Spatial Arrangement and Biofertilizers Enhance the Performance of Legume—Millet Intercropping System in Rainfed Areas of Southern India
- 1Department of Environmental Sciences—Botany, University of Basel, Basel, Switzerland
- 2Programme Area Ecotechnology, M S Swaminathan Research Foundation, Chennai, India
- 3Programme Area Biotechnology, M S Swaminathan Research Foundation, Chennai, India
- 4Programme Area Biodiversity, M S Swaminathan Research Foundation, Chennai, India
- 5Dryland Agriculture Project, University of Agricultural Sciences, GKVK, Bengaluru, India
- 6Centre for Natural Biological Resources and Community Development, Bengaluru, India
- 7Department of Soil Sciences, Research Institute of Organic Agriculture (FiBL), Frick, Switzerland
Intercropping is a well-established practice to enhance the yield in low-input agriculture, and beneficial microbes such as arbuscular mycorrhizal fungi (AMF) combined with plant growth promoting rhizobacteria are being used as an effective and sustainable measure to improve yields. In this study, we tested if biofertilizers can not only enhance the yield of crops in monoculture as has previously been demonstrated but can also enhance the yield of intercropping systems. We hypothesized that because AMF can form common mycorrhizal networks (CMN) that can transfer nutrients and water between different plant species, biofertilization can balance belowground competition between crop species and promote thus overall yields in intercropping systems. In our study, we used a pigeon pea (PP)—finger millet (FM) intercropping system that we grew for two consecutive growing seasons (2016/17 and 2017/18) at two contrasting sites in Bengaluru and Kolli Hills, India. We also tested if the spatial arrangement (i.e., different arrangement of component plants with similar plant density in intercropping system) of intercropped plants, using either a row-wise or a mosaic design, influences the effect of biofertilizers on yield and water relations of the PP-FM intercropping system. Our results demonstrate that intercropping can improve the straw and grain yield of PP and FM compared to the respective monocultures and that intercropping effects vary depending on the site characteristic such as climate and soil type. The spatial arrangement of component plants affected the total, straw, and grain biomass in intercropping treatments, but this effect also varied across sites. Most importantly, the results from the 2017/18 growing season clearly demonstrated a positive effect of biofertilizer on biomass yield, and this effect was irrespective of site, spatial arrangement, mixed or monoculture. Our study therefore shows that yield increase in intercropping systems can further be improved through the application of biofertilizers.
Introduction
Intercropping has been considered a sustainable way to utilize and share natural resources among different crop species and to improve and stabilize crop yield (Brooker et al., 2015; Martin-Guay et al., 2018). In intercropping systems two or more crop species are grown together (Vandermeer, 1989). Crop yield in intercropping systems are often higher than in monocropping systems because resources such as soil moisture and nutrients are utilized more efficiently in intercropping than in monocropping (Lithourgidis et al., 2007; Dahmardeh et al., 2009; Martin-Guay et al., 2018). This is because interspecific competition between intercropping partners is often lower than the intraspecific competition in monocropping, resulting in a yield advantage (Davis and Woolley, 1993). In addition, beneficial effects of intercropping can come from resource facilitation: As an example, legume–cereal intercropping systems have been widely used in areas with poor soil quality (Li et al., 2007), where legumes fix nitrogen (N) and solubilize phosphorus (P) which is then used by both intercropping partners (Hinsinger et al., 2011). In return, cereals can support legumes in two ways, by preventing nitrate-N accumulation in soil which inhibits N fixation by legumes, and by increasing iron availability which enhances N fixation (Zuo et al., 2004; Schipanski and Drinkwater, 2012).
In rainfed areas of the arid and semiarid tropics, intercropping has also been suggested to enhance the water availability of shallow-rooted crops via the facilitation of water supply by deep-rooted plants through hydraulic lift (HL) (Xu et al., 2008; Mao et al., 2012). The water released from deep-rooted plants due to HL into topsoil layer becomes available to neighboring shallow-rooted plants, a process termed “bioirrigation” (Burgess, 2011). The functionality of bioirrigation in intercropping systems has only been tested in a few studies—mainly under controlled conditions in the greenhouse. Sekiya and Yano (2002) showed in a field experiment that pigeon pea (a deep-rooted legume) has the potential to perform HL and could supply deep water to shallow-rooted maize. In another study, Sekiya et al. (2011) showed that plants with deep roots are ideal for intercropping with shallow-rooted crops in water limited agricultural fields and that this kind of intercropping system allows shallow-rooted plant to access deep soil moisture indirectly without having deep roots. Other studies have also shown the transfer of hydraulically lifted water (HLW) from a deep-rooted plant to neighboring shallow-rooted plants (Caldwell and Richards, 1989; Moreira et al., 2003; Brooks et al., 2006; Bogie et al., 2018). While these experiments have suggested that bioirrigation could be an important mechanism for drought stress avoidance of intercropped field crops, evidence for the efficiency of this mechanism in the field is still lacking.
The success of an intercropping system in the field depends on the avoidance of competitive growth inhibition among the intercropping partners. This requires appropriate spacing of the intercropping partners so that competitive, complementary, and facilitative interactions are well balanced and that yield improvements can be achieved. In particular, for bioirrigation to be effective, it seems that an ideal spacing between the intercropping partners is essential. On the one side, intercropping partners have to be arranged with sufficient space among each other in order to avoid competition. On the other side, plants need to be spaced (Burgess, 2011; Prieto et al., 2011) to allow the transfer of bioirrigated water from one rhizosphere to another.
In addition to intercropping approaches, “biofertilization” such as inoculation with arbuscular mycorrhizal fungi (AMF), combined with plant growth promoting rhizobacteria (PGPR), are beginning to become established as an effective and sustainable measure to improve yields (Mäder et al., 2011; Schütz et al., 2018; Mathimaran et al., 2020). The role of AMF for the uptake and transfer of nutrients and water to host plants has been well demonstrated (Augé et al., 2001; Querejeta et al., 2003). Biofertilization might have a particular potential to boost the yield of intercropping systems because AMF can form a common mycorrhizal network (CMN) that can transfer nutrients between two plants and balance as such belowground competition (Smith and Read, 2008). In addition, a CMN between the roots of two plants can also constitute a pathway for the transfer of water. Egerton-Warburton et al. (2007) have demonstrated that arbuscular mycorrhizal hyphae provide a potential pathway for the transfer of HLW between two plants. Our recent work has shown that CMN plays a key role in facilitating the transfer of water between the rhizospheres of two intercropped partners and can in turn improve the water relations of shallow rooted crops during soil drying in a greenhouse experimental setup under controlled conditions (Singh et al., 2019). However, a further experiment with bigger pots (50 L) than in the previous experiment did not show an effect of the CMN on water relations but treatments with CMN had lower foliar damage than treatments without CMN during drought (Singh et al., 2020).
The effects of biofertilizers on stabilizing and improving the yields in intercropping systems by improving water relations via bioirrigation have not yet been tested under field conditions, though recent greenhouse studies have shown evidence of facilitation of bioirrigation by AMF and PGPRs (Saharan et al., 2018; Singh et al., 2019). Furthermore, it is unclear to what extent beneficial effects of biofertilizers in intercropping systems depend on an appropriate spacing of the crops and if—given the appropriate spatial arrangement of crops—the establishment of a CMN can indeed facilitate bioirrigation and improve the water relations of shallow-rooted crops in intercropping systems in dryland agriculture. In this study, we investigated the effects of biofertilization on the yield of a legume—cereal intercropping system and tested different spatial arrangements of the plants in combination with biofertilizer treatments. We used pigeon pea (Cajanus cajan) (PP) as a deep-rooted plant and finger millet (Eleusine coracana) (FM) as shallow-rooted plant to investigate the following research questions: (i) Does the spatial arrangement (i.e., different geometric arrangement of component crops using row-wise and mosaic pattern) of intercropping partners affect straw and grain yield in a FM—PP intercropping system compared to monocropping of the same crops? (ii) Does the application of biofertilizers further improve yields in spatially differently arranged intercropping systems? (iii) Can intercropping in conjunction with a CMN lead to an improvement of the water relations of shallow-rooted crops?
Materials and Methods
Selection of Field Experiment Site and Crop Varieties
To test the influence of spatial arrangement and biofertilizers on crop yields of PP and FM, field trials were carried out at two different locations during the growing seasons 2016/17 and 2017/18. One experimental site was located at the research field of the University of Agricultural Sciences, Gandhi Krishi Vigyana Kendra Campus (GKVK), Bengaluru, Karnataka, India. The other site was located at the research field of M S Swaminathan Research Foundation (MSSRF), Kolli Hills, Tamil Nadu, India. At both sites two field trials were conducted at different plots; at GKVK, coordinates for 2016/17 and 2017/18 are 13° 05' 14” N 77° 34' 13” E and 13° 05' 13” N 77° 34' 15” E, respectively. For the Kolli Hills site, coordinates for 2016/17 and 2017/18 are 11°16' 36.0” N 78° 23' 58.6” and 11° 16' 34.0” N 78° 23' 58.6”, respectively. Both experimental sites were selected because farmers have already adopted a cereal-legume intercropping system there and have been cultivating PP and FM as one of their main crops, and to cover broad growth conditions as both sites differ in their soil characteristics and average annual rainfall. The soil type at the Bengaluru site is an alfisol consisting of 67.8% sand, 7.7% silt and 25.2% clay with pH of 5.1, while Kolli Hills has a vertisol soil type with 33.2% sand, 30% silt and 36.8% clay with pH of 5.2. Based on farmers practice in the region and recommendations from local agronomists, we selected FM (GPU-28) and PP (BRG-2) for the field experiment at the Bengaluru site and PP (SA-1) and FM (Suruttai kelvaragu) at the Kolli Hills site (Mathimaran et al., 2020).
Rainfall
The total annual precipitation at the Bengaluru site was 694 mm in 2016 and 1,104 mm in the year 2017. At Kolli Hills, the total annual precipitation was 281 mm in 2016 and 1,690 mm in 2017. Rainfall data recorded during the experimental period indicate that the Kolli Hills site received less rain than the Bengaluru site (Supplementary Figure 1). Both sites received the maximum amount of rain during the months of May, June and July. The Bengaluru site received up to 40–60 mm rain during September, October, and December, while the Kolli Hills site was completely dry after July during 2016. During the summer 2016, both research sites, received very low precipitation, as recorded by our weather station at the field sites. For the comparison and visulaization, precipitation data from nearest sites as recorded by the Climate Research Unit (Harris et al., 2020) are shown in Supplementary Figure 1.
Intercrop Field Design With Different Spatial Arrangement of PP and FM
The plot size for each treatment was 7.2 × 3.6 m (width × length) with a net plot area of 3.6 × 1.8 m (Figure 1). The net plot area defines the central part of each plot, where all physiological, growth and yield parameters were assessed. The field experiments had six treatments: FM monoculture (T1), PP monoculture (T2), 2:8 (PP:FM) row-wise intercropping (T3), 1:4 (PP:FM) row-wise intercropping (T4), 100% mosaic (T5) and 50% mosaic (T6) (Figure 1B). Each treatment was replicated four times in a randomized block design.
