- 1INRA, LSE, Université de Lorraine, Nancy, France
- 2ECOSYS, INRA, Thiverval-Grignon, France
- 3VERI, Veolia Recherche et Innovation, Limay, France
Urban compost application in agroecosystems enhances soil fertility but can also be a source of (micro) plastics, which are not completely removed during the composting process. Knowledge of the fate of these plastics in regularly-amended soils is thus an issue for the environmental management of these soils. The aims of this study were (1) to develop a method combining soil fractionation, microscopic observation and chemical characterization to follow the fate of plastics in soils and (2) to apply this method on a long-term experimental field, where municipal solid waste composts were applied every other year during 10 years. The presence of plastics was investigated within compost and soil fractions using morphological and analytical characterization by transmission electronic microscopy (TEM-EDX) and pyrolysis coupled to gas chromatography and mass spectrometry (Py/GC/MS). Specific features of plastics allowed us to distinguish these polymers from soil organic matter even in the <200 μm soil fractions. Ti and Ba detection associated with these features, as they are initially added during the polymer production, also constituted plastic tracers within organo-mineral fractions. Plastic fragments as detected by TEM were less abundant in the fine soil fractions compared to the coarsest ones. The abundance of styrene produced upon pyrolysis, used as a molecular tracer of plastics, also decreased relative to produced toluene according to the same particle size gradient. Our results evidenced that plastics and microplastics were present in the soil that was amended for 10 years with compost, while not in the control soil. MPs were mostly observed as individualized particles, present in the coarsest fractions as well as some of the fine soil fractions, but they were little associated with the soil matrix. They mostly did not show any degradation features such as microbial lysis. We thus suggest that their evolution in soil was mainly due to fragmentation. Our methodological approach provides tools to monitor the fate of microplastics over time and specify the contribution of such contaminants in soil amended with bio-based products.
Introduction
In order to promote return of organic matter to soil and thus contribute to the restoration of soil quality, current agricultural practices include the application of composts derived from organic waste materials (Aggelides and Londra, 2000; Weber et al., 2007). In this context of optimization of the recycling of these organic materials, the composting of household waste makes it possible to reduce the landfilling of biodegradable organic materials (Smith, 1996). However, municipal solid waste composts, beside organic matter, contain so-called inert materials, such as plastics but also glass and metals, and can so be a source of such undesirable constituents in soil (Brinton, 2005; Zubris and Richards, 2005). In France, since 2006, composts used in agriculture must comply with the norm on organic soil improvers (NFU 44 051). Thus, they are the subject of a sorting (method XP U44-164, 2004) limiting the compost contents in inerts, to 0.3 MS and 0.8% MS, respectively for films and expanded polystyrene (SPE) larger than 5-mm and other plastics larger than 5-mm. These values correspond to an acceptance threshold justified by the technical constraints on the collection and sorting of raw materials. Limitations of the content of impurities in compost slightly differ in size and amount limit values within national compost regulations and standards of European countries (Orbit, 2008). Microplastics (MPs), i.e., plastics in a size range of < 5 mm (Phuong et al., 2016), will thus be found in the raw organic materials subjected to composting and will end up even more fragmented in the final compost. Incorporated into the soil after compost application, they will evolve and be subjected to fragmentation processes, (bio) degradation, and/or association with soil mineral particles. In this context, the increasing use of biodegradable agricultural plastics appears as a promising environmental solution and is still now the subject of much researches to evaluate this benefit (Briassoulis and Dejean, 2010; Briassoulis et al., 2010). Nevertheless, knowledge on the persistence and accumulation of MPs in soil, as their impact on soil functioning and biodiversity is till now to be acquired (Rillig, 2012; Horton et al., 2017).
Plastic pollution was first recognized in marine environments (Barnes et al., 2009; Andrady, 2011; Galgani et al., 2015; Lusher, 2015) and over the last 10 years, many studies have investigated the distribution and effects of MPs within it (Wright et al., 2013; Vegter et al., 2014; Thompson, 2015). By contrast, only few studies aimed at evaluating land-based sources of MPs (Rillig, 2012; De Souza Machado et al., 2018), as their occurrence and fate in soils (Bläsing and Amelung, 2018). Plastic mulching is a direct source of MPs in soils (Steinmetz et al., 2016), but other inputs have also been identified such as soil amendments with sewage sludge (Zubris and Richards, 2005) or composts (Weithmann et al., 2018), irrigation and flooding, atmospheric input (Dris et al., 2015; Bläsing and Amelung, 2018) that may lead to their potential concentration over time. Bläsing and Amelung (2018) evaluated that in EU the annual input of visible plastics to soil due to compost use on arable fields, depending on application dose, represents 0.16 to 1.2 kg ha−1 (corresponding to a compost annual application rate of 7 t ha−1) up to 0.08 to 6.3 kg ha−1 (annual application rate: 35 t ha−1). However, in this estimation, MPs were not taken into account. MPs in sewage sludge were often studied, as wastewater treatment plants are receptor for the cumulative loading of MPs derived from industry, landfill, domestic wastewater, and stormwater (Steinmetz et al., 2016; Mahon et al., 2017). In household composts, MPs derive from a wide range of sources including synthetic fibers from clothing, polymer manufacturing, processing industries, and personal care products (Brinton, 2005; Hernandez et al., 2017). Thus, there is a need to qualitatively and quantitatively evaluate the contribution of such compounds in composted organic wastes in the context of their agricultural use.
In soils, plastic degradation may depend on four main processes, which vary according to the initial material composition: biodegradation, oxidation, hydrolysis, and photodegradation (Luckachan and Pillai, 2011). Nonetheless, even if some plastic degradation may occur, numerous studies showed persistence and accumulation of small plastic residues for several years to up to decades in soil (Bläsing and Amelung, 2018). Mechanisms related to the fate of micro(nano) plastics in soils may promote long term storage as they are incorporated in soil aggregates (Nizzetto et al., 2016a; Hurley and Nizzetto, 2018). Soils could become a sink for plastic contamination and the number of publications in the area of ecotoxicological research for MPs in soils greatly increases. Soil fauna activity may contribute to the transport of MPs within soil systems (Huerta Lwanga et al., 2016, 2017; Maaß et al., 2017). The uptake of MPs by plants is unlikely but may occur for nano-sized particles (Bandmann et al., 2012; Schwab et al., 2016 in Hurley and Nizzetto, 2018). So, environmental factors (UV, temperature), agricultural practices (tilling), and biological activity (microbial and fauna) contribute to plastic degradation in the top soil. On another side, impact of physico-chemical soil parameters on MPs dynamics still have to be elucidated (Bläsing and Amelung, 2018). As plastics accumulate in soils, we can wonder about its association with the organo-mineral matrix. Plastic residues potentially include organic compounds and also mineral elements, as heavy metals are often added during the plastic production to improve physical and mechanical properties of the polymer (Liu et al., 2005). Thus, identification of the organic or mineral soil phases bearing organic and mineral elements issued from plastics can help to specify the impact of plastic input on soil functioning. Hence, monitoring the fate of plastics in soil appears necessary. The identification and quantification of plastics in soils is a methodological challenge, particularly for the smallest particles.
Although a wide range of sampling techniques is used for monitoring MPs in marine environments or sediments (Hidalgo-Ruz et al., 2012; Löder and Gerdts, 2015; Rocha-Santos and Duarte, 2015; Van Cauwenberghe et al., 2015), MPs sampling is still a challenge by itself (Phuong et al., 2016). MPs in environmental samples can currently be detected down to a size of 1 μm, however few environmental studies identify particles smaller than 50 μm due to methodological limitations and MPs are rarely counted below 250 μm (Brinton, 2005; Hidalgo-Ruz et al., 2012; Fuller and Gautam, 2016; Imhof et al., 2016). Methods for plastic analyses in sediments can potentially be adjusted for application to soil but their applicability on soils still needs to be tested (Bläsing and Amelung, 2018). Four main steps are distinguished during sample processing: density separation, filtration, sieving, and visual sorting of MPs. Visual sorting is one of the most commonly used methods for the identification of MPs, using type, shape, degradation stage or color as criteria. Chemical and physical characteristics (e.g., specific density) can complete these first data. The most reliable methods to identify the chemical composition of MPs appear to be infrared or Raman spectroscopy and pyrolysis coupled to gas chromatography and mass spectrometry (Py/GC/MS) (Fabbri et al., 1998; Hidalgo-Ruz et al., 2012; Dümichen et al., 2017). Toluene and styrene are pyrolysis products present in the Py/GC/MS analysis of natural organic matter, where they generally display a ratio around 4–5. By contrast, in samples containing plastic, the styrene peak might have an additional polystyrene origin and the ratio largely decreases. A ratio below one indicates the presence of plastics (Fabbri et al., 1998). The presence of styrene dimer and trimer in the pyrolysates is a further indication of the presence of plastic (Dignac et al., 2005). Scanning electron microscopy highlighted morphological changes of the surface of plastic residues, (e.g., melting, blistering, shredding, flaking) as a result of their (bio)degradation (Leejarkpai et al., 2011; Khan et al., 2017; Mahon et al., 2017). All these reviews on analytical tools showed the relevance of morphological and analytical studies in the differently-sized compartments of the plastic contaminated ecosystems.
