- 1Newe Ya'ar Research Center, Institute of Soil, Water and Environmental Sciences, Agricultural Research Organization (ARO) – Volcani Institute, Ramat Yishai, Israel
- 2Faculty of Agriculture, Food and Environment, The Hebrew University of Jerusalem, Jerusalem, Israel
Broiler litter (BL) is often contaminated by a variety of zoonotic pathogens. This study attempts to assess the persistence of Salmonella enterica serovar Infantis (S. Infantis) in BL based on spatial and temporal variation of physicochemical properties in a stockpile and composting sleeve. A single trial of two pilot-scale setups, ~35 m3 each, included an open static pile (stockpile) and composting in a polyethylene sleeve with forced aeration. The initial water content was adjusted only for the sleeve (~50% w/w) as in a common composting practice. Both systems were monitored weekly and then biweekly during 2 months in 47–53 sampling points each on every campaign. Measurements included temperature, water content, pH, electrical conductivity (EC), gas-phase oxygen, and ammonia, and the collected data were used to construct multiple contour grid maps. Of the stockpile volume, 83, 71, and 62% did not reach the commonly required minimum temperature of 55°C for three consecutive days during the first, second, and third weeks, respectively. Oxygen levels showed a strong gradient across the stockpile, while anaerobic conditions prevailed in the core. Variation was also recorded within the sleeve, but due to the water content adjustment and active aeration, the conditions favored more intense degradation and higher temperatures. Combining the grid maps drawn in this study with decay rate constants recently published for S. Infantis in BL under 36 combinations of temperature, water content, and pH, we assessed the spatial persistence of S. Infantis in the stockpile and the sleeve. Temperature was shown as a major factor, while water content and pH had only a small effect, in the stockpile only. Co-correlations between temperature, water content, EC, and oxygen suggest that selected physicochemical properties may be sufficient for such assessments. Up to 3 weeks would be recommended to achieve 7–8 log10 reduction in Salmonella in a stockpile, while this would be fully achieved within 1 week in a sleeve. This approach of combining high-resolution spatial field sampling along with decay rates of pathogens under controlled lab conditions may improve quantitative microbial risk assessments and future regulations of manure utilization.
Introduction
The poultry sector is one of the largest and among the fastest growing agriculture-based meat production industries worldwide, due to the growing demand for both meat and egg products (Bolan et al., 2010). Broiler litter (BL) is a byproduct of broiler meat production, consisting of a mixture of fecal droppings, bedding materials (usually sawdust or shavings, rice hulls, or straw), feathers, and wasted feed (Wilkinson, 2007; Kim et al., 2012). Often, pathogens excreted from infected chickens contaminate the litter, which, in turn, becomes a source of zoonotic pathogens such as Salmonella, limiting the safe use of BL (Chinivasagam et al., 2010; Wilkinson et al., 2011; Gould et al., 2013). At the same time, due to the relatively high concentrations of nitrogen and other plant nutrients along with low water content, BL is considered a valuable fertilizer and soil additive in conventional and organic farming (Wilkinson, 1979; Stephenson et al., 1990; Kingery et al., 1994; Chaudhry et al., 1998; Marshall et al., 1998; Mitchell and Tu, 2006; Cassity-Duffey et al., 2015). The extensive global application of BL as a plant nutrient is expected to continue, considering the increasing demand for broiler production; yet, although BL may be contaminated by a variety of zoonotic pathogens, most farmers use it either without composting or after partial stabilization, by temporarily stockpiling the material in the field, covered or uncovered under uncontrolled conditions (Bush et al., 2007; Wilkinson, 2007; Ogejo and Collins, 2009; Wilkinson et al., 2011). In Israel, about 250 million broilers were grown in 2020 (Poultry Council Chicken Health Labs, 2020), generating nearly 290 thousand tons of BL per annum (Cnaan, N., Director of the Growth Division of “Off Tov Group”, Israel; personal communication) of which the majority is used directly in agriculture (Grinhut et al., 2015).
Salmonella outbreaks due to consumption of contaminated fresh produce are still a threat to public health (Beuchat, 2002; Islam et al., 2004a,b; Fatica and Schneider, 2011; Bell et al., 2015; Herman et al., 2015; Chaves et al., 2016; Gu et al., 2018; Jechalke et al., 2019). To minimize such outbreaks, pathogen inactivation prior to land application is commonly sought, which is the main drive for thermophilic composting of manures (Williams and Benson, 1978; Vinnerås et al., 2003; Wichuk and McCartney, 2007; Macklin et al., 2008; Wilkinson et al., 2011). Thermal inactivation may be partially achieved also by stockpiling the litter for some time before spreading, but it cannot be effective compared with controlled thermophilic composting. To ensure effective pathogen elimination during composting, international guidelines require a minimum of 55°C for three consecutive days under in-vessel or aerated static pile methods. In open windrows, this minimal temperature should be maintained for at least 15 consecutive days with a minimum of five turnings during the thermophilic phase (USEPA, 2003; Wichuk and McCartney, 2007). These guidelines correspond with the work of Isobaev et al. (2014) who presented a statistical modeling based on temperature measurements in a covered aerated static pile of biosolids and wood chips. They estimated that following five turnings, the likelihood of every particle to be exposed to the required time and temperature conditions (≥55°C for three consecutive days) was 98%. Multiple studies, however, reported a variety of composting settings in which these temperature requirements have not been fully met in the entire volume of the composting material (Pereira-Neto et al., 1986; Fernandes et al., 1994; Erickson et al., 2010; Wilkinson et al., 2011; Avidov et al., 2017). Although thermophilic temperatures are reported in multiple composting studies, only average or min and max values are often reported, while the spatial distributions remain unknown (e.g., Déportes et al., 1998; Tiquia et al., 1998; Raviv et al., 1999; Larney et al., 2003; Van Herk et al., 2004; Aviani et al., 2010). Clearly, average temperatures or a single measurement point cannot indicate the achievement of pathogen lethal conditions. It should be noted that pathogens surviving the composting process or any phase of stabilization may regrow during storage under favorable conditions or following land application (Yeager and Ward, 1981; Zaleski et al., 2005; Chen and Jiang, 2014; Reynnells et al., 2014; Avidov et al., 2017, 2021a; Hruby et al., 2018). In our previous study (Avidov et al., 2021a), soil-BL mixtures were inoculated with S. Infantis and incubated at 30°C for a period of 90 days. Salmonella decreased by 4–6 log10 but then increased again within 2 weeks by 2–3 log10, in response to the increase in water content from 30 to 70% water holding capacity.
Variable oxygen concentrations during composting was also demonstrated in several studies (Haga et al., 1998; Erickson et al., 2010; Poulsen, 2011; Stegenta et al., 2019). Anaerobic zones within the pile were reported during the first week of composting at the bottom of the core in a static pile of dairy cattle feces (Haga et al., 1998). Similarly, Stegenta et al. (2019) recorded the lowest oxygen concentrations at the core in windrow piles of sewage sludge, and Poulsen (2011) reported the lowest oxygen concentrations at the bottom of the core in a turned windrow pile, which contained sewage sludge, yard and park waste, and screening residue from processing of finished compost. In six unturned static piles of chicken litter and peanut hulls, Erickson et al. (2010) showed oxygen concentrations above 18% at the pile surface and below 10% at about 30-cm depth, during the first week of composting. Aerobic–anaerobic gradients may have an effect on pathogen persistence. Avidov et al. (2021a) observed higher decay rates of S. Infantis in BL at mesophilic temperatures under anaerobic compared with aerobic conditions and postulated that it might be related to the different composition and density of antagonistic microbial populations. Such variability of multiple physicochemical properties has not been used in quantitative assessments of pathogen persistence in stockpiles or composting systems. The combination of temperature with other environmental factors may have varying effects on Salmonella persistence in BL. Several studies have examined the effect of temperature and water content, generally showing that thermal susceptibility of Salmonella spp. increases with increasing water content (Liu et al., 1969; Wilkinson et al., 2011; Singh et al., 2012; Chen et al., 2013). At low water content, desiccation may play a major role in pathogen inactivation. Yet, desiccation-adapted Salmonella spp. persisted longer in aged chicken litter compared with non-adapted cells (Chen et al., 2013). Other mixed physiochemical properties may also play a role in bacterial inactivation, such as the combined and intensified effect of drying and NH3 emissions, as shown by Himathongkham and Riemann (1999). Biological mechanisms, such as competition between indigenous microorganisms and pathogens (Wichuk and McCartney, 2007), and microbial antagonism (Millner et al., 1987; Erickson et al., 2010; Gurtler et al., 2018) may also affect pathogen inactivation. Recently, Avidov et al. (2021a) explored the combined effect of temperature (30, 40, 50, and 60°C), water content (40, 55, and 70%; w/w), and initial pH (6, 7, and 8.5) on the persistence of S. Infantis in BL under laboratory controlled conditions. The authors showed that temperature was the main factor influencing Salmonella decay rates, while water content and initial pH were of secondary level of influence with significant effects mainly at 30 and 40°C.
This study presents a combined field-lab approach for assessing pathogen persistence in BL before land application. It is based on high-resolution spatial field sampling of BL during temporary storage or composting, coupled with lab-scale evaluations of the pathogen persistence under controlled conditions. The first objective was to evaluate in detail the spatial–temporal distribution of key physicochemical properties that can affect the persistence of pathogens in BL. Two systems were examined: 1. A static stockpile (without any special treatment), which represents the common practice in Israel and 2. composting in a closed polyethylene sleeve with forced aeration as a cheap alternative of enclosed composting (Avidov et al., 2017, 2018). The second objective was to combine such field data with recently published decay rate constants of S. Infantis under controlled lab conditions (Avidov et al., 2021a) and use the data in assessing the persistence of the pathogen in real scenarios. Besides being a unique systematic database of decay constants, both the present and the previous study used BL from tunnel-ventilated broiler houses of farms in northern Israel that use raising protocols of the main national poultry cooperatives.
Materials and Methods
Experimental Setup
The study was conducted at the Newe Ya'ar Research Center, Israel, between August and September 2018 during which the ambient min and max temperatures ranged between 18.9–22.8°C and 33.4–36.7°C, respectively (Israel Meteorological Service; data station No. 186; https://ims.data.gov.il). A single trial of two pilot-scale setups, ca. 35-m3 BL each, included one open static pile (stockpile) and one closed polyethylene sleeve with forced aeration (Avidov et al., 2017, 2018). The BL was brought from two broiler houses located in a poultry farm at Moshav Balfouria (northern Israel) at the end of an extensive indoor rearing period of 6 weeks. The stockpile was prepared directly by unloading a truck on a concrete floor located outdoor at the Newe Ya'ar campus. This pile consisted of three subpiles; each was monitored at 15 points (three sampling locations at depths of 10, 30, 50, 70, and 85 cm) plus 8 points at the lower edges of the pile for temperature measurements, or 2 points for other measurements. A total of 47 or 53 sampling points (Figures 1A,B) were employed on the entire stockpile. For composting in a sleeve, the BL was initially brought to a water content of ca. 50% (w/w), considered to be within the optimal range for composting (Bernal et al., 2009; Christian et al., 2009). For that, water was added to the pile manually using a hose and a water flow meter. Then the BL was mixed several times by means of a front-end and backhoe loader. The amount of added water was predetermined based on the initial water content of the BL (drying at 70°C for 24 h). The sleeve was constructed manually, using a polyethylene sheet of 25-m long × 8.5-m wide and 1,500 μm thick (A. A. Politiv Ltd., Kibbutz Einat, Israel). The sheet was placed on the ground, a solid 2.5-inch diameter PVC flexible pipe was connected to a blower on one side (centrifugal Model PB 50-3, Shevah Blowers Ltd., Ashdod, Israel), while the other side was connected to a 3.5-inch diameter perforated PVC flexible pipe (hole area ca. 10 mm2; the pattern included six holes in circumferential rings along the pipe at 1.7-cm intervals). The perforated pipe was placed on a plastic sheet in the form of a longitudinal ring. Another piece of solid pipe was placed for exhaust on the opposite side of the sleeve (Figure 1C). The BL was piled on the sheet (on top of the perforated pipe), and the sleeve was sealed using a manual impulse hand sealer (hpl ISZ, HAWO, Germany). Finally, the sheet and the pipe were taped together on both sides using a heavy-duty masking tape (Avidov et al., 2019). This manual procedure was developed in the present study as an alternative to the use of a dedicated machine [like Euro-bagging CM 1.5 CCS (Avidov et al., 2017, 2018)]. The principles of the technology remain similar, although the specific pipe geometry can affect airflow paths. The blower was operated by a programmable logic controller (PLC; Vision1040™, Unitronics, Israel) for 2 min ON and 30 min OFF with flowrates of ca. 150 m3 h−1. The sleeve was monitored in four cross-sections, at 1.5-, 6-, 10- to 12-, and 16-m distance from the blower (sections I–IV, respectively). Each cross-section was monitored in 12 sampling points, a total of 48 points (Figure 1D). To represent the results of the whole sleeve, we calculated the weighted average of the four cross-sections, considering the relative volume of each section. All sampling campaigns, both in the stockpile and the sleeve, were conducted during daytime, usually between 8 a.m. and 4 p.m.