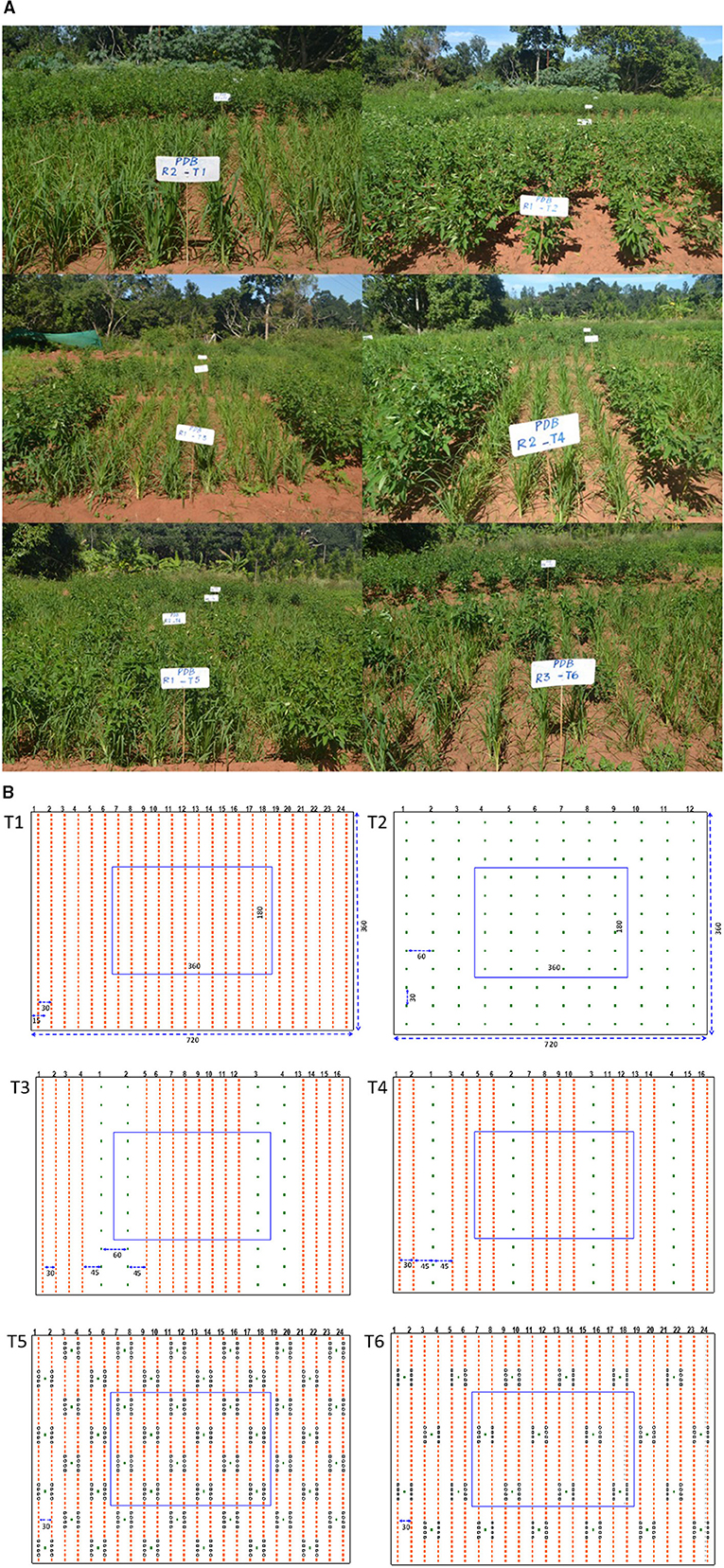
Figure 1. (A) Field site at Kolli Hills, India, with various spatial arrangements of component crops pigeon pea (PP) and finger millet (FM) in intercropping systems. (B) Schematic diagram of the different spatial crop arrangements. Top row: monoculture of FM (T1) and PP (T2). Middle row: 2:8 (PP:FM) row-wise intercropping pattern (T3) and 1:4 (PP:FM) row-wise intercropping pattern (T4). Bottom row: 100% (T5) and 50% (T6) mosaic intercrop design.
In monocultures, the density of FM was 48 plants per m2, and the density of PP was 6 plants per m2. We planted 8 times more individuals for FM than for PP per area and the total number of plants for FM in monoculture (T1) was 1,152 per plot and 288 plants in the net plot area. For PP monocrop (T2), the total number of plants was 144 in the total plot and 36 plants in the net plot area. The spacing between FM rows was 30 cm and the distance between FM plants within a row was 7.5 cm. The spacing between PP rows was 60 cm and the distance between PP plants within a row was 30 cm. In intercropping treatments, the spacing between PP and FM rows was 45 cm.
Intercropping systems were based on FM monocultures, where eight FM plants were substituted by one PP plant. Row-wise intercropping systems (treatment T3 and T4) were based on previous investigations under rainfed conditions in Karnataka, India (Ashok et al., 2010; Padhi et al., 2010; Mathimaran et al., 2020). For T3 (2:8 PP:FM row-wise arrangement), each replicate had thus 48 PP (12 plants × 4 rows) and 768 FM (48 plants × 12 rows in each total plot area). T4 (1:4 PP:FM row-wise arrangement) had the identical number of PP and FM plants as T3 but it differed in row arrangement where one row of PP was planted after four rows of FM. Treatment T5 (100% mosaic) consisted of identical numbers of PP and FM plants as T3 and T4, but PP and FM plants were planted within the same row in a mosaic design (Figure 1B). In treatment T6 (50% mosaic), the number of PP was reduced by 50 % and replaced by FM plants. It consisted of 24 PP plants (2 plants × 12 rows) and 960 FM plants. In the 2017/18 field trial at the Bengaluru site, FM plants in T5 were not substituted by PP but PP was accidentally added into the mosaic design. Therefore, plant density of FM was higher than in the other treatments.
We established the same treatments in the years 2016/17 and 2017/18 except for T6, which was not established in 2017/18 based on results from 2016/17 field trial. While field trials during year 2016/17 had only treatments with biofertilizers, field trials during the year 2017/2018 included treatments with and without biofertilizers (Table 1).
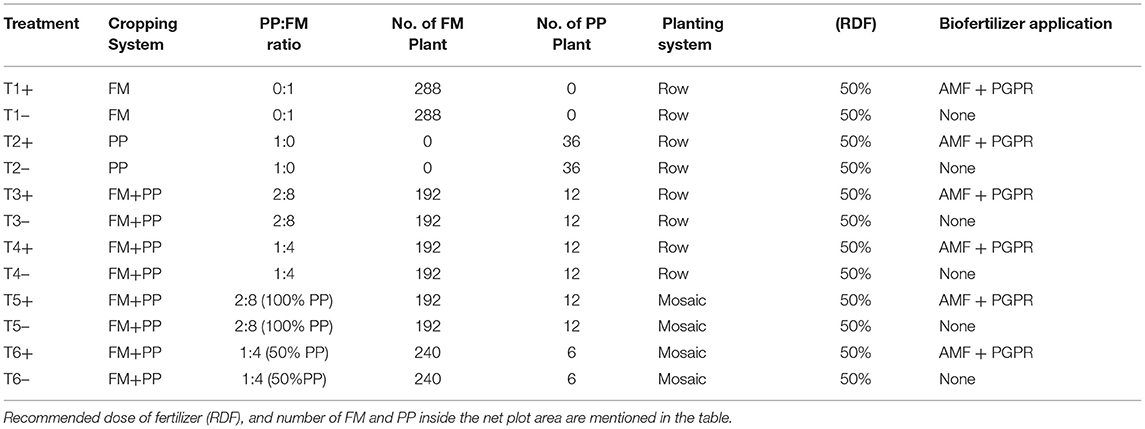
Table 1. Intercropping treatments with (AMF + PGPR) and without (none) biofertilizer application were designed and tested at two experimental sites, Bengaluru and Kolli Hills in India.
Application of Fertilizers and Biofertilizers
Mineral fertilizers were applied at 50% of the recommended dose of fertilizer (RDF) to farmers for all plots as basal application, i.e., at rate of 12.5:25:12.5 NPK kg ha−1 for PP and 25:20:12.5 NPK kg ha−1 for FM. Nitrogen (N) fertilizer was given in the form of Urea (46% N-0P2O5- 0K2O, SPIC India Fertilizer Company), Phosphate (P) fertilizer was given in the form of Single Super Phosphate (SSP, 0N-16% P2O5-0K2O, SPIC India Fertilizer Company), and Potash (K) fertilizer was given in the form of Muriate of Potash (MOP, 0N-0P2O5-60% K2O, SPIC India Fertilizer Company). The remaining 50% of the fertilizer was not added as we wanted to test the effectiveness of biofertilizer at only 50% of RDF, with the objective to minimize the input cost of farmers and potentially achieve long-term soil sustainability by reducing the use of mineral fertilizers.
Biofertilizers PGPRs (Pseudomonas sp. MSSRFD41 and Rhizobium liquid formulation) and AMF (Rhizophagus fasciculatus and Ambispora leptoticha) spores in a vermiculite substrate were applied to PP and FM respective treatments as described by Mathimaran et al. (2020). In the biofertilizer treatments, FM seeds coated with PGPR Pseudomonas sp. MSSRFD41 consisting of 1x 109 CFU per ml at the rate of 10 ml kg−1 seed and the PP seeds coated with both PGPR Pseudomonas sp. MSSRFD41 and Rhizobium consisting of 1x 109 CFU per ml at the rate of 10 ml kg−1 seed were sowed, and for no biofertilizer treatments untreated FM and PP were used. AMF A. leptoticha was applied at the rate of 5 g per PP seedling hole (germinated in a polybag, see below) with a dosage of 278 kg ha−1 (Mathimaran et al., 2020). Similarly, in the rows of FM R. fasciculatus, consisting of 15 spores g−1 of substrate was applied as a band application at the rate of ca. 444 kg ha−1. Additionally, a band application of Pseudomonas sp. MSSRFD41 were applied in the planting rows of biofertilizer treatment plots at the rate of 49.5 L ha−1 with a carrier of 7.5 t ha−1 farmyard manure (FYM) and the treatment details were mentioned in the table 1.0 and described in our earlier work Mathimaran et al. (2020). The PGPR strains were multiplied in a liquid formulation of King's B medium. Pseudomonas sp. MSSRFD41 (Sekar et al., 2018) was obtained from the M. S. Swaminathan Research Foundation (MSSRF), Chennai. Rhizobium (Product of Amaravati Agricultural Station, Andhra Pradesh) and AMFs were obtained from Center for Natural and Biological Resources and Community Development (CNBRCD), Bengaluru.
