- 1Department of Agriculture and Fisheries, Ecosciences Precinct, Brisbane, QLD, Australia
- 2Biosecurity Sciences Laboratory, Department of Agriculture and Fisheries, Health and Food Sciences Precinct, Brisbane, QLD, Australia
- 3Health and Environment Group, Institute of Environmental Science and Research, Christchurch, New Zealand
To inform Salmonella on-farm management during broiler rearing, a 2-year study on two farms compared the Australian practices of new bedding use, partial litter re-use and an alternative, full litter re-use. Six sequential commercial cycles of ~50 days each were tested on each farm, on ~day 7 from placement (litter only), prior to first thin-out, and prior to final removal (litter and ceca). A random number sample collection occurred, defined by shed supports (33, 39), different drinkers, feeders, and shed center. Across the six cycles on both farms, Salmonella levels in ceca just prior to thin-out on full re-use litter were higher (log 3.11 MPN/g, P = 0.008) than for new bedding (log 2.04 MPN/g) and partial re-use (log 2.43 MPN/g) litter (the latter two were not significantly different). Prior to final removal across all practices the Salmonella levels in ceca from new bedding (log 1.72 MPN/g), partial re-use litter (log 1.77 MPN/g), and full re-use litter (log 2.33 MPN/g) were not statistically different, suggesting no effect of litter practice. The Salmonella levels in litter prior to the first (log 1.96–2.31 MPN/g) and second (log 2.24–2.48 MPN/g) removals were also not statistically different. The emergence of Salmonella serovars in the partitioned chicken-free grow-out end (back) of all sheds at ~day 7 did not suggest carry-over. Both the pattern of emergence of Salmonella serovars and Salmonella levels in litter ~day 7 in the brooder-end with chickens (front), suggested the Salmonella present were due to flock contribution and not practice driven. The dominant Salmonella serovar across cycles on both farms was S. Sofia (75 and 77% isolates) followed by S. Typhimurium (11 and 17%). Irrespective of initial serovars, Salmonella Sofia rapidly gained dominance and displaced 14 other serovars including S. Typhimurium on both farms. This study demonstrates that the litter practices are not the major driver of Salmonella prevalence in broiler farming, supporting the commercial re-use of bedding as a sustainable farming practice in Australia. The major contributor of Salmonella load in production is the Salmonella status of the incoming flock, indicating this is the key area to focus future control measures.
Introduction
The United States Centres for Disease Control (CDC) has listed Salmonella (non-typhoidal origin) among the top five organisms linked to domestically acquired food-borne illness with 1,027,561 estimated cases in 2011 (Centres for Disease Control and Prevention, 2011). In Australia, the number of notifications of salmonellosis for 2013 was 12,791 cases (NNDSS Annual Report Writing Group, 2015) though not all these cases are of poultry origin (Andino and Hanning, 2015). The intestines of both humans and farmed animals (including poultry) are the main niche for Salmonella (Jones, 2011). Feed, soil, bedding, litter, feces (Andino and Hanning, 2015), and other vectors (Marin et al., 2009; Wales et al., 2010) all can be common sources of Salmonella on-farm. A direct relationship between the Salmonella levels in on-farm litter (used poultry bedding) and the processed product (carcass rinses) has been demonstrated (Berghaus et al., 2013).
Epidemiological links between poultry meat and human illness have been demonstrated for a small number of Salmonella serovars (among the 2,500) which are endemic in poultry (Gast, 2007). In the EU in 2019, S. Enteritidis (50.3%), S. Typhimurium (11.9%), and monophasic S. Typhimurium (1,4,[5],12:i:-) (8.2%) were responsible for 70% of the human illness [European Food Safety Authority (EFSA) and European Centre for Disease Prevention and Control (ECDC), 2021]. The EFSA has included S. Typhimurium as one of the serovars of concern in breeding flocks due to its occurrence in both broiler flocks and humans [EFSA Panel on Biological Hazards (EFSA BIOHAZ Panel), 2019]. Whilst others serovars do emerge, there is a need for close consideration of S. Typhimurium in poultry production.
Sustainable farming practices can have positive impacts on intensive industries such as broiler farming where bedding or wood shavings are renewable resources (Bolan et al., 2010). The type and extent of waste generated can vary from country to country; for example, 10.2 million tons of litter were generated in the USA annually (Dunkley et al., 2011). Similarly, in Australia, the estimated annual poultry production (2009–2010) of 500 million birds contributed to an estimated 1,216,000 tons/year of litter (McGahan et al., 2013) some of which is re-used. There are advantages in re-using litter due challenges in sourcing quality bedding in an expanding industry, and the environmental impacts of disposing litter, though both attract food safety concerns. The possible impacts of farming practices as risk factors for Salmonella colonization of broilers has been reviewed by the European Food Safety Authority (EFSA) to address management options [EFSA Panel on Biological Hazards (EFSA BIOHAZ Panel), 2019].
Re-using litter is a common practice in Australia (Runge et al., 2007) with a dominant proportion (70%) using new bedding with litter re-use occurring in the rest under a partial litter reuse practice (Runge et al., 2007). Australia (specifically Queensland) has re-used litter for over 30 years, (Collaborators pers. comm.). Due to constraints in sourcing quality bedding the Australian partial litter re-use practice is set to grow in the future. During the Australian partial re-use practice, the litter at the end of the initial broiler cycle is piled, the shed undergoes cleaning, and the windrowed litter is spread over the back grow-out end of the shed. The front brooder end always receives fresh bedding where the young chicks are placed until around 14 days of age, separated from the grow-out end via a curtain. Following which they gain full access to the back of the shed which contains re-used litter. The process will repeat for 3–5 sequential cycles before a full clean-out of all litter occurs and the process restarts again (Some litter is often removed at the end of each cycle to prevent accumulation at the grow-out end) (Chinivasagam et al., 2012). Litter re-use is also common in the USA (Volkova et al., 2009) and both USA and Australia have a history of litter re-use. The success of litter re-use in the USA is suggested to be driven by the efficient treatment methods that occur during the downtime between consecutive flocks, such as de-caking, tilling, and windrowing (Bucher et al., 2020).
Australian studies (Chinivasagam, 2009) have looked at the litter pile-up that occurs between cycles on farms (2 and 3 sequential piles) and assessed pile locations (top, core, bottom) during the pile life. Salmonella die-off was found between 3 and 5 days of pile life across farms. Pile temperatures (35°-60°C; driven by pile locations) and high pH (8.5–9.0) along with pile litter water activity (Aw 0.83–1.00) could have played a contributory role (Chinivasagam, 2009). In vitro studies have shown increased thermal resistance in desiccation adapted Salmonella cells with potential to survive in aged litter (Chen et al., 2013) but this can be reversed by rehydration (Chen and Jiang, 2017). In-house windrowing of broiler litter as adopted in the USA was shown not to increase final Salmonella colonization (Brooks et al., 2015). Competitive exclusion, pH, and temperature influence both pathogen levels and the overall litter microbiome during the downtime between flocks (Bucher et al., 2020). The study found that the beta diversity of the microbiome was significantly affected by litter pH, ammonia, moisture, and water activity. Bacillus subtilis strains were also shown to influence in vitro Salmonella growth (Heidelberg) (Bucher et al., 2020).
A 2.5-year study following 11 flock rotations of a US poultry house that underwent a partial and a total clean-out, and the introduction of new bedding, showed minimal influence of practice on the established in-house litter microbial community (Crippen et al., 2021). It is reported that the continuous transfer of fecal matter can support competitive litter microflora re-establishment by end of the flock cycle, inhibiting pathogen proliferation (Brooks et al., 2015). The impact of the Australian litter re-use practice on Salmonella in the sheds end with new and re-used bedding suggested higher Salmonella levels and serovar diversity in the new bedding end, when assessed across a whole cycle (but no chickens were studied) (Chinivasagam et al., 2012).
Salmonella is widespread in the natural environment and can be isolated from humans or animals with some serovars host adapted and not having a major epidemiological significance (Murray, 1991). There is a close relationship between the Salmonella populations in chicken feces and litter (Santos et al., 2005) with litter Salmonella presence being a typical reflection of flock status at the time (Bhatia et al., 1979). The breeder flock can be the original source of Salmonella in commercial flock (Richardson et al., 2003; Kim et al., 2007). Re-using litter has always generated concerns of pathogens being transferred from the re-used litter environment to the new flock (Payne et al., 2007).
The Salmonella levels in older and built-up litter (cleaned out every 2–5 years) has been shown to be lower than when an annual clean-out of litter occurred, i.e., log <1.0 vs. log 3.6 Most Probable Number (MPN)/g, respectively, in 4–6 weeks old birds (winter) (Payne et al., 2006). Assessing the occurrence of Salmonella in Brazilian farms when litter was re-used up to 14 consecutive times resulted in a low presence of Salmonella i.e., only 2.5, 5.27, and 2.08%, respectively, over three consecutive years (2008–2010) (Roll et al., 2011). Older and built up litter can exhibit an inhibitory effect on Salmonella compared to new litter (Payne et al., 2006). Salmonella has been shown to die-off during the in-shed litter pile up between cycles that occurs as a part of Australian litter re-use practice (Chinivasagam, 2009).
