable electromagnetic actuation system for inverted microscope and its function in motion control of microrobots. J. Magn. Magn. Mat. 564, 170159. doi:10.1016/j.jmmm.2022.170159
Cong, Z. Q., Tang, S. S., Xie, L. M., Yang, M., Li, Y. Y., Lu, D. D., et al. (2022). Magnetic-powered janus cell robots loaded with oncolytic adenovirus for active and targeted virotherapy of bladder cancer. Adv. Mat. 34, 2201042. doi:10.1002/adma.202201042
Dogan, N. O., Ceylan, H., Suadiye, E., Sheehan, D., Aydin, A., Yasa, I. C., et al. (2022). Remotely guided immunobots engaged in anti-tumorigenic phenotypes for targeted cancer immunotherapy. Small 18, 2204016. doi:10.1002/smll.202204016
Dong, M., Wang, X. P., Chen, X. Z., Mushtaq, F., Deng, S. Y., Zhu, C. H., et al. (2020). 3D-Printed soft magnetoelectric microswimmers for delivery and differentiation of neuron-like cells. Adv. Funct. Mat. 30, 1910323. doi:10.1002/adfm.201910323
Dreyfus, R., Baudry, J., Roper, M. L., Fermigier, M., Stone, H. A., and Bibette, J. (2005). Microscopic artificial swimmers. Nature 437, 862–865. doi:10.1038/nature04090
Du, X. Z., Wang, Q. Q., Jin, D. D., Chiu, P. W. Y., Pang, C. P., Chong, K. K. L., et al. (2022). Real-time navigation of an untethered miniature robot using mobile ultrasound imaging and magnetic actuation systems. IEEE Rob. Autom. Lett. 7, 7668–7675. doi:10.1109/lra.2022.3184445
Fan, X. J., Sun, M. M., Lin, Z. H., Song, J. M., He, Q., Sun, L. N., et al. (2018). Automated noncontact micromanipulation using magnetic swimming microrobots. IEEE Trans. Nanotechnol. 17, 666–669. doi:10.1109/tnano.2018.2797325
Field, R. D., Anandakumaran, P. N., and Sia, S. K. (2019). Soft medical microrobots: design components and system integration. Appl. Phys. Rev. 6, 041305. doi:10.1063/1.5124007
Fruchard, M. (2023). Controllability and control synthesis of underactuated magnetic microrobots. Automatica 149, 110823. doi:10.1016/j.automatica.2022.110823
Fusco, S., Huang, H. W., Peyer, K. E., Peters, C., Häberli, M., Ulbers, A., et al. (2015). Shape-switching microrobots for medical applications: the influence of shape in drug delivery and locomotion. ACS Appl. Mat. Interfaces 7, 6803–6811. doi:10.1021/acsami.5b00181
Giltinan, J., Sridhar, V., Bozuyuk, U., Sheehan, D., and Sitti, M. (2021). 3D microprinting of iron platinum nanoparticle-based magnetic mobile microrobots. Adv. Intell. Syst. 3, 2000204. doi:10.1002/aisy.202000204
Ginoya, T., Maddahi, Y., and Zareinia, K. (2021). A historical review of medical robotic platforms. J. Rob. 2021, 6640031. doi:10.1155/2021/6640031
Go, G., Yoo, A., Nguyen, K. T., Nan, M., Darmawan, B. A., Zheng, S., et al. (2022). Multifunctional microrobot with real-time visualization and magnetic resonance imaging for chemoembolization therapy of liver cancer. Sci. Adv. 8, eabq8545. doi:10.1126/sciadv.abq8545
Goudu, S. R., Yasa, I. C., Hu, X. H., Ceylan, H., Hu, W. Q., and Sitti, M. (2020). Biodegradable untethered magnetic hydrogel milli-grippers. Adv. Funct. Mat. 30, 2004975. doi:10.1002/adfm.202004975
Gyak, K. W., Jeon, S., Ha, L., Kim, S., Kim, J. Y., Lee, K. S., et al. (2019). Magnetically actuated SiCN-based ceramic microrobot for guided cell delivery. Adv. Healthc. Mat. 8, 1900739. doi:10.1002/adhm.201900739
Hoang, M. C., Liu, S. L., Nguyen, K. T., Lee, H. S., Hong, A., Bang, S., et al. (2023). DEMA: robotic dual-electromagnet actuation system integrated with localization for a magnetic capsule endoscope. Sens. Actuat a-Phys 361, 114596. doi:10.1016/j.sna.2023.114596