The aims of this study were (1) to develop a relevant protocol to identify plastic residues in all granulometric soil compartments, even in the soil fractions smaller than 50 μm and (2) to monitor the MPs contribution of urban composts in agricultural soils. We adapted some soil fractionation procedures in order to collect all plastic residues present in soil samples. Then, we morphologically and analytically characterized MPs within compost and soil fractions by transmission electronic microscopy (TEM-EDX) and Py/GC/MS. We applied these complementary analytical tools to soils from a long-term experimental field, where municipal solid waste composts were applied every 2 years during 10 years. We compared compost amended and non-amended soils in order to evaluate the traceability and fate of MPs in urban compost amended soil.
Materials and Methods
Experimental Site And Soil Sampling
Soils were sampled on the Qualiagro long-term field experiment (INRA-Veolia partnership) located about 30 km west of Paris (Feucherolles, 78, France, 48°52′N, 1°57′E) and is representative of the agricultural context of this area. At this site, various composts and manures were applied and the soil C contents were measured every other year since 1998 (Peltre et al., 2012). The average annual rainfall is 583 mm and the average annual temperature is 11°C. Soil is a loamy luvisol (according to the French pedological reference–Référentiel Pédologique, 2008) to a depth > 1.2 m and developed on carbonated loess from eolian origin. Main characteristics of the soil are presented in Table 1.
Soil was cultivated according to the usual agricultural practices and was at the sampling date (November 2009) planted with wheat. A household waste compost (municipal solid waste compost, MSW), issued from the composting of the residual fraction of household waste after packaging sorting, was applied every other year at a rate of 18.13 t ha−1 FM, calculated to bring 4t C ha−1. Experimental plots were of 450 m2 and N-fertilized, 4 replicates were realized per treatment. Soil sampling was done in one control and one amended plots in the superficial layer (0–27 cm), each sampling resulting from 10 pooled sub-samples. Part of the soil was dried for physico-chemical analyses, the other part was kept wet until soil fractionation, visual sorting of plastics and sampling for TEM characterization.
Compost Characteristics And Fractionation
The analyzed compost corresponds to the one applied on soil in 2009. Main physico-chemical characteristics of the compost are given in Table 2. Plastic contents were measured in the composts applied from 1998 to 2007 (Figure S1). All composts except the one applied in 2000 were below the threshold limit for light plastics and all composts except the one of 2002 were below the threshold limit for heavy plastics (norm NFU 44 051 set up in 2006).
An aliquot of compost was sub-sampled under microscope in order to check for plastic presence. Another aliquot was fractionated according to a protocol developed on sludge compost (Doublet et al., 2010). Such particle–size fractionation was performed using “dry” (sieving of total dry compost) and “wet” (dispersion of compost in water, followed by sieving) fractionation methods. The dry method was performed on 50 g of dried sample (10 days at 40°C). The sample was shaken manually on three different sieves separating the (0–200 μm), (200 μm−5 mm), and (5–20 mm) “dry” fractions. Then, the total dry compost and the “dry” fractions were fractionated using a “wet” fractionation summarized in Figure S2. Due to the nature of the compost, no light fractions (floating in water) was obtained. Finally, five solid fractions were kept in view of their observation and plastic sorting: >5 mm, 2–5 mm, 200 μm−2 mm, 50–200 μm, and < 50 μm.
Soil Fractionation
The separation of water-stable soil fractions was realized according to standard protocol, in order (i) to obtain the particle size distribution of water-stable soil organo-mineral associations and (ii) to separate the different fractions for further analysis (Watteau et al., 2012). We took particular care to preserve initial organo-mineral associations by not drying nor grinding the samples and by not destroying nor chemically extracting soil organic matter.
Granulodensimetric fractions of soil were separated from the < 2-mm soil particles obtained by dry sieving of fresh soil (Figure 1). Thirty-gram samples were dispersed in 200 ml distilled water and stirred gently for 1 h. (200 μm−2 mm) fractions were obtained by wet sieving. Individualized large organic matter as “particulate organic matter” floated during the fractionation and were recovered on the sieve. The 0–200 μm fractions were dispersed for 16 h in 500 ml distilled water. (50–200 μm) fractions were then obtained by wet-sieving. (20–50 μm) fractions, (2–20 μm) fractions and (0–2 μm) fractions were collected by sedimentation using the Robinson pipette method. All the fractions were oven-dried at 60°C and weighed. Weight distributions were adjusted to 100% and weight amounts of the different fractions were expressed as the means associated with their standard errors of three replicates. In order to obtain enough material for further analyses, (0–2 μm) fractions were collected by five serial elutions from < 50 μm fractions without the addition of flocculating agents. From the resulting 2–50 μm residue, (20–50 μm) and (2–20 μm) fractions were collected separately, according to the Stokes's law. Parts of these fractions were oven-dried at 110°C and finely ground for elementary analyses, and the remainders were left undried and stored at 8°C until they were processed for electron microscopy. Organic C contents of all the samples were determined using the dry combustion method (ISO 10694).
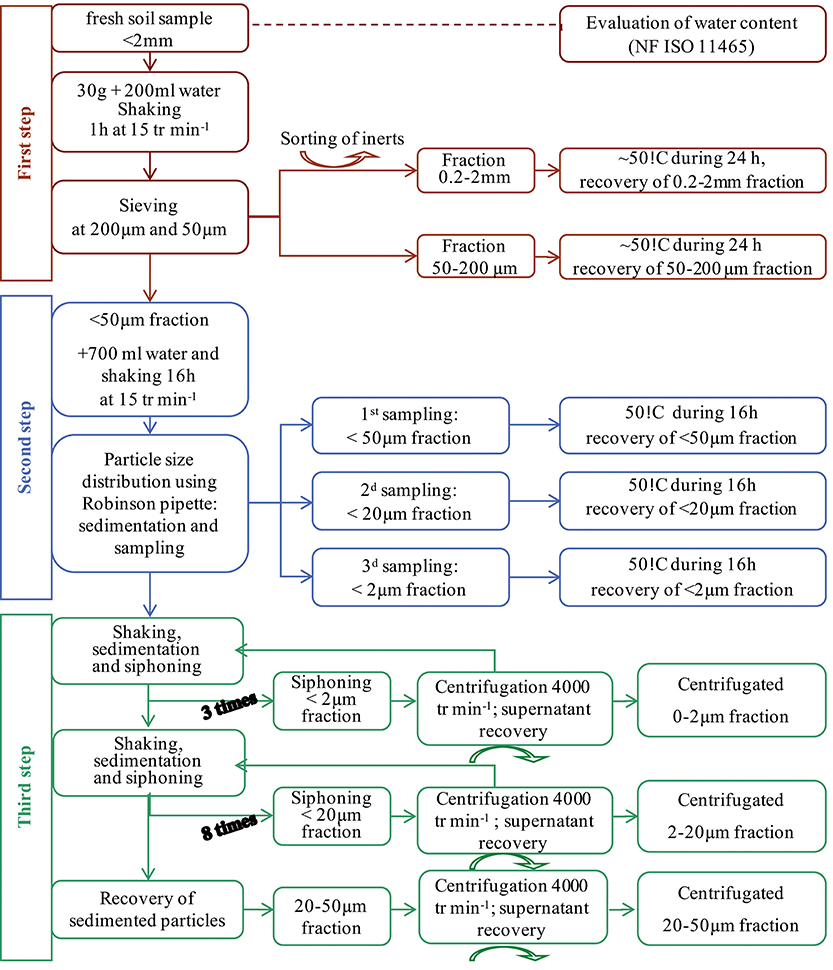
Figure 1. Soil fractionation scheme for the recovery of granulometric fractions and MPs in the solutions of the washing steps.
To complete this fractionation and in order to ensure complete recovery of the plastics present in the liquid fractions, all liquid fractions from the washing steps were recovered (corresponding to the called “supernatant recovery,” step 3, Figure 1) then centrifuged after adding saturated SrCl solution for flocculation. Particles separated by centrifugation were recovered and liquid fractions were filtered at 0.45 μm. Then filters were dried and their surfaces were observed under microscope to pick up all visible particles. Both particles recovered by centrifugation and by 0.45 μm filtration were prepared for TEM characterization. On average, 15 L of water per sample were filtered. These fractions will be, respectively, called “centrifugated” and “filtered” in the following.