It is possible that management practices, such as litter re-use, may only influence Salmonella prevalence and not the Salmonella serovars present at the time (Foley et al., 2011). From an overall perspective controlling Salmonella in integrated broiler operations can be complicated due to the varied sources of the organism (Kim et al., 2007). Studies such as those assessing the “presence/absence” of the organism on-farm can associate common characteristics (e.g., the presence of dusty and dry conditions in houses) and their potential link to increased Salmonella prevalence on-farm (Berghaus et al., 2012). However, Salmonella prevalence in one of several risk factors for addressing salmonellosis (Oscar, 2020). Thus, merely assessing prevalence does not reveal the impact of the various management practices adopted, housing options, or the environmental fate of Salmonella on-farm (Payne et al., 2006).
Given that Salmonella has a close link with the bird and its bedding, and the common belief amongst some farmers that “used bedding will carry higher Salmonella than the new” the main aim of this study was to adopt a quantitative approach comparing the bedding materials and birds simultaneously with three commercially adopted bedding practices. Any associations between bird and bedding leads to more informed risk management. The bedding options tested were the use of new bedding (Australian practice), partial re-use (Australian practice), and full litter re-use. The study was undertaken on two different farms during standard commercial farming cycles. Both Salmonella and Campylobacter (reported elsewhere) (Chinivasagam et al., 2016) were simultaneously studied. It was resource intensive and large but necessary to obtain outcomes applicable to commercial practice. It followed through six sequential broiler cycles lasting a year on each farm with in-shed litter management between cycles. A key aim of the study was to address food-safety (Salmonella levels and serovars) for sustainable broiler farming that incorporates litter re-use in the context of an expanding industry.
Materials and Methods
Farm Sampling
Animal ethics approval (SA 2008-10-265) was obtained for the entire experimental study from the Department of Agriculture and Fisheries (Queensland) Animal Ethics Committee. All farm sampling methods (including the three litter practices adopted) have been previously described for the Campylobacter component of this study (Chinivasagam et al., 2016). Briefly, three separate adjacent sheds were randomly selected to house three different litter practices on two commercially operated farms; the study design was validated via a shed effects study (data not presented). The farms (reasonably close to each other but with no road direct access and separated by forest) were situated in a dominant broiler farming region close to Brisbane, Queensland, Australia and were managed by the same integrator company and thus practices were the same on both farms. Farm 1 (F1), had four sheds (approximately 35,000 chickens per shed) and Farm 2 (F2), had eight sheds (approximately 40,000 chickens per shed). The trial coincided with a normal commercial farming cycle adopted for the rest of the sheds. Thus, other than setting up the three litter practices, all usual practices including diets, thin-out times and final removal for slaughter were determined by company policies and decisions.
During the initial cycle (Cy1) following a full cleanout, all sheds used new bedding. As the usual practice on both farms were the Australian practice of partial re-use, this ensured a starting point which allowed the setting-up of practices. The practices adopted for the study were (a) the use of new bedding for every cycle (an Australian practice), i.e., “new” (N), (b) the use of new bedding in the front end (brooder) of the shed and re-used bedding in the back end (grow-out) of the shed (an Australian practice), i.e., “partial” re-use (P), (c) the sequential re-use of the same bedding (an alternative practice) across the shed i.e., “full re-use” (F). During all practices, the young chicks were segregated via a curtain as previously described. Young chicks on placement on both N and P practices were raised on new bedding and F on full re-use bedding in the partitioned brooder end for around 14 days, until old enough for full shed access. Each cycle lasted around 49–51 days, followed by a turn-around period of about 5 days between cycles. Litter was managed between cycles during the Australian partial re-use practice (P) on F1 and F2 by piling as described in Chinivasagam (2009) and Chinivasagam et al. (2012). During full litter re-use, on F1, litter was heaped across entire length of shed for 5 days and spread and on F2, litter was aerated mechanically, clumps removed, and spread. Detailed litter management adopted within the three test sheds on F1 and F2 for this trial is listed in the Campylobacter component of the study (Chinivasagam et al., 2016).
Collection of Litter and Ceca Samples
During each cycle, both litter (L) and chicken ceca (C) were collected from the three sheds on both farms. The litter and ceca collection are described in the Campylobacter component of the study (Chinivasagam et al., 2016), and is elaborated here. Chickens and litter were sampled from three sheds during each cycle from F1 and F2 as follows: (a) around day 7 following placement—for litter only, (b) just prior to first pick-up (thinning), litter and chickens, and (c) just prior to final pick-up, litter and chickens, where pick-ups represented commercial pick-up days. Litter was collected as in (Chinivasagam et al., 2012) to a depth of 40 cm over an area of 400 cm2 using a stainless-steel sampler. Each shed was defined into four main sections i.e., two in the brooder area (front end 1 and 2) and two in the grow-out area (back-end 3 and 4). The sheds (100 m length) had 39 (F1) and 33 (F2) bays (structure supports along the shed) which were used as markers for routine random number allocation (to allow sample collection) across the shed, Supplementary Figure 1. Sample collection represented a selected bay location that then defined a selected drinker, feeder, or a shed center as sample collection spots (also selected via random number). Thus, initially, 16 bays were selected via random number allocation (i.e., four bays per main section). Within this selected section, eight spots were selected for litter sample collection from an area adjacent to a drinker and/or feeder and/or center of the shed. The eight litter samples were mixed and quartered to from a single composite sample, representative of the relevant section e.g., brooder 1. This was repeated for brooder 2, grow-out 1 and grow-out 2 to form four main samples per shed (from a total of 32 litter samples per shed) representing those four main sections. All compositing was done immediately on collection at the farm and samples stored chilled on ice bricks in chiller bins to maintain a temperature of 4°C. Two chickens were collected in the region of the bays selected for litter sampling resulting in eight chickens per section (e.g., brooder 1). In this manner a total of 32 chickens were collected to represent the four sections as previously described. The chickens were immediately aseptically dissected on collection at farm, the ceca suitably stored chilled as previously described. The litter and ceca were transported to the laboratory and litter was tested immediately on arrival and chickens within 22 h of collection.
Enumeration of Salmonella Levels in Litter and Ceca
The three tube MPN method was used to enumerate Salmonella levels (MPN/g) in both litter and ceca and is previously described (Chinivasagam et al., 2009, 2012). Briefly, 25 g of the composite samples of litter or ceca were weighed to which 225 ml of 0.1% buffered peptone water was added. The ceca were stomached (AES smasher lab blender) and litter blended (Bamix, stick bender (http://bamix.com.au) for 1 min each, then allowed to settle. Appropriate serial dilutions (0.1% buffered peptone) were inoculated into 10 ml of buffered peptone water and incubated at 37°C overnight. Six 30-μl aliquots from each incubated broth were inoculated on to a single MSRV (Oxoid CM0910, Basingstoke, UK) plate, followed by incubation at 42°C overnight. Presumptive positives from MSRV (motile zones) were streaked on both XLD (Oxoid, CM0469, Basingstoke, UK) and CHROMagar Salmonella Agar (Difco, 21492.5, Beckton Dickinson, United States) following overnight incubation at 37°C). Positives from XLD were subcultured onto nutrient agar (Oxoid, Basingstoke, UK), incubated overnight at 37°C, and further confirmed using Salmonella O antiserum, Poly A-I, and Vi (Difco, Beckton Dickinson, United States). Salmonella levels were expressed as MPN/g of litter or ceca. Each original sample (25 g in 225 ml) was also tested (for the presence/absence of Salmonella) as previously described and reported present (+) or absent (–) in 25 g in the event the MPN was below detection limit. Around 500–600 colonies were confirmed as Salmonella spp. from a single cycle across the three practices totalling approximately 3,000–3,600 isolates across the six cycles on a single farm leading to ~7,500 isolates on both farms over a 2-year period. These isolates were randomly chosen from plates used to enumerate Salmonella levels representing the highest to the lowest MPN dilution series to ensure diversity. From these confirmed isolates, an arbitrary subset of isolates from shed segments (e.g., N1, N2, N3, N4), litter practices (new, partial full re-use), cycles (1–6), and farms (F1, F2) were sent for serotyping as described below.
During each cycle, around 16 confirmed positive isolates were randomly selected from litter and ceca from each shed that housed a litter practice per sampling day totalling to ~50 colonies (less at times due to negatives) for the three sheds/sampling day. A total of 1,046 Salmonella isolates (513 from F1 and 533 from F2) across 34 of the 36 sampling dates (two day 7 on F2 were missed) were further streaked for purity on Nutrient agar (Oxoid) and stored. The isolates were then sent to the Salmonella Reference Laboratory, Institute of Medical and Veterinary Science, Adelaide, Australia (IMVS) for serotyping (and phage typing where relevant). The serovar identity is presented based the dilutions (lowest to highest to assess sequential dominance) they were picked from XLD comparing those form both litter and ceca across litter practices, cycles and farms over the 2-year period.
Statistical Analysis
Prior to commencement of the trial, sampling of surface litter to examine for shed effects was undertaken, comparing the three sheds on F1. Analysis of variance for water activity, moisture, pH, and temperature showed no notable impact of the shed environment (data not presented). The study sheds were found to be approximately equal, thus validating the study design adopted on F1 and F2 for the overall study (Chinivasagam et al., 2016).
Statistical analyses were carried out for Salmonella levels in litter and ceca, both overall and separately for the prior first thin-out and final pick-up for both farms. The time-series nature of the data was taken into account by an analysis of variance of repeated measures (Rowell and Walters, 1976), via the AREPMEASURES procedure of GenStat (2013).
