- 1Point Blue Conservation Science, Petaluma, CA, United States
- 2TomKat Ranch, Pescadero, CA, United States
- 3Regenerative Land Solutions, Emigrant, MT, United States
There is increasing interest in using biostimulant products, such as microbial inoculants and alkali-extracted “humic” substances to help manage rangelands regeneratively and rebuild soil health. Understanding how plant and soil communities on rangelands respond to these products is therefore important. In this 3-year study, we examined the combined effects of a commercial inoculant and alkali-extracted “humic” product that are currently on the market (Earthfort Inc. Soil Provide and Revive®) and asked whether they influenced rangeland forage productivity and quality, soil microbial biomass and community composition, and abiotic soil parameters in Central Coastal California. Treatments were established in February 2018 and the products were applied two to three times a year during the growing season (approximately November—May). Sampling of plant and soil samples also began in February 2018 and continued in the fall and spring for three consecutive growing seasons. We found that forage productivity responded positively to the foliar application of these commercial products, with forage production on average 58% percent higher in treated compared to control sites. Some metrics of forage quality (acid detergent fiber, calcium, and fat content) also responded in a desirable way, but these benefits were not mirrored by changes belowground in the microbial community or abiotic parameters. While our study derives from one ranch and therefore requires confirmation of its ubiquity prior to broadscale adoption, our results provide new insights into the usefulness of this approach for managing rangeland productivity in California's Central Coast—and suggest biostimulants could warrant attention as a potential tool for regenerative stewardship of rangelands more broadly.
Introduction
Regenerative agriculture aims to restore soil health and biodiversity, sequester carbon from the atmosphere, maximize water and nutrient use efficiency, and provide ample and nutritious food while reducing reliance on inputs from chemical fertilizers and pesticides (Newton et al., 2020). Management strategies used by those practicing regenerative agriculture are as varied as the production systems themselves, but tend to include practices that minimize soil disturbance, maximize crop diversity, keep the soil covered, maintain living roots, and integrate livestock (Lal, 2020). The use of microbial inoculants and alkali-extracted “humic” products1 are receiving increased attention as a potential tool for regenerative management both of croplands and rangelands, because of their expected ability to act as biostimulants and enhance yield, crop quality, and soil conditions while simultaneously reducing the need for chemical inputs (Adesemoye et al., 2009; Calvo et al., 2014; Arora et al., 2020).
The expected benefits of microbial inoculants and alkali-extracted “humic” products are supported in part by decades of research on their ability to improve crop productivity and quality (reviewed in Hart and Forsythe, 2012; Kong et al., 2018; Jindo et al., 2020). For example, research has shown that microbial inoculants have the potential to improve nutrient availability and plant uptake through the addition of specific microbial taxa with, for example, capabilities to fix atmospheric nitrogen (N2), solubilize phosphorus, or stimulate root growth and elongation (Hamdali et al., 2008; Halpern et al., 2015; Chamizo et al., 2018). Inoculation with putative plant growth promoting (PGP) microorganisms can also alleviate plant environmental stress and prevent infections by phytopathogens through the synthesis of targeted enzymes, signaling molecules, and other compounds such as siderophores (reviewed in Arora et al., 2020; Khatoon et al., 2020).
Like microbial inoculants, alkali-extracted “humic” products have been shown to improve crop productivity and quality (Rose et al., 2014) through a number of proposed mechanisms, which have been reviewed extensively elsewhere (Jindo et al., 2020). These mechanisms include improved nutrient availability, particularly of iron, through increased solubility and mobility in the soil (Nardi et al., 2002; Halpern et al., 2015), as well as enhanced plant uptake of nutrients through root growth (Nardi et al., 2002; Shah et al., 2018). The ability of plants to withstand stressful conditions is also aided by alkali-extracted “humic” products and their effects on secondary metabolism of specialized plant compounds such as auxins (Del Buono, 2021).
Despite promising—albeit equivocal (Hartz and Bottoms, 2010; Haider et al., 2015)—evidence from the literature, the reliability of microbial inoculants and alkali-extracted “humic” products to consistently achieve desired outcomes in agricultural settings remains in question (Hart et al., 2018; Olk et al., 2018). In part, this is because the efficacy of microbial inoculants has been shown to depend on the source (i.e., commercial or homemade), content (i.e., the community members present), and concentration of the product (Maltz and Treseder, 2015). Moreover, benefits of inoculation may be negated if some or all of the introduced microorganisms accidentally shift the soil community in undesirable ways and result in unintended ecological consequences (Hart et al., 2018; Kaminsky et al., 2019). The effects of alkali-extracted “humic” products will also depend on the source, concentration, and molecular weight of the product material—in addition to the mode of application (Jindo et al., 2020). To complicate the picture further, characteristics of the recipient ecosystem have been shown to mediate the outcome of product application as well (Nardi et al., 2002; Olk et al., 2018, 2021).