Pre-germination, Sowing of Seeds Into Field, Growth Period and Harvest
Based on an established practice in the area, PP seeds were pre-germinated in polybags (15 × 10 cm) filled with 1.6 kg of a mixture of field soil: FYM:sand (ratio of 15:1:1), and a seed hole of 4 × 1 cm was made at the top (Mathimaran et al., 2020). The bottom layer of the seed hole was filled with A. leptoticha in vermiculite, two PP seeds coated with rhizobia and PGPR strains were kept above the vermiculite layer and field soil was filled on the top. The seeds were allowed to germinate and grow for 35–45 days. Later, healthy seedlings from these polybags were transplanted into the field on the third week of July 2016 for 2016/17 trial, and on the first week of August 2017 during 2017/18 field trial. FM seeds were line sown in rows directly into the field immediately after transplanting the PP seedlings, and after germination, FM seedlings were thinned out to maintain the plant density as required in different treatments. FM and PP plants were harvested after 120 and 207 days after sowing, respectively, in 2016/17 trial at Kolli Hills, while at the Bengaluru site FM and PP were harvested after 127 and 168 days after sowing, respectively. During 2017/18 field trial, FM and PP were harvested at 133 and 245 days after sowing, respectively, at the Kolli Hills site; at the Bengaluru site, FM and PP were harvested after 124 and 160 days of sowing.
Growth and Yield Parameters
Plant growth parameters such as plant height, number of pods, pod weight per plant, number of panicles, grain weight per panicle, straw and grain biomass (both sun dried and oven dried), weight of 1,000 FM seeds and 100 seeds of PP were measured after harvesting the plant material in the net plot area. For biomass, plants were harvested row-wise in the net plot area and straw and grains were separated. The sun-dried biomass was determined after drying the straw under the sun for 15 and 20 days for FM and PP, respectively. Grains were dried under sun for 10 days for PP and FM. A subsample of the sun-dried straw and grain material was oven dried at 80°C for 24 h for calculating the dry matter per row, moisture content of oven dried samples were not measured. Biomass per plant was calculated by dividing the row biomass by the number of plants in each row; biomass in ton per ha was obtained by multiplying the row biomass with the number of rows per ha.
Land Equivalent Ratio (LER)
The facilitative and competitive interactions between PP and FM in response to the different treatments were calculated using the LER. The LER indicates the efficacy of an intercropping system for using natural resources compared with monoculture (Willey and Osiru, 1972). The baseline for LER is one. If the LER is greater than one, intercropping favors growth and yield of plants, and when it is lower than one, intercropping negatively affects the growth and yield of plants. The LER was calculated as
Where YFM and YPP are yield of PP and FM in its monoculture, YFM, PP is yield of finger millet in intercropping, and YPP, FM is yield of pigeon pea in intercropping.
Measurement of Physiological Parameters
To test if different spatial arrangements of FM and PP, and the application of biofertilizers affect the water relations and growth of FM, we determined FM leaf water potential at predawn (04:00 to 05:00 h) and mid-day (12:30 to 13:30 h) toward the end of the field trial during first three weeks in November during 2016/17 and 2017/18. Due to limitation in resources, particularly manpower and time, these measurements were only performed at the Bengaluru site. Leaf water potential (LWP) was measured using a pressure chamber (model 1000, Pressure Chamber Instrument Company, USA). For predawn measurements, leaf samples were collected between 04:00 and 05:00 h and for midday measurements, leaves were sampled between 12:30 and 13:30 h. After sampling, leaves were packed into airtight Ziploc bags to avoid water loss. Bags were kept in the dark and leaf water potential was measured within 1–2 h after sampling.
Statistical Analysis
Analysis of yield data and LWP from field trials was carried out using GraphPad Prism software (version 7.0 for Mac OS X, GraphPad Software, La Jolla California USA). Data are expressed as mean ± standard error of mean (SEM). Tukey‘s test was used for post hoc multiple treatment comparison following one-way ANOVA or multifactor ANOVA using general linear models. The criterion for significance was p < 0.05.
Results
Total Biomass, Straw, and Grain Yield per Hectare and LER
Intercropping and the spatial arrangement of the intercropping partners had a significant effect on the total biomass yield per hectare at the Bengaluru site in 2016/17 (Figure 2; Supplementary Table 1). In particular, the treatment T3+ produced significantly more biomass per hectare than monocultures of the constitutive crops or other spatial arrangements at Bengaluru in 2016/17. Likewise, treatment T3+ resulted in higher yields for straw and grain as compared to the other treatments in 2016/17 at the Bengaluru site (Figure 2, Supplementary Table 1). For the intercropping treatments, total biomass yield, straw yield and grain yield all declined from the T3+ to T6+. The results differed at the Kolli Hills site, where in 2016/17 PP (T2+) produced the highest yields for total biomass, straw, and grain and where FM (T1+) and the different intercropping treatments produced slightly lower yields with no significant differences among each other (Supplementary Table 1). In summary, in 2016/17 we found a strong positive intercropping effect for total biomass yield, straw yield and grain yield at the Bengaluru site, where the intercropping effect were strongest in the 8:2 row-wise spacing. In contrast, no yield improvements by intercropping irrespective of the spatial arrangement were observed at the Kolli Hills site.
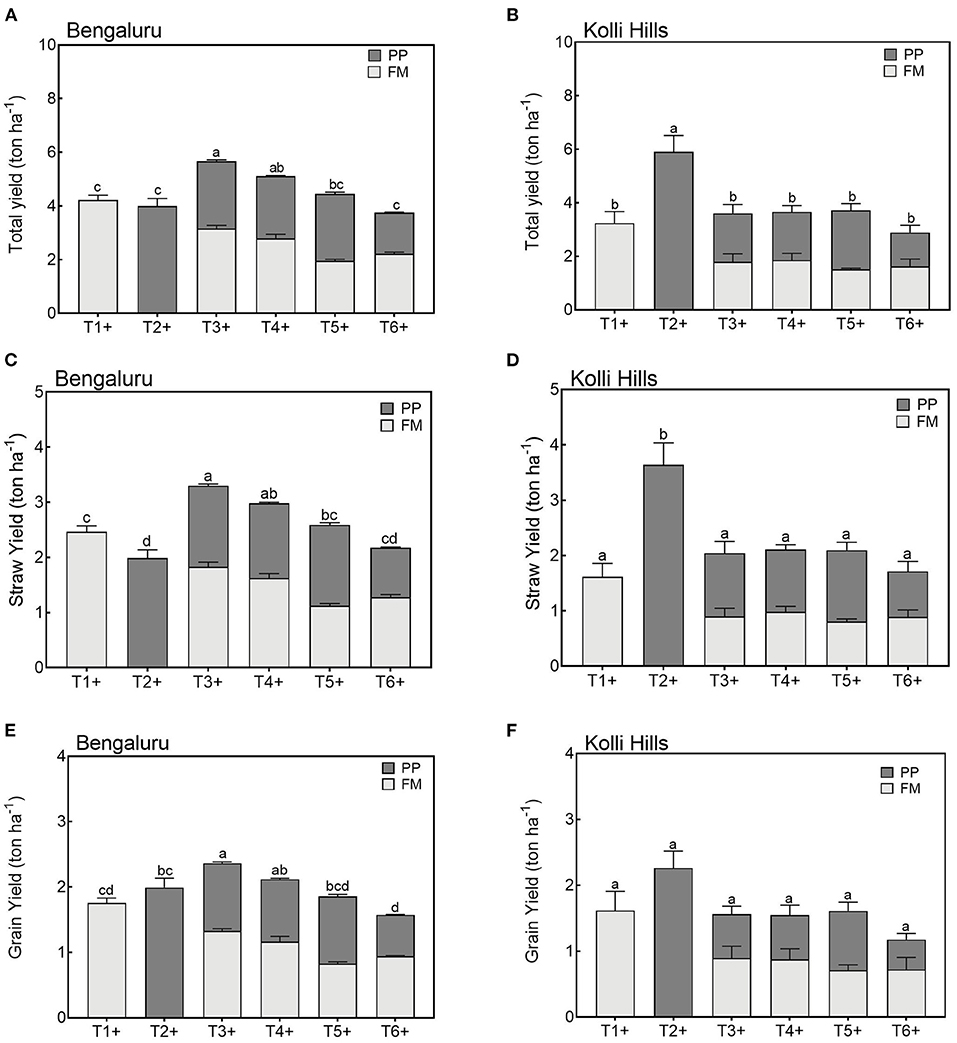
Figure 2. Total biomass, straw and grain biomass of FM and PP at Bengaluru (A,C,E) and Kolli Hills (B,D,F) during year 2016/17. Bars represent the average of four replicates with one standard error of mean. One-Way ANOVA followed by Tukey‘s test (post-hoc test) was used for the combined biomass of FM and PP, separately for each site, and values with same letters are not significantly different from each other at p > 0.05.
These observations are also reflected in LER values. At the Bengaluru site, LER values for total biomass were greater than one for T3+, T4+ and T5+ and where T3+ had the highest LER value. Similarly, for straw biomass, T3+ had higher LER values than T4+, T5+ and T6+. For grain biomass LER values were greater than one for the T3+ and T4+ treatment, equal to one for T5+ and less than one for T6+ (Figure 3). At Kolli Hills LER values for all treatments were less than one (Figure 3).
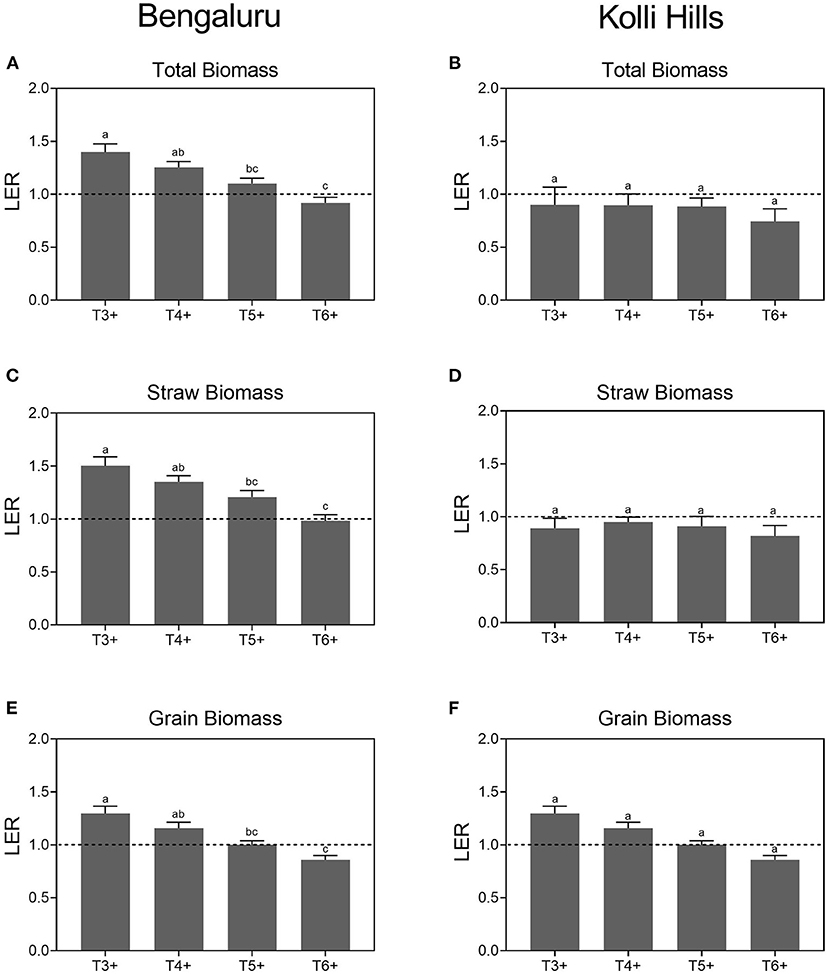
Figure 3. Land equivalent ratio (LER) in different intercropping treatments during 2016/17 at the Bengaluru (A,C,E) and Kolli Hills (B,D,F) sites. Bars represent the average of four replicates with one standard error of mean. Tukey‘s test (one-way ANOVA) was used for multiple comparison, separately for each site, and values with same letters are not significantly different from each other at p > 0.05.
