Legume Cover Crop Contributions to Ecological Nutrient Management in Upper Midwest Vegetable Systems
- 1Department of Horticultural Science, University of Minnesota—Twin Cities, Saint Paul, MN, United States
- 2Department of Geography, Rutgers University, Piscataway, NJ, United States
- 3Department of Agronomy and Plant Genetics, University of Minnesota—Twin Cities, Saint Paul, MN, United States
Cover cropping, especially with legumes, is a critical approach to ecological soil nutrient management as a means to meet Sustainable Development Goal (SDG) 2, addressing food security issues via sustainable agriculture approaches. However, cover cropping in some of the most intensified food production regions of the northern U.S. is challenged by short growing seasons and harsh winters with variable temperatures and increasingly erratic snowfall. In this study, we explore the potential of winter annual legume cover crops to augment soil organic carbon (C) and nitrogen (N) stocks within a horticultural cropping system under climate conditions that allow only modest cover crop biomass production. We compared hairy vetch, cereal rye, red clover, and a hairy vetch/rye biculture in a randomized complete block design at two sites (North Central and Southwest Research and Outreach Centers in Minnesota) over 2 years. Cover crops were established in fall and terminated in spring prior to sweet corn planting, and soils sampled both at pre-cover crop termination and 2 weeks post-termination. We determined several indicators of C and N dynamics, including microbial biomass C (MBC), permanganate-oxidizable C (POXC), particulate organic matter C and N (POMC and POMN, respectively), extractable soil N (EXTN), and potentially mineralizable N (PMN). Out of all treatments evaluated, vetch production increased soil EXTN the greatest after 2 weeks, contributing two to 11 times more EXTN to soils compared to non-vetch treatments, meeting N requirements for sweet corn in three out of four site-years. Overall, time of sampling, either pre-or post-termination, consistently impacted soil measurements, with p < 0.05 in 20 out of 24 soil parameter × site-year combinations. Study results suggest that cover crops planted in colder northern climates during winter fallow periods can supply valuable N following spring termination, but termination effects on labile C and N pools are mixed. Our findings advance understanding of how cover cropping can support SDG 2 outcomes by assessing cover crop legume systems under biophysical conditions that challenge cover crop integration in agroecosystems.
Introduction
The Sustainable Development Goal (SDG) 2 calls for improved understanding of sustainable and ecological agriculture approaches that meet both sustainability and food production goals. With fewer than 10 years remaining to mobilize resources and take action, the time to pay targeted attention to localized solutions that result in increased reliance on ecological processes for food production is now. Integration of legume cover crops as “green manures” into annual cropping systems, especially those producing horticultural crops for human consumption, is of increasing global interest due to their potential to provide ecologically-sourced nitrogen (N) to crops, as well as improve long-term soil sustainability via contributions of organic matter (Robačer et al., 2016; Brennan, 2017; Seman-Varner et al., 2017; Wauters et al., 2021). In humid continental climates, which make up a large portion of global cropland, cover crops could serve to improve food security via targeted ecological management of soil nutrients by utilizing the typically fallow winter months for cover crop establishment and production.
Humid continental northern climates are a prime target for ecological nutrient management because high crop production potentials and demands have resulted in prevalence of monoculture, conversion of grassland to cropland, and high fertility inputs. These combined factors, in addition to a changing climate, have contributed to nutrient decoupling and resulting nutrient losses from these soils (Zhang et al., 2021). However, these northern climates present unique barriers to integrating winter annual cover crops in crop rotations. Summer crop planting windows are relatively short, and maximization of crop production during these periods means that there are frequently few growing degree days (GDD) available for fall cover crop establishment and spring maturation (Hively and Cox, 2001; Teasdale et al., 2004). Cover crop implementation is also limited by extreme minimum winter temperatures and variable snow cover (Larsen et al., 1987), which can threaten winter survival of many common cover crop species that thrive in warmer climates. These challenges limit general cover crop success and derived benefits, as winter cover crops must first establish successfully in the fall in order to promote winter survival, and most soil gains result from ample root and shoot biomass production (Puget and Drinkwater, 2001; Finney et al., 2016). Cooler regions would benefit from a wider range of cover crop options, especially those that include legumes.
While winter cover crop integration into row cropping systems is well studied, vegetable systems are less understood, and may offer unique opportunities for winter annual cover crops in these northern climates because of the shorter growing season required by some vegetables as compared to row crops (Dabney et al., 2001). Cover crop adoption in northern region vegetable systems is a relatively recent phenomenon. Since certified organic vegetable farmers are required to both build soils and diversify rotations, and since they rely on ecologically-based fertility inputs over synthetic ones, these farmers have been early adopters of cover crops as a tool for ecological soil management. A survey of 152 organic vegetable farmers in Wisconsin showed cover crop adoption has increased dramatically from <20% of farmers in the 1980s to 92% in 2013, with most adoption occurring after 2005 (Moore et al., 2016). The primary driver encouraging cover crop use by these adopters was perceived soil benefit, including increases in soil fertility, soil organic matter, and structure.
Diversification with winter annual legume cover crops may be easier with shorter-duration warm season vegetable crops, such as zucchini or cucumbers, than longer-duration ones, such as sweet corn or tomatoes, since crops that mature earlier in the fall leave more time for cover crop establishment prior to winter. Rotation of winter annual cover crops with longer-duration warm season summer vegetables may be more difficult, as longer crops leave less time for cover crop establishment. This, combined with early spring termination to accommodate cool season vegetable crop planting, means that nutrient benefits from winter cover crops may not be achieved due to resulting reduced growing degree days and biomass production (Mirsky et al., 2017). Sweet corn is a major horticultural crop in the Upper Midwest (FarmProgress, 2018), and its higher days to maturity (75–90 days) relative to other warm season vegetables make it a good model for evaluation of cover crop rotations in longer-duration warm season crops in cool climates. The viability of these strategies and the potential for legumes to supply significant N to subsequent horticultural crops under highly restrictive climatic conditions remains largely unknown.
Legumes are valuable for their capacity to fix atmospheric N and improve soils' long-term N cycling activity and organic matter accrual (Marriott and Wander, 2006; Lynch, 2015). Cover crop N contributions are a function of shoot and root biomass production, yet since legume cover crop inclusion in northern climate cropping systems can be restricted by establishment constraints, poor winter survival (Hively and Cox, 2001), and limited winter-hardy varieties (Wilson, 2007; Silva, 2014), these benefits are commonly not achieved. Poorly performing legume cover crops can limit both aboveground and belowground biomass contributions, reducing potential benefits from these crops via N fixation and soil N uptake. Net N mineralization from cover crop decomposition is dependent on residue quantity, quality, incorporation method, as well as soil moisture and temperature, with legume residues known to mineralize more rapidly than those of grasses (O'Connell et al., 2015). While C/N ratios vary depending on maturity and species, spring-terminated legumes in the Upper Midwest can be as low as 10:1 (Lawson et al., 2015), which encourages rapid mineralization prior to cash crop planting. In fact, an analysis of legume cover crop monocultures and bicultures across nine farms in southeastern Michigan showed an increase in C and N mineralization after 2 years relative to the no cover crop control, as well as other labile SOM parameters such as free particulate organic matter (POM) and protected POM (Blesh, 2019). Less is known about even shorter-term effects (~weeks to months) of cover crop residue incorporation on labile nutrient parameters such as permanganate-oxidizable carbon (POXC), particulate organic matter (POM) carbon (C) and N, microbial biomass C, and potentially mineralizable N (PMN), especially in horticultural systems. This time frame is of interest due to these pools' potential to provide nutrients to a cash crop immediately following a cover crop. Together with bioavailable N, these parameters may provide a higher resolution of nutrient coupling dynamics in systems relying on ecological nutrient management.