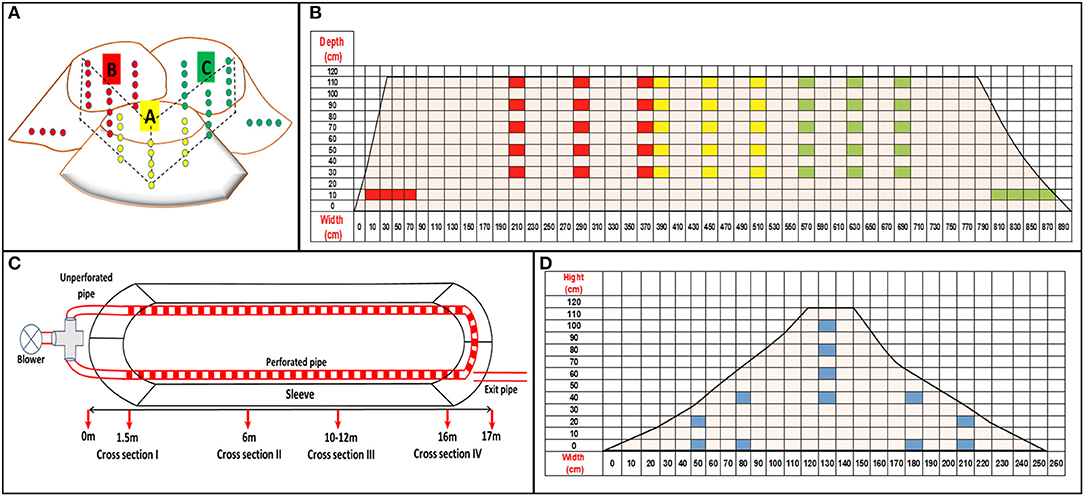
Figure 1. Setup geometry and the location of sampling points in the stockpile (A,B) and the sleeve (C,D). The cross-section of the stockpile connects the centers of three subpiles. The sleeve was divided into four sections, each was represented by a cross-section at 1.5-, 6-, 10- to 12-, and 16-m distance from the blower (denoted as CS-I – CS-IV).
Spatial–Temporal Mapping of Physicochemical Properties of the Stockpile and the Sleeve
Temperature, water content, pH, electrical conductivity (EC), as well as gas-phase oxygen and ammonia, were measured weekly during the first month and biweekly during the second month of the experimental period. Temperatures were measured by a mobile type K thermocouple that was mounted on an 80-cm-long stainless steel rod and connected to a 305 thermometer (Elcon Ltd., Israel). BL samples were collected from the designated depths using a soil auger (Edelman head, 3.5-cm core diameter; Eijkelkamp Soil and Water; Giesbeek, The Netherlands). Water content (w/w) was determined on ca. 5- to 6-g samples by oven drying at 70°C for 48 h. The pH and EC were measured in aqueous extracts at 1:9 dry weight:deionized water. The extracts were prepared by using a reciprocal shaker at 200 rpm for 1 h. The pH was analyzed directly in the suspension (LL-Ecotrode Plus WOC; Metrohm, Herisau, Switzerland), while the EC was determined in the supernatant after centrifugation at 6,000 rpm for 20 min at 25°C (CyberScan CON 11, Eutech Instruments, Thermo Fisher Scientific Inc., Waltham, MA, USA). Gas-phase oxygen and ammonia were monitored in the same designated points (as used for temperature measurements and BL sampling) by pumping the headspace of each point using two pocket pumps (SKC 210 and SKC 220-1000TC, 84, PA, USA). The air was pumped through a Teflon tube (1/4″ inner diameter) that was connected in parallel to an acid trap (for ammonia analyses) and an oxygen sensor (SO-210, Apogee Instruments, Inc., Logan, UT, USA) (Supplementary Figure 1). The oxygen sensor was calibrated, and its readings were validated at the beginning of each sampling day against zero (pure N2; 99.999%) and ambient air (21%). Ammonia (trapped in 0.1 M sulfuric acid) was analyzed using a spectrophotometric method with slight modifications after that of Willis et al. (1996) and similar to that of Avidov et al. (2021b). The method was validated against a standard of 50-ppm ammonia (Balance N2 58L standard gas; Calgaz, Staffordshire UK), showing a bias of <4%. On each sampling campaign, the headspace was withdrawn at a single time in each point of the stockpile, while for the sleeve, it was done at intervals during aeration-off periods. In that case, sampling was done for a period of about 30 min following aeration-on periods of 2 or 7 min. Each spatial dataset was used to construct a grid map, which was then converted into a kriged contour map using the Surfer 7 software (Golden software, CO, USA). The initial average height of the stockpile was 113 (±5.8) cm and that of the sleeve was 120 (±0) cm. For simplicity, both the stockpile and the sleeve were normalized to the same initial height of 120 cm, as well as throughout the experiment, during which we observed height loss (and volume) due to natural compaction and organic matter degradation. Due to normalization of the sampling depths, the relative position of the sampling points in all maps overlapped each other.
BL Analyses
Selected properties of the fresh and processed BL are presented in Table 1. The fresh BL was sampled from nine random locations right after constructing the stockpile and the sleeve (time 0). A composite sample of each setup was then divided into triplicate subsamples. After 2 months, samples were collected from the stockpile and the sleeve from all designated sampling points and combined into composite samples (one for the stockpile and four for each cross-section of the sleeve). Ash content was determined after incinerating samples at 550°C for 4 h. Total C and N were determined after grinding the samples (mixer mill MM 400, Retsch, Haan, Germany) and analyzing them by FlashSmart 2000 Elemental Analyzer (Thermo Fisher Scientific Inc., Waltham, MA, USA). Total P was determined after digestion with H2SO4 and H2O2 using the ascorbic acid method (SM 4500 P-E; APHA, 2005). Analyses of water content, pH, and EC followed the same procedures as described above for the spatial–temporal mapping.
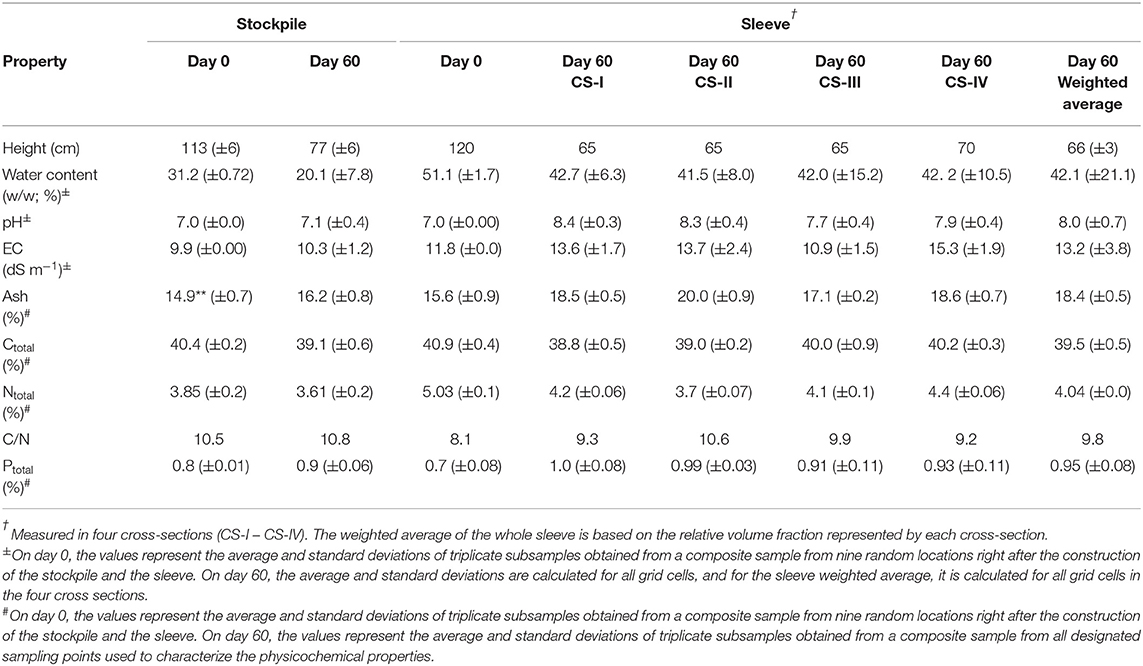
Table 1. Selected properties of the broiler litter (BL) at time 0 and after 60 days in a static stockpile and a closed sleeve with forced aeration.
Assessing the Persistence of S. Infantis in Broiler Litter at the Stockpile and Composting Sleeve
Using exponential decay constants recently published for S. Infantis (Avidov et al., 2021a), along with the contour maps drawn in the present study, we assessed the persistence of the pathogen in the stockpile and the sleeve. The work of Avidov et al. (2021a) was based on controlled static lab vessels, covering 36 combinations of four temperatures (30, 40, 50, and 60°C), three water contents (40, 55, and 70%), and three initial pH (6, 7, and 8.5). Thus, according to the contour maps drawn for temperature, water content, and pH, the stockpile and the sleeve were divided into volume fractions of specific combinations. To link between the discrete values used by Avidov et al. (2021a) and the ranges applied in this study, we assumed the following matching: Temperatures of 30, 40, 50, and 60°C were considered as ≤35°C, 35–45°C, 45–55°C, and >55°C; water contents of 40, 55, and 70% were considered as <47.5%, 47.5–62.5%, and >62.5%; and pH of 6, 7, and 8.5 were considered as ≤6.5, 6.5–7.75, and >7.75. Based on the specific combination, each grid cell was assigned with a respective k value from Avidov et al. (2021a), representing the first-order decay constant according to Equation (1).
where C(t) is the concentration of Salmonella (CFU g−1 dry matter) at point in time t (days), C0 is the initial concentration of Salmonella, and k is the first-order decay constant (days−1). After assigning k values, the log10 reduction of Salmonella (C(t)/C0) during the first week (t =7 days) was calculated for each cell. Then the log10 reduction after 2 weeks (t = 14 days) in each cell was calculated as the sum of log10 reductions in week 1 and week 2. Likewise, the log10 reduction can be calculated for longer times based on the temporal physicochemical grid maps constructed for each week.
Statistical Analyses
JMP Pro 15 (SAS Institute Inc.) was used for all statistical analyses. The comparisons between kriged contour maps (performed using the Surfer 7 software; see above) were made on selected number of grid cells (n value) that is comparable to the number of physical sampling points measured in the sleeve and the stockpile. This limited number of cells were evenly distributed throughout the cross-section (50 points for the single cross-section of the stockpile and 12 points for each of the four cross-sections of the sleeve) to represent the overall spatial distribution of physicochemical properties. Significant differences were determined using Tukey's honestly significant difference (HSD) test at p ≤ 0.05. Pearson's r correlations were determined among the physicochemical variables determined for all grid cells in the stockpile and the sleeve.