In 2017/18, intercropping and the spatial arrangement of the intercropping partners also had a strong and significant effect on the total biomass yield, straw yield, and grain yield at the Bengaluru site (Figure 4). As in 2016/17 the treatment T3– and T3+ produced significantly more biomass per hectare than monocultures of the constitutive crops or other spatial arrangements when compared to the respective treatments with and without biofertilizer. Importantly, the application of biofertilizers enhanced the total biomass yield, straw yield and grain yield in all treatments, and this effect was consistent irrespective of experiment site, mono- or intercropping (Supplementary Table 2). At Kolli Hills, we also found significant treatment effects (Figure 4). However, intercropping treatments did not produce higher yields for total biomass and straw than any of the other treatments with or without biofertilizer. Yet, treatment T5+ was equal in total biomass yield to the most productive monoculture (T2+). For grain yield FM monoculture exceeded the productivity of PP (Figure 4F) and in intercropping T3–, T3+ and T5+ grain yield was similar to monoculture of FM with or without biofertilizer. The effects of biofertilizers on total biomass yield, straw yield, and grain yield that we detected at the Bengaluru site were also observed at the Kolli Hills site and this effect was again consistent across all treatments (Figure 4; Supplementary Table 2). We did not find a significant interaction between treatment and biofertilizers nor a significant three-way interaction between treatment, biofertilizers, and site. However, as indicated above, the effects of biofertilizers at Kolli Hills resulted in total biomass yield, straw yield and grain yield that were of the same magnitude in some intercropping treatments as the highest yield in the corresponding monocultures (e.g., T5+ for total biomass yield and straw yield, and T3+ and T5+ for grain yield, Figure 4). In summary, in 2017/18 we found a strong positive intercropping effect for total biomass yield, straw yield and grain yield at the Bengaluru site. In Kolli Hills, no such intercropping effect was found. Importantly, biofertilizers improved the yields of crops at both sites and independently of treatment. Despite the nonsignificant biofertilization—treatment interaction, intercropping treatments at Kolli Hills yet showed a trend to be more enhanced through biofertilizers than monocultures, to an extent that they produced similar yields as the most productive monoculture, which we did not observe without biofertilizers.
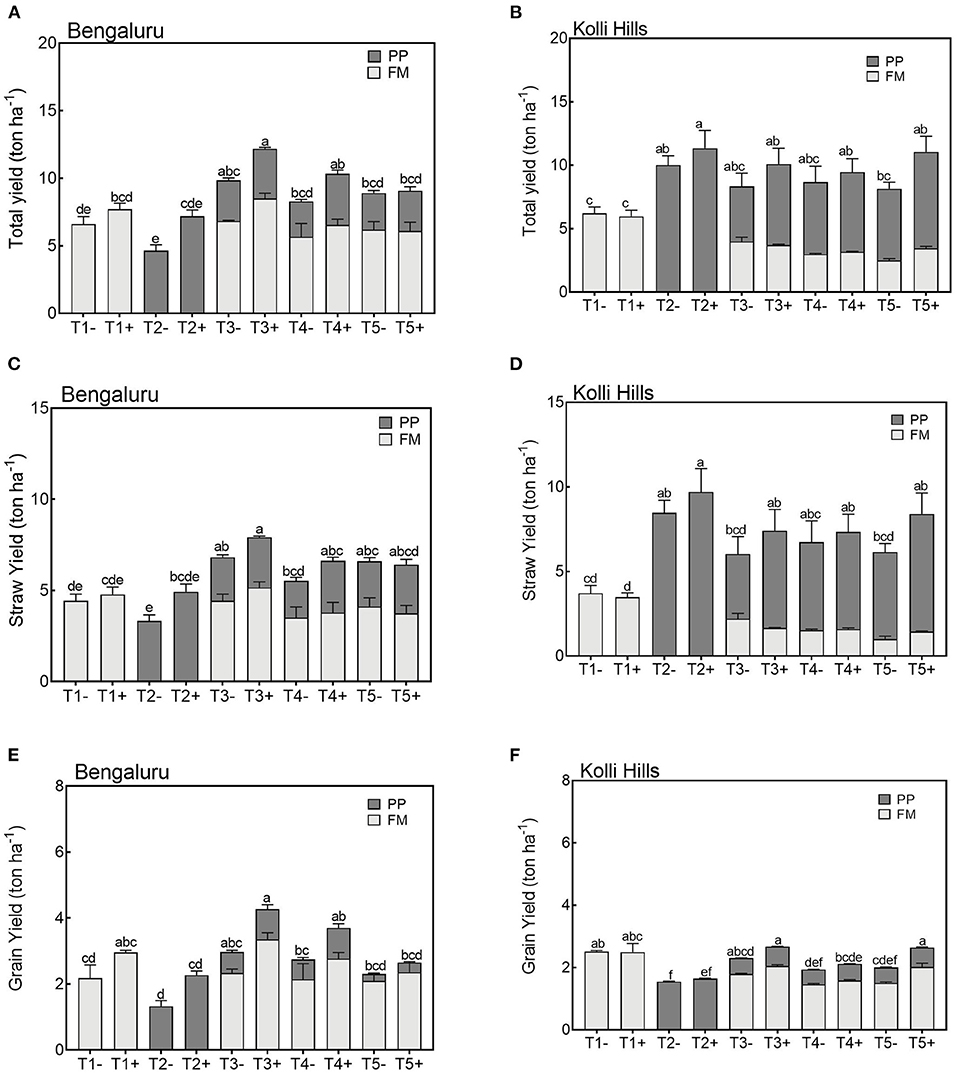
Figure 4. Total biomass, straw and grain biomass at Bengaluru (A,C,E) and Kolli Hills (B,D,F) during year 2017/18. Bars represent the average of four replicates with standard error of mean. One-way ANOVA followed by Tukey‘s test (post-hoc test) was used for the combined biomass of FM and PP, separately for each site, and values with same letters are not significantly different from each other at p > 0.05.
These observations were confirmed by LER values for 2017/18 at both sites (Figure 5). LER was greater than one at the Bengaluru site for all treatments. Also, LER values at the Bengaluru site were largest for T3 and declined in the other treatments. Biofertilizers had a negative effect on LER values in all spatial arrangements at the Bengaluru site. At Kolli Hills, LER values in treatments without biofertilizers were either equal to or less than one. Biofertilizers increased, however, the LER values in all spatial arrangements to values of one or greater than one and the largest values were observed for T3+ and T5+.
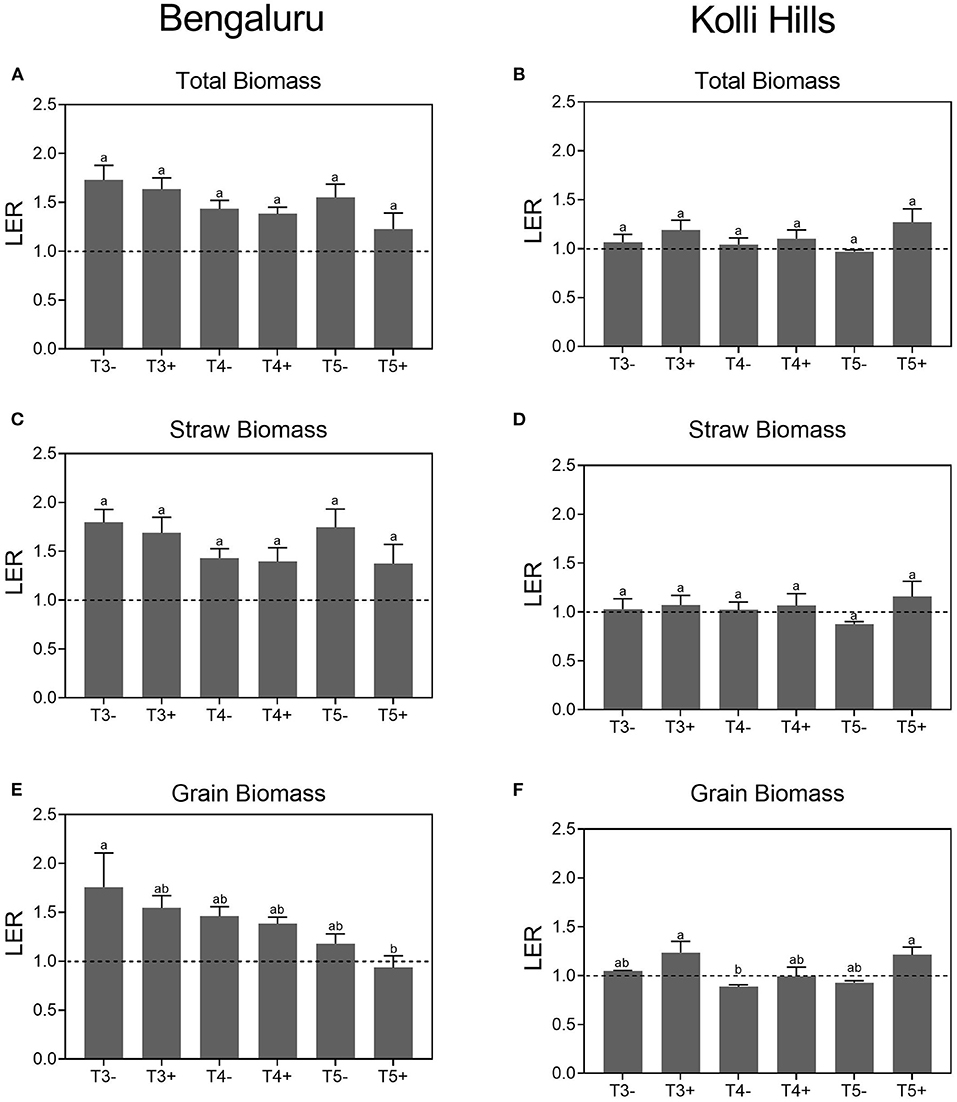
Figure 5. Land equivalent ratio (LER) of total grain yield in different intercropping treatments during 2017/18 at the Bengaluru (A,C,E) and Kolli Hills (B,D,F) sites. Bars represent the average of four replicates with standard error of mean. Tukey‘s test (one-way ANOVA) was used for multiple comparison, separately for each site, and values with same letters are not significantly different from each other at p > 0.05.