As the chemical and biological mechanisms for efficacy continue to be researched (Mao et al., 2013), field studies that aim to evaluate commercial inoculants and alkali-extracted “humic” products under operational conditions can help to disentangle these complex interactions from an applied perspective (Mayer et al., 2010; Olk et al., 2013). This is particularly needed in rangelands, where the use of such products is nascent but rapidly emerging as ranchers, policy-makers, and scientists alike look for new and alternative management practices that help promote outcomes related to forage production, climate stability, and soil health in the face of drought and other environmental threats (Godde et al., 2020). Indeed, while some studies have isolated the effects of “humic” products on focal forage species such as Sorghum bicolor L. (Verlinden et al., 2009; Churkova, 2013; Khaleda et al., 2017) and others have looked at the ability for microbial inoculants to help restore rangeland plant communities (e.g., Perkins and Hatfield, 2016; Koziol and Bever, 2017), there is still much to learn about the ability of commercial products to achieve multifaceted forage production and soil health goals on rangelands. Assessing the combined effects of microbial inoculants and alkali-extracted “humic” products, in particular, could prove useful, as evidence suggests these products can act in tandem to amplify the benefits of application (reviewed in Dos Santos et al., 2021).
The goal of this study was to evaluate the combined effects of a commercially available microbial inoculant and alkali-extracted “humic” product on rangeland dynamics in Central Coastal California. The products were applied via foliar spray because this has been demonstrated to be an effective and efficient mode of application (Wu et al., 2013; Justi et al., 2019), especially for non-irrigated lands. While the detailed composition of these products is proprietary and thus not available to the public, the microbial inoculant is described by the manufacturer as containing “a stable solution of extracted beneficial microbes” that include Bacillus subtilis, Mucor heimalis, and Trichoderma harzianum. The alkali-extracted “humic” product is described as a “water-soluble powder that is composed of humic acid derived from Leonardite, kelp, complex carbohydrates, and amino acids.” The specific objectives of our study were to track the effects of these products on (1) forage productivity, (2) forage quality, (3) soil microbial parameters associated with biomass and community structure (composition and diversity), and (4) abiotic soil properties during three consecutive years of application.
Materials and Methods
Study Site and Experimental Design
This study was conducted at TomKat Ranch (https://tomkatranch.org/), a 728 hectare property located in Pescadero, California, USA (37.261428, −122.360451). TomKat Ranch runs an organic grass-fed/grass-finished cow-calf operation using a planned grazing approach (Henneman et al., 2014). Pastures do not receive any exogenous inputs via irrigation or inorganic fertilizer. The climate is maritime Mediterranean-type characterized by mild relatively dry summers and cool wet winters. Mean annual air temperature is 12.9°C and mean annual precipitation is 750 mm. During the 2017–18, 2018–19, and 2019–20 water years (July—June), cumulative rainfall totaled 491, 877, and 366 mm, respectively (Appendix Figure 1 in Supplementary Material). The ranch contains ~324 hectares of grassland dominated by exotic annual grasses (e.g., Bromus spp.; Brachypodium spp; Avena spp) with some less-abundant native and non-native perennial grass species (primarily Stipa pulchra, Danthonia californica, Phalaris aquatica) and forbs. There are 11 soil series encompassed within the ranch boundaries, all of which derive from sedimentary rock, including mudstone, sandstone, and shale. The dominant soil series are Colma, Pomponio, Diablo, and Santa Lucia, with soil texture predominantly clay loam (sand = 15–60% [median 28.8%], silt = 16–45% [median 36.2%], clay = 18–54% [median 33.8%]).
Treatment and control plots (0.4 hectare) were established in a paired design, with one control and one treatment plot in each of 10 fields. Near the center of each 0.4-ha plot, a 10 x 10 m soil sampling area was delineated and, adjacent to the sampling area, a grazing exclosure was erected (0.8 m radius; Appendix Figure 2 in Supplementary Material). Prior to each growing season, the grazing exclosures were moved to a new adjacent area to minimize any effects that may accrue from prolonged exclusion. Careful site selection ensured that ecosystem characteristics such as slope, aspect, and vegetation type remained consistent between the 10 × 10 m sampling plots and exclosures for each field, but not necessarily across fields.