Results and Discussion
Spatial and Temporal Variations of Key Physicochemical Properties in the Stockpile and the Sleeve
Temperature
Stockpile
A clear trend was evident since the first week, showing higher temperatures in the center of the stockpile and relatively cool temperatures at the margins and top surface (Figure 2A). The temperature gradient ranged from 61°C at the core, down to 33–34°C toward the surface. Within this range, 83% of the total cross-section (considered here as the total pile volume) was below (≤) 55°C. Moreover, 27% of the entire pile volume, within the margins and top surface, did not reach thermophilic conditions (≤45°C). This trend did not change significantly during a period of 2 months (Supplementary Figure 2-1 and Supplementary Table 1B), although this gradient slightly weakened over time, and the hot regions were reduced. A plot of cumulative volume fractions (Figure 2A1) shows a slight cool down on weeks 6 and 8, such that 50% of the pile volume was below 49–50°C, 49–50°C, 50–51°C, 49–50°C, 49–50°C, and 46–47°C, on weeks 1, 2, 3, 4, 6, and 8, respectively. This trend is also expressed by the average temperature of all grid cells, which was 48.8, 49.0, 49.5, 48.8, 48.3, and 46.1°C, on weeks 1, 2, 3, 4, 6, and 8, respectively.
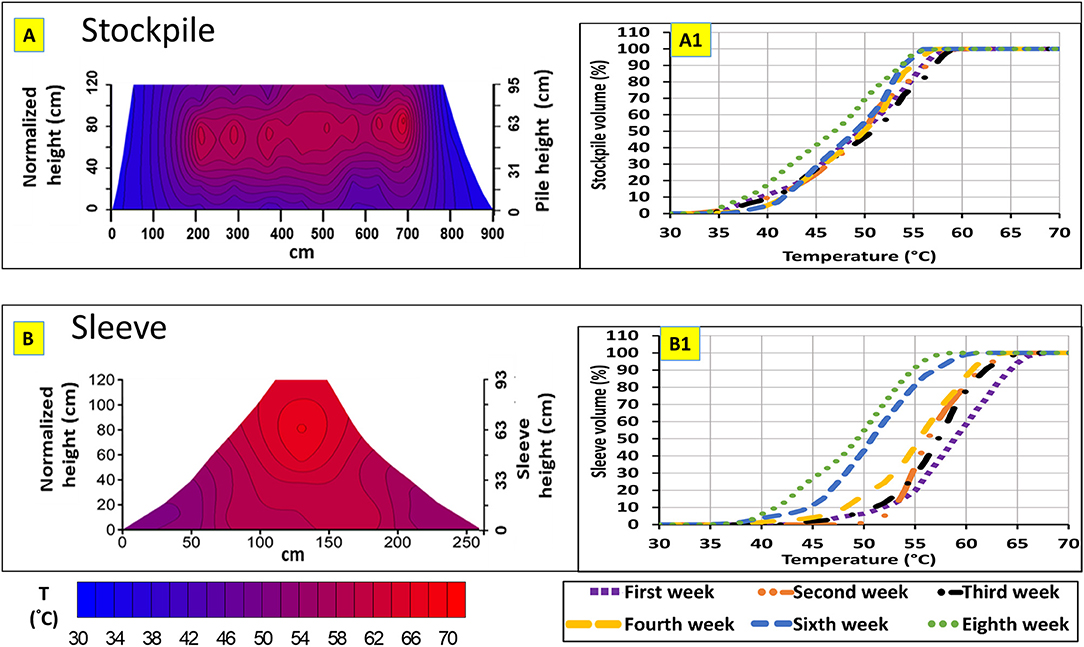
Figure 2. Spatial–temporal variation in temperature (°C). Contour maps (week 1) and the cumulative volumetric percentage of temperature in the stockpile (A,A1) and the sleeve (B; cross-section II, B1; weighted average of cross-sections I–IV).
Sleeve
Temperatures on weeks 1–4 and 6 were significantly higher than in the stockpile (Supplementary Table 1A), while the core and the top of the sleeve were still warmer than the lower sides (Figure 2B; CS-II). During the first week, the weighted average gradient of the four cross-sections ranged from 68 to 69°C in the core, down to 45–46°C at the lower sides. Within this range, 20% of the volume was below 55°C. The proximity to the blower seemed to have a chilling effect only in the first cross-section (CS-I; Supplementary Figure 3-1, significantly lower than other cross-sections only on weeks 2 and 4; Supplementary Table 1C); during the first week, 32, 10, 25, and 11% of the volume exhibited temperatures below 55°C in CS-I, CS-II, CS-III, and CS-IV, respectively. This trend did not change substantially during the entire period of 2 months (Supplementary Figure 3-1), although the gradient weakened over time and the upper part cooled down gradually, such that it finally became less spatially heterogeneous. A plot of the cumulative volume fractions (Figure 2B1) shows a more substantial cool down trend compared with the stockpile, such that 50% of the volume was below 58–59°C, 56–57°C, 57–58°C, 55–56°C, 50–51°C, and 49–50°C, on weeks 1, 2, 3, 4, 6, and 8, respectively. This cool down is also expressed by the weighted average temperature of all grid cells, which was 58.5, 56.7, 56.7, 54.8, 50.6, and 48.6°C, on weeks 1, 2, 3, 4, 6, and 8, respectively. No clear effect of the proximity to the blower was shown on these average temperatures.
Sleeve geometry and the setting of the perforated aeration pipe as well as the selection of blower type (Figure 1C) could all have an effect on temperature distribution and the relatively cool low edges. In our previous studies on biosolids and green waste (Avidov et al., 2017, 2018), the perforated pipe was placed in the center of the sleeve and showed lower temperatures along the centerline in the proximity to the aeration pipe. These studies also showed extensive heat accumulation within the middle and top parts of the sleeve. The sleeve clearly provided better conditions for thermal inactivation of pathogens: Compared with 83, 71, and 62%, of the stockpile volume, only 20, 14, and 8% of the sleeve volume did not reach the commonly required minimum of 55°C for three consecutive days (USEPA, 2003) by the end of the first, second, and third weeks, respectively.
Water Content
Stockpile
The initial water content of the BL was relatively low (31.2 ± 0.72%; Table 1), and it was not adjusted prior to stockpiling as in a common practice. The gradient ranged from 14% at the stockpile edges and up to 39% at some locations in the center (Figure 3A). Within this range, none of the locations were at optimal conditions for biological activity (50–60%; Bernal et al., 2009, 45–65%; Christian et al., 2009). The water content gradient slightly decreased during the 2 months (Supplementary Figure 2-2, significantly lower on weeks 6 and 8; Supplementary Table 1B); a plot of the cumulative volume fractions (Figure 3A1) shows a gradual drying over time, such that 50% of the material was below 28–29%, 24–25%, 25–26%, 24–25%, 22–23%, and 19–20%, on weeks 1, 2, 3, 4, 6, and 8, respectively. This gradual drying is also expressed by the average water content of all grid cells, which was 27.7%, 23.6%, 24.1%, 23.5%, 21.7%, and 20.1% on weeks 1, 2, 3, 4, 6, and 8, respectively.
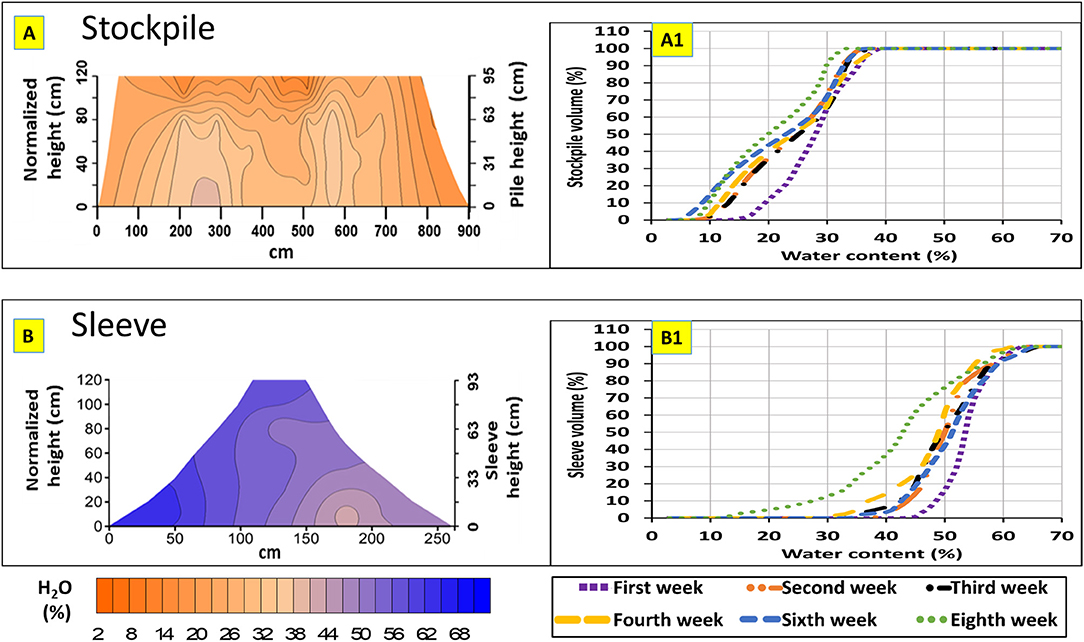
Figure 3. Spatial–temporal variation of water content (%). Contour maps (week 1) and the cumulative volumetric percentage of water content in the stockpile (A,A1) and the sleeve (B; cross-section II, B1; weighted average of cross-sections I–IV).
Sleeve
In contrast to the stockpile, the water content of the BL in the sleeve was pre-adjusted before composting to 51.1 ± 1.7 (Table 1). During the first week, the weighted average gradient of all cross-sections ranged between 43 and 64%. Within this range, only 16% of the sleeve volume was below 50% water content and 7% above 60% (Figure 3B; CS-II). The BL significantly dried out over time (Supplementary Table 1B), without a clear spatial trend. However, slightly drier conditions were observed at the sleeve's base next to the location of the perforated aeration pipe, while the proximity to the blower did not show a consistently significant effect (Supplementary Figure 3-2). A plot of the cumulative volume fractions (Figure 3B1) shows a gradual drying, such that 50% of the material was below water contents of 53–54%, 50%, 49–50%, 49%, 51–52%, and 42–43%, on weeks 1, 2, 3, 4, 6, and 8, respectively. This gradual drying is also expressed by the weighted average water content of all grid cells, which was 53.6, 50.2, 50.1, 47.8, 50.9, and 42.1%, on weeks 1, 2, 3, 4, 6, and 8, respectively. The range of water content that is ideal for composting is not definite (e.g., suggested as 50–60% by Bernal et al., 2009, or 45–65% by Christian et al., 2009). Water contents at the upper range (above 60%) increase water film thickness that fill small pores between particles and, in turn, limit oxygen diffusion throughout the composting material (Richard et al., 2002; Richard, 2004).
The different initial water content of the stockpile vs. the sleeve reflects the standard practice of farmers in which the relatively dry BL is stockpiled (Ogejo and Collins, 2009), while water content is adjusted before composting to optimize biological activity (Walker, 2004). The relatively dry and poorly aerated static stockpile of this study resulted in low biological activity and, in turn, less heat accumulation compared with the moist and forced aerated sleeve setup. Further drying of the stockpile surface was eased by the dry summer weather (a total of 1.7-mm rain) and warm temperatures (max day temperatures of 33.4–36.7°C) during the experimental period.
pH
Stockpile
The initial pH of the BL was 7.0 (Table 1). During the first week (Figure 4A), the pH gradient ranged between ca. 7 at the core and 8.5 at the surface. A similar trend was observed during the whole period (Supplementary Figure 2-3). The average pH was significantly higher in weeks 1–2 than in all other weeks (Supplementary Table 1B). A plot of the cumulative volume fractions (Figure 4A1) does not show a substantial trend over time, such that 50% of the material was below 7–7.5 during the entire period.