Understanding the dynamics between cover crop biomass characteristics and soil nutrient cycling processes is a key step to achieve sustainable agroecosystem nutrient management in cooler regions. Our goal was to explore the extent to which winter annual cover crops influence short-term soil C and N cycling dynamics to realize benefits in cold regions with narrow biomass accumulation windows. Previous studies have evaluated winter annual cover crop biomass accumulation in the Upper Midwest (Silva, 2014; Appelgate et al., 2017; Noland et al., 2018; Perrone et al., 2020), cover crop influences on labile soil C and N pools (Drinkwater et al., 1998; Puget and Drinkwater, 2001; Bair et al., 2008), and crop dynamics following cover crop termination (Griffin et al., 2000; Leavitt et al., 2011). Our objective was to evaluate short-term (~2 weeks) impacts of cover crop biomass incorporation in a horticultural system on key indicators of soil C and N cycling over the period of initial cover crop decomposition after cover crops are terminated. We evaluated hairy vetch (Vicia villosa Roth), cereal rye (Secale cereale L.), red clover (Trifolium pratense L.), and a hairy vetch/rye biculture compared to a fallow control in a sweet corn production system at University of Minnesota research and outreach centers in southwestern and north-central Minnesota. These sites were chosen because of their contrasting soils and site histories, since legacy effects of management may impact soils' responses to inputs (Tardy et al., 2015; Ontivero et al., 2020; Hermans et al., 2021). We hypothesized that despite relatively short cover crop establishment and maturation periods, legume and rye treatments would both impact soil nutrient cycling dynamics following spring termination and would differ in their effects on soil nutrient responses because of differences in biomass amount and biochemical properties of residues.
Materials and Methods
Site Descriptions
The experiment was conducted from September 2015 through August 2017 at two University of Minnesota experiment stations, the North Central Research and Outreach Center, Grand Rapids, MN (LAT: 47.243347, LONG:−93.492622) and the Southwest Research and Outreach Center, Lamberton, MN (LAT: 44.239366, LONG:−95.309855). Grand Rapids is in USDA-designated plant hardiness zone 3b, and Lamberton is in zone 4b. At both locations, fall 2016 was unseasonably wet (see Supplementary Material). Lamberton received above average spring rainfall in both years, and Grand Rapids was unseasonably dry during July 2016. Snow cover was generally above 10 cm between January 1 and April 1, although this differed across site and year (See Supplementary Material). Soils at the two locations differ widely (Table 1, adapted from Perrone et al., 2020). For example, Grand Rapids soil was an Alfisol with 2.8% SOM while Lamberton soil was a Mollisol with 4.3% SOM.
Lamberton experimental fields had an oat (Avena sativa) cover crop preceding experimental cover crop seeding in fall 2015 and 2016 with a 25+ year history of organic management. In contrast, Grand Rapids experimental fields, also certified organic, had been converted from long-term rhizomatous quackgrass (Agropyron repens) and other perennial grasses to annual production the year prior to the experiment and were cover cropped with winter wheat (Triticum aestivum L.) prior to beginning the experiment in fall 2015. In 2016, the preceding crop at Grand Rapids was cereal rye. These two locations had contrasting soil characteristics and management histories; therefore, we refer to the Lamberton site as annual cropping history (ANN) and the Grand Rapids site as unmanaged perennial history (PER) to highlight these differences.
Experimental Design
The experiment was a randomized complete block design replicated across 4 blocks over 2 years, 2015–2016 (Y1) and 2016–2017 (Y2). Separate fields were used at both sites in both years and were characterized as four separate site by year combinations (ANN Y1, PER Y1, ANN Y2, PER Y2). Cover crop species rye (Secale cereale L., RYE, Johnny's Seeds, Winslow, ME), medium red clover (Trifolium pratense L., CLO, Johnny's Seeds, Winslow, ME), hairy vetch ecotype 1 (Vicia villosa Roth, variety-not-stated, Albert Lea Seed, Albert Lea, MN), hairy vetch ecotype 2 (Vicia villosa Roth, variety-not-stated, Buckwheat Growers, Wadena, MN), and a rye/hairy vetch ecotype 2 biculture (MIX) were planted at the two research stations in September 2015. For analyses, vetch ecotypes 1 and 2 were combined (abbr. VET) as they were unable to be differentiated at the level of variety. Due to space limitations, vetch ecotype 1 was eliminated from PER Y2 experimental plots but included at ANN Y2. A bare-ground control (NOCC) was also included at both sites in both years. A pre-study trial eliminated Austrian winter pea as a legume treatment in this study due to complete winter kill at both locations. Plots were 3 × 7.6 m, and blocks were separated by 3 m buffers kept free of weeds.
Cover Crop Management
Seeding rates followed recommended rates for organic production (SARE., 2007). Vetch was planted at 28.0 kg ha−1, clover at 13.5 kg ha−1, rye at 117.7 kg ha−1, and the mix contained 28.0 kg ha−1 of vetch and 84.1 kg ha−1 of rye. Planting depth was 1.25 cm for clover and 2.50 cm for hairy vetch and rye treatments. Cover crop planting occurred in the last week of August or first week of September (for specific dates, see Table 2, adapted from Perrone et al., 2020). A 6-row Jang seeder (Mechanical Transplanter, Holland, MI) was used to plant covers at PER Y1 and Y2 and a five-foot wide Marliss drill (Remlinger Manufacturing Company, Kalida, OH) was used at ANN Y1 and Y2.
Cover crops were terminated in late May or first week of June (Table 2) and growth stages recorded according to the BBCH-scale, a uniform coding of phenologically similar growth stages of all mono- and dicotyledonous plant species (Meier, 2001). Rye had reached or surpassed 55 in the BBCH-scale (50% heading) at both locations in Y1 but only achieved 37–41 in the BBCH-scale (stem elongation and early booting stage) at PER Y2 and early heading in ANN Y2. At that phenology, percentage of vetch flowering was 601 in the BBCH-scale (5–15%), matching cover crop development and termination of similar studies (Leavitt et al., 2011). Optimal maturity for maximum N accumulation at termination in vetch occurs at ~605 in the BBCH-scale (50% flowering; SARE., 2007), and 65 in the BBCH-scale for rye (anthesis) is commonly used as a termination point in roller crimping and reduced tillage cover cropping systems (Mirsky et al., 2011). In our study, cover crops were terminated prior to optimal maturity to provide an adequate growing season for sweet corn.
Cover crops were terminated using a John Deere Z950 mower (Deere & Co., Moline, IL) in PER Y1 and Y2 and a Loftness 90M flail mower (Loftness Manufacturing, Hector, MN) in ANN Y1 and Y2. Mower height was set to maximum height (14 cm). Both sites were tilled within 1 week of cover crop termination. Plots in ANN Y1 and Y2 were tilled using a JD 236 tandem folding disk (Deere & Co., Moline, IL) and plots in PER Y1 and Y2 were rototilled with a King Kutter TG-60 YK rototiller (King Kutter, Winfield, AL). In both locations, secondary bed preparation occurred prior to sweet corn planting. In ANN Y1 and Y2, several passes were made with a Kuhn HK 3004D rotary power harrow (Kuhn North America Inc., Brodhead, WI), and several additional rototiller passes were made in PER Y1 and Y2.