Litter pH, Moisture, and Shed Conditions
In order to have a general understanding of the litter macro-environment across litter practices, litter pH and moisture content was measured as described in Chinivasagam et al. (2012). Water activity was tested (Decagon safe storage quick check, Decagon devices, USA), following sample collection in the shed by inserting the probe for about a minute into the sample bag (following air elimination) until a stable recording. Relative humidity (RH) was monitored at litter level by setting up a handheld weather station (Kestrel 4100 pocket airflow tracker, https://kestrelmeters.com.au/Australia) on a tripod at the mid-point in the shed, at approximately 1 m. height from the bottom of the shed at the commencement of sample collection in the shed.
Results
Salmonella Levels in Litter—Around Placement
The Salmonella levels in litter at the brooder ends with chicks (shed segments 1 and 2) and the chick-free grow-out end (shed segments 3 and 4) were tested at the start of each sequential cycle (i.e., around day 7; Table 1). On F1, at the commencement of the trial (Cy1) following a full clean-out of litter and new bedding incorporated to all sheds, Salmonella was not detected across all three sheds at the brooder end containing chicks, indicating an absence of Salmonella in the litter at placement. Salmonella was also not detected in the chick-free end which serves as an indicator of possible carry-over from the previous cycle. In contrast, on F2, during Cy1, Salmonella levels in the brooder end with chicks ranged from being detected (presence/absence) in 25 g to log 4.0 MPN/g across the three sheds, indicating a Salmonella positive flock, compared to being not detected in litter in the chick-free grow-out end. During the rest of the cycles (Cy2–Cy6) on both farms, when detected at the brooder ends in litter in the presence of chicks, Salmonella levels ranged from a minimum of log 0.7 MPN/g to a maximum of log 5.2 MPN/g. There were many instances of a Salmonella absence at the chick-free grow-out end irrespective of shed litter practice, indicating no carryover (Table 1). When detected at the chick-free grow-out end, on both farms, across cycles, Salmonella was either detected in 25/g (reported when MPN was below detection) or at lower levels (log 0.7–1.6 MPN/g compared to when chicks were present). Similarly, Salmonella was below detection/or at low levels (i.e., presence in 25/g to levels of 2.4 MPN/g) even in the presence of chicks in the brooder end with chickens (Cy 2 partial, full re-use, Cy3, all practices on F1, Cy 6 on F2, all practices) indicating low flock contribution of Salmonella to litter.
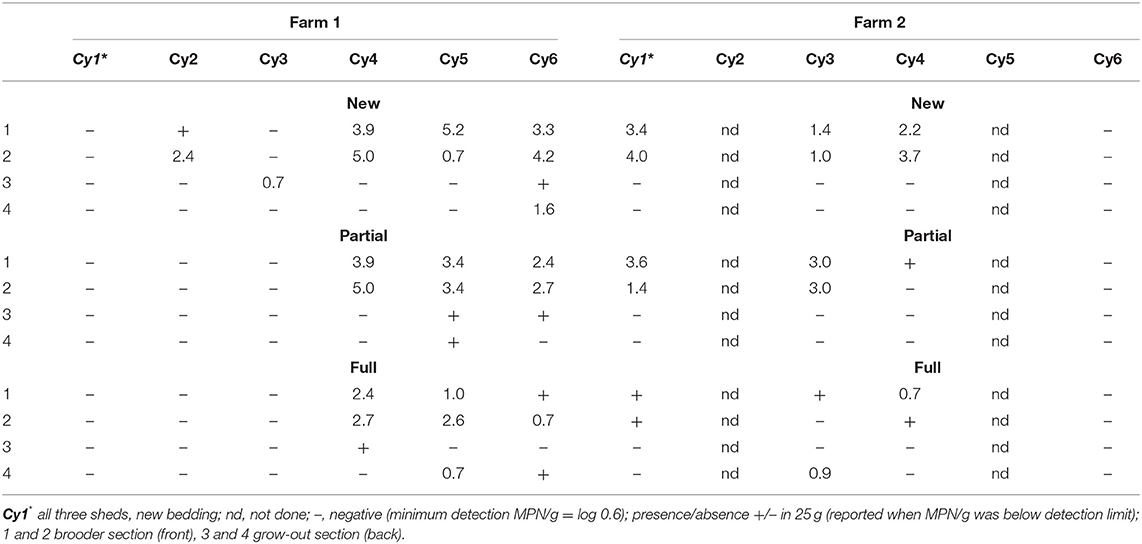
Table 1. Salmonella levels in litter (log MPN/g) on day 7 across brooder (segments 1, 2) and grow-out (segments 3, 4) ends of the sheds housing new, partial, and full re-use litter practices on both farms (F1 and F2), across cycles 1–6.
Summary Statistics, Practices, Cycles, and Farms (First and Final Pick-Up)
The Salmonella levels in ceca prior to thin-out were low (log 2.04–3.11 MPN/g) but were around a log lower (log 1.72–2.33 MPN/g) in mature birds just prior to final removal, irrespective of litter practice, thus varied with bird age Table 2. Whereas, the Salmonella levels in litter prior to first and final removals, was also low (log 1.96–2.48 MPN/g), irrespective of practice. In analyses across the six cycles on both farms Table 2, Salmonella levels in ceca just prior to thin-out on full re-use bedding were higher (log 3.11 MPN/g, P = 0.008) than for new (log 2.04 MPN/g) and partial re-use (log 2.43 MPN/g) bedding (the latter two were not significantly different). Prior to final removal across all the practices the Salmonella levels in ceca log 1.72 MPN/g, (new), log 1.77 MPN/g (partial), and to log 2.33 MPN/g (full re-use) were not statistically different, suggesting no litter practice driven influence. The Salmonella levels in litter prior both removals across practices (prior first log 1.96–2.31 MPN/g and prior second 2.24–2.48 MPN/g) were also not statistically different.
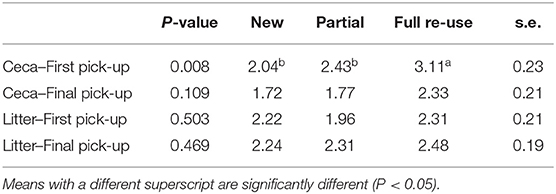
Table 2. Probability values (P-value), overall mean Salmonella levels for litter practices (MPN/g), and standard errors (s.e.) for each pick-up.
Salmonella Levels—Prior First Thin-Out and Final Pick-Up—Ceca and Litter
Two patterns of a ceca–litter relationship were apparent on F1and F2 across cycles prior to thin out and prior to final removal (Figure 1); In the first pattern, there was an absence of Salmonella in ceca and litter, irrespective of litter practice (highlighted orange arrows). This was observed on F1, Cy1 at trial commencement when all sheds had new bedding in two of the three sheds both prior thin-out and final removal and during Cy2 in the partial re-use shed. On F2 an absence in ceca and litter was apparent during Cy5 (partial) and Cy6 (partial and full re-use). The second pattern was an absence in ceca, but low Salmonella levels detected in litter [i.e., shed with new bedding, 0.7 MPN/g (F1 Cy2), prior to thin out]. A similar situation (i.e., absent in ceca, low levels in litter) occurred on F2 (new) during Cy6, first and final removals (highlighted with blue arrows in Figure 1).
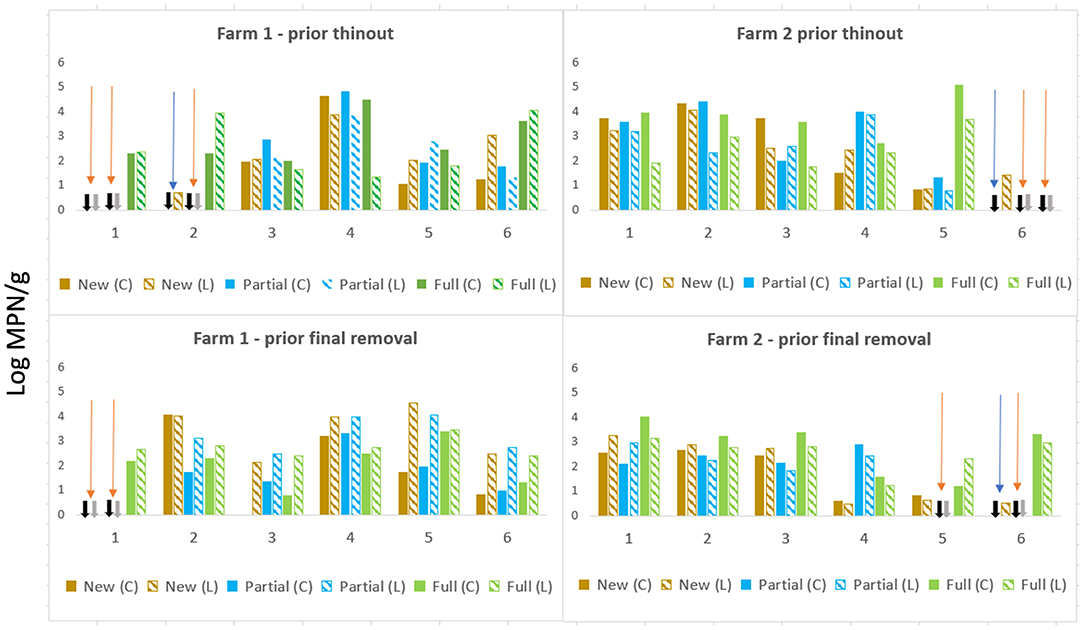
Figure 1. Salmonella levels ceca (log MPN/g) and litter (log MPN/g) prior to thin-out and final removal across new bedding, partial, and full re-use litter across sequential farming cycles on both, farms F1 and F2.