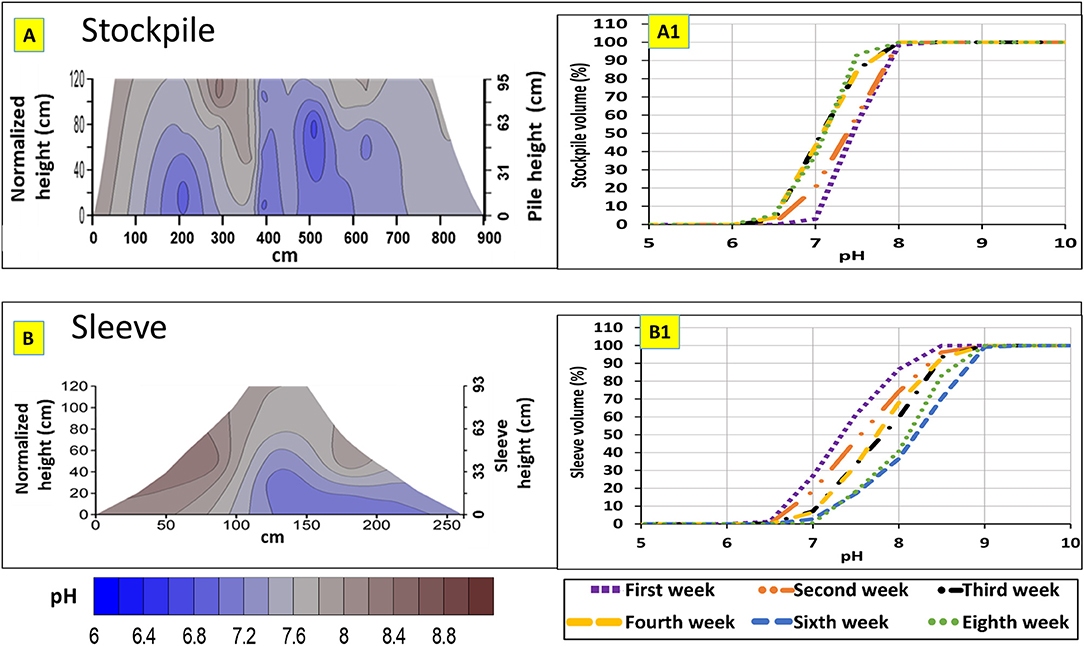
Figure 4. Spatial–temporal variation of pH. Contour maps (week 1) and the cumulative volumetric percentage of pH in the stockpile (A,A1) and the sleeve (B; cross-section II, B1; weighted average of cross-sections I–IV).
Sleeve
The initial pH of the BL was 7.0 (Table 1). During the first week, the weighted average gradient of all cross-sections ranged between 6.5 and 8.5 with a slightly lower pH in the core (Figure 4B; CS-II). Except week 1, the average pH values were significantly higher in the sleeve compared with the stockpile (Supplementary Table 1A). A similar spatial pattern was observed in all cross-sections during the 2 months, while it was more noticeable in CS-I and CS-II (Supplementary Figure 3-3). A plot of the cumulative volume fractions (Figure 4B1) shows a gradual increase in pH, such that 50% of the material was below 7–7.5 on week 1, 7.5–8 on weeks 2–4, and 8–8.5 on weeks 6 and 8. This increase in pH, which was more apparent in the sleeve, is also expressed by the weighted average pH values of all grid cells during the entire period, which was 7.4, 7.6, 7.8, 7.8, 8.1, and 8.0, on weeks 1, 2, 3, 4, 6, and 8, respectively. No consistently significant effect of the proximity to the blower was observed.
In general, the higher pH values in the sleeve can be expected due to the more intense aerobic activity, which, in turn, results in the degradation of organic acids and the release of ammonia during mineralization of proteins, peptides, and amino acid (Gigliotti et al., 2012). On the other hand, poor aeration would lead to the production of acidic compounds, which might explain the slightly lower pH in the core of the stockpile and in some locations within the sleeve. Avidov et al. (2021a) showed a pH decrease from 6.5–7 to 5.5 and an increase from 6.5–6.8 to 7.5–7.6 under anaerobic vs. aerobic degradation of BL, respectively.
Electrical Conductivity (EC)
Stockpile
The initial EC of the BL was 9.9 dS m−1 (Table 1). During the first week (Figure 5A) the EC gradient ranged between 7 and 8 dS m−1 at the surface and 13 dS m−1 at the core and the bottom of the stockpile. A similar trend was observed during the whole period (Supplementary Figure 2-4). A plot of the cumulative volume fractions (Figure 5A1) does not show a trend over time, such that 50% of the material was below 10–11 dS m−1 during the entire period. The average EC values of all grid cells were also similar during the 2 months.
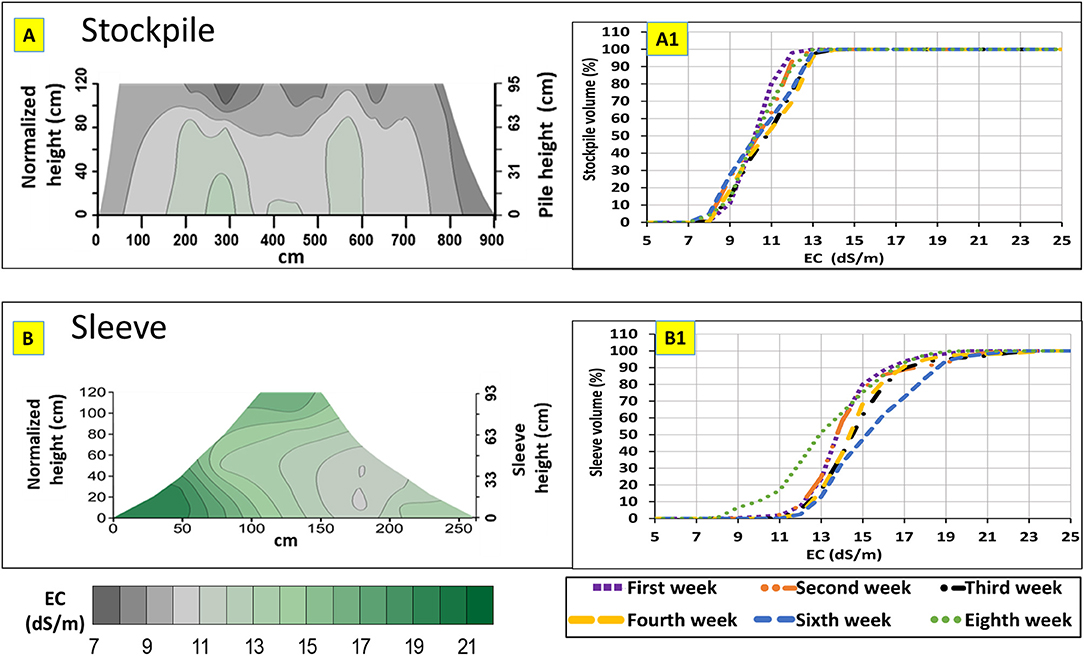
Figure 5. Spatial–temporal variation of EC (dS m−1). Contour maps (week 1) and the cumulative volumetric percentage of EC in the stockpile (A,A1) and the sleeve (B; cross-section II, B1; weighted average of cross-sections I–IV).
Sleeve
The initial EC of the BL was 11.8 dS m−1 (Table 1). In general, significantly higher EC values were measured in the sleeve compared with the pile (Supplementary Table 1A). During the first week, the average gradient of all cross-sections ranged between 8 and 20 dS m−1, with the lower values appearing on one side (Figure 5B; CS-II). Compared with the stockpile in which the maximum value on week 1 was 13 dS m−1, between 63 and 88% of the sleeve cross-sections was above that value. No clear spatial trend was observed during the whole period, while a more noticeable gradient was observed in CS-II and CS-IV (Supplementary Figure 3-4). A plot of the cumulative volume fractions (Figure 5B1) shows some EC increase and then another decrease, such that 50% of the material exhibited values below 13–14 dS m−1 on weeks 1–2, 14–15 dS m−1 on weeks 3–4, 15–16 dS m−1 on week 6, and finally again below 12–13 dS m−1 on week 8. However, no clear spatial trend was observed for the weighted average EC values of all grid cells during the whole period; neither any consistently significant effect could be related to the proximity to the blower.
On the average, the higher EC values in the sleeve can be the result of salt dissolution under higher water content compared with the stockpile. It can also reflect the more intensive aerobic degradation, which in turn results in the reduction of organic matter and increase in mineral (ash) content. Such increase in EC values during composting was reported in multiple studies (Zaha et al., 2013; Avidov et al., 2017; Karanja et al., 2019), although the opposite trend has been reported as well and was attributed to the release of volatile organic sulfur compounds, precipitation of mineral salts, microbial consumption of salts, and leaching of compost piles (Gondek et al., 2020).
Gas-Phase Oxygen
Stockpile
During the first week (Figure 6A), a strong gradient was observed, ranging from 21% at the surface, down to 0–1% at the core. A similar spatial trend was observed during the whole period, whereas the anaerobic fraction was lower on weeks 1 and 8. Of the pile volume, 14, 35, 32, 31, 37, and 19% was below 5% of oxygen on weeks 1, 2, 3, 4, 6, and 8, respectively (Supplementary Figure 2-5). A plot of the cumulative volume fractions (Figure 6A1) is another indication for this trend, such that 50% of the material was below 12–13%, 9–10%, 9–10%, 10–11%, 9–10%, and 12–13% oxygen, on weeks 1, 2, 3, 4, 6, and 8, respectively. Similarly, the average oxygen values of all grid cells were 12.1, 9.5, 9.7, 9.9, 9.1, and 11.8%, on weeks 1, 2, 3, 4, 6, and 8, respectively, although these differences were not significant.
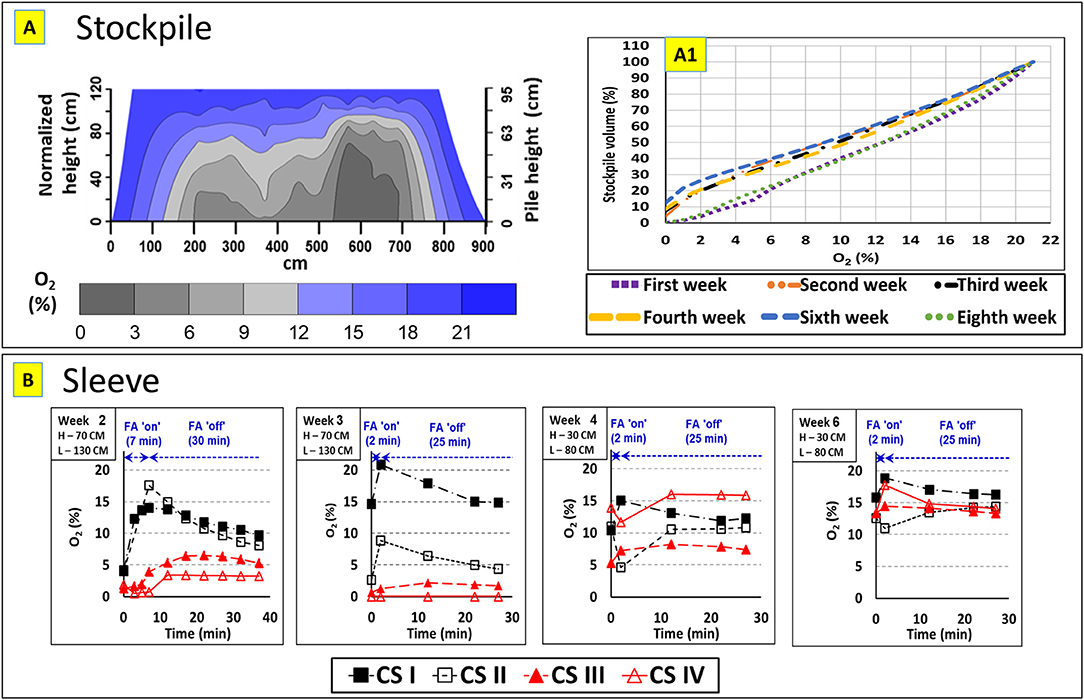
Figure 6. Spatial–temporal variation of gas-phase oxygen (%). A contour map (week 1) and the cumulative volumetric percentage of oxygen are presented for the stockpile (A,A1). Gas-phase oxygen in the sleeve is presented at time windows during which the blower was operated for 2–7 min, and the air was sampled at time intervals up to 30 min thereafter (B).