Cover Crop Biomass and Soil Sampling
Cover crop and weed biomass were sampled immediately prior to cover crop termination (Table 2), pooling four randomly cut 0.1 m2 quadrats per plot (Wiegert, 1962). Biomass was dried at 60°C for 72 h. After determining dry weight, samples were ground and passed through a 2 mm sieve and then pulverized using a 2010 Geno/Grinder ball grinder (SPEX SamplePrep, Metuchen, NJ) using 5 mm stainless steel ball bearing balls (Craig Ball Sales, Seaford, DE) for 3–10 min at 1,500 rpm.
Soil samples to 15 cm depth were collected immediately prior to cover crop termination as a baseline and ~2 weeks following termination. These time points were chosen to assess the contribution of cover crop biomass to labile C and N parameters immediately prior to cash crop planting so that the in-season nutrient transfer potential as a result of cover crop biomass incorporation could be evaluated. In each plot, 8–12 2.5-cm diameter cores were collected randomly after removing surface residue, composited, and mixed until homogenous. Fresh soils were immediately placed into coolers after sampling and temperature was maintained at 4°C until conducting analyses within 7 days. Samples for dry analyses were dried at 35°C for 48 h and then ground to 2 mm. Soil moisture was determined gravimetrically.
Soil Analyses
Permanganate-Oxidizable Carbon
Permanganate oxidizable carbon (POXC) is a fraction of active C, chemically defined by the quantity of potassium permanganate reduced in reaction with a quantity of soil (Weil et al., 2003). POXC was performed based on methods outlined by Culman et al. (2012). Briefly, 20 mL 0.02 M KMnO4 was added to 2.5 g soil, shaken for 2 min, and settled for 10 min. Absorbance of the resulting supernatant was determined on a 300 μL subsample using a spectrophotometer at 550 nm and averages were taken across the three replicates and converted to mg kg−1 soil using the following equation:
where 0.02 M KMnO4 is the concentration of the starting solution; a and b are the intercept and slope of the standard curve, respectively; Abs is the absorption of the sample; 9,000 mg is the quantity of C oxidized by 1 mol KMnO4; 0.02 L is the volume of KMnO4 solution reacted; and x is the mass of soil (kg) in the reaction.
Particulate Organic Matter Fractionation
Coarse fraction particulate organic matter (CF POM) > 53 μm was determined using size fractionation by shaking soils with 10% w/v sodium hexametaphosphate for 4 h, then passing contents through a 53 μm sieve (Wander, 2004). Contents collected on sieves were transferred to metal weighing tins and dried for 24 h at 65°C. Organic C and N contents were determined using a VarioMax C/N analyzer (Elementar, Mt. Laurel, NJ) and reported as % C or % N.
Microbial Biomass C
Microbial biomass C (MBC) was determined by chloroform-fumigation extraction technique (Brookes et al., 1985; Vance et al., 1987). Total C of microbial biomass extracts was determined using a Shimadzu TOC-L/TN analyzer (Shimadzu, Kyoto Prefecture, Japan). Microbial biomass C was calculated by subtracting the total C in non-fumigated samples from total C in fumigated samples, corrected for initial soil moisture levels and amount of soil reacted. Microbial biomass C levels were not corrected for extraction efficiency, therefore representing a comparative flush of C across treatments rather than total microbial biomass (Fierer and Schimel, 2002).
Potentially Mineralizable N and Extractable N
Potentially mineralizable nitrogen (PMN) was determined using a 7-day anaerobic incubation technique, based on Drinkwater et al. (1997). Total extractable N was determined using Shimadzu TOC-L/TN analyzer pre- and post-incubation. PMN was calculated by subtracting the total N in baseline (pre-incubated) samples from total N in incubated samples. Values were corrected based on amount of dry soil reacted in solution. The initial baseline extractable N, which consists of mineral and dissolved organic forms of N, is hereafter referred to as EXTN.
Statistical Analyses
Soil parameters were analyzed using a mixed effects model with cover crop, time, and cover crop × time as fixed effects and block as a random effect (SAS 2006). Significant treatment by site by year interactions prevented pooling across locations and years, so each was analyzed separately. ANOVA model assumptions of normal variances and homoschedasticity were evaluated using residual plots.
Square root transformations were performed in PER Y1 for POMC, POMN, and PMN, in ANN Y1 for POM, and in ANN Y2 for EXTN and log-transformations were conducted for EXTN in PER Y1 to meet ANOVA assumptions. Means and standard errors were back-transformed (Jorgensen and Pedersen, 1998). Mean differences within each site by year combination in biomass, soil, and yield parameters were compared across cover crop treatments using post-hoc Tukey's HSD tests. All figures were made using the {ggplot2} package in R (Wickham, 2009).
Results
Time of sampling and cover crop treatment were the two independent variables evaluated in this study. Time of sampling considerably impacted soil parameters, with p < 0.05 in 20 out of 24 soil parameter by site by year combinations (Table 3). In contrast, cover crop treatment did not impact soil parameters in most cases, and the NOCC control did not differ from some, or all, of the cover cropped treatments. For example, POMC, POMN, and MBC were not affected by cover crops, except in ANN Y1. Similarly, POXC was not affected by cover crop planting in any site-year. Cover crop by time interactions occurred most frequently for EXTN (PER Y1, p < 0.001; ANN Y1, p < 0.001; ANN Y2, p = 0.007) and did not occur for POXC or POMN parameters. Overall cover crop performance, quantity, and quality in this experiment are described in Perrone et al. (2020), showing considerable differences in biomass produced each study year. To briefly summarize, ANN Y2 exhibited the overall highest biomass production, with treatments yielding 4.6–9.3 Mg ha−1, while PER Y2 exhibited the least amount of overall biomass, with treatments ranging from 0.2–4.9 Mg ha−1. In PER Y2, substantial winter kill occurred. Vetch monocultures contributed among the greatest total N from aboveground biomass across all site by year combinations, and clover contributed among the lowest amounts of total N due to overall lower biomass production.
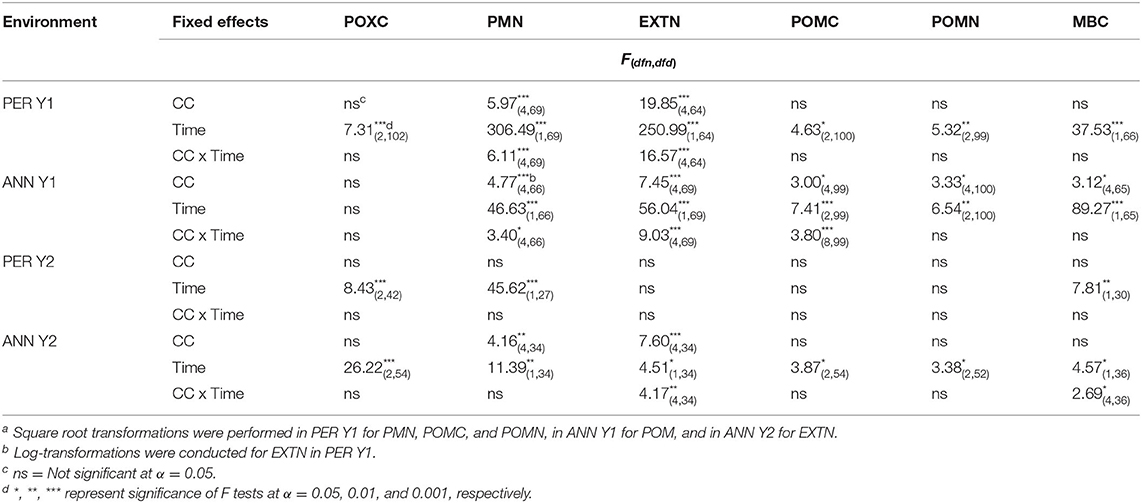
Table 3. Mixed-model analysis of variance of permanganate oxidizable carbon (POXC), potentially mineralizable nitrogen (PMN)a, extractable N (EXTN)a, b, particulate organic matter C and N (POMC and POMN)a, and microbial biomass C (MBC) due to cover crop species (CC), time, and CC x time.