Between February 20 and 24, 2018, we established the treatments by applying Soil Provide® (Earthfort Inc., Corvallis, CO, USA), a commercially available microbial inoculant, and Soil Revive® (Earthfort Inc., Corvallis, CO, USA), a commercially available alkali-extracted “humic” product to each treatment plot. The Revive mixture contained 6% “humic acid” derived from leonardite, and nutrients derived from Ascophyllum nodosum and potassium hydroxide in the following concentrations: 0.5% total nitrogen, 0.5 % available phosphate (P2O5), and 4% soluble potash (K2O). The initial application rate in February 2018 varied across fields to mimic real-world recommendations made to farmers and ranchers by the manufacturer (Appendix Table 1 in Supplementary Material). After the initial application, we applied the “humic” product and microbial inoculant to all 10 treatment plots at a constant rate of 1.12 kg per hectare and 9.35 liters per hectare, respectively, two to three times during each growing season (Appendix Figure 3 in Supplementary Material). Products were suspended and applied in 280 liters of water per hectare; however, given the intention of the experiment was to test overall treatment effects (which inherently includes water as a part of it), and given this is a minimal amount of water compared to background levels of fog deposition (Hiatt et al., 2012), we did not add water to the controls. All treatments were applied using a spray tank with a constant spray rate and 3.7 m wide swath.
Aboveground Plant Biomass and Forage Quality
Plant Biomass
We collected plant metrics near the time of peak biomass across three growing seasons to determine treatment effects (Appendix Figure 3 in Supplementary Material). In May 2018, 2019, and 2020, we measured aboveground plant biomass. Briefly, vegetation clippings were taken to the soil surface from within a 16.5 cm diameter ring at the center of each grazing exclosure. The biomass samples were then air dried for 3 days and oven dried at 60°C to constant mass before weighing. Due to COVID-19 processing complications, we were unable to obtain reliable results for the 2020 plant biomass estimates and therefore excluded them from subsequent analysis.
Forage Quality
In May 2018, 2019, and 2020, we also assessed metrics of forage quality. To do so, four randomly-selected vegetation clippings per treatment were taken from just outside the soil sampling area, composited, and shipped to Ward Laboratories (Kearney, NE, USA). Because they were randomly selected, clippings encompassed numerous and variable plant species. At Ward Laboratories, the samples were analyzed via near infrared reflectance spectrometry (NIRS; Weiss and Hall, 2020). Metrics of forage quality included those related to digestibility (acid detergent fiber [ADF], neutral detergent fiber [NDF], lignin), protein, fat, and mineral content (crude protein, crude fat, potassium [K+], calcium [Ca2+], magnesium [Mg2+], and phosphorus [P]). Raw results are reported on a % dry matter basis.
Microbial and Abiotic Soil Properties
We collected soils across the same three growing seasons to determine belowground treatment effects (Appendix Figure 3 in Supplementary Material), although not all analyses were conducted at every time point. Specifically, we collected soils in early February 2018 (just prior to treatment application) and on five subsequent dates in May 2018, November 2018, May 2019, December 2019, and May 2020. Fall sampling dates were timed to occur after the first rains. Each treatment had 10 replicates (n = 10), where one replicate was a composite of 10 soil cores (1.9 cm diameter × 10 cm deep) taken from random locations within the 10 × 10 m sampling area. This sampling strategy provided us with a good estimate of the mean soil properties of the sampling area, which was our unit of replication. Composited soil samples were mixed, stored, and shipped for analysis as described below.
Microbial Biomass
At each collection date, we subsampled and labeled soils using a treatment-anonymous labeling scheme, and shipped them to Earthfort Inc. (Corvallis, OR, USA) for analysis of active and total microbial biomass using direct enumeration, which allows for the separate quantification of bacteria, fungi, and protozoa. Soil subsamples were stored at 4°C until shipment, which occurred within 24 h of collection. For all sampling dates, total and active bacteria were estimated by direct enumeration using fluorescein isothiocyanate (FITC; Babiuk and Paul, 1970) and fluorescein diacetate (FDA; Ingham and Klein, 1984) methods, respectively. Total and active fungi were calculated by measuring the diameter and length of hyphae (Lodge and Ingham, 1991), with the active fungi method also using FDA. In December of 2019 and May of 2020, subgroups of protozoa (flagellates, amoeba, ciliates) were also enumerated by direct counting of serial dilutions. The subgroups are based on body type and method of movement, and the direct counts were used to estimate population sizes for each subgroup using the most probable number approach (Darbyshire et al., 1974).