Sleeve
The levels of oxygen are expected to fluctuate according to aeration on/off episodes. Thus, air sampling was conducted during off-periods following operation of the blower for 2–7 min (Figure 6B). On weeks 2 and 3, it was evident that aeration was more effective in proximity to the blower (CS-I and CS-II), whereas CS-III was mainly below 5% oxygen and CS-IV was around 0% on week 3. This effect decreased on weeks 4 and 6, during which no clear advantage was shown for the proximity to the blower. Moreover, as the composting process progressed, a lower rate of oxygen decline was observed following aeration episodes.
There is a basic difference between gas sampling of the stockpile and the sleeve: Oxygen levels measured in the static stockpile represent the headspace around the sampling point, while those measured in the sleeve following aeration episodes represent an unknown mix of headspace volumes depending on the dynamics of airways, and thus, a full spatial–temporal picture is hardly resolved. Without active turning, the stockpile was poorly aerated, whereas the extensive heat built up at the core did not trigger efficient air convection (the “chimney effect;” Stegenta et al., 2019). Since the water content in the stockpile was low, this poor passive aeration cannot be attributed to reduced air transport due to water-filled pores. Notably, poor aeration was also reported in turned windrow piles with high oxygen variability (Poulsen, 2011; Stegenta et al., 2019).
Regarding the sleeve, the inefficient aeration at the cross-sections away from the blower has not been reported in our earlier studies in which we monitored oxygen levels at limited sampling points (Avidov et al., 2017, 2018). Evidently, the specific configuration of the perforated pipe would affect the flow paths within the sleeve and, in turn, the magnitude of this effect. Compaction of the BL during composting may also reduce aeration efficiency (Avidov et al., 2017), although the present study shows that aeration was improved with time in sections III and IV, presumably due to the gradual drying of the composting material (Figure 3B1). In any case, as long as the holes of the perforated pipe are uniform in size and density (a shelf product), the loss of pressure along the sleeve would reduce aeration efficiency at increased distances from the blower. Practically, longer “on” periods may be applied during sleeve operation to improve aerobic conditions. Alternatively, a stronger blower may be used.
Gas-Phase Ammonia
Stockpile
During the first week (Figure 7A) and later on (Supplementary Figure 2-6), no clear spatial trends were observed. A large variability of ammonia concentrations of 50–700 ppm was recorded throughout the pile, with significantly higher values on week 8 (extreme values over 1,000 ppm). A plot of the cumulative volume fractions (Figure 7A1) shows fluctuations over time, such that 50% of the material was below 100–200, 10–50, 10–50, 10–50, 50–100, and 200–300 ppm on weeks 1, 2, 3, 4, 6, and 8, respectively. These fluctuations are also expressed by the average ammonia concentrations of all grid cells, which were 227, 46.1, 27.7, 47.6, 80.2, and 305 ppm on weeks 1, 2, 3, 4, 6, and 8, respectively.
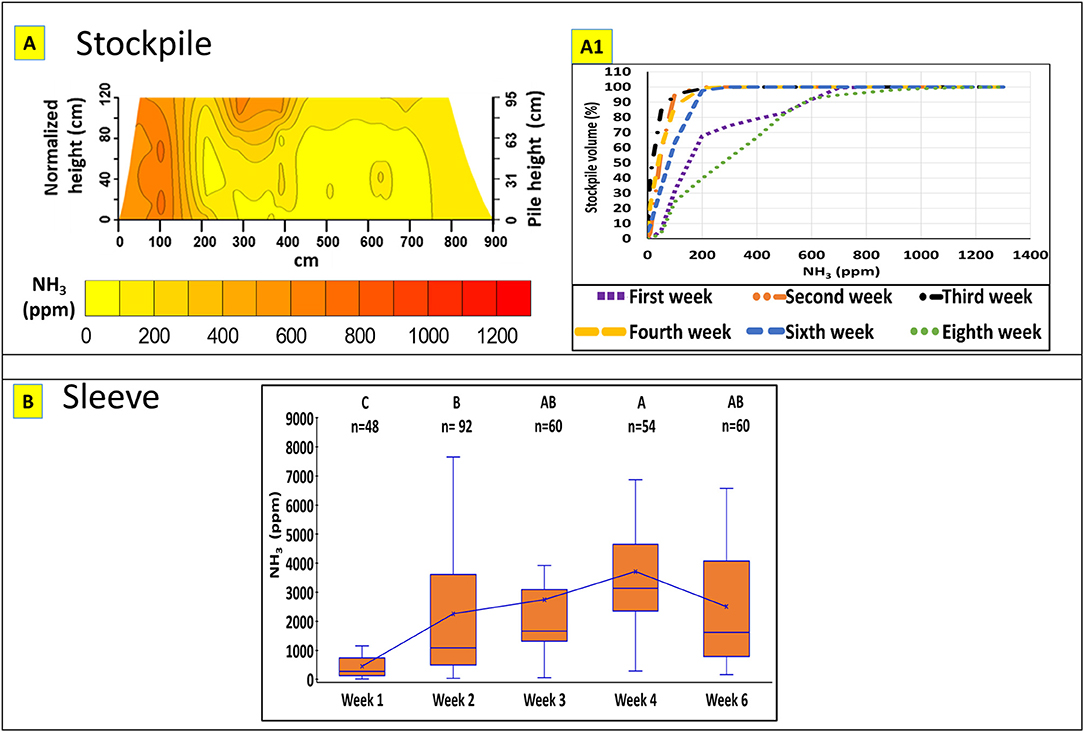
Figure 7. Spatial–temporal variation of gas-phase ammonia (ppm). A contour map (week 1) and the cumulative volumetric percentage of ammonia are presented for the stockpile (A,A1). Gas-phase ammonia in the sleeve is presented in a boxplot of the collective results obtained from all cross-sections on each sampling week (B).
Sleeve
Like oxygen, gas-phase ammonia concentrations were clearly related to the blower operation. However, in contrast to oxygen, no clear dynamics was found during aeration episodes; neither any effect of the proximity to the blower has been observed. Thus, the collective results of all cross-sections are presented for each week on a boxplot (Figure 7B). Generally, as composting proceeded, ammonia emissions increased, but no significant trend was observed between weeks 2, 3, and 6. Overall, ammonia concentrations during aeration episodes were an order of magnitude higher than those measured at the static pile, with average values of 451, 2,258, 2,742, 3,711, and 2,507 ppm, on weeks 1, 2, 3, 4, and 6, respectively.
As noted for oxygen, measurements of gas-phase ammonia in the stockpile represent the headspace around the sampling point, while following aeration episodes of the sleeve, it represents an unknown mix of headspace volumes. Higher ammonia emissions in the sleeve compared with the stockpile are expected, whereas the overall microbial activity is enhanced under aerobic conditions and due to the initially adjusted water content. Kirchmann and Witter (1989) reported that during composting of poultry manure, <1% of the nitrogen was volatilized as NH3 during anaerobic decomposing due to the low pH values.
Mass Degradation and Element Losses in the Stockpile and the Sleeve
The average height of the stockpile was reduced during an 8-week process by 32%, from 113 to 77 cm, and that of the sleeve was reduced by 45%, from 120 to 66 cm. About 50% of this decline occurred during the first week in both setups, indicating the effect of material compaction after BL piling (Table 1 and Supplementary Figure 4). The four cross-sections of the sleeve had a similar height at the beginning, then slightly differentiated, and finally became similar (Supplementary Figure 4B). Other properties measured at the beginning and after 2 months are summarized in Table 1. Ash content slightly increased in both setups, while the ratios between the initial and the final contents were used to estimate the losses of dry matter (Larney and Buckley, 2007; Avidov et al., 2018), yielding 8.4 and 15.5% losses in the stockpile and the sleeve, respectively. The gap between height reductions and dry matter loss estimations is another indication of the co-effect of material compaction and biodegradation. Total nitrogen concentrations decreased by 6.2% in the stockpile and by 19.6% in the sleeve. Based on dry matter losses, these values are equivalent to 14.1 and 32.0% nitrogen losses in the stockpile and the sleeve, respectively. Likewise, the general increase in total P concentrations (Table 1) reflects organic matter degradation and an increase in mineral concentrations (Sommer and Dahl, 1999; Osada et al., 2001). Presumably, dry matter losses are mainly attributed to the degradation of the broiler droppings, and much less to the sawdust that is used for bedding, which is expected to be fairly recalcitrant due to its high contents of lignin and cellulose (Leconte et al., 2009). Nevertheless, the sawdust in Israel is estimated to comprise only about 7% of the total BL on a dry matter basis (N. Cnaan, Director of the Growth Division at “Off Tov Group”, Israel; personal communication). The lower N losses in the stockpile (also expressed by lower ammonia emissions; Figure 7) can be attributed to the anaerobic conditions prevailing in extensive zones of the stockpile (Figure 6). In contrast, the larger amounts of N losses in the sleeve correspond with the study of Avidov et al. (2017), who reported 38–45% losses during 6 months of municipal biosolid composting in sleeves. Ignoring sanitary issues that trigger the motivation for thermal treatment through composting, ammonia volatilization is a negative consequence, being a major mechanism of N losses. From the point of view of plant nutrition, stabilizing BL in a stockpile would be preferred, as less N is lost through ammonia volatilization.
Co-correlations Between Physicochemical Properties in the Stockpile and the Sleeve
Co-correlations between the measured physicochemical properties are visually apparent from the contour maps. Pearson's r linear correlations were determined for every pair of variables, including the values of all grid cells in the constructed contour maps (Table 2 and Supplementary Table 2). Due to the large dataset (all grid cells), the significance of the correlations is always very high (low p-value) and, therefore, not indicated. Generally, higher correlations, either positive or negative, were obtained for the stockpile compared with the sleeve. Considering the contour maps constructed for all weeks in one dataset (Table 2A), high positive correlations (r = 0.74–0.90) were obtained in the stockpile for the pairs of temperature/water content, temperature/EC, water/EC, and pH/oxygen, while high negative correlations (r = −0.60 to −0.87) were observed for the pairs of temperature/pH, temperature/oxygen, water/pH, water/oxygen, pH/EC, and EC/oxygen. The highest correlations were obtained for EC/water (r = 0.90), EC/oxygen (r = −0.87), and water/oxygen (r = −0.85). Ammonia was the least correlated with any of the other properties. The same trends (positive or negative), but less strong correlations, were obtained for the sleeve (Table 2B). These correlations do not include ammonia and oxygen due to the different methodologies used to characterize the gas phase (during “off” aeration, following active aeration episodes). The pair of water/pH did not show a correlation in the sleeve, although it was negatively correlated in the pile. Also, the pair of temperature/EC showed a positive correlation only in CS-III and CS-IV (away from the blower). The dynamics of these correlations was not consistent as composting progressed, except for the sleeve on the first week, during which temperature and water positively correlated with pH, while the opposite trend was observed in the stockpile and sometimes in the sleeve (Supplementary Table 2).