Per Plant Biomass Yield of PP and FM
We found a significant effect of the intercropping treatments on total biomass per plant, total straw yield per plant and total grain yield per plant of PP and FM at the Bengaluru site but not at Kolli Hills in 2016/17 (Figure 6; Supplementary Tables 3, 4). At Bengaluru, total biomass per plant in FM was highest in the monoculture (T1+), the 2:8 treatment (T3+) and the 1:4 treatment (T4+). The biomass of individual plant was significantly reduced in the mosaic treatments (T5+ and T6+) compared to monoculture (T1+) and row-wise intercropping (T3+ and T4+, Figure 6A). PP showed highest total biomass in the mosaic treatment T6+, followed by other intercropping treatments and lowest biomass in the monoculture T2+ (Figure 6C). At Kolli Hills, total biomass per plant in PP and FM did not differ significantly among treatments (Figures 6B,D). However, the trend was similar to the Bengaluru site where FM showed a reduction in biomass in mosaic treatments while PP showed an increase in biomass in mosaic treatments.
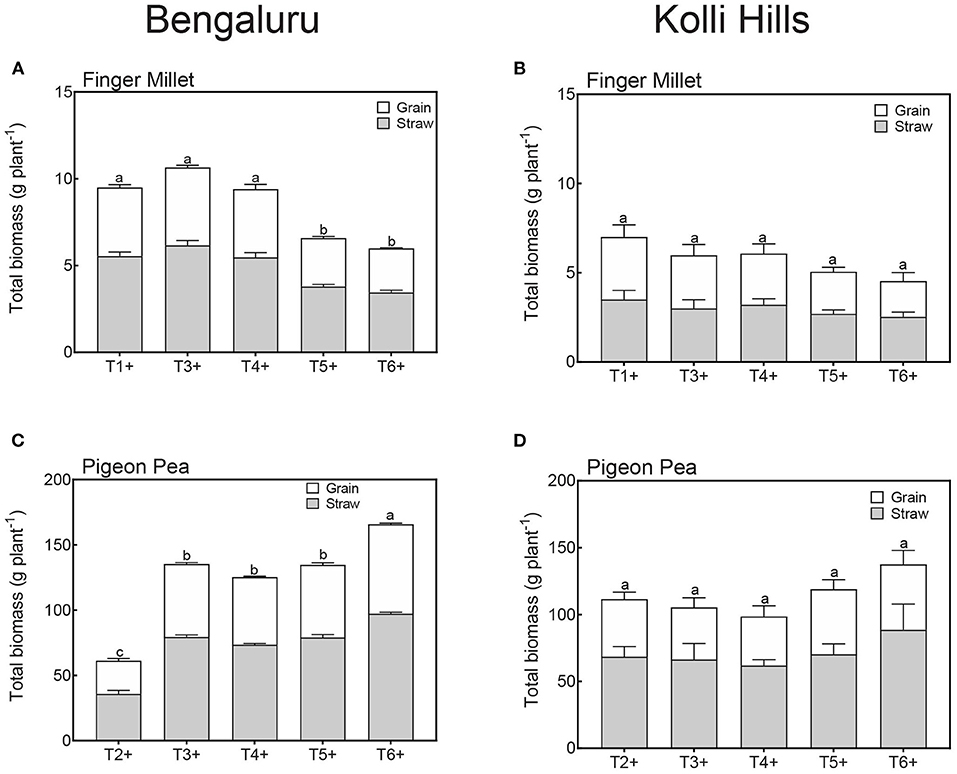
Figure 6. Total biomass per plant of FM and PP at Bengaluru (A,C) and Kolli Hills (B,D) during 2016/17 field trial. Bars represent the average of four replicates with standard error of mean. One-way ANOVA followed by Tukey‘s test (post-hoc test) was used for the combined biomass of grain and straw, separately for each site, and values with same letters are not significantly different from each other at p > 0.05.
In 2017/18, we also found a significant treatment effect on the total biomass, straw yield and grain yield of FM and PP at the Bengaluru site but only for PP at Kolli Hills (Figure 7; Supplementary Tables 5, 6). At the Bengaluru site, total biomass of FM plants in T3+ was significantly larger than total biomass of plants in treatments T1–, T1+ and T4–. Total biomass of PP plants was largest in T3+ and T4+ compared to T2–, T2+, and T4– (Figure 7C). At Kolli Hills total biomass per plant in FM did not show any significant difference among intercropping and monoculture. For PP, in contrast, total biomass per plant was largest in treatments T4+ and T5+ compared to T2– and T2+ (Figure 7D).
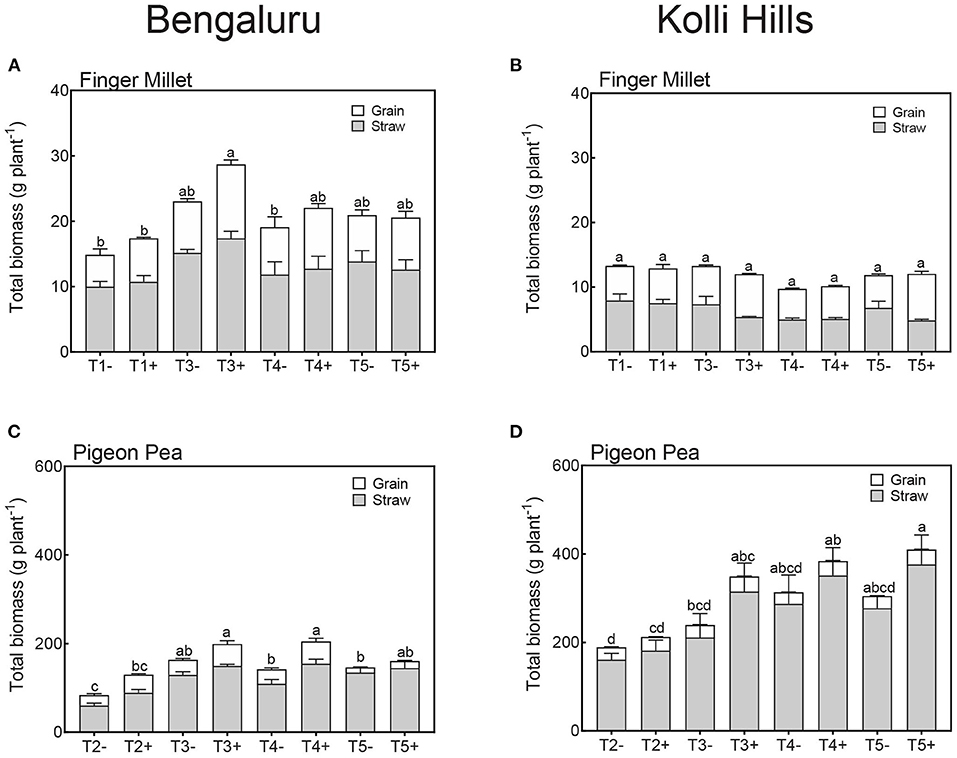
Figure 7. Total biomass per plant of FM and PP at Bengaluru (A,C) and Kolli Hills (B,D) during 2017/18 field trial. Bars represent the average of four replicates with standard error of mean. One-way ANOVA followed by Tukey‘s test (post-hoc test) was used for the combined biomass of grain and straw, separately for each site, and values with same letters are not significantly different from each other at p > 0.05.
A two-way ANOVA analysis was performed to test the effects of spatial arrangement and biofertilization on per plant yield (Supplementary Tables 7, 8). At both sites in 2017/18 FM yield did not show any significant effect of biofertilizer application. However, PP showed a strong significant effect of biofertilization at the Bengaluru site, and at the Kolli Hills site the effect was marginally significant. At both sites, the effect of biofertilization did not differ among treatments with different spatial arrangements of the component plants of the intercropping system.
Water Relations of PP and FM in Intercropping Treatments
Measurements of predawn leaf water potential (LWP) were done on FM leaves at the Bengaluru site to evaluate the effects of spatial arrangement and biofertilizer application on the water relations of FM in different intercropping treatments (Figure 8; Supplementary Table 9). In 2016/17 in week 1 of the measurements (1st week of November 2016), FM in treatment T1+ had the most positive values (−0.70 MPa). FM in the mosaic treatment T5+ had the lowest predawn LWP of −2.5 MPa, which is significantly lower than in the row-wise intercropping treatment (T3+, −0.95 MPa). In week 2 (2nd week of November 2016), FM in monoculture (T1+) maintained a significantly higher predawn LWP of −1.15 MPa than in any other intercropping treatment (Figure 8A). At week 3, (3rd week of November 2016) FM in treatments T4+ and T5+ were dead (desiccated & drooped), while FM in T3+ and T6+ showed a significantly lower LWP of −1.89 and −1.90 MPa than FM in monoculture (−1.34 MPa).
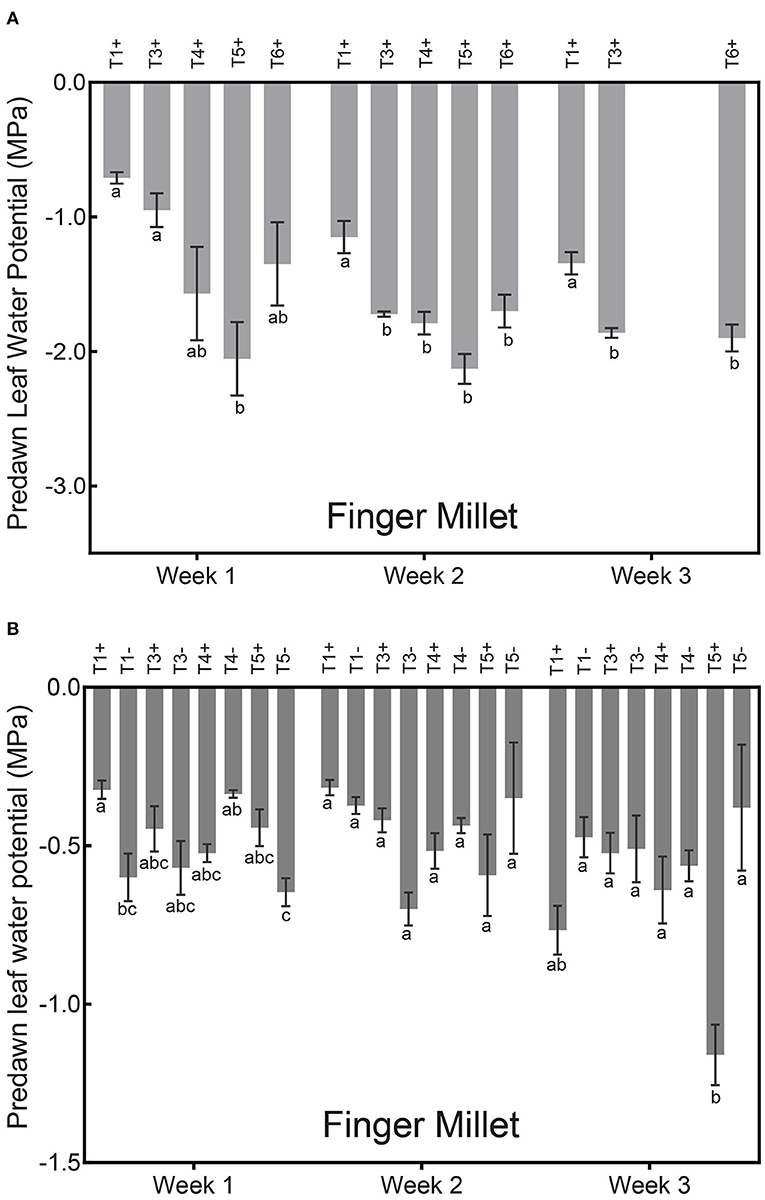
Figure 8. Predawn leaf water potential of FM in different intercropping treatments during the field trials of 2016/17 (A) and 2017/18 (B) at Bengaluru. Weeks represent first, second and third week of November in 2016 and 2017, during which measurement was done. Bars represent the average of four replicates with standard error of mean. Tukey‘s test (One-way ANOVA) was used for multiple comparison and values with same letters are not significantly different from each other at p > 0.05.