Time was a significant factor for POXC across environments except ANN Y1. From pre- to post-termination, POXC decreased in PER Y1 and ANN Y2, but increased in PER Y2, when cover crops performed poorly. Similarly, the time at which soil was sampled affected MBC in all sites and years, as well as POMC and POMN in all sites and years excluding PER Y2, again when biomass production was lowest. In all cases where sampling time was significant, POMC and POMN decreased after termination. Surprisingly, the inclusion of cover crop biomass quantity, defined as the amount of aboveground material produced by cover crop treatments, as a covariate did not affect any soil parameter and thus was omitted from analysis.
Cover crop species effects on soil responses after termination were mixed across sites, years, and response variables (Table 3). In particular, vetch production impacted extractable soil N (EXTN), with EXTN increasing more in VET plots than RYE and CLO plots in a majority of site by year combinations following cover crop biomass incorporation (Figure 1). Approximately 2 weeks post-termination, EXTN was 2 times greater in VET than in CLO and RYE treatments in ANN Y1. The following year (ANN Y2), the difference was 11.5 times greater (means presented in Supplementary Material). In PER Y1, EXTN in VET was 35% higher than CLO and RYE post-termination. Treatments RYE and CLO consistently exhibited among the lowest soil EXTN post-termination, in some cases lower than NOCC, suggesting N immobilization.
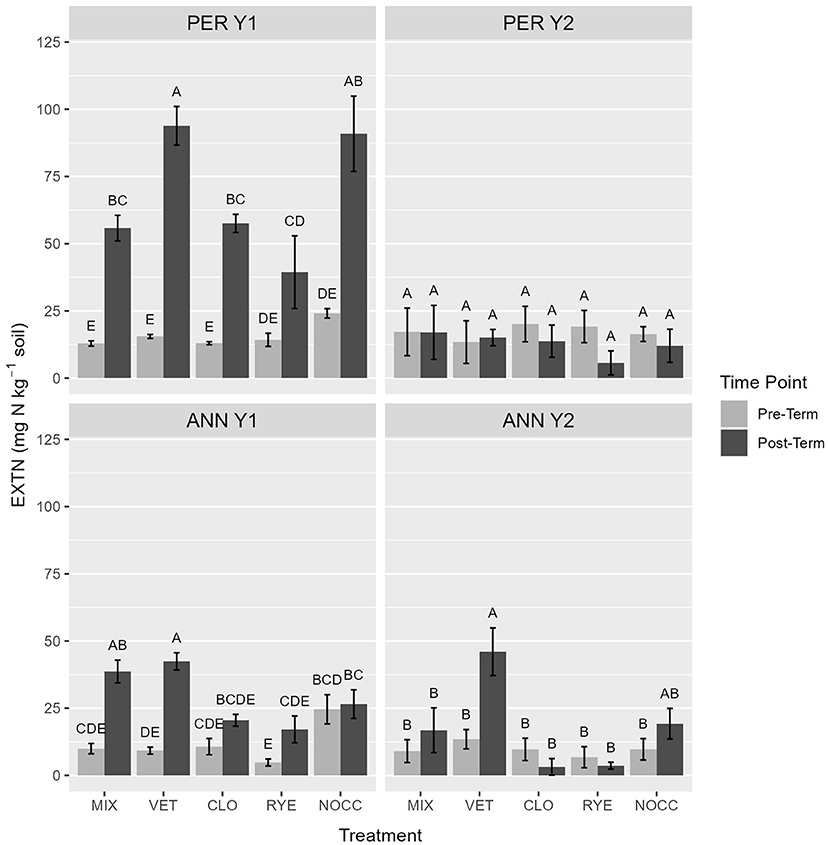
Figure 1. Extractable nitrogen (mg N kg soil−1) at experimental environments pre- vs. post-termination. Letters represent means separation within environment performed using a Tukey Honest Significant Difference post-hoc test (α = 0.05). Error bars indicate plus or minus one standard error of the mean.
Potentially mineralizable nitrogen varied by site, year, time, and cover crop species (Figure 2), but few clear trends were evident. In PER Y1—the year that land was converted from unmanaged perennial grasses to annual crop production and cover crop biomass production was highest—PMN increased following cover crop termination and incorporation in all treatments relative to the NOCC control (p < 0.001). In PER Y2, when little biomass was produced, all plots showed a sharp decrease in PMN post-termination, as compared to levels pre-termination, by ~50% (p < 0.001). For many soil parameters, PER Y1 exhibited greater overall values than in other site-years. For example, EXTN and PMN were considerably greater at PER Y1, with post-termination EXTN values ~5 times greater, and post-termination PMN values ~3 times greater, in Y1 than Y2. Additionally, POMC was ~1.75 times higher in PER Y1 than all other site-years.
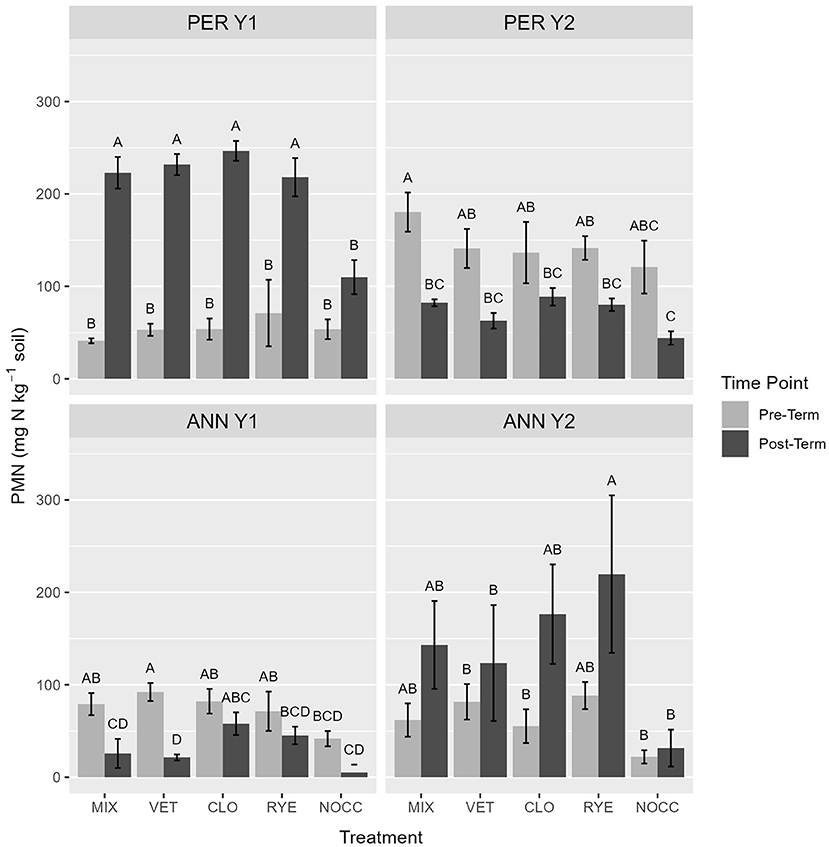
Figure 2. Potentially mineralizable nitrogen (mg N kg soil−1) at experimental environments pre- vs. post-termination. Letters represent means separation within environment performed using a Tukey Honest Significant Difference post-hoc test (α = 0.05). Error bars indicate plus or minus one standard error of the mean.