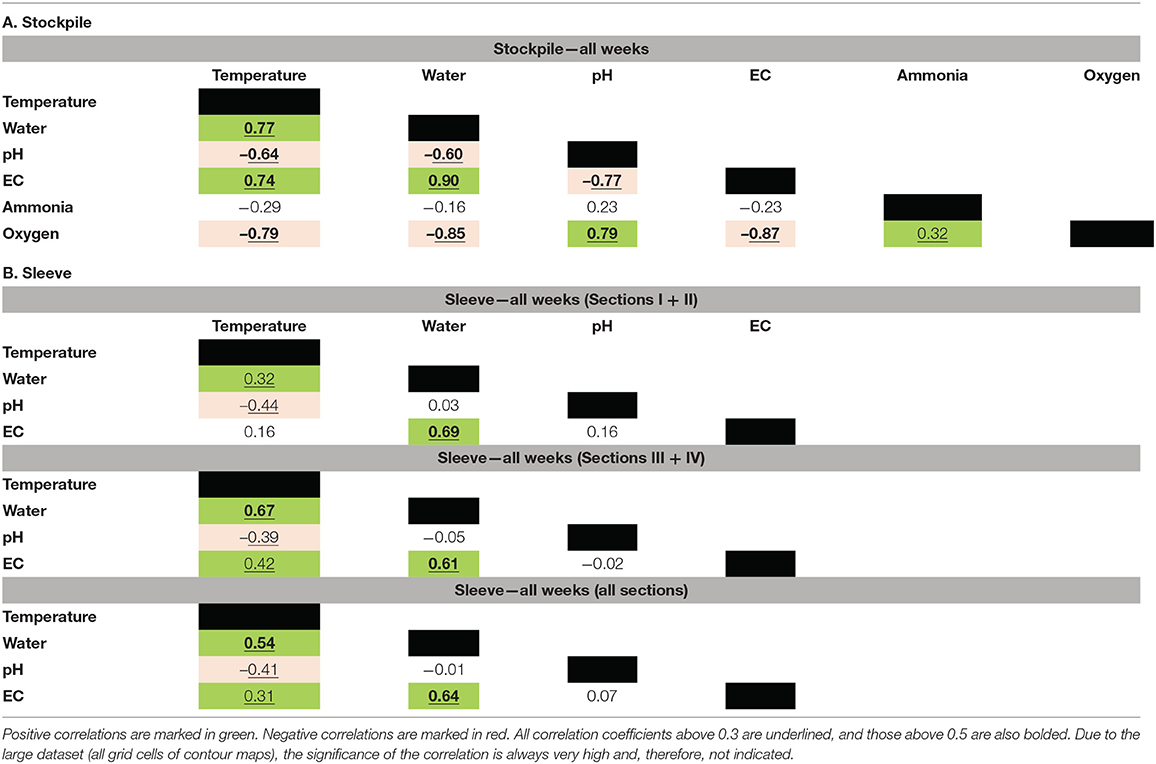
Table 2. Pearson's r linear correlation coefficients among the physicochemical properties measured in the stockpile and the sleeve during 2 months.
These correlations reflect the co-dynamics of key properties during composting, while the static vs. dynamic settings of the stockpile and the sleeve affected such interactions differently. Under static conditions, passive aeration is inefficient, which is reflected by a strong oxygen gradient. Oxidation reactions within the core of the stockpile still result in heat accumulation (less than in the sleeve), while temperature correlates with the water content, which is required for biological activity, on one hand, and is a byproduct of aerobic degradation, on the other hand. Also, biological activity at the stockpile margins and the top surface may respond to the cooling and drying effect of the ambient atmosphere. Intense aerobic degradation also correlated with EC, thus, reflecting, on one hand, possible salt dissolution at higher water content, and it is also related to the process of increases in ash content. The same trends, which were found in the sleeve, yet of less strong correlations, probably reflect highly dynamic spatial–temporal distribution of oxygen and related physicochemical properties, as resulting from active aeration. This interpretation is supported by the slightly stronger correlations observed for CS-III and CS-IV that were aerated less efficiently and, thus, presented similar environments to the static stockpile.
Assessing the Persistence of S. Infantis in the Stockpile and the Sleeve
Assessment of the spatial persistence of S. Infantis in the stockpile is presented in Figure 8, based on the exponential decay constants reported by Avidov et al. (2021a) and the grid maps constructed in this study. As shown in Supplementary Table 3, the measured conditions of temperature, water content, and pH, are matched with 10 (stockpile) and 17 (sleeve) out of the 36 combinations studied by Avidov et al. (2021a), whereas combinations including temperatures of ≤35°C prevailed only in the stockpile. The spatial persistence of Salmonella is expressed by log10 reduction after 1 and 2 weeks (Figure 8). For example, 68.3% of the stockpile volume reached the conditions under which at least 8 log10 reduction is expected after 1 week. Thus, assuming an initial concentration of 7–8 log10 CFU g−1 BL, we expect that 25.3% of the stockpile volume (6–7 log10 reduction) plus 1.4% (3–4 log10 reduction) will still be contaminated with the pathogen after 1 week. Similarly, a volume fraction of 1.3% will still be contaminated by the end of 2 weeks. If the initial concentration of the pathogen would be lower, e.g., 2–3 log10 CFU g−1 BL, then it will be eliminated from the entire stockpile volume within 1 week only. The zones that are most prone to incomplete inactivation of the pathogen would be located at the pile margins, which were generally cooler than the core. Moreover, the physicochemical properties at the pile margins are affected by the environmental condition, like temperature, wind, precipitations, shading, or direct sun exposure (the potential role of UV radiation is considered irrelevant since the polyethylene sheet becomes heavily dirty). A secondary effect of both water content and pH, is shown at the top of the stockpile, which was also relatively cool but matched with a different combination of temperature, water content, and pH, for which Avidov et al. (2021a) reported higher Salmonella persistence.
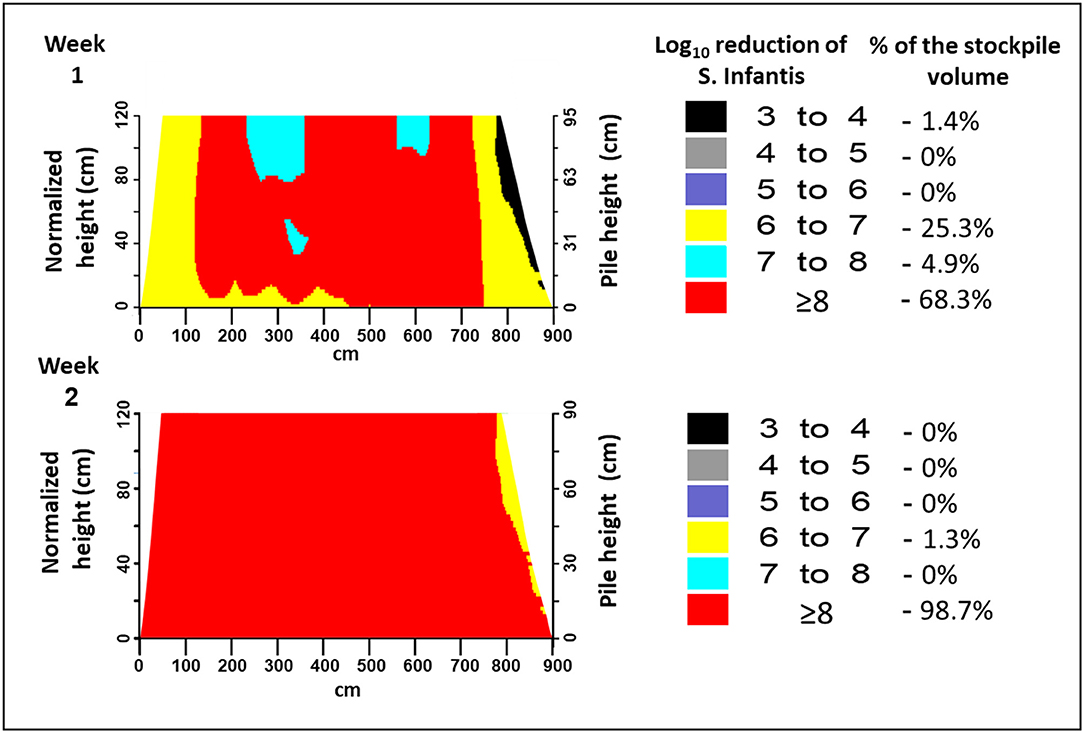
Figure 8. Assessments of the spatial persistence of S. Infantis in the stockpile, based on the exponential decay constants from Avidov et al. (2021a) and the grid maps constructed in this study for temperature, water content, and pH.
Since Salmonella concentrations in poultry litter are typically in the range of 3–5 log10 CFU g−1 litter (Chinivasagam et al., 2009, 2010; Brooks et al., 2010), the assessments shown in Figure 8 suggest that 2 weeks should be sufficient for S. Infantis elimination in a stockpile. Yet, under certain conditions, such as drying and re-wetting, Salmonella can multiply in the BL and increase by several orders of magnitude (Avidov et al., 2021a). Thus, for minimizing risks, a total of 3 weeks may be recommended before land application of BL stockpiles. In contrast, the same analysis for the sleeve yields over 8 log10 reduction of S. Infantis in the entire volume after 1 week only. This result is a direct expression of the significantly higher temperatures prevailing in the sleeve, while the relatively cool margins were still warm enough to be represented by a relatively low persistence of the pathogen. A secondary effect of water content and pH was noticeable only in the relatively cool stockpile, in which mesophilic temperatures were recorded in the first few weeks. Avidov et al. (2021a) showed that temperature was the main factor influencing Salmonella decay rates, while water content and pH mainly had an influence at 30 and 40°C.
Overall, this approach that combines high-resolution spatial field data along with decay rates of pathogens under controlled lab conditions may improve quantitative microbial risk assessments of manure utilization. Such assessments can hardly be validated in the field, since BL stockpiles or other field-scale setups cannot be artificially inoculated with any specific pathogen. Moreover, homogeneous inoculation of such volumes is technically impossible and would not be allowed for sanitary reasons. Yet, the approach presented in this study can assist with regulations for any non- or forced-aerated static setups. It is much more informative than common regulations, e.g., the requirement for a minimum of 55°C for three consecutive days. As stated, although thermophilic temperatures are reported in multiple composting studies, only average or min and max values are often presented, while the spatial distribution remains unknown. In such cases, a pseudo-compliance with regulations may be shown just because of misrepresentation of the litter. As shown in this study, 83, 71, and 62% of the stockpile volume did not reach the commonly required minimum temperature of 55°C for three consecutive days during the first, second, and third weeks, respectively. Using the combined field-lab assessments, we would recommend a period of 3 weeks before safe land application. The assessment made in this study are based on temperature, water content, and pH only. Yet, the co-correlations found between temperature, water content, EC, and oxygen suggest that selected physicochemical properties may be sufficient for such assessments. Moreover, upon mapping the spatial distribution of key physical properties of relevant manure processing setups, this approach can be used to assess the spatial persistence of any pathogen that is tested under lab-controlled physicochemical conditions.
Conclusions
Static BL stockpiles are expected to develop a highly spatially non-homogeneous environment. The spatial–temporal distribution of physicochemical properties, combined with decay models, can be used to determine the persistence of zoonotic pathogens residing within livestock manure. Out of the measured properties, temperature is expected to remain a major factor, although the distribution of other properties should be considered, based on lab-scale controlled experiments. Co-effects of other physicochemical properties besides temperature are most relevant under mesophilic conditions and, thus, are applicable for manure stockpiles, but not for enclosed sleeves with forced aeration or any enclosed setups that ensure high thermophilic temperatures in the entire volume of the BL. Although only a single trial was conducted due to the intense labor demand in such a study, we assume that similar trends will be observed in future trials of such representing setups. Up to three weeks would be recommended to achieve 7–8 log10 reduction of S. Infantis in BL stockpile, while this would be fully achieved within 1 week in composting sleeves. Ammonia volatilization during composting, on one hand, and the cost and labor associated with the sleeves, on the other hand, imply that composting in such setups may be longer than the time needed for pathogen inactivation but is still relatively short, sufficient enough to achieve other goals like reduction of odors and vector attraction. An approach that combines high-resolution field data, along with decay rates of pathogens under controlled lab conditions, may improve quantitative microbial risk assessments of manure utilization. It can be recommended as a universal approach in assessing the spatial persistence of other pathogens tested under controlled physicochemical conditions and analyzed against field-based detailed grid maps.