Salmonella Serovar Dynamics Across Litter Practices and Cycles
There was a total of 15 different serovars (including different phage types) detected on farms across the six cycles (Table 3). Despite the farms being geographically separated and studied a year apart, the serovar distribution on both farms was striking similar with Salmonella Sofia the dominant serovar in both litter and ceca (75% F1 and 77% F2). This was followed by S. Typhimurium PT135a (9.7% F1 and 16.7% F2). The rest of the serovars formed a minor component (14% on F1, 6% on F2). Those that formed the minor component were included S. Chester, S. Give var 15+, S. Montevideo, S. Ohio, S. Senftenberg, S. Tennessee, and S. Zanzibar (F1). S. Infantis, S. Virchow, S. Singapore, Salmonella sups1 ser 16:1v:-, and S. Taksony are detailed in Table 3.
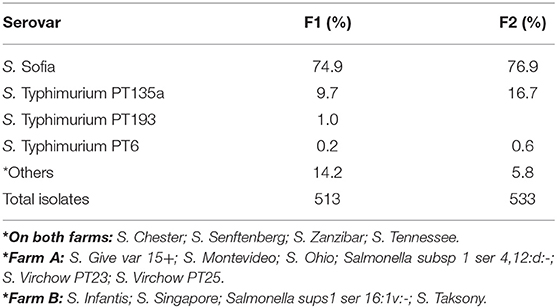
Table 3. Serovars isolated (%) from both litter and ceca from F1 and F2 from three litter practices across six sequential farming cycles.
Examination the serovars present in each cycle on F1 (Table 4) and F2 (Table 5) revealed the serovar present in ceca was also commonly isolated from litter. Salmonella Sofia was dominant across all 12 cycles with some serovars unique to cycles (Tables 4, 5). The detailed pattern of emergence of Salmonella serovars on F1 and F2 for selected cycles on day 7, prior to first and second removal, for new bedding (sections, N1, N2, N3, N4), partial (sections P1, P2, P3, P4), and full litter re-use (sections F1, F2, F3, F4) is shown in Figures 2–4.
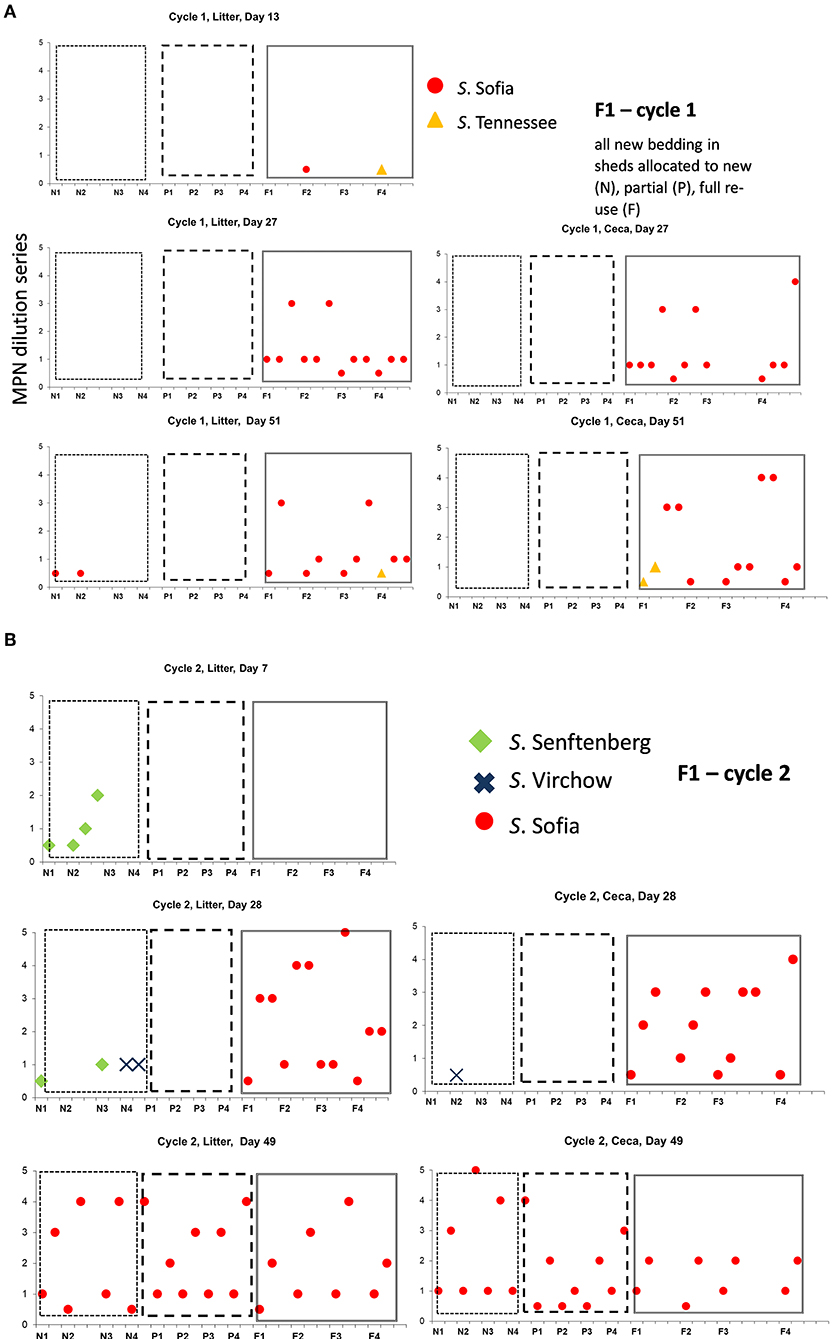
Figure 2. Distribution of Salmonella serovars for F1 cycles 1 (A) and 2 (B), day 7, first and second pick-up, across of new (sections, N1, N2, N3, N4), partial (sections P1, P2, P3, P4), and full re-use (sections F1, F2, F3, F4), based on highest and lowest 10-fold serial dilutions used for Salmonella (log MPN/g), litter, and ceca.
On F1 Cy 1 (Figure 2A) a minor contribution of S. Tennessee and S. Sofia at the chick-free grow-out end (a possible residue from a previous cycle) is observed. Subsequently S. Sofia begins to gradually dominate in both litter and ceca initially in one shed and then across all three sheds. Similarly, on F1, Cy2, S. Senftenberg and S. Virchow though not detected in Cy1 (Figure 2B) emerge only in the shed allocated to new bedding with S. Sofia initially dominant (litter and ceca) in the shed allocated to full reuse and continues to widely populate all three litter practices (both in ceca and litter).
The Salmonella dynamics during Cy1, F2 is interesting with S. Typhimurium spread widely in litter across the chick populated brooder ends (rather than the chick-free ends) of all three litter practices suggestive of flock contribution at placement (Figure 3A). This is the initial cycle, and all sheds are on new bedding. Prior to first removal there is a mix of both serovars in two sheds and only S. Sofia in the third shed, after which S. Sofia gains dominance across all three sheds prior final removal. Similarly, S. Sofia–S. Typhimurium dynamics was also apparent almost a year earlier during Cy4, F1 (Figure 3B), irrespective of the minor presence of S. Chester (full re-use ceca) and S. Tennessee (full re-use litter).
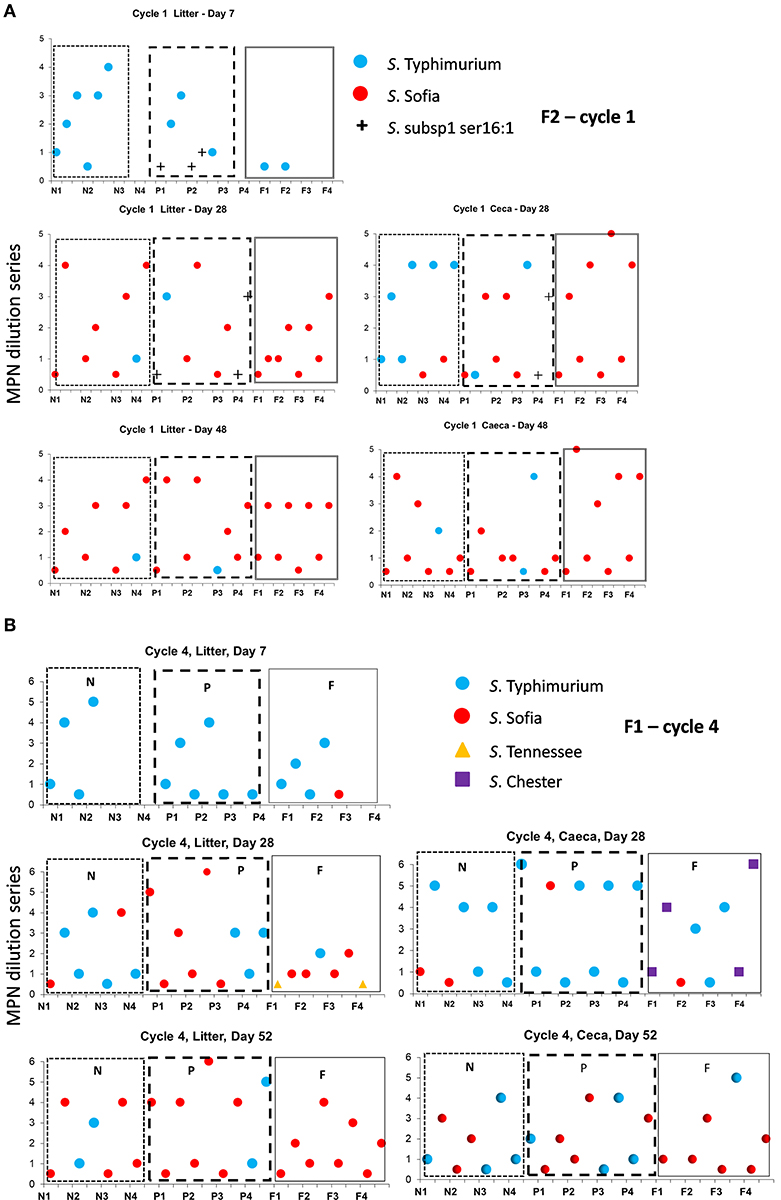
Figure 3. Distribution of Salmonella serovars for F2 cycle 1 (A) and F1 (B) cycle 4, day 7, prior to first and second pick-up, across of new (sections, N1, N2, N3, N4), partial (sections P1, P2, P3, P4), and full re-use (sections F1, F2, F3, F4), based on highest and lowest 10-fold serial dilutions used for Salmonella (log MPN/g) litter and ceca.