Jeon, S., Kim, S., Ha, S., Lee, S., Kim, E., Kim, S. Y., et al. (2019). Magnetically actuated microrobots as a platform for stem cell transplantation. Sci. Rob. 4, eaav4317. doi:10.1126/scirobotics.aav4317
Jiang, J. L., Yang, Z. X., Ferreira, A., and Zhang, L. (2022). Control and autonomy of microrobots: recent progress and perspective. Adv. Intell. Syst. 4, 2100279. doi:10.1002/aisy.202100279
Kim, J. Y., Jeon, S., Lee, J., Lee, S., Lee, J., Jeon, B. O., et al. (2018). A simple and rapid fabrication method for biodegradable drug-encapsulating microrobots using laser micromachining, and characterization thereof. Sens. Actuators B Chem. 266, 276–287. doi:10.1016/j.snb.2018.03.033
Kummer, M. P., Abbott, J. J., Kratochvil, B. E., Borer, R., Sengul, A., and Nelson, B. J. (2010). OctoMag: an electromagnetic system for 5-DOF wireless micromanipulation. IEEE Trans. Rob. 26, 1006–1017. doi:10.1109/Tro.2010.2073030
Le, T. A., Hadadian, Y., and Yoon, J. (2023). A prediction model for magnetic particle imaging-based magnetic hyperthermia applied to a brain tumor model. Comput. Meth Prog. Bio 235, 107546. doi:10.1016/j.cmpb.2023.107546
Le, V. N. T., Nguyen, N. H., Alameh, K., Weerasooriya, R., and Pratten, P. (2016). Accurate modeling and positioning of a magnetically controlled catheter tip. Med. Phys. 43, 650–663. doi:10.1118/1.4939228
Lee, Y. W., Ceylan, H., Yasa, I. C., Kilic, U., and Sitti, M. (2021). 3D-Printed multi-stimuli-responsive mobile micromachines. ACS Appl. Mat. Interfaces 13, 12759–12766. doi:10.1021/acsami.0c18221
Lee, Y. W., Kim, J. K., Bozuyuk, U., Dogan, N. O., Khan, M. T. A., Shiva, A., et al. (2023). Multifunctional 3D-printed pollen grain-inspired hydrogel microrobots for on-demand anchoring and cargo delivery. Adv. Mat. 35, 2209812. doi:10.1002/adma.202209812
Li, D. F., Niu, F. Z., Li, J. Y., Li, X. J., and Sun, D. (2020). Gradient-enhanced electromagnetic actuation system with a new core shape design for microrobot manipulation. IEEE Trans. Ind. Electron. 67, 4700–4710. doi:10.1109/tie.2019.2928283
Li, J. Y., Li, X. J., Luo, T., Wang, R., Liu, C. C., Chen, S. X., et al. (2018). Development of a magnetic microrobot for carrying and delivering targeted cells. Sci. Rob. 3, eaat8829. doi:10.1126/scirobotics.aat8829
Li, T. L., Chang, X. C., Wu, Z. G., Li, J. X., Shao, G. B., Deng, X. H., et al. (2017). Autonomous collision-free navigation of microvehicles in complex and dynamically changing environments. Acs Nano 11, 9268–9275. doi:10.1021/acsnano.7b04525
Liao, Z. A., Hou, X., Lin-Hu, E. Q., Sheng, J. Q., Ge, Z. Z., Jiang, B., et al. (2016). Accuracy of magnetically controlled capsule endoscopy, compared with conventional gastroscopy, in detection of gastric diseases. Clin. Gastroenterol. Hepatol. 14, 1266–1273. doi:10.1016/j.cgh.2016.05.013
Liu, D., Sun, D. Y., Zhou, J. X., Liu, H. R., Guo, R. R., Wang, B., et al. (2023). Bionic morphological design and interface-free fabrication of halfmoon microrobots with enhanced motion performance. Chem. Eng. J. 452, 139464. doi:10.1016/j.cej.2022.139464
Liu, J., Wu, X. Y., Huang, C. Y., Manamanchaiyaporn, L., Shang, W. F., Yan, X. H., et al. (2021). 3-D autonomous manipulation system of helical microswimmers with online compensation update. IEEE Trans. Autom. Sci. Eng. 18, 1380–1391. doi:10.1109/tase.2020.3006131
Liu, J., Xu, T. T., Yang, S. X., and Wu, X. Y. (2020). Navigation and visual feedback control for magnetically driven helical miniature swimmers. IEEE Trans. Ind. Inf. 16, 477–487. doi:10.1109/tii.2019.2913762