Data Availability Statement
The raw data supporting the conclusions of this article will be made available by the authors, without undue reservation.
Author Contributions
RA: conceptualization, methodology, data analysis, and writing original draft. VV: conceptualization and methodology. IS: methodology and resources. OK: methodology. YC: supervision and reviewing. YL: supervision, conceptualization, writing, and editing. All authors contributed to the article and approved the submitted version.
Funding
This study was supported by the Chief Scientist of the Israel Ministry of Agriculture and Rural Development (Project title: Mitigation of Salmonella and Campylobacter contamination in poultry, No. 20-14-0032) and the Division of Poultry at the Israel Ministry of Agriculture and Rural Development (Project title: Composting of poultry carcasses in closed sleeves inside the poultry house—a contingency solution for mass mortality events, No. 356-1111-17).
Conflict of Interest
The authors declare that the research was conducted in the absence of any commercial or financial relationships that could be construed as a potential conflict of interest.
Publisher's Note
All claims expressed in this article are solely those of the authors and do not necessarily represent those of their affiliated organizations, or those of the publisher, the editors and the reviewers. Any product that may be evaluated in this article, or claim that may be made by its manufacturer, is not guaranteed or endorsed by the publisher.
Supplementary Material
The Supplementary Material for this article can be found online at: https://www.frontiersin.org/articles/10.3389/fsufs.2022.811530/full#supplementary-material
References
APHA (2005). Standard Methods for the Examination of Water and Wastewater (21st edn). Washington, DC: American Public Health Association.
Aviani, I., Laor, Y., Medina, S., Krassnovsky, A., and Raviv, M. (2010). Co-composting of solid and liquid olive mill wastes: management aspects and the horticultural value of the resulting composts. Bioresour. Technol. 101, 6699–6706. doi: 10.1016/j.biortech.2010.03.096
Avidov, R., Saadi, I., Krasnovsky, A., Medina, S., Raviv, M., Chen, Y., et al. (2018). Using polyethylene sleeves with forced aeration for composting olive mill wastewater pre-absorbed by vegetative waste. Waste Manag. 78, 969–979. doi: 10.1016/j.wasman.2018.06.021
Avidov, R., Saadi, I., Krassnovsky, A., Hanan, A., Medina, S., Raviv, M., et al. (2017). Composting municipal biosolids in polyethylene sleeves with forced aeration: process control, air emissions, sanitary and agronomic aspects. Waste Manag. 67, 32–42. doi: 10.1016/j.wasman.2017.05.035
Avidov, R., Varma, V. S., Saadi, I., Hanan, A., Lublin, A., Chen, Y., et al. (2021a). Factors influencing the persistence of Salmonella infantis in broiler litter during stabilization and composting processes and following soil incorporation. Front. Sustain. Food Syst. 5, 106. doi: 10.3389/fsufs.2021.645721
Avidov, R., Varma, V. S., Saadi, I., Hanan, A., Medina, Sh., Lublin, A., et al. (2019). Composting of poultry carcasses in closed sleeves inside the chicken coops - a “contingency solution” for mass mortality events in poultry farms. Meshek Ha'Ofot April 2019. p. 82–88 (In Hebrew).
Avidov, R., Varma, V. S., Saadi, I., Hanan, A., Yoselevich, I., Lublin, A., et al. (2021b). Physical and chemical indicators of transformations of poultry carcass parts and broiler litter during short term thermophilic composting. Waste Manage. 119, 202–214. doi: 10.1016/j.wasman.2020.09.040
Bell, R. L., Zheng, J., Burrows, E., Allard, S., Wang, C. Y., Keys, C. E., et al. (2015). Ecological prevalence, genetic diversity, and epidemiological aspects of Salmonella isolated from tomato agricultural regions of the Virginia Eastern Shore. Front. Microbiol. 6, 415. doi: 10.3389/fmicb.2015.00415
Bernal, M. P., Alburquerque, J. A., and Moral, R. (2009). Composting of animal manures and chemical criteria for compost maturity assessment. A review. Bioresource Technol. 100, 5444–5453. doi: 10.1016/j.biortech.2008.11.027
Beuchat, L. R. (2002). Ecological factors influencing survival and growth of human pathogens on raw fruits and vegetables. Microbes Infect. 4, 413–423. doi: 10.1016/S1286-4579(02)01555-1
Bolan, N. S., Szogi, A. A., Chuasavathi, T., Seshadri, B., Rothrock, M. J., and Panneerselvam, P. (2010). Uses and management of poultry litter. Worlds Poult. Sci. J. 66, 673–698. doi: 10.1017/S0043933910000656
Brooks, J. P., McLaughlin, M. R., Scheffler, B., and Miles, D. M. (2010). Microbial and antibiotic resistant constituents associated with biological aerosols and poultry litter within a commercial poultry house. Sci. Total Environ. 408, 4770–4777. doi: 10.1016/j.scitotenv.2010.06.038
Bush, D. J., Poore, M. H., Rogers, G. M., and Altier, C. (2007). Effect of stacking method on Salmonella elimination from recycled poultry bedding. Bioresour. Technol. 98, 571–578. doi: 10.1016/j.biortech.2006.02.017
Cassity-Duffey, K., Cabrera, M., and Rema, J. (2015). Ammonia volatilization from broiler litter: effect of soil water content and humidity. Soil Sci. Soc. Am. J. 79, 543–550. doi: 10.2136/sssaj2014.07.0294
Chaudhry, S. M., Fontenot, J. P., and Naseer, Z. (1998). Effect of deep stacking and ensiling broiler litter on chemical composition and pathogenic organisms. Anim. Feed Sci. Technol. 74, 155–167. doi: 10.1016/S0377-8401(98)00125-4
Chaves, R. D., Martinez, R. C. R., Rezende, A. C. B., Rocha, M. D., Oteiza, J. M., Sant'Ana, A., et al. (2016). “Salmonella and Listeria monocytogenes in ready-to-eat leafy vegetables,” in Food Hygiene and Toxicology in Ready-to-Eat Foods, ed P. Kotzekidou (Academic Press), 123–149. doi: 10.1016/B978-0-12-801916-0.00008-X
Chen, Z., Diao, J., Dharmasena, M., Ionita, C., Jiang, X., and Rieck, J. (2013). Thermal inactivation of desiccation-adapted Salmonella spp. in aged chicken litter. Appl. Environ. Microbiol. 79, 7013–7020. doi: 10.1128/AEM.01969-13
Chen, Z., and Jiang, X. (2014). Microbiological safety of chicken litter or chicken litter-based organic fertilizers: a review. Agriculture 4, 1–29. doi: 10.3390/agriculture4010001
Chinivasagam, H. N., Redding, M., Runge, G., and Blackall, P. J. (2010). Presence and incidence of food-borne pathogens in australian chicken litter. Br. Poult. Sci. 51, 311–318. doi: 10.1080/00071668.2010.499424
Chinivasagam, H. N., Tran, T., Maddock, L., Gale, A., and Blackall, P. J. (2009). Mechanically ventilated broiler sheds: a possible source of aerosolized Salmonella, Campylobacter, and Escherichia coli. Appl. Environ. Microbiol. 75, 7417–7425. doi: 10.1128/AEM.01380-09
Christian, A. H., Evanylo, G. K., and Pease, J. W. (2009). On Farm Composting – A Guide to Principles, Planning and Operations. Virginia Cooperative Extension Publications No. 452-232. Petersburg, VA: Virginia State University.
Déportes, I., Benoit-Guyod, J. L., Zmirou, D., and Bouvier, M. C. (1998). Microbial disinfection capacity of municipal solid waste (MSW) composting. J. Appl. Microbiol. 85, 238–246. doi: 10.1046/j.1365-2672.1998.00484.x
Erickson, M. C., Liao, J., Boyhan, G., Smith, C., Ma, L., Jiang, X., et al. (2010). Fate of manure-borne pathogen surrogates in static composting piles of chicken litter and peanut hulls. Bioresour. Technol. 101, 1014–1020. doi: 10.1016/j.biortech.2009.08.105
Fatica, M. K., and Schneider, K. R. (2011). Salmonella and produce: survival in the plant environment and implications in food safety. Virulence 2, 573–579. doi: 10.4161/viru.2.6.17880
Fernandes, L., Zhan, W., Patni, N. K., and Jui, P. Y. (1994). Temperature distribution and variation in passively aerated static compost piles. Bioresour. Technol. 48, 257–263. doi: 10.1016/0960-8524(94)90155-4
Gigliotti, G., Proietti, P., Said-Pullicino, D., Nasini, L., Pezzolla, D., Rosati, L., et al. (2012). Co-composting of olive husks with high moisture contents: organic matter dynamics and compost quality. Int. Biodeterior. Biodegrad. 67, 8–14. doi: 10.1016/j.ibiod.2011.11.009
Gondek, M., Weindorf, D. C., Thiel, C., and Kleinheinz, G. (2020). Soluble salts in compost and their effects on soil and plants: a review. Compost Sci. Utilization 28, 59–75. doi: 10.1080/1065657X.2020.1772906
Gould, L. H., Mungai, E. A., Johnson, S. D., Richardson, L. C., Williams, I. T., Griffin, P. M., et al. (2013). Surveillance for foodborne disease outbreaks—United States, 2009–2010. MMWR Morb. Mortal.Wkly. Rep. 62, 41. Available online at: https://www.jstor.org/stable/24806072
Grinhut, Z., Zadikov, I., Fradkin, A., Goldfrb, O., Almod, R., Hadas, E., et al. (2015). By-Products in Israeli Agriculture. A Summary Document for Policy Determination and Cost Estimation. Ministry of Agriculture and Ministry of Environmental Protection. (In Hebrew).
Gu, G., Strawn, L. K., Oryang, D. O., Zheng, J., Reed, E. A., Ottesen, A. R., et al. (2018). Agricultural practices influence Salmonella contamination and survival in pre-harvest tomato production. Front. Microbiol. 9, 2451. doi: 10.3389/fmicb.2018.02451
Gurtler, J. B., Doyle, M. P., Erickson, M. C., Jiang, X., Millner, P., and Sharma, M. (2018). Composting to inactivate foodborne pathogens for crop soil application: a review. J. Food Prot. 81, 1821–1837. doi: 10.4315/0362-028X.JFP-18-217
Haga, K., Osada, T., Harada, Y., Izawa, T., and Nishimura, Y. (1998). Constituents of the anaerobic portion occurring in the pile during composting of cattle wastes. Nogyo Shisetsu 29, 125–130.