Salmonella Sofia also could proliferate in both ceca and litter across a cycle following minor emergence in a single shed (new bedding) irrespective of another serovar and the litter practice as seen in Cy3, F1 (Figure 4). Additional detailed data (such as presented here) for all 12 cycles in F1 and F2 are presented in Supplementary Figures 2, 3.
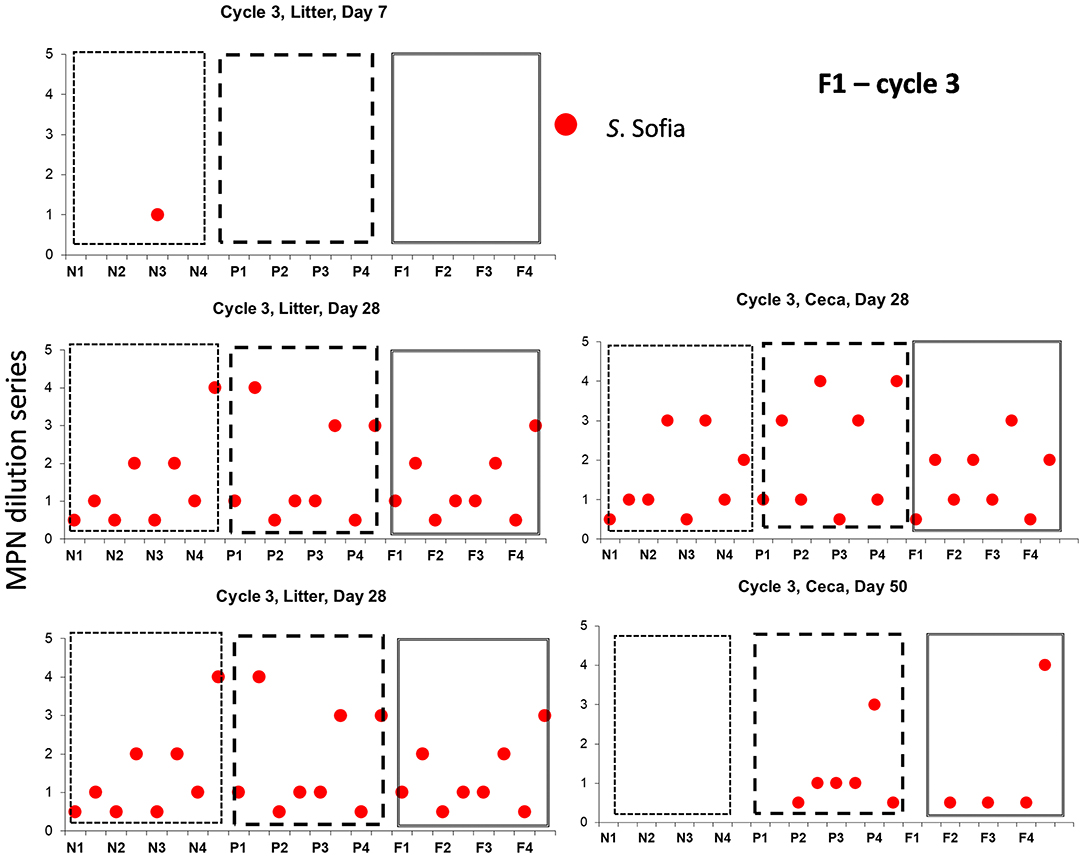
Figure 4. Distribution of Salmonella serovars for F1 cycle 3, day 7, prior to first and second pick-up, across of new (sections, N1, N2, N3, N4), partial (sections P1, P2, P3, P4), and full re-use (sections F1, F2, F3, F4), based on highest and lowest 10-fold serial dilutions used for Salmonella (log MPN /g), litter and ceca.
Litter pH, Moisture, and Litter Level RH
The litter pH for F1 (Chinivasagam et al., 2016) and F2 (Supplementary Figure 4) show no major differences in range prior to first pick-up (8.0–8.8) and prior to final removal (8.4–8.8) across practices. On F1, full re-use (which had more aged litter) the pH was significantly different (P < 0.05) in only five instances to new across both pick-ups and only a single instance, when comparing partial re-use with new (P < 0.05) (Chinivasagam et al., 2016). This demonstrates no major practice driven variations. The litter moisture (Supplementary Figure 5) on day 0 (with chicks) after a full clean-out (all new shavings) was ~15–16% (F1) did not vary markedly to the litter moisture of the chick- free grow-out area around day 7 after placement across practices (~15–20%, F1, F2). This point can be compared to the starting point of the moisture content of re-used bedding, at the commencement of a cycle. Just prior to first removal and final removal the litter moisture was generally in the same range (21.5–29%, F1, F2) with no major practice driven variations. Litter water activity on F1 (Supplementary Figure 6), in the presence of new shavings (no chicks) at day 0 ranged of Aw 0.70–0.79, which was not much variable across practices prior to first pick-up (Aw 0.74–0.84) and final removal 0.74–0.91 suggesting no major practice driven influence variations. The RH in the shed at litter level (F1) across the six cycles and practices (Supplementary Figure 7) ranged around 40–60% across practices. In summary, the litter macro environment was reasonably similar between litter practices.
Discussion
The current study is unique in that it adopted a multifaceted approach exploring sustainable commercial litter practices and Salmonella colonization dynamics in broilers. The adoption of a quantitative rather than a qualitative approach in Salmonella investigations has been shown to uncover unique facets of the organism's contribution on-farm (Lahellec et al., 1986). This study used quantitative methods to address Salmonella entry, extent in litter and ceca plus the emergence of 15 Salmonella serovars at various points tested through 12 sequential cycles on F1 and F2 across three litter practices. Li et al. (2021) using a quantitative approach, assessed the origins and dissemination of S. Enteritidis to breeding stock, demonstrating its value to risk management. The study provided an understanding of the origins of S. Typhimurium (a serovar of concern) and S. Sofia including their interactions in the presence the others, simultaneously in the bird and litter, which otherwise would not be possible. Identifying the relationship between on-farm Salmonella populations and environmental parameters together with adopted farm management practices can aid in informing on-farm pathogen control strategies, such as those adopted for HACCP (hazard analysis critical control point) (Payne et al., 2006) during food production. The current study provided a comprehensive understanding of potential critical control points than can facilitate informed decision making to address on-farm Salmonella in broiler production.
But in the current study there were no patterns of Salmonella emergence or levels driven by litter practice, statistically or any serovar influence connected to placement across cycles and farms. The Australian practice of placing the flock in the front of the shed (for ~14 days) and only spreading litter in the partitioned (chick-free) back of the shed presented a unique opportunity to assess the Salmonella status of litter used for each cycle. Around placement, amongst the 36 shed sections on F1 that defined the chick-free end (i.e., two sections/shed; three sheds/cycle; six cycles), only low Salmonella levels were detected (maximum of log 1.6 MPN/g) in eight sections. Similarly, on F2, of a total of 24 shed sections tested, Salmonella was only detected once at a low level (log 0.9 MPN/g).
In comparison, at the ends that received chickens at placement, the organism was more widespread across the shed (sections) with litter Salmonella levels reaching a maximum of log 5.2 MPN/g (F1) and log 3.7 MPN/g (F2), indicative of transfer via placed flock to litter. There were instances of low litter Salmonella levels (log 0.7–log 1.0 MPN/g; F1, F2), even with flock presence, which reflects that the Salmonella contribution from flock to litter levels can be variable. It has been suggested that native intestinal microorganisms (Lactobacilli) can contribute to colonization resistance in the ceca which may influence litter levels (Dunkley et al., 2009) in colonized flocks. Overall, the study outcomes suggest that the major contributor of Salmonella to emerging cycles are the incoming flock rather than possible residual carryover via litter.