MPs Characterization by Transmission Electron Microscopy
Visible MPs identified in the compost and in the coarsest soil fractions were sorted and proceeded for TEM characterization. Particulate organic matter of the coarsest fractions and homogeneous sub-samples of < 50-μm soil fractions resulting from water fractionation were also collected for TEM examination. Thus, visible MPs fragments, coarsest organic matter, and subsamples of the (20–50 μm), (2–20 μm), and (0–2 μm) fractions were fixed in osmium tetroxide by a method that preserves the pre-existing organo-mineral associations (Villemin and Toutain, 1987). Osmium-fixed samples were dehydrated in graded acetone, and embedded in epoxy resin (Epon 812) until complete polymerization (16 h at 60°). Ultra-thin sections (80–100 nm) were cut with a diamond knife under a Leica Ultracut S ultramicrotome. Some sections were filed on Ni grids, stained with uranyl acetate and lead citrate and examined in a JEOL EMXII transmission electron microscope operating at 80 kV. Other ultra-thin sections were filed on Ni-grids covered with a carbon-film, to ensure the EDX analyses (JEOL EXII microscope equipped with a EDAX spectrometer, using Silicon Drift detector) allowing us to realize in situ analyses of the elemental composition.
TEM observations allowed us to obtain information on (i) the origin (plant or microbial), the constitution and the biodegradation state of the organic matter, (ii) the nature of mineral particles, and (iii) their kind of aggregation (Watteau et al., 2012). Unusual features were looked for as being potential plastic residues. The organic or inorganic nature of those unusual features was confirmed using EDX analyses. A minimum of 20 observation fields at a magnification of 4,000 was conducted to highlight MPs presence within soil fractions. Morphological and analytical characterization by TEM/EDX and comparison with the MPs microstructures identified in the initial compost allowed us to confirm or not the presence of MPs in the samples.
MPs Characterization by Py/GC/MS
Bulk soil and soil fractions were analyzed by Py/GC/MS. Approximately 0.5–1 mg of sample was loaded in a quartz tube closed at both ends with quartz wool. The tube, loaded in a CDS Pyroprobe 5,000 Series pyrolysis unit, was heated to 650°C within 0.15 s (30 s hold). A transfer line maintained at 280°C transferred the pyrolysis products to the injector of a Hewlett Packard HP-6890 gas chromatograph (GC), maintained at 280°C and operated in the splitless mode. The pyrolysis products were then separated on a 60 m fused silica capillary SolGelWax column (SGE, 0.32 mm i.d., film thickness 0.5 μm), with helium as carrier gas (1 mL/min). A polar capillary column was chosen for the GC separation because it more accurately separates the polar compounds originating from sugars, proteins and lignins (Dignac et al., 2006). The GC oven was programmed to increase the temperature from 30 to 280°C, at 2°C/min, and the final temperature was maintained for 15 min. The compounds were identified with an HP-5973 Electron ionization mass spectrometer (MS) used in the scan mode (70 eV, scan range m/z 40–700, 1.2 scan/s). Compounds were identified on the basis of their mass spectra, GC retention times, and comparison with the Wiley mass spectra library and with published mass spectra. A number of pyrolysis products were identified for each sample, some of them being specific for a macromolecular source. Peaks were integrated using the HP MS Chemstation (Version C.01.05) on the total ion current trace in triplicate analysis of each sample. All pyrograms are displayed with toluene having the same peak height. The 20–50 μm fractions of the amended and non-amended soils were treated with hydrofluoric acid to concentrate the organic matter before pyrolysis.
Results
MPs in Compost Fractions
Compost Fractions
Compost fractionation was performed in two replicates. The 200–2,000 μm and < 50 μm fractions, respectively, represented one half and one fourth of the compost mass (Table 3). The 2–5 mm fraction was mostly made of some nodules in which plastic fibers were inserted (Figure S3). Plastics as fragments, fibers or membranes of different colors (e.g., white, red, green, blue; see Figure S3) were easily recognizable in the coarsest fractions (> 5 mm, 2–5 mm and 200–2,000 μm) and were sampled for TEM examination.
TEM Examination of Compost MPs
The specific features of the MPs sampled in the compost coarsest fractions, as examined with TEM, evidenced three types of MP structures: membranes, alveolar structures, and thick electron-dense particles (Figure 2). Membrane surfaces appeared either smooth or heterogeneous and sometimes displayed some electron-dense granules. EDX analyses of these granules, revealing a large Ti peak, identified them as titanium oxides (EDX spectrum 1). Other membranes were mainly associated to Ca or to Ba or to minerals as silicates (EDX spectrum 2). EDX analyses of the alveolar structures showed their organic nature without any mineral association, while electron-dense particles were mainly associated to Ti.
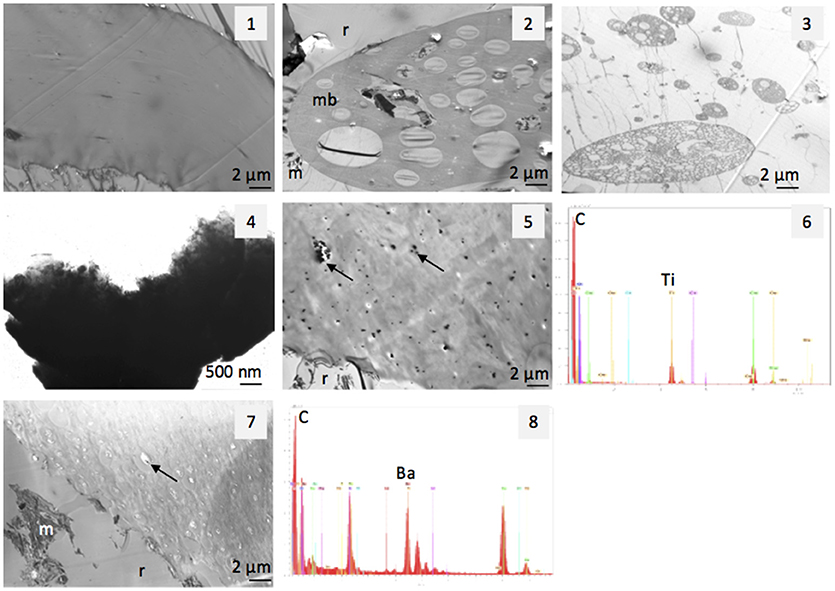
Figure 2. TEM views of MPs in compost–membrane structured (1, 5, 7), alveolar structured (2, 3) and electron-dense (4) and EDX spectra (spectrum 6 and 8 corresponding, respectively, to arrows in views 5 and 7). m, mineral; mb, alveolar membrane; r, resin surrounding the membrane.
TEM observation of the 2–5 mm fraction of the compost showed typical features of organic materials derived from the raw materials, such as cellulosic fibers or plant tissue fragments (Figure 3.1). It also revealed the presence of large amounts of the three types of MPs features, which were frequently observed (Figures 3.2–3.4). MPs fragments with a size comprised between 5 and 30 μm were identified within the other observed constituents of the compost. MPs were also observed in the 200–2,000 μm fraction with however a lower frequency (Figure S4, as an example of a membrane structure). The two finest fractions, i.e., 50–200 μm and < 50 μm, presented themselves as fine powders without any eye-recognizable MPs. Observation of sub-samples of the 50–200 μm showed that they consisted of plant residues, but no MPs were identified by TEM. In the < 50-μm fractions, electron-dense particles containing Ti were characterized, with a size comprised between 4 and 30 μm (Figure S4). These thick particles were often observed as “projected”, i.e., as particles deposited on the thin section and that can hide the rest of the sample. This separation from the resin has probably occurred during the section making.
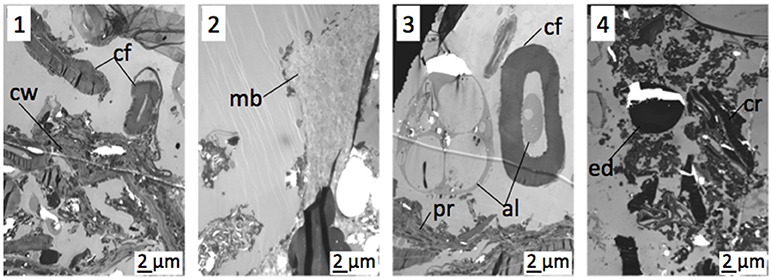
Figure 3. TEM views of 2–5 mm compost fractions, main organic residues (1), with membrane structured MPS (2), with alveolar MPs (3) or with electron-dense particle (4). al, alveolar MPs; cf, cellulosic fiber; cr, cell residue; cw, cell wall; ed, electron-dense MPs; mb, membrane structured MPs; pr, plant residue.
The TEM observations are summarized in Table 4: large MPs were eye-recognizable in the coarsest compost fractions; MPs were identified in all compost fractions except the 50–200 μm one and the observation frequency of < 50-μm MPs decreased with the decreasing size gradient of the fractions.
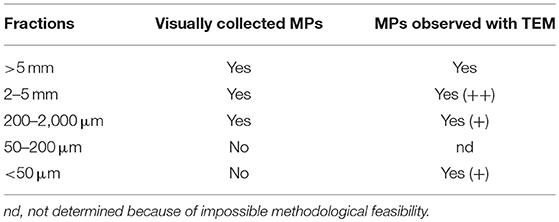
Table 4. identification of the MPs presence in the compost fractions at photonic and electronic scales; “+” as indication of the observation frequency.