In 2017/18 at week 1 (1st week of November 2017), predawn LWP of FM in monoculture with biofertilizer (T1+) had values of −0.32 MPa which is significantly more positive than FM in monoculture without biofertilizer (T1–) (−0.60 MPa) or any of the intercropping treatments (Figure 8B). Later, FM did not show any significant difference in LWP compared to the other intercropping treatments. Interestingly, treatments without biofertilizer showed generally lower values for LWP as compared to the respective treatments with biofertilizer. The biofertilizer application did not have a significant effect on LWP of FM, but intercropping treatments showed a strong significant effect (Supplementary Table 9). There was a significant interaction between the effect of biofertilizers and the intercropping treatments. As shown in Figure 8, treatments T1–, T1+, T5–, and T5+ consistently showed a large difference in LWP of FM with or without biofertilizer.
Discussion
The results obtained from the field trials during 2016/17 and 2017/18 showed that intercropping can improve the straw and grain yield in PP–FM intercropping compared to the respective monocultures but that intercropping effects vary depending on the site characteristic such as climate and soil type as well as crop variety. The spatial arrangement of component plants also affected the total, straw, and grain biomass in intercropping treatments, and this effect also varied across sites. The results from 2017/18 clearly demonstrated a positive effect of biofertilizer on biomass yield, and this effect was irrespective of site, spatial arrangement, mixed or monoculture. Despite the positive effect of intercropping and biofertilization on FM and PP yield, water relations of FM were not enhanced in the intercropping treatments or by biofertilizers. Most likely this was due to interspecific competition between PP and FM for soil moisture in the topsoil layer. On the basis of these results, we propose that intercropping and the application of biofertilizer both enhance the yield of cropping systems and effects on yield are strongest if intercropping and biofertilization are applied in combination. However, the spatial arrangement of component crops is a key factor that affects the productivity of the involved intercropping partners and needs to be considered in the design of intercropping systems.
Is PP–FM Intercropping Beneficial Over Monocropping?
The yield advantage in intercropping systems is typically assigned to resource sharing and facilitation (Loreau and Hector, 2001; Li et al., 2014; Duchene et al., 2017). Resource complementarity reduces the niche overlap and competition between two species and allows crops to take up a greater amount of resources than the sole crops. Ghanbari et al. (2010) reported resource complementarity in maize-cowpea intercropping systems, where intercropping increased the light interception, reduced evaporation, and improved soil moisture conservation compared to maize sole crops. In most cases, facilitation occurs through increased availability of soil resources such as water and nutrients (Jensen et al., 2020). Intercropping systems with legume species (such as PP in this study) can increase agricultural productivity by providing increased nitrogen availability through N2 fixation, and are therefore used very frequently (Hauggaard-Nielsen and Jensen, 2005; Altieri et al., 2012). Non-legumes (such as cereals) in an intercropping system with legumes may obtain additional N released by legumes into the soil, which can contribute up to 15% of their N content (Zuo et al., 2004; Li et al., 2007).
According to our expectation, we found that at the Bangalore research site, the intercropping treatments (T3+ and T4+) produced higher yields (Figures 2, 4) than monocultures in both growing seasons. In contrast, at Kolli Hills, there was no significant effect of intercropping in the 2016/17 season. Also in 2017/18, we did not observe strong intercropping effects but yields in some intercropping treatments (T5+) were as high as the highest yields in the monocrop. Accordingly, LER values were above one at Bangalore in both years but below one at Kolli Hills in 2016/17 and near zero or above in 2017/18. This illustrates that intercropping effects depend on the weather of the growing season and soil type at the experiment site. The total rainfall at Kolli Hills in 2017 was 1690 mm, while the 2016 growing season was shaped by a severe drought with a total rainfall of only 281.7 mm. Additionally, both locations differ in their soil properties. At the Bangalore site, the soil is an Alfisol with 67.8 % sand, 7.7 % silt, 25.2 % clay, Corg 0.5 % and a pH of 4.8. At the Kolli Hills site, the soil type is a Vertisol with 33.2 % sand, 30.0 % silt, 36.8 % clay, Corg 0.8 % and a pH of 5.2 (see Mathimaran et al., 2020, supplimentary data). The relationship between crop yield and soil depends on complex interactions between physico-chemical properties of soil and other climatic factors (Stenberg, 1998). Juhos et al. (2015), using a multivariate statistical approach, show that in years of drought, the sodification, salinization, soil texture, and nutrient content determined the yield, while in humid years soil organic matter and nutrient content were the main determining factors for crop yields. Our results indicate that the low amount of rainfall and inherent soil properties could have caused different intercropping effects at the two sites and between the two growing seasons (Figure 2).
Effect of Spatial Arrangement on Yield in PP—FM Intercropping
At the Bengaluru site, straw and grain yield (per hectare) was higher in the row-wise than in the mosaic intercropping treatments during 2016/17 and 2017/18 field trial. The results from Kolli Hills were inconsistent, perhaps because of rainfall and soil properties as indicated above. Effects of the spatial arrangements can be explained by intra- and inter-specific competition, as illustrated when data are expressed per plant biomass (Figures 6, 7). Results from Bengaluru site clearly indicate that PP benefits in terms of per plant biomass in intercropping treatments likely due to reduction in intra-specific competition that PP faces in monoculture. In contrast, FM faces higher inter-specific competition in mosaic treatments, which leads to a reduction in per plant biomass in mosaic treatments (T5+ and T6+). The field trial results from Kolli Hills, however, did not show any significant effect of spatial arrangement on per plant biomass in PP and FM during 2016/17 trial (Figures 6B,D). During 2017/18, only PP showed a significant increase in per plant biomass in intercropping treatments T4+ and T5+ compared to monoculture treatments T2– and T2+. The effect of spatial arrangement on FM per plant biomass was not significant and it was consistent during both years at Kolli Hills.
The results of this study consistently show that PP growth is favored in intercropping systems due to a reduction in the intraspecific competition, while FM faces a higher interspecific competition in the mosaic intercropping than in row-wise intercropping. This effect is modulated by the variety of intercropped PP (different varieties of PP were grown at the Bangalore and Kolli Hills research sites), soil quality and local weather. There are several factors, such as light, soil moisture and nutrient, that affect the yield of each component crop in intercropping (Bedoussac et al., 2015). The difference in penetration of light into canopy is considered to be a key factor affecting photosynthesis and ultimately growth and yield (Gwathmey and Clement, 2010; Kaggwa-Asiimwe et al., 2013). In our study, the reduction in light availability to relatively short FM plants standing next to taller PP plants in the mosaic intercropping treatments T5 & T6 (see supplementary data) could be a factor impacting growth, since in all row-wise intercropping designs PP and FM rows were well spaced to avoid a shading effect, which is not the case in the mosaic design. Similar results were reported by Martin and Snaydon (1982) and Dubey et al. (1995), who reported higher yield for barley-beans and sorghum-soybean in row-wise intercropping than mosaic (mixed within rows), respectively.
The intercropping designs tested in this study illustrate that the row-wise intercropping treatment T3+ (2:8 with biofertilizer) performed consistently better than the other arrangements, most probably due to the release of intraspecific competition. Effects of the spatial arrangement of component plants in intercropping have been shown to be species specific. Chen et al. (2004), Lauk and Lauk (2008) and Aynehband et al. (2010) have shown mixing of component plants within rows (mosaic pattern) to be the best arrangement for barley-peas, maize-soybean, and maize-amaranth, respectively. In contrast, Martin and Snaydon (1982) and Dubey et al. (1995) reported higher yields for barley-beans and sorghum-soybean sown in alternate rows than mixed within rows, respectively. Inter-specific competition could occur when two species are planted together and such competition could lead to a decrease in plant growth and yield (Jensen, 1996). In a cereal-legume intercropping system there is a significant number of days of overlapping growth period, and inter-specific competition between component crops could lead to a decrease in yield (Clément et al., 1992; Oljaca et al., 2000; Karasawa and Takebe, 2012). Therefore, the spatial arrangement between the plants needs to be carefully optimized. In this study, PP had a head start of 45 days (polybag transplantation) compared to FM, which provided PP a competitive advantage to acquire more resources (light, nutrients, and water) through its well-established root network, and FM may face, additionally, shading effects due to tall PP plants.
Effects of Biofertilizers
In the 2017/18 field trial, at both experimental sites, the effect of biofertilizer application was positive and showed an increase in total yield (Figure 7). The positive effect of biofertilization did not differ among intercropping treatments with different spatial arrangements (Supplementary Tables 7, 8). The effect of biofertilization was, however, specific to each component plants in the PP–FM intercropping system. Total biomass and straw yield per plant in FM was not significantly affected by biofertilization, but grain yield was significantly increased (Supplementary Table 5) similar to the observations made by Mathimaran et al. (2020). In the case of PP, the effect of biofertilization was significant on total biomass, straw, and grain yield per plant. The results of this study are in agreement with findings of Mäder et al. (2011) who reported that combined application of AMF and PGPR improves grain yield. Previous studies (Reddy, 2012; Mathimaran et al., 2017) have shown that better phosphorus uptake and crop tolerance to biotic and abiotic stresses via PGPR are among the most common mechanisms through which biofertilizers improve crop growth. The increase in grain yield in both component plants (FM and PP) in intercropping was the result of an increased number of panicle and grain weight per panicle in FM and number of pod and pod weight per plant in PP (see supplementary data). Since the process of pod and panicle formation is influenced by light availability, nutrients and soil moisture (Härdter and Horst, 1991; Thorsted et al., 2006; Raza et al., 2020), the yield improvement in row-wise intercropping could be attributed to efficient utilization of nutrients through the applied biofertilizers.
Effect of Intercropping and Biofertilizers on Water Relations of FM
In this study, the water relations (predawn LWP) of FM decreased significantly in mosaic treatments as compared to row-wise and monoculture treatments (Figures 8A,B). The trend in predawn LWP (Figures 8A,B) can also be compared with the trend in biomass production per plant (Figures 6A, 7A), therefore, competition for water could be the limiting factor here which influenced the yield and effectiveness of intercropping treatments at the Bengaluru site. Our results suggest that there exists an important degree of below-ground competition for water between PP and FM, and the facilitative effect of bioirrigation is suppressed. Similar results have been reported by Ludwig et al. (2004), They found that HL performing trees extracted a significant amount of water from the topsoil layer that resulted in lower LWP in understorey grasses. However, grasses were able to absorb soil moisture released by tree due to HL.