Salmonella is closely associated with the chicken and has been shown to adhere to the mucosa of the ceca together with other cecal microflora (Soerjadi et al., 1981, 1982) in an anaerobic cecal environment (Barnes, 1972). Competing flora in the chicken gut can inhibit Salmonella via competitive exclusion (Nurmi and Rantala, 1973) by adhering to the sites Salmonella may occupy. Across the study, there were instances of an absence of Salmonella in ceca and a simultaneous absence in litter when assessed either prior thin-out only or prior both removals irrespective of litter practice and/or cycles. When detected in ceca either prior to thin-out or final removal the levels were low (log 1.72–3.1 MPN/g) with no statistical differences attributed to practices, cycles and farms. In comparison, Campylobacter levels (log 7.0–9.0 CFU/g) measured in the same ceca, detected simultaneously across the current study (reported elsewhere in Chinivasagam et al., 2016) were much higher (around 6 log CFU/g). Though, the levels of neither Salmonella nor Campylobacter (Chinivasagam et al., 2016) in the ceca were influenced by litter practices, cycles or farms.
Salmonella levels in the ceca were higher prior to thin-out (log 2.04–3.11 MPN/g) compared with prior to final removal (log 1.72–2.33 MPN/g). Bird age has been shown to influence Salmonella litter levels re-used bedding (Chinivasagam et al., 2012). In young chicks the diversity of microbial populations in the small intestine and ceca can change with bird age (Amit-Romach et al., 2004) influencing Salmonella colonization. Varying chicken diets across the bird's growth cycle may also contribute to nutrient availability which can influence Salmonella colonization of the gastrointestinal tract (Vandeplas et al., 2010). As per usual commercial practice the birds were subjected to a starter, grower, finisher, and withdrawal diets across their growth cycle, which may have influenced Salmonella colonization of the ceca.
Amongst the 1,046 Salmonella isolates selected for serotyping across the study, 15 serovars were isolated from F1 and F2, all associated with Australian broilers. Multiple phage types are reported for two serovars (S. Typhimurium, PT 135a, 193, 6; S. Virchow PT 23, 25). Across the 12 cycles, two serovars dominated (S. Sofia 75% at F1 and 77% at F2; S. Typhimurium 11% at F1 and 17% at F2). The rest were minor contributors but were key in providing an understanding of serovar emergence patterns across sequential cycles on F1 and F2 and thus the influence of used litter and ceca. The serovar isolated on the chicks' first day have been found to be the major contributor to serovars in the broiler house compared to those isolated later in the rearing period (Lahellec et al., 1986). This was apparent in the current study with the incoming flock the major contributor to the litter environment.
Salmonella serovar dynamics across the 12 cycles tended to follow three general patterns. The first pattern involved the emergence of a single or multiple serovars, generally at the chick-end of the shed at placement which were replaced by S. Sofia as the cycle progressed (e.g., S. Tennessee, Cy1 F1, new bedding, chick-free end); the serovars were not generally related to the previous cycle; The second pattern showed S. Typhimurium dominance at placement, predominantly in the flock-end and irrespective of litter practice followed by S. Sofia dominance as the cycle progresses (e.g., Cy1 F2 new, partial and full re-use; Cy 4, F1 new, partial and full re-use chick populated end). The third pattern was “S. Sofia only cycles,” shows a minor emergence of S. Sofia early, or widespread midcycle, which continued to gain dominance as the cycles progressed (e.g., Cy3 F1 re-use end; Cy6 F2 new).
Across the 12 cycles, S. Sofia (in ceca and litter) generally dominated mid-cycle rather than, as seen on few occasions, around placement. On F2, cycles 1 and 2 indicated a follow on of S. Typhimurium from Cy1 to Cy2, where litter may have played a role. But unfortunately, Cy 2 litter was not tested due to unforeseen operational circumstances at placement. Irrespective of origin, the S. Sofia–S. Typhimurium replacement was apparent across both cycles. Given the importance of S. Typhimurium from a regulatory context, the S. Sofia–S. Typhimurium dynamics observed in Australian poultry is intriguing.
The EU Salmonella food safety criteria prescribes Salmonella be not detected in 25 or 10 g of products as per the sampling criteria. In addition, as per Regulation (EC) No 1086/2011 the target serovars, one of which is S. Typhimurium (including monophasic S. Typhimurium), be not detected in 25 g in fresh poultry meat [European Food Safety Authority (EFSA) and European Centre for Disease Prevention and Control (ECDC), 2021]. It is unknown what triggers S. Sofia emergence as there can be S. Sofia free cycles. Collaborators (pers. comm.) have indicated to the author that S. Sofia is not associated with flock, unlike the other serovars. Given the interest on S. Sofia's ability to proliferate the chicken, experimental studies have previously explored the potential of using S. Sofia to decrease the level of S. Typhimurium colonization in chickens (Heuzenroeder et al., 2004). The current study, using a quantitative approach, has demonstrated the potential for S. Sofia to naturally replace S. Typhimurium (and other serovars) due to an ability to rapidly gain dominance on exposure to the chicken (ceca).
This brings the question as to the origins of S. Sofia in the chicken production environment. Salmonella Sofia was first isolated in chickens in 1980s (Harrington et al., 1991) and rapidly spread across the broiler industry, though rarely isolated from layers (Murray, 1994). It is of low virulence to humans but has good ability to colonize the broiler gut (Heuzenroeder et al., 2001). In the United States, S. Kentucky, a dominant serovar is also a prolific colonizer in chicken ceca but rarely linked with human illness and more specifically food-borne illness of chicken origin (Foley et al., 2011). Both serovars do present some similarity in this context. A prior study (Chinivasagam et al., 2012) assessing the “dual litter environment” of the Australian litter re-use practice tracked S. Sofia's initial emergence to the re-use end (back) of the shed following on to the front end (i.e., at days 25, 14 re-used end vs. days 31, 22 new bedding ends, respectively), but with chicken presence at a later stage when the birds were no longer segregated and had access to the re-use end. The isolation of S. Sofia across the two farms as the dominant serovar is not uncommon. Salmonella Sofia was also the dominant serovar (70%) in both new and re-used litter in a survey of three major Australian states along with S. Virchow, S. Chester (10%) S. Bovismorbificans, and S. Infantis (at 8%) demonstrating a wide distribution of this serovar in Australia (Chinivasagam et al., 2010).
In the present study, S. Sofia did also emerge in two instances only (across 36 shed samplings) at the new bedding end, but with chickens present. The emergence of another serovar, S. Taksony was detected in ceca from both ends of partial re-use shed (P1, P3) just prior to final flock removal (Cy3 F2). This serovar was reported in the 1970's when it was commonly isolated from animal feeds but has been rare in recent times (Australian Salmonella Reference Centre, 2011). The earlier study (Chinivasagam et al., 2010) may indicate that some serovars such as S. Taksony and S. Sofia may have a yet uncharacterized niche in the chicken production environment which leads to sporadic emergence, but no conclusive evidence was seen in the current study. It is notable that S. Sofia is a very good colonizer of broilers which suggests long-term adaptation to the host.
In the current study, the low Salmonella litter levels (i.e., log 1.96–2.48 MPN/g) prior to first and final removal reflected the low ceca levels (which were around one-log higher). The litter Salmonella levels across the study were not significantly different when assessed across litter practices, cycles and farms. Higher Salmonella levels (log 4–6 MPN/g) in re-used litter with the presence of chickens has been reported in a weekly assessment of the partial litter re-use practice (Chinivasagam et al., 2012). The Salmonella litter levels noted across both these studies suggests that levels are primarily driven by the extent of bird colonization. Therefore, flock Salmonella levels are a critical control point for in-shed litter management between re-use broiler cycles. Cecal Salmonella levels in broilers raised on litter were lower than those raised in cages (log 3.8 vs. 4.4 MPN/g) with litter mediated competitive exclusion possibly playing a role (Santos et al., 2008).
Aged litter has also been shown to be inhibitory to Salmonella (Olesiuk et al., 1971). Chickens are known to ingest litter (Hetland et al., 2003) and a significant consumption of litter among broilers from the shed floor has been demonstrated (Svihus et al., 2009). Studies on reused litter have also shown that at 14 and 28 days of chicken age the cecal microbiota of birds varied (Torok et al., 2009). Salmonella colonization resistance has also been linked to higher (P < 0.05) cecal concentrations of volatile fatty acids in chicks placed on used litter compared to those chicks on new litter (Corrier et al., 1992) which in turn can contribute to reducing Salmonella (Pivnick and Nurmi, 1982). The interactions between microbial communities including beneficial bacteria in shed environments can be modified by scheduling production practices and maximizing microbiome contributions to bird production and minimizing environmental impacts (Crippen et al., 2019). So, while litter re-use has been viewed by some farmers with concern, the current study shows there was no significant differences in Salmonella levels and serovars with reuse litter compared to the use of new bedding. These outcomes support sustainable litter re-use backed by sound and informed in-shed management between cycles.
In summary, this study shows that use of new or re-used bedding had no influence on Salmonella levels or serovars on-farm compared to the use of new bedding, when assessed across 12 sequential commercial cycles on two farms. The study done over 2 years thus demonstrates that the litter practices are not the major driver of Salmonella prevalence in broiler farming. These outcomes should lead to an increase in the sustainability of broiler farming due to the potential to re-use bedding. Managing incoming Salmonella loads via the placement flock on-farm can contribute to modifying Salmonella levels and serovars impacting end-product safety at processing. The major contributor of Salmonella load in production is the Salmonella status of the incoming flock, indicating this is the key area to focus future control measures. The outcomes of this study provide a framework for addressing on-farm Salmonella in poultry production.