MPs in Soil Fractions
Weight Distribution And Carbon Amounts of Soil Granulometric Fractions
The distribution of soil granulometric fractions was in line with the silty loam texture of the soil. The < 50-μm fractions represented 90% of the soil mass and the weight distribution of the water-stable granulometric fractions were similar for both control and amended soils (Table 5). C contents in the control and amended soils were, respectively, of 10.3 and 12.4 g kg−1 soil. C contents in the soil fractions were also slightly larger in the amended soil compared to the control soil (Table 5).

Table 5. Weight distribution of granulometric fractions (g kg−1 soil) expressed as the means of three replicates (standard errors in brackets) and C amounts in the soil fractions (g kg−1 soil).
Plastics in Granulometric Soil Fractions
The MPs fragments sampled in the bulk amended soil and its coarsest fraction (200–2,000 μm) showed the same TEM features and the same elemental compositions as those found in the compost (Figure 2). Nonetheless, associations between alveolar MPs and soil aggregate (Figure 4.1) or bacteria (Figures 4.1–4.3) were observed. TEM observations of the particulate organic matter of the amended soil showed typical plant tissues, on which could be identified “projected” electron-dense MPs associated with Ti (Figure 4.4, EDX spectrum not shown). Such particles were not observed in the coarse organic matter of the control soil. The 20–50 μm soil fractions were made of single mineral or organic particles along with organo-mineral aggregates involving organic matter of plant and microbial origin (Figure 4.5). In the 20–50 μm fraction of the amended soil, only few MPs with a membrane structure associated with Ti were observed within microaggregates (Figure 4.6). In the 2–20 μm amended soil fraction, only few MPs were identified, as “projected” electron-dense particles associated with Ti (Figure 4.7). Finally, no MPs were detected in the 0–2 μm amended soil fraction (Figure 4.8). TEM examination did not reveal MPs presence in any of the control soil fractions.
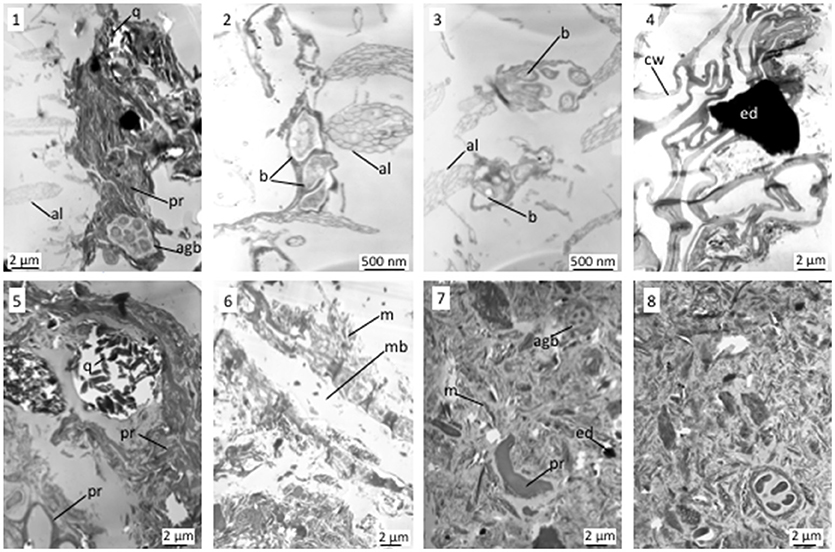
Figure 4. TEM views of granulometric fractions of the amended soil 1–3: alveolar MPs associated with soil aggregate or bacteria; 4: electron-dense MPs projected on plant residue; 5, 6: 20–50 μm organo-mineral fraction and membrane structured MPs associated to organo-mineral fraction (7.6); 7: 2–20 μm fraction showing an electron-dense MPs; 8: 0–2 μm fraction. agb, bacterial aggregate; al, alveolar MPs; b, bacteria; cw, cell wall; ed, electron dense MPs; m, mineral; mb, membrane structured MPs; pr, plant residue; q, quartz.
In summary, MPs were not as frequently observed in the soil fractions as compared to the compost fractions. However, as for the compost, MPs observation frequency decreased as the size of the fraction decreased and few MPs were associated with soil minerals or organic matter.
MPs in the Solutions Recovered During the Washing Steps of the Soil Fractionation
“Centrifugated” and “filtered” water solutions obtained during the recovery of the 20–50 μm, 2–20 μm, and 0–2 μm fractions were examined by TEM (Figure S5). The centrifugated solution of the 20–50 μm fraction of both soils consisted mainly of minerals (e.g., microquartz, alumino-silicates), few organo-mineral aggregates (Figure S5.1) and some individualized organic matter fragments some of which were bacteria. Electron-dense organic particles, from 2 to 20 μm sized and associated with Ti, were also quite frequently identified in this fraction of the amended soil. The same composition was observed for the 20–50 μm filtered solutions but no MPs elemental markers were identified (Figure S5.2). Similar observations were made for the 2–20 μm centrifugated and filtered solutions and only one particle (10 μm) that could be associated with MPs was identified (Figure S5.3). In the 0–2 μm fraction, no MPs marker was identified neither in the centrifugated part nor in the filtered solution, which were both mainly constituted of bacteria (Figure S5.4).
Pyr/GC/MS
The Py/GC/MS total ion current (TIC) traces of amended and non-amended soils and their fractions are compared on Figure 5. The global profiles of the soils were similar, except for the peaks of some aromatic pyrolysis products being larger in the amended compared to the non-amended soil and fractions. Styrene was the only larger peak in amended compared to non-amended samples for bulk soil and 2–20 μm fraction. Styrene and ethyl-benzene were both larger in the 50–200 μm and HF treated 20–50 μm fractions of the amended soil compared to the corresponding fractions of the non-amended soil. In the 200 μm−2 mm and 50–200 um fractions of the amended soil, three peaks were larger for the amended compared to the control soil: styrene, ethyl-benzene and methyl-styrene. Dimer and trimer and other styrene derivatives were observed using their specific ion (m/z 208) only in the 200 μm−2 mm fraction of the amended soil (Figure 5). Other peaks of benzene derivatives (notably ethyl-benzene at 12 min increased in the amended soil compared to the control, particularly in the 200 μm−2 mm fraction.
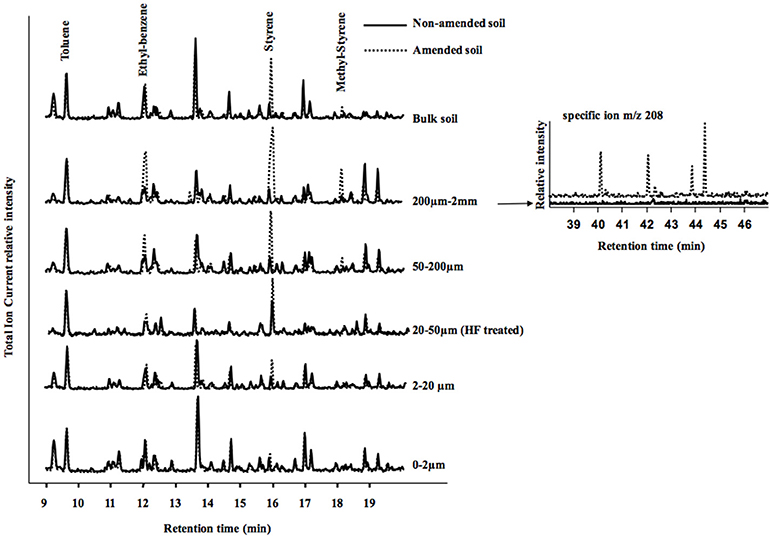
Figure 5. On the left: total Ion Current (TIC) traces of pyrograms in the 9–20 min region showing toluene, styrene and methyl styrene peaks for control and amended soil samples (bulk soil) and their particle size fractions. Pyrograms are presented with the toluene peak at the same height for easier graphical comparison. Fractions 20–50 μm were analyzed after HF treatment. On the right: traces of the ion m/z 208, specific for styrene dimers and trimers (according to Dignac et al., 2005), peaks only detected in the 200 μm−2 mm fractions of the amended soil (compared to the control).
The ratio of the peak areas of toluene and styrene was calculated (Figure 6), since it has been suggested to vary according to the contribution of plastics (Fabbri et al., 1998; Dignac et al., 2005). In the bulk soil as well as in the 2–20 μm, 50–200 μm, and 200 μm−2 mm fractions the toluene/styrene ratio significantly decreased from the control to the amended sample, indicating the presence of plastics in the amended samples. In the bulk soil, the 50–200 μm and 200 μm−2 mm fractions, the Toluene-to-styrene ratio decreased from more than 4 to 1 or less between the control and the amended soil (Figure 6). The lowest ratio was observed for the 200 μm−2 mm fraction of the amended soil. A decrease was also observed for the 2–20 μm fraction and the 0–2 μm fraction, without going below one, and thus it cannot be attributed to the presence of plastics. For the 20–50 μm fraction treated with HF the ratio was already low for the control soil with a slight decrease in the amended soil.