Mahoney, A. W., and Abbott, J. J. (2014). Generating rotating magnetic fields with a single permanent magnet for propulsion of untethered magnetic devices in a lumen. IEEE Trans. Rob. 30, 411–420. doi:10.1109/tro.2013.2289019
Maria-Hormigos, R., Mayorga-Martinez, C. C., and Pumera, M. (2023). Soft magnetic microrobots for photoactive pollutant removal. Small Methods 7, 2201014. doi:10.1002/smtd.202201014
Mayorga-Martinez, C. C., Fojtu, M., Vyskocil, J., Cho, N. J., and Pumera, M. (2022). Pollen-based magnetic microrobots are mediated by electrostatic forces to attract, manipulate, and kill cancer cells. Adv. Funct. Mat. 32, 2207272. doi:10.1002/adfm.202207272
Mayorga-Martinez, C. C., Zelenka, J., Klima, K., Kubanova, M., Ruml, T., and Pumera, M. (2023). Multimodal-Driven magnetic microrobots with enhanced bactericidal activity for biofilm eradication and removal from titanium mesh. Adv. Mat. 35, 2300191. doi:10.1002/adma.202300191
Mousavi, A., Khaksar, H., Ahmed, A., Choi, H., and Hoshiar, A. K. (2022). “Magnetic microrobot control using an adaptive fuzzy sliding-mode method,” in IEEE/RSJ International Conference on Intelligent Robots and Systems (IROS), Huntington Place in Detroit, Michigan, USA, October 1 – 5, 2023, 6484–6489.
Nguyen, K. T., Go, G. J., Jin, Z., Darmawan, B. A., Yoo, A., Kim, S., et al. (2021). A magnetically guided self-rolled microrobot for targeted drug delivery, real-time X-ray imaging, and microrobot retrieval. Adv. Healthc. Mat. 10, 2001681. doi:10.1002/adhm.202001681
Noh, S., Jeon, S., Kim, E., Oh, U., Park, D., Park, S. H., et al. (2022). A biodegradable magnetic microrobot based on gelatin methacrylate for precise delivery of stem cells with mass production capability. Small 18, 2107888. doi:10.1002/smll.202107888
Park, J., Jin, C., Lee, S., Kim, J. Y., and Choi, H. (2019). Magnetically actuated degradable microrobots for actively controlled drug release and hyperthermia therapy. Adv. Healthc. Mat. 8, 1900213. doi:10.1002/adhm.201900213
Rajabasadi, F., Schwarz, L., Medina-Sáncehz, M., and Schmidt, O. G. (2021). 3D and 4D lithography of untethered microrobots. Prog. Mater Sci. 120, 100808. doi:10.1016/j.pmatsci.2021.100808
Ramos-Sebastian, A., Gwak, S. J., and Kim, S. H. (2022). Multimodal locomotion and active targeted thermal control of magnetic agents for biomedical applications. Adv. Sci. 9, 2103863. doi:10.1002/advs.202103863
Ryan, P., and Diller, E. (2017). Magnetic actuation for full dexterity microrobotic control using rotating permanent magnets. IEEE Trans. Rob. 33, 1398–1409. doi:10.1109/tro.2017.2719687
Son, D., Yim, S., and Sitti, M. (2016). A 5-D localization method for a magnetically manipulated untethered robot using a 2-D array of Hall-effect sensors. IEEE ASME Trans. Mechatron. 21, 708–716. doi:10.1109/tmech.2015.2488361
Soto, F., and Chrostowski, R. (2018). Frontiers of medical micro/nanorobotics: in vivo applications and commercialization perspectives toward clinical uses. Front. Bioeng. Biotechnol. 6, 170. doi:10.3389/fbioe.2018.00170
Soto, F., Karshalev, E., Zhang, F. Y., de Avila, B. E. F., Nourhani, A., and Wang, J. (2022). Smart materials for microrobots. Chem. Rev. 122, 5365–5403. doi:10.1021/acs.chemrev.0c00999
Tang, X. Q., Li, Y. K., Liu, X. M., Liu, D., Chen, Z., and Arai, T. (2022). Vision-based automated control of magnetic microrobots. Micromachines 13, 337. doi:10.3390/mi13020337
Tiryaki, M. E., and Sitti, M. (2022). Magnetic resonance imaging-based tracking and navigation of submillimeter-scale wireless magnetic robots. Adv. Intell. Syst. 4, 2100178. doi:10.1002/aisy.202100178