Discussion
Cover Crop Impacts on C and N Pools Were Generally Modest
We hypothesized that despite relatively short establishment and maturation periods, cover cropped treatments would impact short-term soil nutrient cycling dynamics following spring termination, and would differ in their soil responses based on amount of biomass contributed to soils. Instead, winter cover crop treatments drove only minimal changes to soil C and N pools relative to the no cover crop control, with no observed differences correlated to the relative amounts of biomass contributed.
In Y1 at the perennial history site, EXTN in all plots increased following termination. This included increased EXTN in the no-cover crop control, but the degree of increase in the control was less than in cover cropped plots, suggesting some impact from mineralization of new biomass in cover cropped plots. In the same site and year, PMN increased in all plots except the no-cover crop control, suggesting the high biomass produced in this year increased the pool of plant available N that can be mineralized from labile SOM pools over the long-term. This site had a unique management legacy in that plots were established on an abandoned field, where perennial grasses had dominated for at least two decades until field preparations in summer 2014. Since perennial vegetation can increase organic N stocks (Cambardella and Elliott, 1992; Kantola et al., 2017), while landscape conversion rapidly reduces oxidizable C stocks and microbial biomass (DuPont et al., 2010), it is possible that soil disturbance resulting from plot management, cover crop termination, and mechanical weed control required in organic systems increased mineralization rates of existing protected and stabilized organic matter pools at this site in the first year of the study. However, since we did not include an undisturbed control in our experiment, the effect of disturbance-related management practices on C and N pools remains speculative. The higher percentages of POMC and POMN, a fraction largely comprised of leaf and root fragments (Wander, 2004), observed at the perennial history site pre-termination in Y1 in comparison to all other site-years may also be related to this landscape legacy. This increased POM persisted to a lesser extent in Y2 at that location, with no effect of time of sampling on observed values. Notably, both POMC and POMN also declined after cover crop termination in both years at the annual cropping history site.
Permanganate oxidizable C is thought to be a source of labile carbon that is sensitive to changes in agricultural management (Culman et al., 2012), but less is known about individual species effects on POXC. While evidence suggests cover crop species can affect POXC and that an interaction between species and sampling time may exist (Ghimire et al., 2019), we found no effect of cover crop species on POXC in our study. Instead, POXC was influenced by location and sampling time. At the perennial history site in Y1, POXC was higher than other reported cultivated agricultural fields (Diederich et al., 2019), possibly due to the plot's management history and long-term legacy of living roots in the soil (Ginakes et al., 2020). Values of POXC in Y2 at this site are more typical for a soil under annual crop production (Diederich et al., 2019). At the perennial site in Y2, an early snowfall followed by an atypical freeze-thaw cycle in the fall (Supplementary Material) reduced winter survival of cover crops and may have accounted for the lack of influence of cover crop treatments on soil parameters. Indeed, VET and CLO treatments experienced significant winter kill, while RYE only produced about 63% of the biomass from the previous year, and roughly half the biomass as the annual cropping history site (Perrone et al., 2020). It is also possible that measurable impacts on the observed C pools may occur beyond the sampling timeline of this study as cover crop residue undergoes further decomposition, for example after the 2 week window between termination and sampling (Sievers and Cook, 2018).
The absence of cover crop biomass quantity and species impact on soil C parameters contradicts long-term evidence from corn cropping systems showing correlations between higher total C inputs and soil C (Coulter et al., 2009), yet likely reflects the need for decomposition models that reflect the interaction between microbial functioning and biomass quality, as well as macrobiotic and abiotic influences on SOM transformations (Wieder et al., 2014; Campbell and Paustian, 2015). That is, influences such as microbial community composition, biochemical characteristics of crop residues, invertebrate population dynamics, and soil temperature and moisture may be more likely to change over a 2-week period and therefore illustrative of short-term soil changes due to residue incorporation. These complex models are especially needed in systems that rely on ecological nutrient management and may be distinct in organic systems and those utilizing legume inputs.
Functioning of Legumes in Temperate Zone Horticultural Crop Agroecosystems
The vetch treatment had the strongest effect on soil N parameters in this study. Data supported that while most cover crop treatments impacted EXTN to some degree, especially in Y1 at both sites, the vetch treatment consistently ranked among the highest EXTN post-termination. Further, according to a recommendation of >25 ppm (Peterson, 2020), the amount of EXTN in vetch plots 2 weeks after termination was sufficient to fertilize a sweet corn crop at the perennial history site in Y1 (76.55 ± 7.15 mg N kg−1 soil), the annual cropping history site in Y1 (42.43 ± 2.14 mg N kg−1 soil), and the annual cropping history site in Y2 (39.16 ± 4.50 mg N kg−1 soil; see Supplementary Material). Vetch biomass at the perennial history site in Y2 was low, likely impacting the low EXTN observed after termination at this site and year. Overall, the amount of N released and measured as EXTN by cover cropped treatments in our experiment is similar to other studies employing winter annual legumes (Kuo and Sainju, 1998; St. Luce et al., 2013) and general crop residues (Christensen and Olesen, 1998). Potentially mineralizable nitrogen was comparable across both legume and grass treatments in almost all site-years, suggesting that legumes and grasses may not differ in terms of contribution of soil organic N in the form of PMN over short decomposition windows, especially when total biomass N values are similar.
Cover Crops and Ecological Nutrient Management in Vegetable Systems
This study showed only marginal contributions of winter cover crops to soil N and C pools in systems growing longer-duration vegetable crops, such as sweet corn, 2 weeks after termination. Although cereal rye and similar cool-season grasses remain the most common cover crops in cooler climates (Singer, 2008), opportunities for diversifying northern vegetable rotations with legumes and less common non-legume species exist, but face management challenges. In fall-planted cover crop systems, weed biomass is frequently greater in legume and brassica monocultures compared to grasses, driven by greater growing degree days that support weeds during early germination of weakly-competitive fall cover crops (Baraibar et al., 2018). When grown in mixtures, legumes are frequently outcompeted by non-legume components (Lawson et al., 2013; Wauters et al., 2021), reducing the value of legumes as an ecological nutrient management strategy. Clearly, work is needed to optimize legume success. Despite this competition, a metanalysis of cover crop N accumulation showed N content of hairy vetch-cereal rye mixtures to be 150% greater than that of cereal rye monocultures (Thapa et al., 2018), indicating a clear N benefit if optimization of legume performance can be realized. Laboratory incubations further support that that “fine-tuning” of nutrient delivery may be possible, where a pure hairy vetch and a 75% rye-25% hairy vetch mixture were found to release similar amounts of N after 70 days, with the initial release of N from the pure vetch stand higher than the mixture (Lawson et al., 2013).