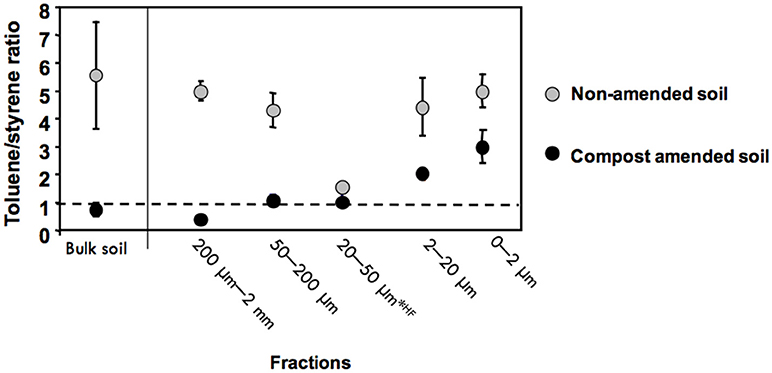
Figure 6. Toluene-to-Styrene ratio in the control and the amended soil and in their fractions (means and error bars from three replicate pyrolysis analysis). Fractions 20–50 μm were analyzed after HF treatment.
Discussion
A New Methodological Approach For MPs Detection in Complex Organo-Mineral Matrices: Relevance of Highlighted MPs Markers
Due to their carbonaceous nature, MPs are difficult to detect in complex organo-mineral samples such as soils (Bläsing and Amelung, 2018). The analytical approach presented here highlighted morphological, analytical and molecular markers of MPs in composts and soils. Identification of MPs was possible among either other organic products or the complex organo-mineral soil matrix. Despite their carbonaceous nature, MPs were detected among other organic particles, either as naked eye recognizable constituents for macro-MPs (>0.2-mm), or thanks to their ultrastructural specific features for micro-MPs (< 50 μm) or their specific elemental association (e.g., Ti), and their molecular constituents in whole samples and fractions.
The Py/GC/MS results were consistent with these TEM morphological and analytical markers. Styrene, toluene and methyl-styrene are unspecific pyrolysis products, originating from natural organic matter such as degraded lignins, proteins and peptides (Fabbri et al., 1998; Dignac et al., 2005), but in samples containing plastics, the peaks of styrene and derivatives might have an additional polystyrene origin. Here, the methyl-styrene peak was not detected in any of the control soil fractions. Its presence in the total amended soil and 200 μm−2 mm, 50–200 μm, and 20–50 μm (Figure 5) suggests the presence of plastics in these samples. The ratio of the peak areas of toluene to styrene is another indication for plastics, since it becomes lower than one with the presence of plastics (Fabbri et al., 1998). The ratios of toluene/ styrene in the pyrolysis of the total amended soil sample and its three coarsest fractions, 200 μm−2 mm, 50–200 μm, and 20–50 μm is another indication of the presence of plastics. It was not methodologically possible to detect MPS in the 50–200 μm by TEM (Table 4), because (i) too large minerals that can damage the diamond knife used for ultra-thin sections are present in this fraction and (ii) any plastics present are too small to be individually sampled under magnifying glass. Otherwise pyrolysis/GC/MS clearly revealed that the 50–200 μm fraction is affected as well by plastics.
The presence of styrene dimer and trimer in the pyrolysates is a further indication of the presence of plastics (Dignac et al., 2005). The 200 μm-2 mm fraction was the only fraction where styrene dimers and trimers were found and thus appeared to be the most affected by the presence of plastics. The 20–50 μm fraction of the amended soil also appears to be affected by plastics, while its C content increases very little compared to the control soil. Although the Toluene/Styrene ratio remained greater than or equal to 1 in the 0–2 and 2–20 μm fractions of the amended soil and methyl styrene was not observed, the slight relative increase in styrene contribution was the only difference between the control and amended soil pyrograms and suggests that organic matter in these fractions could also be affected by plastics.
The TEM as well as the Py/GC/MS methods both have their own limitations. With TEM, the visual characterization of MPs may be operator dependent and may not be completely exhaustive or repeatable between different operators. However, an inter-operator comparison based on a unique and well-established protocol for identifying and counting MPs can remove this bias. On the other hand, styrene and its derivatives monitored with Py/GC/MS might not be representative of all MPs, but only of polystyrene based ones. Further studies are necessary to define molecular markers of most other types of plastics encountered in waste and composts. However, the results of these analytical methods were consistent, both highlighting that the presence of MPs was decreasing according to the decreasing size of the soil fractions. So, both methods corroborated each other. Moreover, the combination of these two analytical methods not only detected MPs presence but also informed about their size and their potential association with the organo-mineral soil matrix. Our results showed the presence of micro-MPs even in 200–2,000 μm soil fractions.
The methods used for compost and soil fractionation were adapted from standard soil fractionation procedures and were consistent with the protocols used in marine research for plastic sorting and identification (Hidalgo-Ruz et al., 2012; Van Cauwenberghe et al., 2015; Mahon et al., 2017). Existing protocols are based on organic matter extraction implying sedimentation, filtration or flocculation. Here, all solid or liquid compartments obtained during fractionation were examined, thus ensuring that all plastics potentially present were taken into account. Furthermore, dispersive treatments are commonly used during the fractionation, e.g., saline solutions or ultrasonic treatment, in order to release plastics embedded in the soil organo-mineral matrix. Our protocol was designed without such dispersion step, preserving the initial organo-mineral associations in order to highlight the possible incorporation of micro(nano)plastics within aggregates. This protocol also avoids the artificial formation of smaller plastic residues as it was observed in sediments (Löder and Gerdts, 2015).
In our fractionation protocol, we propose to analyze the solutions obtained during the different washing steps. The results obtained on these liquid fractions were consistent with those on the solid fractions, suggesting that the time-consuming step of recovery and analysis of the washing solutions could be avoided. However, for a better consideration of MPs diversity in nature and reactivity, we suggest to collect and analyze all the compartments.
Since the fractionation protocol was based on density separation, all MPs morphological types were encountered (e.g. fibers, films, spheres, more or less fragmented particles). However, the size of the collected MPs did not always correspond to the studied fraction (e.g., 10 μm electron-dense particle were found in the 0–2 μm fraction or inversely 5 μm sized MPs in the coarsest fractions). Measuring MPs size is thus of interest and the use of TEM relevant. Further development of automatic image analysis protocols should improve MPs size measurements. Fourier transformed infrared (FT-IR) is usually used for plastic identification, nonetheless presence of organic matter may make the result interpretation difficult (Rocha-Santos and Duarte, 2015). Once the fractions are obtained with the protocol used here, they can be analyzed with such complementary techniques.
When MPs were too small to be morphologically identified, our analytical approach was able to detect them, thanks to their association with elements such as Ti or Ba, which appeared as relevant analytical markers allowing to differentiate MPs from other organic matter. The chemical markers obtained with Py/GC/MS analysis are also independent of the MPs size and visual observation. Ti oxide can be added to MPs as inorganic white pigment and UV blocker (Wang et al., 2017) and Ba sulfate associated with nonylphenol as heat stabilizer or as fillers (Hahladakis et al., 2018). Other elements such Cd, Pb, and Cu are also found in plastics (Imhof et al., 2016). Measuring the Ti amounts in total soil fractions could be an interesting perspective to highlight the plastic presence, as far as the geochemical content of the soil in Ti is not too high. As plastics are more concentrated in composts than in soils, evaluation of Ti contents as a MPs tracer could be still more accurate in composts compared to soils.
In summary, our analytical approach, combining granulo-densimetric fractionation, TEM observation, EDX analysis and Py-GC-MS characterization was able to identify MPs in compost and soil, as well as estimate their contribution and specify their size and their potential input of inorganic elements. Further studies may investigate the detection limits of such analyses in order to test their relevance when lesser amounts of composts are applied. Moreover, one data base, constituted of TEM features and pyrograms specific to different types of plastics, could be realized in order to conduct more generic researches. This protocol could also be improved and completed with a quantitative analysis in order to compare different samples for temporal monitoring and study of the fate (e.g. degradation, fragmentation) of plastics in soil compartments.
MPs Evolution Within Soil Matrix
The question of plastic degradation in marine environment has been addressed by many studies. It was suggested that plastics are mainly degraded in water due to UV radiation and physical abrasion (Barnes et al., 2009; Song et al., 2017). Both processes might be limited in soils where plastic degradation might thus be very slow (Krueger et al., 2015). Our results showed that the luvisol, which was amended for 10 years with large doses (2 to 4 time the French classical practices) of MSW compost, presented more plastics and microplastics than the control luvisol. The size of identified MPs ranged from few mm up to a few micrometers. Since MPs were isolated based on a granulo-densimetric fractionation, some small MPs could be detected in the coarsest soil fractions. MPs observation frequency and styrene/toluene pyrolysis ratio showed that MPs abundance decreased as the size of the soil fractions decreased. However, they were identified even in the small, potentially stable fractions of the soil, down to 200–2,000 μm with TEM and 50–200 μm with pyrolysis.