Microbial Community Structure
To determine treatment effects on soil bacterial/archaeal and fungal community structure, soil samples from February 2018, May 2018, and November 2018 were analyzed using DNA amplicon sequencing. Subsamples were frozen at −20°C prior to being shipped overnight on dry ice to Argonne National Laboratory's Environmental Sample Preparation and Sequencing Facility (Lemont, IL, USA). Samples were processed following current Earth Microbiome Project protocols (Gilbert et al., 2010; https://earthmicrobiome.org/protocols-and-standards/). Specifically, microbial DNA was extracted as in Marotz et al. (2018) and amplicon libraries were prepared for the V4 region of the 16S rRNA gene (forward barcoded 515f-806r primer pair; Walters et al., 2016) and internal transcribed spacer (ITS1) region (reverse barcoded ITS1f-ITS2 primer pair; Smith and Peay, 2014). Amplicons were subsequently sequenced on an Illumina MiSeq PE 2x150 run (16S rRNA) and a separate Illumina MiSeq PE 2x250 run (ITS1 region).
Abiotic Soil Properties
In May 2020, soil subsamples were stored at 4°C and shipped to Ward Laboratories within 24 h of collection for analysis of abiotic soil properties. Specifically, organic matter content was measured using loss on ignition (LOI), soil pH was measured on a 1:1 (w/v) soil to water suspension, pools of nitrate () were measured by 2M KCl extraction, and base cations (K+, Ca2+, Mg2+, and Na+) were measured via ammonium acetate extraction (Robertson et al., 1999; Ward, 2020).
Microbial Bioinformatics
Except where stated otherwise, all DNA sequences were bioinformatically processed using Quantitative Insights into Microbial Ecology 2 (QIIME2; Bolyen et al., 2019). Forward and reverse bacterial/archaeal (16S rRNA) sequences were demultiplexed using the q2-demux plugin then quality filtered, merged, and de-replicated using the q2-dada2 plugin. Q2-dada2 also removes PhiX and chimeras before producing sequence clusters with 100% similarity known as amplicon sequence variants (ASV). After quality control, including removal of singletons, taxonomy was assigned to ASVs using the q2-feature-classifier (Bokulich et al., 2018) with the classify-sklearn method against the Silva reference database (Quast et al., 2013).
Fungal (ITS1) sequences were processed using forward reads only, and the ITS1 region was extracted using itsxpress (Rivers et al., 2018) after demultiplexing with the q2-demux plugin. The sequences were then denoised using the q2-dada2 plugin as described above. Singletons were removed along with ASVs that could not be identified to the Kingdom Fungi and those with no BLAST hits. Taxonomy was assigned to ASVs using the q2-feature-classifier with the classify-sklearn method against the UNITE ITS reference database (Nilsson et al., 2019). All raw 16S and ITS sequences were deposited to the NCBI Sequence Read Archive under BioProject PRJNA808421.
Statistical Analysis
To assess treatment effects on plant biomass, metrics of forage quality, microbial biomass, and abiotic soil properties, we used mean effect sizes and their 95% confidence intervals (CI). An effect size for each paired treated and untreated site within a field was calculated using the natural log of the response ratio:
Mean effect sizes and 95% CIs from all 10 fields were then produced by bootstrapping 10,000 replicates in the rcompanion package v2.4.1 (Mangiafico, 2021), with the CIs calculated using the basic bootstrap method. This approach offers an intuitive way to interpret treatment effects while providing an alternative to traditional null-hypothesis significance testing (Hubbard and Lindsay, 2008; Brennan and Acosta-Martinez, 2019). Here, the CIs provide a range of possible effect sizes in which the true treatment effect is likely to reside, and the width of the interval indicates the precision of an estimate. Confidence intervals can also be used to infer significance: when the 95% CIs do not overlap zero, this can be considered as equivalent to a significant difference between treated and untreated sites at α = 0.05. In some cases, to aid interpretation, we back-transformed the effect sizes and associated CIs into percentage change using the following equation:
In a single case (i.e., one paired treatment), both the numerator and denominator were 0 and to facilitate inclusion in the effect size calculation, we added a constant of 1 to both.
Treatment effects on bacterial/archaeal and fungal community composition via DNA sequencing were analyzed separately from the other metrics. First, effects were visualized using NMDS of the bray-curtis metric and by plotting grouped pairwise differences in this metric between March 2018 (pre application) and each subsequent sampling date. This visualization exercise was conducted using the vegan package v2.5-7 and ggplot2 package in R (Wickham, 2016; Oksanen et al., 2020). Treatment effects were then analyzed statistically by using a Kruskal Wallis test to determine whether the pairwise bray-curtis distance between pre and post samples differed by treatment. This analysis was conducted using the longitudinal pairwise-distances command in QIIME2 (Bokulich et al., 2018), which assesses the distance between pre and post sample pairs and determines whether these paired differences differ significantly between two groups.