Herman, K. M., Hall, A. J., and Gould, L. H. (2015). Outbreaks attributed to fresh leafy vegetables, United States, 1973–2012. Epidemiol. Infect. 143, 3011–3021. doi: 10.1017/S0950268815000047
Himathongkham, S., and Riemann, H. (1999). Destruction of Salmonella typhimurium, Escherichia coli O157:H7 and Listeria monocytogenes in chicken manure by drying and/or gassing with ammonia. FEMS Microbiol. Lett. 171, 179–182. doi: 10.1111/j.1574-6968.1999.tb13430.x
Hruby, C. E., Soupir, M. L., Moorman, T. B., Pederson, C., and Kanwar, R. (2018). Salmonella and fecal indicator bacteria survival in soils amended with poultry manure. Water Air Soil Pollut. 229, 32. doi: 10.1007/s11270-017-3667-z
Islam, M., Doyle, M. P., Phatak, S. C., Millner, P., and Jiang, X. (2004a). Survival of Escherichia coli O157:H7 in soil and on carrots and onions grown in fields treated with contaminated manure composts or irrigation water. Food Microbiol. 22, 63–70. doi: 10.1016/j.fm.2004.04.007
Islam, M., Morgan, J., Doyle, M. P., Phatak, S. C., Millner, P., and Jiang, X. (2004b). Fate of Salmonella enterica Serovar Typhimurium on carrots and radishes grown in fields treated with contaminated manure composts or irrigation water. Appl. Environ. Microbiol. 70, 2497–2502. doi: 10.1128/AEM.70.4.2497-2502.2004
Isobaev, P., Bouferguene, A., Wichuk, K. M., and McCartney, D. (2014). An enhanced compost temperature sampling framework: case study of a covered aerated static pile. Waste Manag. 34, 1117–1124. doi: 10.1016/j.wasman.2014.03.016
Jechalke, S., Schierstaedt, J., Becker, M., Flemer, B., Grosch, R., Smalla, K., et al. (2019). Salmonella establishment in agricultural soil and colonization of crop plants depend on soil type and plant species. Front. Microbiol. 10, 967. doi: 10.3389/fmicb.2019.00967
Karanja, A. W., Njeru, E. M., and Maingi, J. M. (2019). Assessment of physicochemical changes during composting rice straw with chicken and donkey manure. Int. J. Recycl. Organic Waste Agric. 8, 65–72. doi: 10.1007/s40093-019-0270-x
Kim, J., Diao, J., Shepherd, M. W., Singh, R., Heringa, S. D., Gong, C., et al. (2012). Validating thermal inactivation of Salmonella spp. in fresh and aged chicken litter. Appl. Environ. Microbiol. 78, 1302–1307. doi: 10.1128/AEM.06671-11
Kingery, W. L., Wood, C. W., Delaney, D. P., Williams, J. C., and Mullins, G. L. (1994). Impact of long-term land application of broiler litter on environmentally related soil properties. J. Environ. Qual. 23, 139–147. doi: 10.2134/jeq1994.00472425002300010022x
Kirchmann, H., and Witter, E. (1989). Ammonia volatilization during aerobic and anaerobic manure decomposition. Plant Soil 115, 35–41. doi: 10.1007/BF02220692
Larney, F. J., and Buckley, K. E. (2007). Dry matter mass balance estimates for composted feedlot manure. Compost Sci. Util. 15, 222–227. doi: 10.1080/1065657X.2007.10702337
Larney, F. J., Yanke, L. J., Miller, J. J., and McAllister, T. A. (2003). Fate of coliform bacteria in composted beef cattle feedlot manure. J. Environ. Qual. 32, 1508. doi: 10.2134/jeq2003.1508
Leconte, M. C., Mazzarino, M. J., Satti, P., Iglesias, M. C., and Laos, F. (2009). Co-composting rice hulls and/or sawdust with poultry manure in NE Argentina. Waste Manag. 29, 2446–2453. doi: 10.1016/j.wasman.2009.04.006
Liu, T. S., Snoeyenbos, G. H., and Carlson, v L. (1969). The effect of moisture and storage temperature on a Salmonella Senftenburg 775W population in meat and bone meal. Poult. Sci. 48, 1628–1633. doi: 10.3382/ps.0481628
Macklin, K. S., Hess, J. B., and Bilgili, S. F. (2008). In-house windrow composting and its effects on foodborne pathogens. J. Appl. Poult. Res. 17, 121–127. doi: 10.3382/japr.2007-00051
Marshall, S. B., Wood, C. W., Braun, L. C., Cabrera, M. L., Mullen, M. D., and Guertal, E. A. (1998). Ammonia volatilization from tall fescue pastures fertilized with broiler litter. J. Environ. Qual. 27, 1125–1129. doi: 10.2134/jeq1998.00472425002700050018x
Millner, P. D., Powers, K. E., Enkiri, N. K., and Burge, W. D. (1987). Microbially mediated growth suppression and death of Salmonella in composted sewage sludge. Microb. Ecol. 14, 255–265. doi: 10.1007/BF02012945
Mitchell, C. C., and Tu, S. (2006). Nutrient accumulation and movement from poultry litter. Soil Sci. Soc. Am. J. 70, 2146–2153. doi: 10.2136/sssaj2004.0234
Ogejo, J. A., and Collins, E. R. (2009). Storing and Handling Poultry Litter. Virginia Cooperative Extension publication 442-054.
Osada, T., Sommer, S. G., Dahl, P., and Rom, H. B. (2001). Gaseous emission and changes in nutrient composition during deep litter composting. Acta Agric. Scand. Sect. B Soil Plant Sci. 51, 137–142. doi: 10.1080/09064710127614
Pereira-Neto, J. T., Stentiford, E. I., and Smith, D. V. (1986). Survival of faecal indicator micro-organisms in refuse/sludge composting using the aerated static pile system. Waste Manag. Res. 4, 397–406. doi: 10.1177/0734242X8600400158
Poulsen, T. G. (2011). Oxygen and carbon dioxide distribution and movement in passively aerated compost piles. Compost Sci. Util. 19, 25–32. doi: 10.1080/1065657X.2011.10736973
Poultry Council and Chicken Health Labs (2020). Report of the Chicken Health Laboratories for the Year 2020. Availabel online at: http://www.ofotm.org.il/Media/Uploads/%D7%93%D7%95%D7%97_%D7%A9%D7%A0%D7%AA%D7%99__2020.pdf (accessed January 3, 2022).
Raviv, M., Medina, S., and Shamir, Y. (1999). Cocomposting – a method to improve results of poultry manure composting. Compost Sci. Util. 7, 70–73. doi: 10.1080/1065657X.1999.10701966
Reynnells, R., Ingram, D. T., Roberts, C., Stonebraker, R., Handy, E. T., Felton, G., et al. (2014). Comparison of U.S. environmental protection agency and U.S. composting council microbial detection methods in finished compost and regrowth potential of Salmonella spp. and Escherichia coli O157:H7 in finished compost. Foodborne Pathog. Dis. 11, 555–567. doi: 10.1089/fpd.2013.1698
Richard, T. (2004). “Fundamentals parameters of aerobic solid-state bioconversion processes,” in Resource Recovery and Reuse in Organic Solid Waste Management, eds P. Lens, B. Hamelers, H. Hoitink, and W. Bidlingmaier (London: IWA Publishing).
Richard, T. L., Hamelers, H. V. M., Veeken, A., and Silva, T. (2002). Moisture relationships in composting processes. Compost Sci. Utiliz. 10, 286–302. doi: 10.1080/1065657X.2002.10702093
Singh, R., Kim, J., and Jiang, X. (2012). Heat inactivation of Salmonella spp. in fresh poultry compost by simulating early phase of composting process. J. Appl. Microbiol. 112, 927–935. doi: 10.1111/j.1365-2672.2012.05268.x
Sommer, S. G., and Dahl, P. (1999). Nutrient and carbon balance during the composting of deep litter. J. Agric. Eng. Res. 74, 145–153. doi: 10.1006/jaer.1999.0446
Stegenta, S., Sobieraj, K., Pilarski, G., Koziel, J. A., and Białowiec, A. (2019). Analysis of the spatial and temporal distribution of process gases within municipal biowaste compost. Sustainability 11. doi: 10.3390/su11082340
Stephenson, A. H., McCaskey, T. A., and Ruffin, B. G. (1990). A survey of broiler litter composition and potential value as a nutrient resource. Biol. Wastes 34, 1–9. doi: 10.1016/0269-7483(90)90139-J
Tiquia, S. M., Tam, N. F. Y., and Hodgkiss, I. J. (1998). Salmonella elimination during composting of spent pig litter. Bioresour. Technol. 63, 193–196. doi: 10.1016/S0960-8524(97)00113-2
USEPA (2003). Environmental Regulations and Technology: Control of Pathogens and Vector Attraction in Sewage Sludge, Washington, DC.
Van Herk, F. H., McAllister, T. A., Larney, F. J., Miller, J. J., Cockwill, C. L., Guselle, N., et al. (2004). Inactivation of giardia cysts and cryptosporidium oocysts in beef feedlot manure by thermophilic windrow composting. Compost Sci. Util. 12, 235–241. doi: 10.1080/1065657X.2004.10702188
Vinnerås, B., Björklund, A., and Jönsson, H. (2003). Thermal composting of faecal matter as treatment and possible disinfection method - laboratory-scale and pilot-scale studies. Bioresour. Technol. 88, 47–54. doi: 10.1016/S0960-8524(02)00268-7
Walker, F. (2004). On-Farm Composting of Poultry Litter. The University of Tennessee, Agricultural Extension Service. PandSS Info: 319. Available online at: http://www.agriculture.utk.edu/ansci/poultry/PSS319.htm (accessed December 30, 2021).
Wichuk, K. M., and McCartney, D. (2007). A review of the effectiveness of current time-temperature regulations on pathogen inactivation during composting. J. Environ. Eng. Sci. 6, 573–586. doi: 10.1139/S07-011
Wilkinson, K. G. (2007). The biosecurity of on-farm mortality composting. J. Appl. Microbiol. 102, 609–618. doi: 10.1111/j.1365-2672.2006.03274.x
Wilkinson, K. G., Tee, E., Tomkins, R. B., Hepworth, G., and Premier, R. (2011). Effect of heating and aging of poultry litter on the persistence of enteric bacteria. Poult. Sci. 90, 10–18. doi: 10.3382/ps.2010-01023
Wilkinson, S. R. (1979). Plant nutrient and economic valus of animal manures. J. Anim. Sci. 48, 121–133. doi: 10.2527/jas1979.481121x
Williams, J. E., and Benson, S. T. (1978). Survival of Salmonella typhimurium in poultry feed and litter at three temperatures. Avian Dis. 22, 742–747. doi: 10.2307/1589652
Willis, R. B., Montgomery, M. E., and Allen, P. R. (1996). Improved method for manual, colorimetric determination of total kjeldahl nitrogen using salicylate. J. Agric. Food Chem. 44, 1804–1807. doi: 10.1021/jf950522b
Yeager, J. G., and Ward, R. L. (1981). Effects of moisture content on long-term survival and regrowth of bacteria in wastewater sludge. Appl. Environ. Microbiol. 41, 1117–1122. doi: 10.1128/aem.41.5.1117-1122.1981
Keywords: poultry manure, pathogens, grid maps, contour maps, decay constants, quantitative microbial risk assessment, Salmonella survival/die-off
Citation: Avidov R, Varma VS, Saadi I, Khoury O, Chen Y and Laor Y (2022) A Combined Field–Lab Approach for Assessing Salmonella Infantis Persistence in Broiler Litter in a Stockpile and Composting Sleeve. Front. Sustain. Food Syst. 6:811530. doi: 10.3389/fsufs.2022.811530
Received: 08 November 2021; Accepted: 24 January 2022;
Published: 17 March 2022.
Edited by:
Jesus Fernandez Bayo, University of California, Davis, United StatesReviewed by:
Keith Warriner, University of Guelph, CanadaJuliano Toniato, University of California, Davis, United States
Copyright © 2022 Avidov, Varma, Saadi, Khoury, Chen and Laor. This is an open-access article distributed under the terms of the Creative Commons Attribution License (CC BY). The use, distribution or reproduction in other forums is permitted, provided the original author(s) and the copyright owner(s) are credited and that the original publication in this journal is cited, in accordance with accepted academic practice. No use, distribution or reproduction is permitted which does not comply with these terms.
*Correspondence: Yael Laor, bGFvckB2b2xjYW5pLmFncmkuZ292Lmls
†Present address: Vempalli Sudharsan Varma, Ralph E. Martin Department of Chemical Engineering, University of Arkansas, Fayetteville, AR, United States