Some plastics might be subject to microbial attack, by fungi (Khan et al., 2017) or bacteria (Rummel et al., 2017). Here associations between alveolar MPs and bacteria have been highlighted, suggesting a possible biodegradation. Moreover, we did not observe alveolar structures in thinnest soil fractions, suggesting that these structures are more degradable, this being confirmed by the TEM observation of alveolar MPs-bacteria associations in the coarsest soil fractions. Those specific structures of MPs could be assimilated to those previously observed using scanning electron microscopy and described as indices of degradation (Wang et al., 2017). We should check if such features exist or not in newly produced MPs to verify if it represents any degradation features. MPs have hydrophobic surfaces that stimulate rapid biofilm formation, e.g., < 1 week on MPs in water or in contact with sediments (Zettler et al., 2013). Our fractionation protocol should not break down water-stable biofilms within soil aggregates (Watteau et al., 2012) and only few observations highlighted some bacteria in contact with MPs-membrane type, even if no typical association between microorganisms and MPs involving exopolymers as adhering agents was observed. The physico-chemical conditions of the studied soil do not seem as favorable as those of aquatic environments for the formation of stable biofilms. Specific monitoring of the fate in soils of biodegradable or bio-based plastics should facilitate the identification of the role of soil microorganisms in the MPs degradation.
We did not notice any obvious degradation features such as microbial lysis, and we thus suggest that MPs evolution in soil was mostly due to fragmentation. However, we also observed micro- and nano-MPs in composts, which should be considered when evaluating MPs fragmentation in soils. Finally, the smallest detected MPs could result from the evolution of larger plastics in soil, e.g. impact of physical environmental conditions or soil plowing, or to the compost input on soil, e.g. compost manufacturing and incorporation within soil. Monitoring the MPs presence in soil over time using the presented approach could distinguish the origin of MPs fragmentation.
MPs input in soil was also reflected by the presence of nanoparticles. Membrane structured and electron-dense particles of MPs were associated with inorganic elements, in particular Ti as TiO2. We can wonder about the availability of such associated elements as MPs were degraded. TiO2 could be released when the composites degrade in the environment, and thus MPs act as vectors for TiO2. More, TiO2 in marine ecosystems would be toxic to bacteria, algae, invertebrates and fishes (Handy et al., 2008) and Ti association with plastics could affect their mobility in soils by modification of their physico-chemical properties. It was recently suggested that nanoparticles such as TiO2 can be retained in the soil (Pachapur et al., 2016). Here the few EDX analyses carried out on the soil organic matter did not show any Ti transfer (results not presented), but more investigations have to be realized to ensure that no Ti or some other metals were adsorbed on organic matter or ingested by living organisms.
Observed MPs were little associated within soil matrix and seemed to persist as individualized particles. Few associations between MPs and soil organic matter or minerals were highlighted in this study and further analyses have to be investigated in different agrosystems, in order to confirm or not this result as a generic one. From researches in marine ecosystems, it was hypothesized that, due to their small sizes, MPs in the water column will behave in the same way as sediment particles (Browne et al., 2010). Yet, no clear relationship was observed between MPs (< 1 mm) abundance and the proportion of clay in the sediment (Browne et al., 2010). It was therefore argued that other processes such as aggregation with organic material might play a more important role in the movement of MPs. Indeed, Long et al. (2015) demonstrated in a laboratory study that different algae species (Chaetoceros neogracile and Rhodomonas salina) incorporate and concentrate MPs, substantially increasing microplastic sinking rates. Moreover, Strand et al. (2013) demonstrated that there is a strong relationship between MPs abundance and both organic (%TOC) and fine fraction (< 63 μm) content in sediments, supporting the hypothesis that MPs will accumulate in depositional areas. Aggregation with organic matter was also considered the main route of transport for MPs to deep-sea sediments. MPs are our newest emerging contaminants (Browne et al., 2007; Sedlak, 2017) and agrosystems appears as a major entry point for MPs in continental systems (Nizzetto et al., 2016b). Plastics, although made of C, behave very differently from natural organic matter in soils (Rillig, 2018) and their input and fate urgently needs research (Rillig et al., 2017). Impacts of soil texture, soil organic matter pool, MPs type (i.e. biodegradable or not), agricultural practices, biological activity on plastic fate have to be investigated. The combined approach presented here can help to specify the dynamics of interactions of MPs with soil aggregates. Obtained data will contribute to specify in particular the accumulation rate of MPs in soils according to soil use, their potential toxicity due to their organic pollutant constituents and input of inorganic elements (i.e. Ti), or their behavior in soils over time.
Conclusion
Identification and quantification of MPs in soils is a crucial step to overcome in order to specify the MPs fate in soil and thus their potential impact on soil health. Combining TEM and Py/GC/MS allows to identify and localize some MPs within soil fractions. Such monitoring over time should be used to specify the contribution of urban composts to MPs pool in soil and their impact on the quality of the different compartments of the agroecosystems, i.e., plant, fauna, soil aggregates, soil water. Accumulation of MPs following 10 years of large applications of standard municipal waste compost was evidenced in a loamy soil and in almost all its size fractions, down to 50 μm. Further investigations are needed to specify the rate of this accumulation, as well as the possibly low MPs incorporation in stabilized fractions. Expected results of such future works will help the more accurate characterization of the risks and environmental impact of soil management practices. They also may contribute to improve ways and rules concerning waste management practices, having in mind to supply composts with high agronomic value and high environmental quality.
Author contributions
FW and SH designed the research. FW, M-FD, and AB realized the analyses and produced the data. FW prepared the first draft of the manuscript and all authors contributed to the writing of the final version of the manuscript.
Conflict of interest statement
The authors declare that the research was conducted in the absence of any commercial or financial relationships that could be construed as a potential conflict of interest.
Acknowledgments
We thank V. Mercier (ECOSYS) for conducting the experimental site, M. Joly (VERI) for realizing the compost fractionation and M. Klein (LSE) for part of the soil fractionation and plastic sorting.
Supplementary material
The Supplementary Material for this article can be found online at: https://www.frontiersin.org/articles/10.3389/fsufs.2018.00081/full#supplementary-material
Figure S1. Plastic contents (% dry matter) in the composts applied every year on the experimental field compared to the French thresholds for heavy and light plastics in composts set up in 2006 (NFU 44 051).
Figure S2. Fractionation scheme for isolating particle-size fractions of the compost (from Doublet et al., 2010).
Figure S3. Nodule (1) and MPs observed in the compost. With view (2) as a whole view of individualized MPs corresponding to views (3–10).
Figure S4. TEM views of < 2 mm compost fractions, 5: 200–2,000 μm fraction (1), 50–200 μm fraction (2, 3); < 50 μm fraction (4). cf, cellulosic fiber; cr, cell residue; cw, cell wall; ed, electron-dense MPs; h, hole due to resin disruption; mb, membrane structured MPs.
Figure S5. TEM views of the washed solutions recovered during the washing steps of the soil fractionation. 1, aggregate of the centrifugated 20–50 μm fraction; 2, filtered 20–50 μm fraction; 3, centrifugated 2–20 μm fraction; 4, filtered 0–2 μm fraction. b, bacteria; m, mineral; MPs, microplastic; om, organic matter; pr, plant residue; q, quartz.