Integration of cover crops with a diversity of available vegetable cash crops, each with unique days to maturity, could provide unique timing windows for cover crop inclusion in rotation between shorter duration crops. Horticultural systems thus may allow one to “mix and match” cash and cover crop species to fit available fallow periods. However, vegetable farmers in the Upper Midwest often grow multiple consecutive vegetable crops during the short growing season, which makes inclusion of cover crops into short rotational planting windows challenging if systems are intensified with fewer crops and higher acreages (Brainard et al., 2013; Moore et al., 2016). To best take advantage of the flexibility that vegetable rotations can offer, alternative rotational systems must be further explored. For example, cover crops inserted into short planting windows between cool season spring and fall vegetable crops could take advantage of a summer fallow period that, in cooler regions, is too short to cultivate a longer-duration warm season crop such as tomatoes or peppers. However, such cover cropping methods may reduce yields of subsequent crops, illustrating the challenges of cover crop integration in intensive horticultural systems in temperate zones. When comparing broccoli yields following short season (30–50 d) summer-planted cover crops in Minnesota and Wisconsin, yields in bare plots were found to be 30% higher than any cover cropped treatment in MN (p = 0.062), and 26% in WI (p = 0.096; Wauters et al., 2021). Clearly, optimization of warm season cover cropping systems in cool northern climates is still needed.
Ecological nutrient management in vegetable systems is undeniably complex, since the many opportunities for cover crop inclusion means that residue will be provided in varied amounts at different points in the growing season, thereby interacting with key drivers that govern nutrient provision via mineralization, including seasonal soil moisture and temperature. Our study provided data to support that even with short growing seasons that may limit aboveground biomass accumulation, winter cover cropping could provide benefits to soil N pools in the spring following termination, especially to slow N pools such as potentially mineralizable nitrogen. Because organic systems frequently suffer from N deficiencies, augmenting both available and organic forms of N via cover crops could improve crop yields via ecological nutrient provision (Berry et al., 2002; Drinkwater and Snapp, 2007), ultimately serving as a model to improve capacity for global food production.
Conclusion
Our study results suggest that cover crops, especially vetch, planted in colder northern climates during winter fallow periods can supply N following spring termination and contribute to longer term pools of labile C and N, but that these outcomes are highly dependent on site history and specific climatic events. The utilization of cover crops in locations with long winter seasons, regions which often intersect with the most productive and intensified agricultural regions in the U.S., provides an opportunity to shift agricultural paradigms toward greater farmer reliance on ecological nutrient management strategies. However, complex modeling of SOM transformations may be needed to optimize cover crop benefits. Data that supports introduction of cover crops into a wide range of vegetable production systems (organic, sustainable, or otherwise) will ultimately support a broad but often overlooked audience of farmers in adopting practices that supply nutrients to cash crops. A call to action to address SDG 2 is not only improved understanding of ecological nutrient management through research efforts, but also parallel farmer encouragement to understand and apply such principles in their farming context.
Data Availability Statement
The raw data supporting the conclusions of this article will be made available by the authors, without undue reservation.
Author Contributions
SP and AL were graduate students on the project and lead all fieldwork, and co-contributed to manuscript body. AL wrote the original draft and SP significantly modified later versions. JG supervised graduate student field experiments, project design, and reviewed and edited multiple drafts of the manuscript. NJ supervised graduate students and contributed to drafts of the manuscript. SW led statistical analysis. TS contributed to conceptual framework of process, experimental design, and development of figures. All authors contributed to the article and approved the submitted version.
Conflict of Interest
The authors declare that the research was conducted in the absence of any commercial or financial relationships that could be construed as a potential conflict of interest.
Publisher's Note
All claims expressed in this article are solely those of the authors and do not necessarily represent those of their affiliated organizations, or those of the publisher, the editors and the reviewers. Any product that may be evaluated in this article, or claim that may be made by its manufacturer, is not guaranteed or endorsed by the publisher.
Acknowledgments
We gratefully acknowledge the support of the North Central Sustainable Agriculture Research and Education program of USDA project number LNC14-364 and the University of Minnesota's Forever Green Initiative for funding and the reviewers that graciously volunteered their time to provide this manuscript with meaningful feedback.
Supplementary Material
The Supplementary Material for this article can be found online at: https://www.frontiersin.org/articles/10.3389/fsufs.2022.712152/full#supplementary-material
References
Appelgate, S. R., Lenssen, A. W., Wiedenhoeft, M. H., and Kaspar, T. C. (2017). Cover crop options and mixes for upper midwest corn–soybean systems. Agron. J. 109, 968–984. doi: 10.2134/agronj2016.08.0453
Bair, K. E., Davenport, J. R., and Stevens, R. G. (2008). Release of available nitrogen after incorporation of a legume cover crop in concord grape. HortScience 43, 875–880. doi: 10.21273/HORTSCI.43.3.875
Baraibar, B., Mortensen, D. A., Hunter, M. C., Barbercheck, M. E., Kaye, J. P., Finney, D. M., et al. (2018). Growing degree days and cover crop type explain weed biomass in winter cover crops. Agron. Sustain. Dev. 38, 65. doi: 10.1007/s13593-018-0543-1
Berry, P., Sylvester-Bradley, R., Philipps, L., Hatch, D., Cuttle, S., Rayns, F., and Gosling, P. (2002). Is the productivity of organic farms restricted by the supply of available nitrogen? Soil Use Manage 18, 248–255. doi: 10.1079/SUM2002129
Blesh, J. (2019). Feedbacks between nitrogen fixation and soil organic matter increase ecosystem functions in diversified agroecosystems. Ecol. Appl. 29, 1–12. doi: 10.1002/eap.1986
Brainard, D., Peachey, R., Haramoto, E., Luna, J., and Rangarajan, A. (2013). Weed ecology and nonchemical management under strip-tillage: Implications for northern U.S. vegetable cropping systems. Weed Tech. 27, 218–230. doi: 10.1614/WT-D-12-00068.1
Brennan, E. B. (2017). Can we grow organic or conventional vegetables sustainably without cover crops? Horttechnology 27, 151–161. doi: 10.21273/HORTTECH03358-16
Brookes, P. C., Landman, A., Pruden, G., and Jenkinson, D. S. (1985). Chloroform fumigation and the release of soil nitrogen: a rapid direct extraction method to measure microbial biomass nitrogen in soil. Soil Biol. Biochem. 17, 837–842. doi: 10.1016/0038-0717(85)90144-0
Cambardella, C. A., and Elliott, E. T. (1992). Particulate soil organic-matter changes across a grassland cultivation sequence. Soil Sci. Soc. Am. J. 56, 777–783. doi: 10.2136/sssaj1992.03615995005600030017x
Campbell, E., and Paustian, K. (2015). Current developments in soil organic matter modeling and the expansion of model applications: a review. Environ. Res. Lett. 10, 123004. Available online at: https://iopscience.iop.org/article/10.1088/1748-9326/10/12/123004
Christensen, B. T., and Olesen, J. E. (1998). Nitrogen mineralization potential of organomineral size separates from soils with annual straw incorporation. Eur. J. Soil Sci. 49, 25–36. doi: 10.1046/j.1365-2389.1998.00130.x
Coulter, J. A., Nafziger, E. D., and Wander, M. M. (2009). Soil organic matter response to cropping system and nitrogen fertilization. Agron. J. 101, 592–599. doi: 10.2134/agronj2008.0152x
Culman, S. W., Snapp, S. S., Freeman, M. A., Schipanski, M. E., Beniston, J., Lal, R., et al. (2012). Permanganate oxidizable carbon reflects a processed soil fraction that is sensitive to management. Soil Biol. Biochem. 76, 494–504. doi: 10.2136/sssaj2011.0286
Dabney, S. M., Delgado, J. A., and Reeves, D. W. (2001). Using winter cover crops to improve soil and water quality. Commun. Soil Sci. Plant Anal. 3624, 1221–1250. doi: 10.1081/CSS-100104110
Diederich, K. M., Ruark, M. D., Krishnan, K., Arriaga, F. J., and Silva, E. M. (2019). Increasing labile soil carbon and nitrogen fractions require a change in system, rather than practice. Soil Sci. Soc. Am. J. 83, 1733–1745. doi: 10.2136/sssaj2018.11.0458
Drinkwater, L.E., and Snapp, S. S. (2007). Nutrients in agroecosystems: rethinking the management paradigm. Adv. Agron. 92, 163–186. doi: 10.1016/S0065-2113(04)92003-2
Drinkwater, L.E., Wagoner, P., and Sarrantonio, M. (1998). Legume-based cropping systems have reduced carbon and nitrogen losses. Nature 396, 262–265. doi: 10.1038/24376
Drinkwater, L. E., Cambardella, C. A., Reeder, J. D., and Rice, C. W. (1997). “Potentially mineralizable nitrogen as an indicator of biologically active soil nitrogen,” in Methods for Assessing Soil Quality, editors A. J. J. John and W. Doran. 49th Edn. (Madison, WI: Soil Science Society of America).