Urso, M., Ussia, M., and Pumera, M. (2021). Breaking polymer chains with self-propelled light-controlled navigable hematite microrobots. Adv. Funct. Mat. 31, 2101510. doi:10.1002/adfm.202101510
Walker, D., Käsdorf, B. T., Jeong, H. H., Lieleg, O., and Fischer, P. (2015). Enzymatically active biomimetic micropropellers for the penetration of mucin gels. Sci. Adv. 1, e1500501. doi:10.1126/sciadv.1500501
Wang, J. Y., Jiao, N. D., Tung, S., and Liu, L. Q. (2016). Automatic path tracking and target manipulation of a magnetic microrobot. Micromachines 7, 212. doi:10.3390/mi7110212
Wang, Q. Q., Du, X. Z., Jin, D. D., and Zhang, L. (2022a). Real-time ultrasound Doppler tracking and autonomous navigation of a miniature helical robot for accelerating thrombolysis in dynamic blood flow. Acs Nano 16, 604–616. doi:10.1021/acsnano.1c07830
Wang, Q. Q., Tian, Y., Du, X. Z., Ko, H., Ip, B. Y. M., Leung, T. W. H., et al. (2022b). Magnetic navigation of collective cell microrobots in blood under ultrasound Doppler imaging. IEEE ASME Trans. Mechatron. 27, 3174–3185. doi:10.1109/tmech.2021.3109346
Wang, X., Hu, C., Pane, S., and Nelson, B. J. (2022c). Dynamic modeling of magnetic helical microrobots. IEEE Rob. Autom. Lett. 7, 1682–1688. doi:10.1109/lra.2020.3049112
Wang, Z. B., Xu, Z. J., Zhu, B., Zhang, Y., Lin, J. W., Wu, Y. G., et al. (2022d). Design, fabrication and application of magnetically actuated micro/nanorobots: a review. Nanotechnology 33, 152001. doi:10.1088/1361-6528/ac43e6
Wang, Z. H., Mu, X. L., Tan, L. Y., Zhong, Y. K., and Cheang, U. K. (2022e). A rolled-up-based fabrication method of 3D helical microrobots. Front. Rob. AI 9, 1063987. doi:10.3389/frobt.2022.1063987
Wei, T. Y., Liu, J., Li, D. F., Chen, S. X., Zhang, Y. C., Li, J. Y., et al. (2020). Development of magnet-driven and image-guided degradable microrobots for the precise delivery of engineered stem cells for cancer therapy. Small 16, 1906908. doi:10.1002/smll.201906908
Wu, Z. G., Troll, J., Jeong, H. H., Wei, Q., Stang, M., Ziemssen, F., et al. (2018). A swarm of slippery micropropellers penetrates the vitreous body of the eye. Sci. Adv. 4, eaat4388. doi:10.1126/sciadv.aat4388
Wu, Z. H., Zhang, Y. T., Ai, N. N., Chen, H. R., Ge, W., and Xu, Q. S. (2022). Magnetic mobile microrobots for upstream and downstream navigation in biofluids with variable flow rate. Adv. Intell. Syst. 4, 2100266. doi:10.1002/aisy.202100266
Xin, C., Jin, D. D., Hu, Y. L., Yang, L., Li, R., Wang, L., et al. (2021). Environmentally adaptive shape-morphing microrobots for localized cancer cell treatment. Acs Nano 15, 18048–18059. doi:10.1021/acsnano.1c06651
Xu, T. T., Guan, Y. M., Liu, J., and Wu, X. Y. (2020). Image-based visual servoing of helical microswimmers for planar path following. IEEE Trans. Autom. Sci. Eng. 17, 325–333. doi:10.1109/tase.2019.2911985
Xu, T. T., Liu, J., Huang, C. Y., Sun, T. F., and Wu, X. Y. (2022). Discrete-time optimal control of miniature helical swimmers in horizontal plane. IEEE Trans. Autom. Sci. Eng. 19, 2267–2277. doi:10.1109/tase.2021.3079958
Xu, Y. H., Huang, C. Y., Li, D., Liu, J., Fu, S. X., Wu, X. Y., et al. (2023). Cooperative control strategy of magnetic microrobots in bifurcated microfluidic channels. IEEE Rob. Autom. Lett. 8, 1889–1896. doi:10.1109/lra.2023.3243472
Xu, Y. X., Li, K. Y., Zhao, Z. Q., and Meng, M. Q. H. (2021). A novel system for closed-loop simultaneous magnetic actuation and localization of WCE based on external sensors and rotating actuation. IEEE Trans. Autom. Sci. Eng. 18, 1640–1652. doi:10.1109/tase.2020.3013954