One of our objectives was to find out if CMN can facilitate the transfer of bioirrigated water from PP to FM and improve the water relations of FM in intercropping treatments. The results from the 2017/18 field trial showed that CMN did not affect the water relations (predawn LWP) of FM in intercropping treatments. However, at week 1 and 2 (first and second week of November 2017) FM in T3+ had higher (less negative), but not significant, LWP than T3–. Similarly, FM in monoculture treatment showed a higher LWP with CMN than without CMN (Figure 8B). Since, we observed similar effects of CMN in both monoculture and 2:8 row-wise intercropping, we cannot assign this to bioirrigation. The effect of CMN changed over time, and at week 3 (third week of November 2017) treatments T1+, T3+, and T5+ (with CMN) had a lower LWP than T1–, T3–, and T5– (without CMN). The effect of different treatments, biofertilization and times (weekly measurement) had significant interaction with each other (Supplementary Table 9).
In this study, we could not find out if the positive intercropping effect by CMN was due to bioirrigation. This is in contrast to two greenhouse studies that we performed earlier (Singh et al., 2019, 2020) where such an effect of bioirrigation was detectable. The discrepancy among these studies illustrated the difficultly to upscale plant-plant interactions obtained in controlled green-house studies to the field. The average hyphal spread rate of Glomus species is 0.7–0.8 mm per day (Jakobsen et al., 1992; Harinikumar and Bagyaraj, 1995), We did not check for the spread of CMN between PP and FM, but it is possible that the AMF introduced with the biofertilizer could not cover the distance of 45 cm between PP and FM in intercropping treatments and thus, a potential facilitative effect of bioirrigation through CMN was not observed in the field.
Conclusions
This study shows that intercropping has a positive effect on total yield of PP and FM, but that this effect varies across the sites, likely based on site characteristics such as soil type and weather. Importantly, our experiment demonstrates for the first time that the positive effect of intercropping on yield can be enhanced by the application of biofertilizers. In conclusion, the answers to our three research questions are therefore as follows: (i) the spatial arrangement of intercropping partners does affect the straw and grain yield in a FM—PP intercropping system, and the optimal spatial arrangement for PP—FM intercropping system depends on geographic location (local weather conditions) and plant variety. In general, the row-wise treatment (T3+) resulted in better yields than the mosaic treatments at the Bengaluru site, while at the Kolli Hills site in 2017/18, both the row-wise treatment (T3+) and the mosaic treatment (T5+) performed equally well. In addition, (ii) we show that the application of biofertilizer promotes yield in intercropping systems, and the spatial arrangement of component plants does not affect the effect of biofertilization. The effect of biofertilization is mainly due to the promotion of PP. We further show that (iii) the spatial arrangement of plants is a key factor that affects the competition for topsoil moisture between PP and FM.
Data Availability Statement
The original contributions presented in the study are included in the article/Supplementary Material, further inquiries can be directed to the corresponding author/s.
Author Contributions
AK, NM, PR, IK, TN, PM, TB, DS, and JS: conceptualization. AK, NM, PR, TN, PM, TB, DS, and JS: methodology. DS: formal analysis and writing—original draft preparation. AK, NM, PR, RR, IK, TN, and DB: resources. DS, JS, YP, KR, NM, IK, MN, BC, SS, and TN: data curation. DS, NM, JS, PR, YP, KR, RR, IK, TN, MN, BC, SS, DB, PM, TB, and AK: writing—review and editing. All authors contributed to the article and approved the submitted version.
Funding
This research was funded by the Swiss Agency for Development and Cooperation (SDC), and the Department of Biotechnology (DBT), India, and the BIOFI project under the auspices of the Indo-Swiss Collaboration in Biotechnology (ISCB).
Conflict of Interest
The authors declare that the research was conducted in the absence of any commercial or financial relationships that could be construed as a potential conflict of interest.
Publisher's Note
All claims expressed in this article are solely those of the authors and do not necessarily represent those of their affiliated organizations, or those of the publisher, the editors and the reviewers. Any product that may be evaluated in this article, or claim that may be made by its manufacturer, is not guaranteed or endorsed by the publisher.
Supplementary Material
The Supplementary Material for this article can be found online at: https://www.frontiersin.org/articles/10.3389/fsufs.2021.711284/full#supplementary-material
References
Altieri, M. A., Funes-Monzote, F. R., and Petersen, P. (2012). Agroecologically efficient agricultural systems for smallholder farmers: contributions to food sovereignty. Agron. Sustain. Dev. 32, 1–13. doi: 10.1007/s13593-011-0065-6
Ashok, E. G., Dhananjaya, B. N., Kadalli, G. G., Kiran, B. K., Mathad, V., and Gowda, K. (2010). Augumenting production and profitability of finger millet+pigeonpea intercropping system. Environ. Ecol. 28, 28–33.
Augé, R. M., Stodola, J. W., Tims, J. E., and Saxton, A.M. (2001). Moisture retention properties of a mycorrhizal soil. Plant Soil 230, 87–97. doi: 10.1023/A:1004891210871
Aynehband, A., Behrooz, M., and Afshar, A. H. (2010). Study of intercropping agroecosystem productivity influenced by different crops and planting ratios. Am. Eurasian J. Agric. Environ. Sci. 7, 163–169.
Bedoussac, L., Journet, E. P., Hauggaard-Nielsen, H., Naudin, C., Corre-Hellou, G., Jensen, E. S., et al. (2015). Ecological principles underlying the increase of productivity achieved by cereal-grain legume intercrops in organic farming. a review. Agron. Sustain. Dev. 35, 911–935. doi: 10.1007/s13593-014-0277-7
Bogie, N. A., Bayala, R., Diedhiou, I., Dick, R. P., and Ghezzehei, T. A. (2018). Intercropping with two native woody shrubs improves water status and development of interplanted groundnut and pearl millet in the Sahel. Plant Soil 435, 143–159. doi: 10.1007/s11104-018-3882-4
Brooker, R. W., Bennett, A. E., Cong, W. F., Daniell, T. J., George, T. S., Hallett, P. D., et al. (2015). Improving intercropping: a synthesis of research in agronomy, plant physiology and ecology. New Phytol. 206, 107–117. doi: 10.1111/nph.13132
Brooks, J. R., Meinzer, F. C., Warren, J. M., Domec, J. C., and Coulombe, R. (2006). Hydraulic redistribution in a Douglas-fir forest: lessons from system manipulations. Plant Cell Environ. 30:1409. doi: 10.1111/j.1365-3040.2005.01409.x
Burgess, S. S. O.. (2011). Can hydraulic redistribution put bread on our table? Plant Soil 341, 25–29. doi: 10.1007/s11104-010-0638-1
Caldwell, M. M., and Richards, J. H. (1989). Hydraulic lift: water efflux from upper roots improves effectiveness of water uptake by deep roots. Oecologia 79, 1–5. doi: 10.1007/BF00378231
Chen, C., Westcott, M., Neill, K., Wichman, D., and Knox, M. (2004). Row configuration and nitrogen application for barley–pea intercropping in Montana. Agron. J. 96, 1730–1738. doi: 10.2134/agronj2004.1730
Clément, A., Chalifour, F.-P., Gendron, G., and Bharati, M. P. (1992). Effects of nitrogen supply and spatial arrangement on the grain yield of a maize/soybean intercrop in a humid subtropical climate. Can. J. Plant Sci. 72, 57–67. doi: 10.4141/cjps92-007
Dahmardeh, M., Ahmad, G., Syasar, B., and Ramroudi, M. (2009). Effect of intercropping maize (Zea mays L.) with cow pea (Vigna unguiculata L.) on green forage yield and quality evaluation. Asian J. Plant Sci. 8, 235–239. doi: 10.3923/ajps.2009.235.239
Davis, J. H. C., and Woolley, J. N. (1993). Genotypic requirement for intercropping. F. Crop. Res. 34, 407–430. doi: 10.1016/0378-4290(93)90124-6
Dubey, D. N., Kulmi, G. S., and Girish, J. (1995). Relative productivity and economics of sole, mixed and intercropping systems of sorghum (Sorghum bicolor) and grain legumes under dryland condition. Indian J. Agric. Sci. 65, 469–473.
Duchene, O., Vian, J. F., and Celette, F. (2017). Intercropping with legume for agroecological cropping systems: complementarity and facilitation processes and the importance of soil microorganisms. A review. Agric. Ecosyst. Environ. 240, 148–161. doi: 10.1016/j.agee.2017.02.019
Egerton-Warburton, L. M., Querejeta, J. I., and Allen, M. F. (2007). Common mycorrhizal networks provide a potential pathway for the transfer of hydraulically lifted water between plants. J. Exp. Bot. 58, 1473–1483. doi: 10.1093/jxb/erm009
Ghanbari, A., Dahmardeh, M., Siahsar, B. A., and Ramroudi, M. (2010). Effect of maize (Zea mays L.) - cowpea (Vigna unguiculata L.) intercropping on light distribution, soil temperature and soil moisture in arid environment. J. Food, Agric. Environ. 8:239.
Gwathmey, C. O., and Clement, J. D. (2010). Alteration of cotton source-sink relations with plant population density and mepiquat chloride. F. Crop. Res. 116, 101–107. doi: 10.1016/j.fcr.2009.11.019
Härdter, R., and Horst, W. J. (1991). Nitrogen and phosphorus use in maize sole cropping and maize/cowpea mixed cropping systems on an Alfisol in the northern Guinea Savanna of Ghana. Biol. Fertil. Soils 10, 267–275. doi: 10.1007/BF00337377
Harinikumar, K. M., and Bagyaraj, D. J. (1995). Spread of vesicular arbuscular mycorrhizal fungal hyphae in soil. Microbiol. Res. 150, 77–80. doi: 10.1016/S0944-5013(11)80037-6
Harris, I., Osborn, T. J., Jones, P., and Lister, D. (2020). Version 4 of the CRU TS monthly high-resolution gridded multivariate climate dataset. Sci. Data 7:109. doi: 10.1038/s41597-020-0453-3
Hauggaard-Nielsen, H., and Jensen, E. S. (2005). Facilitative root interactions in intercrops BT,” in Root physiology: from Gene to Function, eds H. Lambers, and T. D. Colmer (Dordrecht: Springer Netherlands), 237–250.