DuPont, S. T., Culman, S. W., Ferris, H., Buckley, D. H., and Glover, J. D. (2010). No-tillage conversion of harvested perennial grassland to annual cropland reduces root biomass, decreases active carbon stocks, and impacts soil biota. Agric. Ecosyst. Environ. 137, 25–32. doi: 10.1016/j.agee.2009.12.021
Fierer, N., and Schimel, J. P. (2002). Effects of drying-rewetting frequency on soil carbon and nitrogen transformations. Soil Biol. Biochem. 34, 777–787. doi: 10.1016/S0038-0717(02)00007-X
Finney, D. M., White, C. M., and Kaye, J. P. (2016). Biomass production and carbon/nitrogen ratio influence ecosystem services from cover crop mixtures. Agron. J. 108, 39–52. doi: 10.2134/agronj15.0182
Ghimire, R., Ghimire, B., Mesbah, A. O., Sainju, U. M., and Idowu, O.J. (2019). Soil health response of cover crops in winter wheat-fallow system. Agron. J. 111, 2108–2115. doi: 10.2134/agronj2018.08.0492
Ginakes, P., Grossman, J. M., Baker, J. M., and Sooksa-nguan, T. (2020). Living mulch management spatially localizes nutrient cycling in organic corn production. Agriculture 10, 1–10. doi: 10.3390/agriculture10060243
Griffin, T., Liebman, M., and Jemison, J. (2000). Cover crops for sweet corn production in a short-season environment. Agron. J. 92, 144–151. doi: 10.2134/agronj2000.921144x
Hermans, S. M., Taylor, M., Grelet, G., Curran-Cournane, F., Buckley, H. L., Handley, K. M., and Lear, G. (2021). From pine to pasture: Land use history has long-term impacts on soil bacterial community composition and functional potential. FEMS Microbiol. Ecol. 96, 1–12. doi: 10.1093/femsec/fiaa041
Hively, W. D., and Cox, W. J. (2001). Interseeding cover crops into soybean and subsequent corn yields. Agron. J. 93, 308–313. doi: 10.2134/agronj2001.932308x
Jorgensen, E., and Pedersen, A. R. (1998). How to obtain those nasty standard errors from transformed data - and why they should not be used. Department of Biometry and Informatics, Tjele, Denmark. Available online at: http://citeseerx.ist.psu.edu/viewdoc/download?doi=10.1.1.47.9023&rep=rep1&type=pdf
Kantola, I. B., Masters, M. D., and DeLucia, E. H. (2017). Soil particulate organic matter increases under perennial bioenergy crop agriculture. Soil Biol. Biochem. 113, 184–191. doi: 10.1016/j.soilbio.2017.05.023
Kuo, S., and Sainju, U. M. (1998). Nitrogen mineralization and availability of mixed leguminous and non-leguminous cover crop residues in soil. Biol. Fertil. Soils 26, 346–353. doi: 10.1007/s003740050387
Larsen, J. K., Brun, L. J., Enz, J. W., and Cox, D. J. (1987). Minimizing the risk of producing winter wheat in North Dakota. I. The effect of tillage on snow depth, soil temperature, and winter wheat survival. Farm Res. 44, 9–13.
Lawson, A., Fortuna, A. M., Cogger, C., Bary, A., and Stubbs, T. (2013). Nitrogen contribution of rye-hairy vetch cover crop mixtures to organically grown sweet corn. Renew. Agri. Food Syst. 28, 59–69. doi: 10.1017/S1742170512000014
Lawson, A., Cogger, C., Bary, A., and Fortuna, A. M. (2015). Influence of seeding ratio, planting date, and termination date on rye-hairy vetch cover crop mixture performance under organic management. PLoS ONE 10:e0129597. doi: 10.1371/journal.pone.0129597
Leavitt, M. J., Sheaffer, C. C., Wyse, D. L., and Allan, D. L. (2011). Rolled winter rye and hairy vetch cover crops lower weed density but reduce vegetable yields in no-tillage organic production. HortScience 46, 387–395. doi: 10.21273/HORTSCI.46.3.387
Lynch, D. H. (2015). Nutrient cycling and soil health in organic cropping systems - importance of management strategies and soil resilience. Sustain. Agric. Res. 4, 80. doi: 10.5539/sar.v4n3p80
Marriott, E. E., and Wander, M. M. (2006). Total and labile soil organic matter in organic and conventional farming systems. Soil Sci. Soc. Am. J. 70, 950. doi: 10.2136/sssaj2005.0241
Meier, U. (2001). BBCH Monograph. Growth Stages of Mono-and Dicotyledonous Plants. 2nd Edn. Federal Biological Research Centre for Agriculture and Forestry, Braunschweig, Germany.