Yan, X. H., Zhou, Q., Vincent, M., Deng, Y., Yu, J. F., Xu, J. B., et al. (2017). Multifunctional biohybrid magnetite microrobots for imaging-guided therapy. Sci. Rob. 2, eaaq1155. doi:10.1126/scirobotics.aaq1155
Yang, L. D., Jiang, J. L., Gao, X. J., Wang, Q. L., Dou, Q., and Zhang, L. (2022). Autonomous environment-adaptive microrobot swarm navigation enabled by deep learning-based real-time distribution planning. Nat. Mach. Intell. 4, 480–493. doi:10.1038/s42256-022-00482-8
Yang, L. D., Zhang, Y. B., Wang, Q. Q., Chan, K. F., and Zhang, L. (2020a). Automated control of magnetic spore-based microrobot using fluorescence imaging for targeted delivery with cellular resolution. IEEE Trans. Autom. Sci. Eng. 17, 490–501. doi:10.1109/tase.2019.2937232
Yang, Z. X., and Li, Z. (2020). Magnetic actuation systems for miniature robots: a review. Adv. Intell. Syst. 2, 2000082. doi:10.1002/aisy.202000082
Yang, Z. X., Yang, L. D., and Zhang, L. (2020b). 3-D visual servoing of magnetic miniature swimmers using parallel mobile coils. IEEE Trans. Med. Rob. Bionics 2, 608–618. doi:10.1109/tmrb.2020.3033020
Yang, Z. X., Yang, L. D., and Zhang, L. (2021). Autonomous navigation of magnetic microrobots in a large workspace using mobile-coil system. IEEE ASME Trans. Mechatron. 26, 3163–3174. doi:10.1109/tmech.2021.3054927
Yu, J. F., Yang, L. D., Du, X. Z., Chen, H., Xu, T. T., and Zhang, L. (2022). Adaptive pattern and motion control of magnetic microrobotic swarms. IEEE Trans. Rob. 38, 1552–1570. doi:10.1109/Tro.2021.3130432
Zeeshan, M. A., Grisch, R., Pellicer, E., Sivaraman, K. M., Peyer, K. E., Sort, J., et al. (2013). Hybrid helical magnetic microrobots obtained by 3D template-assisted electrodeposition. Small 10, 1284–1288. doi:10.1002/smll.201302856
Zhang, M. Q., Yang, L. D., Zhang, C., Yang, Z. X., and Zhang, L. (2023). A 5-D large-workspace magnetic localization and actuation system based on an eye-in-hand magnetic sensor array and mobile coils. IEEE Trans. Instrum. Meas. 72, 7501411. doi:10.1109/tim.2023.3238693
Zhou, H. J., Mayorga-Martinez, C. C., Pané, S., Zhang, L., and Pumera, M. (2021). Magnetically driven micro and nanorobots. Chem. Rev. 121, 4999–5041. doi:10.1021/acs.chemrev.0c01234
Keywords: magnetic actuation, medical microrobot, control system, design system, system integration
Citation: Zhou J, Li M, Li N, Zhou Y, Wang J and Jiao N (2023) System integration of magnetic medical microrobots: from design to control. Front. Robot. AI 10:1330960. doi: 10.3389/frobt.2023.1330960
Received: 31 October 2023; Accepted: 28 November 2023;
Published: 19 December 2023.
Edited by:
Manh Cuong Hoang, Korea Institute of Medical Microrobotics, Republic of KoreaReviewed by:
Ahmet Fatih Tabak, Istanbul Commerce University, TürkiyeRoberto Bernasconi, Polytechnic University of Milan, Italy
Copyright © 2023 Zhou, Li, Li, Zhou, Wang and Jiao. This is an open-access article distributed under the terms of the Creative Commons Attribution License (CC BY). The use, distribution or reproduction in other forums is permitted, provided the original author(s) and the copyright owner(s) are credited and that the original publication in this journal is cited, in accordance with accepted academic practice. No use, distribution or reproduction is permitted which does not comply with these terms.
*Correspondence: Niandong Jiao, ndjiao@sia.cn; Jingyi Wang, wangjingyi@syau.edu.cn