References
Aggelides, S. M., and Londra, P. A. (2000). Effects of compost produced from town wastes and sewage sludge on the physical properties of a loamy and a clay soil. Bioresour. Technol. 71, 253–259. doi: 10.1016/S0960-8524(99)00074-7
Andrady, A. L. (2011). Microplastics in the marine environment. Mar. Poll. Bull. 62, 1596–1605. doi: 10.1016/j.marpolbul.2011.05.030
Bandmann, V., Müller, J. D., Köhler, T., and Homann, U. (2012). Uptake of fluorescent nano beads into BY2-cells involves clathrin-dependent and clathrin-independent endocytosis. FEBS Lett. 586, 3626–3632. doi: 10.1016/j.febslet.2012.08.008
Barnes, D. K., Galgani, F., Thompson, R. C., and Barlaz, M. (2009). Accumulation and fragmentation of plastic debris in global environments. Philos. Transac. R. Soc. B 364, 1985–1998. doi: 10.1098/rstb.2008.0205
Bläsing, M., and Amelung, W. (2018). Plastics in soil: analytical methods and possible sources. Sci. Tot. Envir. 612, 422–433. doi: 10.1016/j.scitotenv.2017.08.086
Briassoulis, D., and Dejean, C. (2010). Critical review of norms and standards for biodegradable agricultural plastics. Part I. Biodegradation in soil. J. Polym. Environ. 8, 384–400. doi: 10.1007/s10924-010-0168-1
Briassoulis, D., Dejean, C., and Picuno, P. (2010). Critical review of norms and standards for biodegradable agricultural plastics. Part II. Composting. J. Polym. Environ. 18, 364–383. doi: 10.1007/s10924-010-0222-z
Brinton, Jr. W. F. (2005). Characterization of man-made foreign matter and its presence in multiple size fractions from mixed waste composting. Compost Sci. Util. 13, 274–280. doi: 10.1080/1065657X.2005.10702251
Browne, M. A., Galloway, T., and Thompson, R. (2007). Microplastic an emerging contaminant of potential concern? Integr. Environ. Assess. Manage. 3, 559–561. doi: 10.1002/ieam.5630030412
Browne, M. A., Galloway, T. S., and Thompson, R. C. (2010). Spatial patterns of plastic debris along estuarine shorelines. Environ. Sci. Technol. 44, 3404–3409. doi: 10.1021/es903784e
De Souza Machado, A. A., Kloas, W., Zarfl, C., Hempel, S., and Rillig, M. C. (2018). Microplastics as an emerging threat to terrestrial ecosystems. Global Change Biol. 24, 1405–1416. doi: 10.1111/gcb.14020
Dignac, M. F., Houot, S., and Derenne, S. (2006). How the polarity of the separation column may influence the characterization of compost organic matter by pyrolysis-GC/MS. J. Analyt. Appl. Pyrolysis 75, 128–139. doi: 10.1016/j.jaap.2005.05.001
Dignac, M. F., Houot, S., Francou, C., and Derenne, S. (2005). Pyrolytic study of compost and waste organic matter. Organic Geochem. 36, 1054–1071. doi: 10.1016/j.orggeochem.2005.02.007
Doublet, J., Francou, C., Pétraud, J. P., Dignac, M. F., Poitrenaud, M., and Houot, S. (2010). Distribution of C and N mineralization of a sludge compost within particle-size fractions. Bioresour. Technol. 101, 1254–1262. doi: 10.1016/j.biortech.2009.09.037
Dris, R., Gasperi, J., Rocher, V., Saad, M., Renault, N., and Tassin, B. (2015). Microplastic contamination in an urban area: a case study in Greater Paris. Environ. Chem. 12, 592–599. doi: 10.1071/EN14167
Dümichen, E., Eisentraut, P., Bannick, C. G., Barthel, A. K., Senz, R., and Braun, U. (2017). Fast identification of microplastics in complex environmental samples by a thermal degradation method. Chemosphere 174, 572–584. doi: 10.1016/j.chemosphere.2017.02.010
Fabbri, D., Trombini, C., and Vassura, I. (1998). Analysis of Polystyrene in polluted sediments by pyrolysis-gas chromatography-mass spectrometry. J. Chromatogr. Sci. 36:12. doi: 10.1093/chromsci/36.12.600
Fuller, S., and Gautam, A. (2016). A procedure for measuring microplastics using pressurized fluid extraction. Environ. Sci. Technol. 50, 5774–5780. doi: 10.1021/acs.est.6b00816
Galgani, F., Hanke, G., and Maes, T. (2015). “Global distribution, composition and abundance of marine litter,” in Marine Anthropogenic Litter, eds M. Bergmann, L. Gutow, and M. Klages (Cham: Springer), 29–56. doi: 10.1007/978-3-319-16510-3_2
Hahladakis, J. N., Velis, C. A., Weber, R., Iacovidou, E., and Purnell, P. (2018). An overview of chemical additives present in plastics: migration, release, fate and environmental impact during their use, disposal and recycling. J. Hazardous Mat. 344, 179–199. doi: 10.1016/j.jhazmat.2017.10.014
Handy, R. D., Henry, T. B., Scown, T. M., Johnston, B. D., and Tyler, C. R. (2008). Manufactured nanoparticles: their uptake and effects on fish–a mechanistic analysis. Ecotoxicology 17, 396–409. doi: 10.1007/s10646-008-0205-1
Hernandez, E., Nowack, B., and Mitrano, D. M. (2017). Polyester textiles as a source of microplastics from households: a mechanistic study to understand microfiber release during washing. Envir. Sci.Technol. 51, 7036–7046. doi: 10.1021/acs.est.7b01750
Hidalgo-Ruz, V., Gutow, L., Thompson, R. C., and Thiel, M. (2012). Microplastics in the marine environment: a review of the methods used for identification and quantification. Environ. Sci. Technol. 46, 3060–3075. doi: 10.1021/es2031505
Horton, A. A., Walton, A., Spurgeaon, D. J., Lahive, E., and Svendsen, C. (2017). Microplastics in freshwater and terrestrial environments: evaluating the current understanding to identify the knowledge gaps and future research priorities. Sci. Tot. Envir. 586, 127–141. doi: 10.1016/j.scitotenv.2017.01.190
Huerta Lwanga, E., Gertsen, H., Gooren, H., Peters, P., Salánki, T., van der Ploeg, M., et al. (2016). Microplastics in the terrestrial ecosystem: implications for Lumbricus terrestris (Oligochaeta, Lumbricidae). Environ. Sci. Technol. 50, 2685–2691. doi: 10.1021/acs.est.5b05478
Huerta Lwanga, E. H., Gertsen, H., Gooren, H., Peters, P., Salánki, T., van der Ploeg, M., et al. (2017). Incorporation of microplastics from litter into burrows of Lumbricus terrestris. Environ. Pollut. 220, 523–531. doi: 10.1016/j.envpol.2016.09.096
Hurley, R. R., and Nizzetto, L. (2018). Fate and occurrence of micro(nano)plastics in soils: knowledge gaps and possible risks. Curr. Opin. Environ. Sci. Health 1, 6–11. doi: 10.1016/j.coesh.2017.10.006
Imhof, H. K., Laforsch, C., Wiesheu, A. C., Schmid, J., Anger, P. M., Niessner, R., et al. (2016). Pigments and plastic in limnetic ecosystems: a qualitative and quantitative study on microparticles of different size classes. Water Res. 98, 64–74. doi: 10.1016/j.watres.2016.03.015
Khan, S., Nadir, S., Shah, Z. U., Shah, A. A., Karunarathna, S. C., Xu, J., et al. (2017). Biodegradation of polyester polyurethane by Aspergillus tubingensis. Environ. Pollut. 225, 469–480. doi: 10.1016/j.envpol.2017.03.012
Krueger, M. C., Harms, H., and Schlosser, D. (2015). Prospects for microbiological solutions to environmental pollution with plastics. Appl. Microbiol. Biotechnol. 21, 8857–8874. doi: 10.1007/s00253-015-6879-4
Leejarkpai, T., Suwanmanee, U., Rudeekit, Y., and Mungcharoren, T. (2011). Biodegradable kinetics of plastics under controlled composting conditions. Waste Manag. 31, 1153–1161. doi: 10.1016/j.wasman.2010.12.011
Liu, G., Li, Y. F., Yan, F. Y., Zhao, Z. X., Zhou, L. C., and Xue, Q. J. (2005). Effect of nanoscale SiO2 and TiO2 as the fillers of the mechanical properties and aging behavior of linear low-density polyethylene/low density polyethylene blends. J. Polym. Environ. 13, 339–348. doi: 10.1007/s10924-005-5528-x
Löder, M. G. J., and Gerdts, G. (2015). “Methodology Used for the Detection and Identification of Microplastics—A Critical Appraisal,” in Marine Anthropogenic Litter, eds M. Bergmann, L. Gutow, and M. Klages (Cham: Springer), 201–227. doi: 10.1007/978-3-319-16510-3_8
Long, M., Moriceau, B., Gallinari, M., Lambert, C., Huvet, A., Raffray, J., et al. (2015). Interactions between microplastics and phytoplankton aggregates: impact on their respective fates. Marine Chem. 175, 39–46. doi: 10.1016/j.marchem.2015.04.003
Luckachan, G., and Pillai, C. K. S. (2011). Biodegradable polymers – A review on recent trends and emerging perspectives. J. Polym. Environ. 19, 637–676. doi: 10.1007/s10924-011-0317-1
Lusher, A. (2015). “Microplastics in the marine environment: distribution, interactions and effects,” in Marine Anthropogenic Litter, eds M. Bergmann, L. Gutow, and M. Klages (Cham: Springer International Publishing), 245–307. doi: 10.1007/978-3-319-16510-3_10
Maaß, S., Daphi, D., Lehmann, A., and Rillig, M. C. (2017). Transport of microplastics by two collembolan species. Environ. Pollut. 225, 456–459. doi: 10.1016/j.envpol.2017.03.009
Mahon, A. M., O'Connell, B., Healy, M. G., O'Connor, I., Officer, R., Nash, R., and Morrison, L. (2017). Microplastics in sewage sludge: effects of treatment. Environ. Sci. Technol 51, 810–818. doi: 10.1021/acs.est.6b04048
Nizzetto, L., Bussi, G., Futter, M. N., Butterfield, D., and Whitehead, P. G. (2016a). A theoretical assessment of microplastic transport in river catchments and their retention by soils and river sediments. Environ. Sci. Process Impacts 18, 1050–1059. doi: 10.1039/C6EM00206D.