Hinsinger, P., Betencourt, E., Bernard, L., Brauman, A., Plassard, C., Shen, J., et al. (2011). P for two, Sharing a scarce resource: Soil phosphorus acquisition in the rhizosphere of intercropped species. Plant Physiol. 156, 1078–1086. doi: 10.1104/pp.111.175331
Jakobsen, I., Abbott, L. K., and Robson, A. D. (1992). External hyphae of vesicular-arbuscular mycorrhizal fungi associated with Trifolium subterraneum L. New Phytol. 120, 371–380. doi: 10.1111/j.1469-8137.1992.tb01077.x
Jensen, E. S.. (1996). Grain yield, symbiotic N2fixation and interspecific competition for inorganic N in pea-barley intercrops. Plant Soil 182, 25–38. doi: 10.1007/BF00010992
Jensen, E. S., Carlsson, G., and Hauggaard-Nielsen, H. (2020). Intercropping of grain legumes and cereals improves the use of soil N resources and reduces the requirement for synthetic fertilizer N: a global-scale analysis. Agron. Sustain. Dev. 40:07. doi: 10.1007/s13593-020-0607-x
Juhos, K., Szabó, S., and Ladányi, M. (2015). Influence of soil properties on crop yield : a multivariate statistical approach. Int. Agrophysics 433–440. doi: 10.1515/intag-2015-0049
Kaggwa-Asiimwe, R., Andrade-Sanchez, P., and Wang, G. (2013). Plant architecture influences growth and yield response of upland cotton to population density. F. Crop. Res. 145, 52–59. doi: 10.1016/j.fcr.2013.02.005
Karasawa, T., and Takebe, M. (2012). Temporal or spatial arrangements of cover crops to promote arbuscular mycorrhizal colonization and P uptake of upland crops grown after nonmycorrhizal crops. Plant Soil 353, 355–366. doi: 10.1007/s11104-011-1036-z
Lauk, R., and Lauk, E. (2008). Pea-oat intercrops are superior to pea-wheat and pea-barley intercrops. Acta Agric. Scand. Sect. B Soil Plant Sci. 58, 139–144. doi: 10.1080/09064710701412692
Li, L., Li, S.-M., Sun, J.-H., Zhou, L.-L., Bao, X.-G., Zhang, H.-G., and Zhang, F.-S. (2007). Diversity enhances agricultural productivity via rhizosphere phosphorus facilitation on phosphorus-deficient soils. Proc. Natl. Acad. Sci. 104, 3–7. doi: 10.1073/pnas.0704591104
Li, L., Tilman, D., Lambers, H., and Zhang, F. S. (2014). Plant diversity and overyielding: Insights from belowground facilitation of intercropping in agriculture. New Phytol. 203, 63–69. doi: 10.1111/nph.12778
Lithourgidis, A. S., Dhima, K. V., Vasilakoglou, I. B., Dordas, C. A., and Yiakoulaki, M. D. (2007). Sustainable production of barley and wheat by intercropping common vetch. Agron. Sustain. Dev. 27, 95–99. doi: 10.1051/agro:2006033
Loreau, M., and Hector, A. (2001). Partitioning selection and complementarity in biodiversity experiments. Nature 412, 72–76. doi: 10.1038/35083573
Ludwig, F., Dawson, T. E., Prins, H. H. T., Berendse, F., and De Kroon, H. (2004). Below-ground competition between trees and grasses may overwhelm the facilitative effects of hydraulic lift. Ecol. Lett. 7, 623–631. doi: 10.1111/j.1461-0248.2004.00615.x
Mäder, P., Kaiser, F., Adholeya, A., Singh, R., Uppal, H. S., Sharma, A. K., et al. (2011). Inoculation of root microorganisms for sustainable wheat-rice and wheat-black gram rotations in India. Soil Biol. Biochem. 43, 609–619. doi: 10.1016/j.soilbio.2010.11.031
Mao, L., Zhang, L., Li, W., van der Werf, W., Sun, J., Spiertz, H., et al. (2012). Yield advantage and water saving in maize/pea intercrop. F. Crop. Res. 138, 11–20. doi: 10.1016/j.fcr.2012.09.019
Martin, M. P. L. D., and Snaydon, R. W. (1982). Intercropping barley and beans I. Effects of planting pattern. Exp. Agric. 18, 139–148. doi: 10.1017/S0014479700013612
Martin-Guay, M. O., Paquette, A., Dupras, J., and Rivest, D. (2018). The new green revolution: Sustainable intensification of agriculture by intercropping. Sci. Total Environ. 615, 767–772. doi: 10.1016/j.scitotenv.2017.10.024
Mathimaran, N., Jegan, S., Thimmegowda, M. N., Prabavathy, V. R., Yuvaraj, P., Kathiravan, R., et al. (2020). Intercropping transplanted pigeon pea with finger millet: Arbuscular mycorrhizal fungi and plant growth promoting rhizobacteria boost yield while reducing fertilizer input. Front. Sustain. Food Syst. 4, 1–12. doi: 10.3389/fsufs.2020.00088
Mathimaran, N., Sharma, M. P., Raju, M. B., and Bagyaraj, D. J. (2017). Arbuscular mycorrhizal symbiosis and drought tolerance in crop plants. Mycosphere 8, 361–376. doi: 10.5943/mycosphere/8/3/2
Moreira, M. Z., Scholz, F. G., Bucci, S. J., Sternberg, L. S., Goldstein, G., Meinzer, F. C., et al. (2003). Blackwell Publishing Ltd. Hydraulic lift in a neotropical savanna. Funct. Ecol. 17, 573–581. doi: 10.1046/j.1365-2435.2003.00770.x
Oljaca, S., Cvetkovic, R., Kovacevic, D., Vasic, G., and Momirovic, N. (2000). Effect of plant arrangement pattern and irrigation on efficiency of maize (Zea mays) and bean (Phaseolus vulgaris) intercropping system. J. Agric. Sci. 135, 261–270. doi: 10.1017/S0021859699008321
Padhi, A. K., Panigrahi, R. K., and Jena, B. K. (2010). Effect of planting geometry and duration of intercrops on performance of pigeonpea-finger millet intercropping systems. Indian J. Agric. Res. 44, 43–47.
Prieto, I., Padilla, F. M., Armas, C., and Pugnaire, F. I. (2011). The role of hydraulic lift on seedling establishment under a nurse plant species in a semi-arid environment. Perspect. Plant Ecol. Evol. Syst. 13, 181–187. doi: 10.1016/j.ppees.2011.05.002
Querejeta, J. I., Egerton-Warburton, L. M., and Allen, M. F. (2003). Direct nocturnal water transfer from oaks to their mycorrhizal symbionts during severe soil drying. Oecologia 134, 55–64. doi: 10.1007/s00442-002-1078-2
Raza, M. A., Cui, L., Qin, R., Yang, F., and Yang, W. (2020). Strip-width determines competitive strengths and grain yields of intercrop species in relay intercropping system. Sci. Rep. 10, 1–12. doi: 10.1038/s41598-020-78719-y
Saharan, K., Schütz, L., Kahmen, A., Wiemken, A., Boller, T., and Mathimaran, N. (2018). Finger millet growth and nutrient uptake is improved in intercropping with pigeon pea through “Biofertilization” and “Bioirrigation” mediated by arbuscular mycorrhizal fungi and plant growth promoting rhizobacteria. Front. Environ. Sci. 6:46. doi: 10.3389/fenvs.2018.00046
Schipanski, M. E., and Drinkwater, L. E. (2012). Nitrogen fixation in annual and perennial legume-grass mixtures across a fertility gradient. Plant Soil 357, 147–159. doi: 10.1007/s11104-012-1137-3
Schütz, L., Gattinger, A., Meier, M., Müller, A., Boller, T., Mäder, P., et al. (2018). Improving crop yield and nutrient use efficiency via biofertilization—A global meta-analysis. Front. Plant Sci. 8. doi: 10.3389/fpls.2017.02204
Sekar, J., Raju, K., Duraisamy, P., and Vaiyapuri, P. R. (2018). Potential of finger millet indigenous rhizobacterium Pseudomonas sp. MSSRFD41 in blast disease management-growth promotion and compatibility with the resident rhizomicrobiome. Front. Microbiol. 9, 1–16. doi: 10.3389/fmicb.2018.01029
Sekiya, N., Araki, H., and Yano, K. (2011). Applying hydraulic lift in an agroecosystem: Forage plants with shoots removed supply water to neighboring vegetable crops. Plant Soil 341, 39–50. doi: 10.1007/s11104-010-0581-1
Sekiya, N., and Yano, K. (2002). Water acquisition from rainfall and groundwater by legume crops developing deep rooting systems determined with stable hydrogen isotope compositions of xylem waters. F. Crop. Res. 78, 133–139. doi: 10.1016/S0378-4290(02)00120-X
Singh, D., Mathimaran, N., Boller, T., and Kahmen, A. (2019). Bioirrigation: a common mycorrhizal network facilitates the water transfer from deep-rooted pigeon pea to shallow-rooted finger millet under drought. Plant Soil 440, 277–292. doi: 10.1007/s11104-019-04082-1
Singh, D., Mathimaran, N., Boller, T., and Kahmen, A. (2020). Deep-rooted pigeon pea promotes the water relations and survival of shallow-rooted finger millet during drought—Despite strong competitive interactions at ambient water availability. PLoS ONE 15, 1–22. doi: 10.1371/journal.pone.0228993
Smith, S. E., and Read, D. (2008). “Mycorrhizas in agriculture, horticulture, and forestry,” in eds S. E. Smith, M. S. Read (Academic Press, London), 611–XVIII.
Stenberg, B.. (1998). Soil attributes as predictors of crop production under standardized conditions. Biol. Fertil. Soil 27, 104–112. doi: 10.1007/s003740050407
Thorsted, M. D., Olesen, J. E., and Weiner, J. (2006). Width of clover strips and wheat rows influence grain yield in winter wheat/white clover intercropping. F. Crop. Res. 95, 280–290. doi: 10.1016/j.fcr.2005.04.001
Willey, R. W., and Osiru, D. S. O. (1972). Studies on mixtures of maize and beans (Phaseolus vulgaris) with particular reference to plant population. J. Agric. Sci. 79, 517–529. doi: 10.1017/S0021859600025909
Xu, B. C., Li, F. M., and Shan, L. (2008). Switchgrass and milkvetch intercropping under 2:1 row-replacement in semiarid region, northwest China: aboveground biomass and water use efficiency. Eur. J. Agron. 28, 485–492. doi: 10.1016/j.eja.2007.11.011
Keywords: bioirrigation, drought, finger millet, intercropping, mycorrhiza, pigeon pea, rainfed agriculture, biofertilizer
Citation: Singh D, Mathimaran N, Sekar J, Ramalingam PV, Perisamy Y, Raju K, Raj R, King IO, Nanjundegowda TM, Narayanswamy MB, Chikkegowda BN, Siddegowda SM, Bagyaraj DJ, Mäder P, Boller T and Kahmen A (2021) Spatial Arrangement and Biofertilizers Enhance the Performance of Legume—Millet Intercropping System in Rainfed Areas of Southern India. Front. Sustain. Food Syst. 5:711284. doi: 10.3389/fsufs.2021.711284
Received: 18 May 2021; Accepted: 04 November 2021;
Published: 15 December 2021.
Edited by:
Maryke T. Labuschagne, University of the Free State, South AfricaReviewed by:
Deepranjan Sarkar, Banaras Hindu University, IndiaRakesh S, National Academy of Agricultural Research Management (ICAR), India
Copyright © 2021 Singh, Mathimaran, Sekar, Ramalingam, Perisamy, Raju, Raj, King, Nanjundegowda, Narayanswamy, Chikkegowda, Siddegowda, Bagyaraj, Mäder, Boller and Kahmen. This is an open-access article distributed under the terms of the Creative Commons Attribution License (CC BY). The use, distribution or reproduction in other forums is permitted, provided the original author(s) and the copyright owner(s) are credited and that the original publication in this journal is cited, in accordance with accepted academic practice. No use, distribution or reproduction is permitted which does not comply with these terms.
*Correspondence: Ansgar Kahmen, ansgar.kahmen@unibas.ch
†Present address: Devesh Singh, Division of Environmental Sciences, Yale-NUS College, Singapore, Singapore