Minnesota Leads Nation in Sweet Corn Green Pea Production. FarmProgress. (2018). Available online at: https://www.farmprogress.com/crops/minnesota-leads-nation-sweet-corn-green-pea-production
Mirsky, S. B., Curran, W. S., Mortensen, D. M., Ryan, M. R., and Shumway, D. L. (2011). Timing of cover-crop management effects on weed suppression in no-till planted soybean using a roller-crimper. Weed Sci. 59, 380–389. doi: 10.1614/WS-D-10-00101.1
Mirsky, S. B., Ackroyd, V. J., Cordeau, S., Curran, W. S., Hashemi, M., Reberg-Horton, S. C., et al. (2017). Hairy vetch biomass across the eastern united states: effects of latitude, seeding rate and date, and termination timing. Agron. J. 109, 1510–1519. doi: 10.2134/agronj2016.09.0556
Moore, V. M., Mitchell, P. D., Silva, E. M., and Barham, B. L. (2016). Cover crop adoption and intensity on Wisconsin's organic vegetable farms. Agroecol. Sustain. Food Syst. 40, 693–713. doi: 10.1080/21683565.2016.1181694
Noland, R. L., Wells, M. S., Sheaffer, C. C., Baker, J. M., Martinson, K. L., and Coulter, J. A. (2018). Establishment and function of cover crops interseeded into corn. Crop Sci. 58, 863–873. doi: 10.2135/cropsci2017.06.0375
O'Connell, S., Shi, W., Grossman, J. M., Hoyt, G. D., Fager, K. L., and Creamer, N. G. (2015). Short-term nitrogen mineralization from warm-season cover crops in organic farming systems. Plant Soil 396, 353–367. doi: 10.1007/s11104-015-2594-2
Ontivero, R. E., Voyron, S., Risio Allione, L. V., Bianco, P., Bianciotto, V., Iriarte, H. J., et al. (2020). Impact of land use history on the arbuscular mycorrhizal fungal diversity in arid soils of Argentinean farming fields. FEMS Microbiol. Lett. 367, 1–11. doi: 10.1093/femsle/fnaa114
Perrone, S., Grossman, J., Liebman, A., Sooksa-nguan, T., and Gutknecht, J. (2020). Nitrogen fixation and productivity of winter annual legume cover crops in Upper Midwest organic cropping systems. Nutrient Cycling Agroecosyst. 117, 61–76. doi: 10.1007/s10705-020-10055-z
Peterson, D. (2020). Nitrogen management key for organic sweet corn. The Organic Grower. Available online at: https://organicgrower.info/article/nitrogen-management-key-for-organic-sweet-corn/
Puget, P., and Drinkwater, L. E. (2001). Short-term dynamics of root- and shoot-derived carbon from a leguminous green manure. Soil Sci. Soc. Am. J. 65, 771–779. doi: 10.2136/sssaj2001.653771x
Robačer, M., Canali, S., Kristensen, H. L., Bavec, F., Mlakar, S. G., Jakop, M., and Bavec, M. (2016). Cover crops in organic field vegetable production. Sci. Hortic. 208, 104–110. doi: 10.1016/j.scienta.2015.12.029
SARE. (2007). Managing Cover Crops Profitably. (Andy Clark, Ed.) (3rd Edn.). College Park, MD: SARE.
Seman-Varner, R., Varco, J., and O'Rourke, M. (2017). Nitrogen benefits of winter cover crop and fall-applied poultry litter to corn. Agron. J. 109, 2881–2888. doi: 10.2134/agronj2016.11.0670
Sievers, T., and Cook, R. L. (2018). Aboveground and root decomposition of cereal rye and hairy vetch cover crops. Soil Sci. Soc. Am. J. 82, 147–155. doi: 10.2136/sssaj2017.05.0139
Silva, E. M. (2014). Screening five fall-sown cover crops for use in organic no-till crop production in the upper midwest. Agroecol. Sustain. Food Syst. 38, 748–763. doi: 10.1080/21683565.2014.901275
Singer, J. (2008). Corn Belt assessment of cover crop management and preferences. Agron. J. 100, 1670–1672. doi: 10.2134/agronj2008.0151
St. Luce, M., Ziadi, N., Zebarth, B. J., Whalen, J. K., Grant, C. A., Gregorich, E. G., et al. (2013). Particulate organic matter and soil mineral nitrogen concentrations are good predictors of the soil nitrogen supply to canola following legume and non-legume crops in western Canada. Can. J. Soil Sci. 93, 607–620. doi: 10.4141/cjss2013-005
Tardy, V., Chabbi, A., Charrier, X., de Berranger, C., Reignier, T., Dequiedt, S., et al. (2015). Land use history shifts in situ fungal and bacterial successions following wheat straw input into the soil. PLoS ONE 10, 1–17. doi: 10.1371/journal.pone.0130672
Teasdale, J. R., Devine, T. E., Mosjidis, J. A., Bellinder, R. R., and Beste, C. E. (2004). Growth and development of hairy vetch cultivars in the northeastern United States as influenced by planting and harvesting date. Agron. J. 96, 1266–1271. doi: 10.2134/agronj2004.1266
Thapa, R., Poffenbarger, H., Tully, K. L., Ackroyd, V. J., and Kramer, M. Mirsky, S. B. (2018). Biomass production and nitrogen accumulation by hairy vetch-cereal rye mixtures: A meta-analysis. Agron. J. 110, 1197–1208. doi: 10.2134/agronj2017.09.0544
Vance, E. D., Brookes, P. C., and Jenkinson, D. S. (1987). An extraction method for measuring soil microbial biomass C. Soil Biol. Biochem. 19, 703–707. doi: 10.1016/0038-0717(87)90052-6
Wander, M. (2004). “Soil organic matter fractions and their relevance to soil function,” in Soil Organic Matter in Sustainable Agriculture, eds F. Magdoff and R. R. Weil (Boca Raton, FL: CRC Press).
Wauters, V. M., Grossman, J. M., Pfeiffer, A., and Cala, R. (2021). Ecosystem services and cash crop tradeoffs of summer cover crops in northern region organic vegetable rotations. Front. Sustain. Food Syst. 5, 1–11. doi: 10.3389/fsufs.2021.635955
Weil, R. R., Islam, K. R., Stine, M. A., Gruver, J. B., and Samson-Liebig, S. E. (2003). Estimating active carbon for soil quality assessment: a simplified method for laboratory and field use. Am. J. Alter. Agric. 18, 3–17. doi: 10.1079/AJAA2003003
Wieder, W. R., Grandy, A. S., Kallenbach, C. M., and Bonan, G. B. (2014). Integrating microbial physiology and physio-chemical principles in soils with the MIcrobial-MIneral Carbon Stabilization (MIMICS) model. Biogeosciences, 11, 3899–3917. doi: 10.5194/bg-11-3899-2014
Wiegert, R. G. (1962). The selection of an optimum quadrat size for sampling the standing crop of grasses and forbs. Ecology 43, 125–129. doi: 10.2307/1932047
Wilson, D. (2007). Earlier-Flowering Hairy Vetch a Great Advance, but Northern Farmers Need More. Kutztown, PA: New Farm Research.
Keywords: cover crops, legumes, nutrient cycling, organic cropping systems, soil organic matter
Citation: Perrone S, Grossman J, Liebman A, Wells S, Sooksa-nguan T and Jordan N (2022) Legume Cover Crop Contributions to Ecological Nutrient Management in Upper Midwest Vegetable Systems. Front. Sustain. Food Syst. 6:712152. doi: 10.3389/fsufs.2022.712152
Received: 20 May 2021; Accepted: 08 March 2022;
Published: 15 April 2022.
Edited by:
Marney E. Isaac, University of Toronto, CanadaReviewed by:
Stephen J. Ventura, University of Wisconsin-Madison, United StatesAdnane Bargaz, Mohammed VI Polytechnic University, Morocco
Copyright © 2022 Perrone, Grossman, Liebman, Wells, Sooksa-nguan and Jordan. This is an open-access article distributed under the terms of the Creative Commons Attribution License (CC BY). The use, distribution or reproduction in other forums is permitted, provided the original author(s) and the copyright owner(s) are credited and that the original publication in this journal is cited, in accordance with accepted academic practice. No use, distribution or reproduction is permitted which does not comply with these terms.
*Correspondence: Julie Grossman, jgross@umn.edu
†ORCID: Sharon Perrone orcid.org/0000-0001-6875-3162