Data Availability Statement
The original contributions presented in the study are included in the article/Supplementary Material, further inquiries can be directed to the corresponding author/s.
Ethics Statement
The animal study was reviewed and approved by Animal Ethics Approval (SA 2008-10-265) was obtained for the entire experimental study from the Department of Agriculture and Fisheries (Queensland) Animal Ethics Committee.
Author Contributions
HC with industry collaborators designed the on-farm trials, administered the project, curated the data, and wrote the original version. HC, WE, HR, CW, TT, and AO executed the farm trials and lab experiments. HC and DM analyzed the data. HC, CB, DM, and ID contributed to the final manuscript. All authors contributed to the article and approved the submission.
Funding
The financial support of the Rural Industries Research and Development Corporation (Agrifutures Australia) (RIRDC Project Number: PRJ-002798), Chicken Meat Program is gratefully acknowledged. The publication of this work was made possible by Queensland Department of Agriculture and Fisheries (DAF), Queensland, Australia.
Conflict of Interest
The authors declare that the research was conducted in the absence of any commercial or financial relationships that could be construed as a potential conflict of interest.
Publisher's Note
All claims expressed in this article are solely those of the authors and do not necessarily represent those of their affiliated organizations, or those of the publisher, the editors and the reviewers. Any product that may be evaluated in this article, or claim that may be made by its manufacturer, is not guaranteed or endorsed by the publisher.
Acknowledgments
We gratefully acknowledge Dr. Margaret MacKenzie for guidance, support, planning, and access to facilities. We gratefully acknowledge, Ms. Kelly McTavish for logistical support and executing of on-farm trials. We gratefully acknowledge both Mr. Guy Douglas and Mr. Geoff O'Meara for farm access and maintaining trial conditions for over a year each without which this trial would not have been possible.
Supplementary Material
The Supplementary Material for this article can be found online at: https://www.frontiersin.org/articles/10.3389/fsufs.2022.816181/full#supplementary-material
References
Amit-Romach, E., Sklan, D., and Uni, Z. (2004). Microflora ecology of the chicken intestine using 16S ribosomal DNA primers. Poult. Sci. 83, 1093–1098. doi: 10.1093/ps/83.7.1093
Andino, A., and Hanning, I. (2015). Salmonella enterica: survival, colonization, and virulence differences among serovars. ScientificWorldJournal. 2015, 520179. doi: 10.1155/2015/520179
Barnes, E. (1972). The Avian intestinal flora with particular reference to the possible ecological significance of the caecal anaerobic bacteria. Am. J. Clin. Nutr. 25, 1475–1479. doi: 10.1093/ajcn/25.12.1475
Berghaus, R. D., Mathis, D. L., Bramwell, R. K., Macklin, K. S., Wilson, J. L., Wineland, M. J., et al. (2012). Multilevel analysis of environmental Salmonella prevalences and management practices on 49 broiler breeder farms in four south-eastern states, USA. Zoonoses Public Health 59, 365–374. doi: 10.1111/j.1863-2378.2012.01464.x
Berghaus, R. D., Thayer, S. G., Law, B. F., Mild, R. M., Hofacre, C. L., and Singer, R. S. (2013). Enumeration of Salmonella and Campylobacter spp. in environmental farm samples and processing plant carcass rinses from commercial broiler chicken flocks. Appl. Environ. Microbiol. 79, 4106–4114. doi: 10.1128/AEM.00836-13
Bhatia, T. R., McNabb, G. D., Wyman, H., and Nayar, G. P. (1979). Salmonella isolation from litter as an indicator of flock infection and carcass contamination. Avian Dis. 23, 838–847. doi: 10.2307/1589599
Bolan, N. S., Szogi, A. A., Chuasavathi, T., Seshadri, B., Rothrock, M. J. Jr., and Panneerselvam, P. (2010). Uses and management of poultry litter. Worlds Poult. Sci. J. 66, 673–698. doi: 10.1017/S0043933910000656
Brooks, J. P., McLaughlin, M. R., Adeli, A., and Miles, D. M. (2015). Pathogen re-colonization of in-house composted and noncomposted broiler litter. J. Appl. Poult. Res. 24, 157–167. doi: 10.3382/japr/pfv013
Bucher, M. G., Zwirzitz, B., Oladeinde, A., Cook, K., Plymel, C., Zock, G., et al. (2020). Reused poultry litter microbiome with competitive exclusion potential against Salmonella Heidelberg. J. Environ. Qual. 49, 869–881. doi: 10.1002/jeq2.20081
Centres for Disease Control and Prevention (2011). CDC Estimates of Foodborne Illness in the United States.
Chen, Z., Diao, J., Dharmasena, M., Ionita, C., Jiang, X., and Rieck, J. (2013). Thermal inactivation of desiccation-adapted Salmonella spp. in aged chicken litter. Appl. Environ. Microbiol. 79, 7013–7020. doi: 10.1128/AEM.01969-13
Chen, Z., and Jiang, X. P. (2017). Thermal resistance and gene expression of both desiccation-adapted and rehydrated Salmonella enterica serovar typhimurium cells in aged broiler litter. Appl. Environ. Microbiol. 83, 17–17. doi: 10.1128/AEM.00367-17
Chinivasagam, H. N. (2009). Re-Use of Chicken Litter Across Broiler Cycles – Managing the Food-Borne Pathogen Risk, Final Report, Project No: 05-16, Poultry CRC, Australia. Available online at: http://www.poultryhub.org/wp-content/uploads/2012/07/Final-Report-05-16.pdf (accessed January 29, 2022).
Chinivasagam, H. N., Estella, W., Rodrigues, H., Mayer, D. G., Weyand, C., Tran, T., et al. (2016). On-farm Campylobacter and Escherichia coli in commercial broiler chickens: re-used bedding does not influence Campylobacter emergence and levels across sequential farming cycles. Poult. Sci. 95, 1105–1115. doi: 10.3382/ps/pew003
Chinivasagam, H. N., Redding, M., Runge, G., and Blackall, P. J. (2010). Presence and incidence of food-borne pathogens in Australian chicken litter. Br. Poult. Sci. 51, 311–318. doi: 10.1080/00071668.2010.499424
Chinivasagam, H. N., Tran, T., and Blackall, P. J. (2012). Impact of the Australian litter re-use practice on Salmonella in the broiler farming environment. Food Res. Int. 45, 891–896. doi: 10.1016/j.foodres.2011.06.014
Chinivasagam, H. N., Tran, T., Maddock, L., Gale, A., and Blackall, P. J. (2009). Mechanically ventilated broiler sheds: a possible source of aerosolized Salmonella, Campylobacter, and Escherichia coli. Appl. Environ. Microbiol. 75, 7417–7425. doi: 10.1128/AEM.01380-09
Corrier, D. E., Hinton, A. Jr., Hargis, B., and DeLoach, J. R. (1992). Effect of used litter from floor pens of adult broilers on Salmonella colonization of broiler chicks. Avian Dis. 36, 897–902. doi: 10.2307/1591548
Crippen, T. L., Sheffield, C. L., Singh, B., Byrd, J. A., and Beier, R. C. (2019). How management practices within a poultry house during successive flock rotations change the structure of the soil microbiome. Front. Microbiol. 10:2100. doi: 10.3389/fmicb.2019.02100
Crippen, T. L., Sheffield, C. L., Singh, B., Byrd, J. A., Beier, R. C., and Anderson, R. C. (2021). Poultry litter and the environment: microbial profile of litter during successive flock rotations and after spreading on pastureland. Sci. Total Environ. 780, 146413. doi: 10.1016/j.scitotenv.2021.146413
Dunkley, C. S., Cunningham, D. L., and Harris, G. H. (2011). The Value of Poultry Litter in South Georgia. Bulletin 1386. Available online at: http://www.caes.uga.edu/applications/publications/files/pdf/B%201386_1.PDF (accessed August 9, 2013).
Dunkley, K. D., Callaway, T. R., Chalova, V. I., McReynolds, J. L., Hume, M. E., Dunkley, C. S., et al. (2009). Foodborne Salmonella ecology in the avian gastrointestinal tract. Anaerobe 15, 26–35. doi: 10.1016/j.anaerobe.2008.05.007
EFSA Panel on Biological Hazards (EFSA BIOHAZ Panel) (2019). Salmonella control in poultry flocks and its public health impact. EFSA J. 17, e05596. doi: 10.2903/j.efsa.2019.5596
European Food Safety Authority (EFSA) and European Centre for Disease Prevention and Control (ECDC) (2021). The European Union One Health 2019 Zoonoses Report. EFSA J. 19, 6406. doi: 10.2903/j.efsa.2021.6406
Foley, S. L., Nayak, R., Hanning, I. B., Johnson, T. J., Han, J., and Ricke, S. C. (2011). Population dynamics of Salmonella enterica serotypes in commercial egg and poultry production. Appl. Environ. Microbiol. 77, 4273–4279. doi: 10.1128/AEM.00598-11
Gast, R. K. (2007). Serotype-specific and serotype-independent strategies for preharvest control of food-borne Salmonella in poultry. Avian Dis. 51, 817–828. doi: 10.1637/8090-081807.1
Harrington, C. S., Lanser, J. A., Manning, P. A., and Murray, C. J. (1991). Epidemiology of Salmonella sofia in Australia. Appl. Environ. Microbiol. 57, 223–227. doi: 10.1128/aem.57.1.223-227.1991
Hetland, H., Svihus, B., and Krogdahl, A. (2003). Effects of oat hulls and wood shavings on digestion in broilers and layers fed diets based on whole or ground wheat. Br. Poult. Sci. 44, 275–282. doi: 10.1080/0007166031000124595
Heuzenroeder, M. W., Murray, C. J., Dalcin, R. M., and Barton, M. (2001). Molecular Basis of Benign Colonisation of Salmonella Sofia in Chickens. Canberra, ACT: Rural Industries Research and Development Corporation.