Nizzetto, L., Futter, M., and Langaas, S. (2016b). Are agricultural soils dumps for microplastics of urban origin? Environ. Sci. Technol. 50, 10777–11077. doi: 10.1021/acs.est.6b04140
Orbit, (2008). Compost Production and Use in the EU. Available online at: http://susproc.jrc.ec.europa.eu/activities/waste/documents/080229_EoW_final-report_v1.0.pdf.
Pachapur, V. L., Dalila Larios, A., Cledón, M., Brar, S. K., Verma, M., and Surampalli, R. Y. (2016). Behavior and characterization of titanium dioxide and silver nanoparticles in soils. Sci. Total Environ. 563, 933–943. doi: 10.1016/j.scitotenv.2015.11.090
Peltre, C., Christensen, B. T., Dragon, S., Icard, C., Kätterer, T., and Houot, S. (2012). RothC simulation of carbon accumulation in soil after repeated application of widely different organic amendments. Soil Biol. Biochem. 52, 49–60. doi: 10.1016/j.soilbio.2012.03.023
Phuong, N. N., Zalouk-Vergnoux, A., Poirier, L., Kamari, A., Châtel, A., Mouneyrac, C., et al. (2016). Is there any consistency between the microplastics found in the field and those used in laboratory experiments? Environ. Pollut. 211, 111–123. doi: 10.1016/j.envpol.2015.12.035
Référentiel Pédologique, (2008). Association Française Pour L'étude des Sols. Baize and Girard, coordinators. Ed. Quae.
Rillig, M. C. (2012). Microplastic in terrestrial ecosystems and the soil? Environ. Sci. Technol. 46, 6453–6454. doi: 10.1021/es302011r
Rillig, M. C. (2018). Microplastic disguising as soil carbon storage. Environ. Sci. Technol. 52, 6079–6080. doi: 10.1021/acs.est.8b02338
Rillig, M. C., Ingraffia, R., and de Souza Machado, A. A. (2017). Microplastic incorporation into soil in agroecosystems. Front. Plant Sci. 8:1805. doi: 10.3389/fpls.2017.01805
Rocha-Santos, T., and Duarte, A. C. (2015). A critical overview of the analytical approaches to the occurrence, the fate and the behavior of microplastics in the environment. Trends Anal. Chem. 65, 47–53. doi: 10.1016/j.trac.2014.10.011
Rummel, C. D., Jahnke, A., Gorokhova, E., Kühnel, D., and Schmitt-Jansen, M. (2017). Impacts of biofilm formation on the fate and potential effects of microplastic in the aquatic environment. Environ. Sci. Technol. Lett. 4, 258–267. doi: 10.1021/acs.estlett.7b00164
Schwab, F., Zhai, G., Kern, M., Turner, A., Schnoor, J. L., and Wiesner, M. R. (2016). Barriers, pathways and processes for uptake, translocation and accumulation of nanomaterials in plants – critical review. Nanotoxicology 10, 257–278. doi: 10.3109/17435390.2015
Sedlak, D. (2017). Three lessons for the microplastics voyage. Environ. Sci. Technol. 51, 7747–7748. doi: 10.1021/acs.est.7b03340
Smith, W. H. (1996). “Utilizing composts in land management to recycle organics,” in The Science of Composting eds M. De Bertoldi, P. Sequi, B. Lemmes, and T. Papi (Glasgow: Blackie Academic & Professional), 413–422. doi: 10.1007/978-94-009-1569-5_39
Song, Y. K., Hong, S. H., Jang, M., Han, G. M., Jung, S. W., and Shim, W. J. (2017). Combined effects of UV exposure duration and mechanical abrasion on microplastic fragmentation by polymer type. Environ. Sci. Technol. 8, 4368–4376. doi: 10.1021/acs.est.6b06155
Steinmetz, Z., Wollmann, C., Schaefer, M., Buchmann, C., David, J., Tröger, J., et al. (2016). Plastic mulching in agriculture. Trading short-term agronomic benefits for long-term soil degradation? Sci. Total Environ. 550, 690–705. doi: 10.1016/j.scitotenv.2016.01.153
Strand, J., Lassen, P., Shashoua, Y., and Andersen, J. H. (2013). Microplastic Particles in Sediments from Danish Waters. Poster at the ICES Annual Conference, Reykjavik, Iceland.
Thompson, R. C. (2015). “Microplastics in the Marine Environment: Sources, Consequences and Solutions,” in Marine Anthropogenic Litter, eds M. Bergmann, L. Gutow, and M. Klages (Cham: Springer), 185–200. doi: 10.1007/978-3-319-16510-3_7
Van Cauwenberghe, L., Devriese, L., Galgani, F., Robbens, J., and Janssen, C. R. (2015). Microplastics in sediments: a review of techniques, occurrence and effects. Mar. Environ. Res. 111, 5–17. doi: 10.1016/j.marenvres.2015.06.007
Vegter, A., Barletta, M., Beck, C., Borrero, J., Burton, H., Campbell, M., et al. (2014). Global research priorities to mitigate plastic pollution impacts on marine wildlife. Endang. Species Res. 25, 225–247. doi: 10.3354/esr00623
Villemin, G., and Toutain, F. (1987). “Method to stabilize organomineral soil samples for transmission microscopy,” in Soil Micromorphology, Proceedings of the VIIth International Working Meeting on Soil Micromorphology, eds N. Fedoroff, L. M. Bresson, and M. A. Courty (Wroclaw), 43–48.
Wang, J., Peng, J., Tan, Z., Gao, Y., Zhan, Z., Chen, Q., et al. (2017). Microplastics in the surface sediments from the Beijiang River littoral zone: composition, abundance, surface textures and interaction with heavy metals. Chemosphere 171, 248–258. doi: 10.1016/j.chemosphere.2016.12.074
Watteau, F., Villemin, G., Bartoli, F., Schwartz, C., and Morel, J. L. (2012). 0-20 μm aggregate typology based on the nature of aggregative organic materials in a cultivated silty topsoil. Soil Biol. Biochem. 4, 103–114.doi: 10.1016/j.soilbio.2011.11.021
Weber, J., Karczewska, A., Drozd, J., Licznar, M., Licznar, S., Jamroz, E., et al. (2007). Agricultural and ecological aspects of a sandy soil as affected by the application of municipal solid waste composts. Soil Biol. Biochem. 39, 1294–1302. doi: 10.1016/j.soilbio.2006.12.005
Weithmann, N., Möller, J. N., Löder, M. G. J., Piehl, S., Laforsch, C., and Freitag, R. (2018). Organic fertilizer as a vehicle for the entry of microplastic into the environment. Sci. Adv. 4:eaap8060. doi: 10.1126/sciadv.aap8060
Wright, S. L., Thompson, R. C., and Galloway, T. S. (2013). The physical impacts of microplastics on marine organisms: a review. Environ. Pollut. 178, 483–492. doi: 10.1016/j.envpol.2013.02.031
Zettler, E. R., Mincer, T. J., and Amaral-Zettler, L. A. (2013). Life in the “Plastisphere”: microbial communities on plastic marine debris. Environ. Sci. Technol. 47, 7137–7146. doi: 10.1021/es401288x
Keywords: plastic indicators, soil microaggregates, soil fractionation, Ti, contaminants, styrene
Citation: Watteau F, Dignac M-F, Bouchard A, Revallier A and Houot S (2018) Microplastic Detection in Soil Amended With Municipal Solid Waste Composts as Revealed by Transmission Electronic Microscopy and Pyrolysis/GC/MS. Front. Sustain. Food Syst. 2:81. doi: 10.3389/fsufs.2018.00081
Received: 13 June 2018; Accepted: 20 November 2018;
Published: 11 December 2018.
Edited by:
Raul Moral, Universidad Miguel Hernández de Elche, SpainReviewed by:
Jose Antonio Pascual, Spanish National Research Council (CSIC), SpainTeresa A. P. Rocha-Santos, University of Aveiro, Portugal
Copyright © 2018 Watteau, Dignac, Bouchard, Revallier and Houot. This is an open-access article distributed under the terms of the Creative Commons Attribution License (CC BY). The use, distribution or reproduction in other forums is permitted, provided the original author(s) and the copyright owner(s) are credited and that the original publication in this journal is cited, in accordance with accepted academic practice. No use, distribution or reproduction is permitted which does not comply with these terms.
*Correspondence: Francoise Watteau, ZnJhbmNvaXNlLndhdHRlYXVAdW5pdi1sb3JyYWluZS5mcg==
Agathe Revallier, YWdhdGhlLnJldmFsbGllckB2ZW9saWEuY29t
Sabine Houot, c2FiaW5lLmhvdW90QGlucmEuZnI=