Heuzenroeder, M. W., Murray, C. J., Davos, D., and Ross, I. L. (2004). Salmonella Typing and Colonisation of Chickens by Characterised Salmonella Sofia. Canberra, ACT: Rural Industries Research and Development Corporation.
Jones, F. T. (2011). A review of practical Salmonella control measures in animal feed. J. Appl. Poult. Res. 20, 102–113. doi: 10.3382/japr.2010-00281
Kim, A., Lee, Y. J., Kang, M. S., Kwag, S. I., and Cho, J. K. (2007). Dissemination and tracking of Salmonella spp. in integrated broiler operation. J. Vet. Sci. 8, 155–161. doi: 10.4142/jvs.2007.8.2.155
Lahellec, C., Colin, P., Bennejean, G., Paquin, J., Guillerm, A., and Debois, J. C. (1986). Influence of resident Salmonella on contamination of broiler flocks. Poult. Sci. 65, 2034–2039. doi: 10.3382/ps.0652034
Li, S., He, Y., Mann, D. A., and Deng, X. (2021). Global spread of Salmonella Enteritidis via centralized sourcing and international trade of poultry breeding stocks. Nat. Commun. 12, 5109. doi: 10.1038/s41467-021-25319-7
Marin, C., Hernandiz, A., and Lainez, M. (2009). Biofilm development capacity of Salmonella strains isolated in poultry risk factors and their resistance against disinfectants. Poult. Sci. 88, 424–431. doi: 10.3382/ps.2008-00241
McGahan, E., Barker, S., Poad, G., Wiedemann, S., and Batstone, D. (2013). Conversion of Waste to Energy in the Chicken Meat Industry. Rural Indutries Research and Development Corporation.
Murray, C. J. (1991). Salmonellae in the environment. Rev. Scient. Techn. Office Int. Epizoo. 10, 765–785. doi: 10.20506/rst.10.3.568
Murray, C. J. (1994). Salmonella serovars and phage types in humans and animals in Australia 1987–1992. Aust. Vet. J. 71, 78–81. doi: 10.1111/j.1751-0813.1994.tb03332.x
NNDSS Annual Report Writing Group (2015). Australia's Notifiable Disease Status, 2013. Annual Report of the National Disease Surveillance System. Communicable Diseases Intelligence 39.
Nurmi, E., and Rantala, M. (1973). New aspects of Salmonella infection in broiler production. Nature 241, 210–211 doi: 10.1038/241210a0
Olesiuk, O. M., Snoeyenbos, G. H., and Smyser, C. F. (1971). Inhibitory effect of used litter on Salmonella typhimurium transmission in the chicken. Avian Dis. 15, 118–124. doi: 10.2307/1588396
Oscar, T. (2020). Salmonella prevalence alone is not a good indicator of poultry food safety. Risk Analysis 41, 110–130. doi: 10.1111/risa.13563
Payne, J. B., Li, X., Santos, F. B. O., and Sheldon, B. W. (2006). Characterization of Salmonella from three commercial North Carolina broiler farms. Int. J. Poult. Sci. 5, 1102–1109. doi: 10.3923/ijps.2006.1102.1109
Payne, J. B., Osborne, J. A., Jenkins, P. K., and Sheldon, B. W. (2007). Modeling the growth and death kinetics of Salmonella in poultry litter as a function of pH and water activity. Poult. Sci. 86, 191–201. doi: 10.1093/ps/86.1.191
Pivnick, H., and Nurmi, E. (1982). “The Nurmi concept and its role in control of Salmonella in poultry,” in Developments in Food Microbiology, 3rd Edn., ed R. Davis (Applied Science Publishers), 41–70.
Richardson, L. J., Hofacre, C. L., Mitchell, B. W., and Wilson, J. L. (2003). Effect of electrostatic space charge on reduction of airborne transmission of Salmonella and other bacteria in broiler breeders in production and their progeny. Avian Dis. 47, 1352–1361. doi: 10.1637/7013
Roll, V. F. B., Dai Pra, M. A., and Roll, A. P. (2011). Research on Salmonella in broiler litter reused for up to 14 consecutive flocks. Poult. Sci. 90, 2257–2262. doi: 10.3382/ps.2011-01583
Rowell, J. G., and Walters, R. E. (1976). Analysing data with repeated observations on each experimental unit. J. Agric. Sci. 87, 423–432. doi: 10.1017/S0021859600027763
Runge, G. A., Blackall, P. J., and Casey, K. D. (2007). Chicken Litter Issues Associated with Sourcing and Use. Canberra, ACT: Rural Industries Research and Development Corporation.
Santos, F. B. O., Li, X., Payne, J. B., and Sheldon, B. W. (2005). Estimation of most probable number Salmonella populations on commercial North Carolina turkey farms. J. Appl. Poult. Res. 14, 700–708. doi: 10.1093/japr/14.4.700
Santos, F. B. O., Sheldon, B. W., Santos, A. A., and Ferket, P. R. (2008). Influence of housing system, grain type, and particle size on Salmonella colonization and shedding of broilers fed triticale or corn-soybean meal diets. Poult. Sci. 87, 405–420. doi: 10.3382/ps.2006-00417
Soerjadi, A. S., Rufner, R., Snoeyenbos, G. H., and Weinack, O. M. (1982). Adherence of salmonellae and native gut microflora to the gastrointestinal mucosa of chicks. Avian Dis. 26, 576–584. doi: 10.2307/1589904
Soerjadi, A. S., Stehman, S. M., Snoeyenbos, G. H., Weinack, O. M., and Smyser, C. F. (1981). The influence of Lactobacilli on the competitive exclusion of paratyphoid salmonellae in chickens. Avian Dis. 25, 1027–1033. doi: 10.2307/1590078
Svihus, B., Hetland, H., Mikkelsen, L., and Wu, S. (2009). Role of Voluntary Litter Consumption by Broiler Chickens on Gut Function and Gut Health. Final Report to the Poultry CRC.
Torok, V. A., Hughes, R. J., Ophel-Keller, K., Ali, M., and MacAlpine, R. (2009). Influence of different litter materials on cecal microbiota colonization in broiler chickens. Poult. Sci. 88, 2474–2481. doi: 10.3382/ps.2008-00381
Vandeplas, S., Dubois Dauphin, R., Beckers, Y., Thonart, P., and Thewis, A. (2010). Salmonella in chicken: current and developing strategies to reduce contamination at farm level. J. Food Prot. 73, 774–785. doi: 10.4315/0362-028X-73.4.774
Volkova, V. V., Bailey, R. H., and Wills, R. W. (2009). Salmonella in broiler litter and properties of soil at farm location. PLoS ONE 4, e6403. doi: 10.1371/journal.pone.0006403
Wales, A. D., Carrique-Mas, J. J., Rankin, M., Bell, B., Thind, B. B., and Davies, R. H. (2010). Review of the carriage of zoonotic bacteria by arthropods, with special reference to Salmonella in mites, flies and litter beetles. Zoonoses Public Health 57, 299–314. doi: 10.1111/j.1863-2378.2008.01222.x
Keywords: Salmonella, broilers, litter, ceca, re-use, management, farms
Citation: Chinivasagam HN, Estella W, Rodrigues H, Mayer DG, Tran T, Onysk A, Weyand C, Diallo I and Billington C (2022) Re-used or New Bedding Are Not Drivers of Salmonella Levels and Serovar Emergence in Commercially Farmed Broilers in Australia. Front. Sustain. Food Syst. 6:816181. doi: 10.3389/fsufs.2022.816181
Received: 16 November 2021; Accepted: 17 January 2022;
Published: 23 February 2022.
Edited by:
Anne Tremier, Irstea - Centre de Rennes, FranceReviewed by:
Keith Warriner, University of Guelph, CanadaAgnes Kilonzo-Nthenge, Tennessee State University, United States
Copyright © 2022 Billington, and The State of Queensland (through the Department of Agriculture and Fisheries) for the contribution of Chinivasagam, Estella, Rodrigues, Mayer, Tran, Onysk, Weyand and Diallo. This is an open-access article distributed under the terms of the Creative Commons Attribution License (CC BY). The use, distribution or reproduction in other forums is permitted, provided the original author(s) and the copyright owner(s) are credited and that the original publication in this journal is cited, in accordance with accepted academic practice. No use, distribution or reproduction is permitted which does not comply with these terms.
*Correspondence: Helene Nalini Chinivasagam, bmFsaW5pLmNoaW5pdmFzYWdhbUBkYWYucWxkLmdvdi5hdQ==; bmFsaW5pY0BtZS5jb20=