For a more integrated evaluation of treatment effects across metrics, we performed a principal components analysis (PCA) on the full dataset from May 2020 using the prcomp function and factoextra package v1.0.7 in R (Kassambara and Mundt, 2020). We paired the PCA with a multi-response permutation procedure (MRPP) based on Euclidean distance to test for a significant treatment difference (Mielke et al., 1981), using the vegan package v2.5-7 (Oksanen et al., 2020). The MRPP is a non-parametric procedure that tests the null hypothesis of no difference between groups.
In addition to determining treatment impacts across all 10 fields, for forage productivity we also compared effect sizes by field to % soil organic matter (SOM) values from each control site using a simple correlation coefficient. This approach allowed us to determine whether the response of forage productivity to treatment application was related to pre-existing levels of this central soil health indicator (Bradford et al., 2019).
Results
Plant Biomass and Forage Quality
Treatment effects on forage dynamics varied by year and metric. Forage production ranged from 559.58−11042.43 kg/ha and was on average 58% higher in treated compared to untreated sites (CI: 25, 96%; Figure 1; Appendix Table 2 in Supplementary Material). The average difference between treatments remained relatively constant between years (66 and 50% increase with treatment in 2018 and 2019, respectively), but the CI was wider in May 2019 (CI: −0.63, 117%) than May 2018 (CI: 34, 104%). Variation in effect sizes were not moderated by background SOM levels (Appendix Figure 4 in Supplementary Material); the correlation coefficient between forage production effect size and % SOM was −0.29 in 2018 and −0.03 in 2019 (P > 0.05).
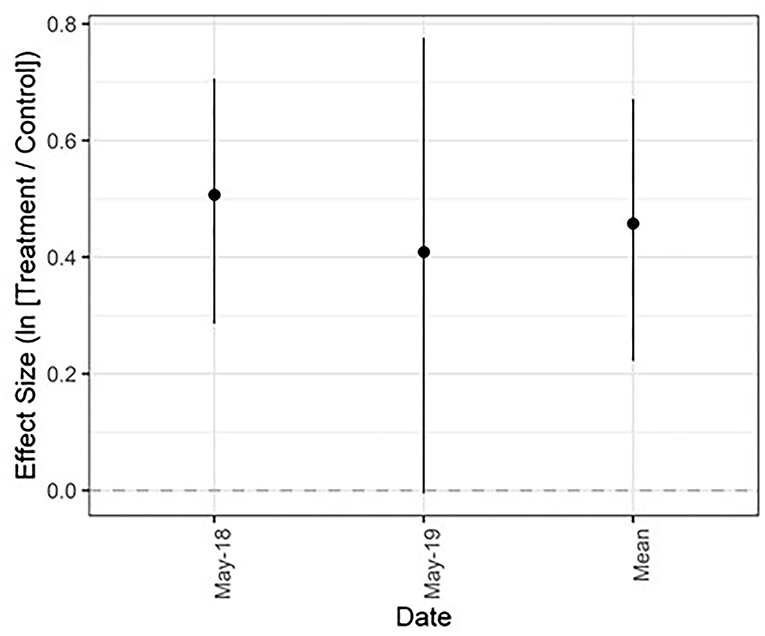
Figure 1. Mean effect size (natural log of the response ratio) and 95% confidence intervals for forage productivity across 2 years. The mean effect size is a product of all dates combined. The dashed horizontal line at 0 denotes no treatment effect (treatment value = control value). For values that fall above that line, treatment > control. For values that fall below that line, treatment < control.
In May 2018 and 2019, sites treated with the microbial inoculant and alkali-extracted “humic” product showed elevated levels of forage protein, calcium, and fat content compared to untreated sites—and decreased levels of ADF and NDF (Figure 2; Appendix Table 3 in Supplementary Material). However, these effect sizes were generally small and disappeared in May 2020. In contrast, levels of phosphorus and potassium showed a decline in treated compared to control plots in May 2020, whereas previous to that there were no discernable treatment effects (Figure 2). When averaged across all 3 years, ADF, calcium, and fat content had means and CIs that did not contain zero. ADF was 4% lower in treated sites (CI: −7.7, −0.4%), calcium was 18.8% higher (CI: 0.2, 40.6%), and fat was 6.4% higher (CI: 1.5, 11.5%).
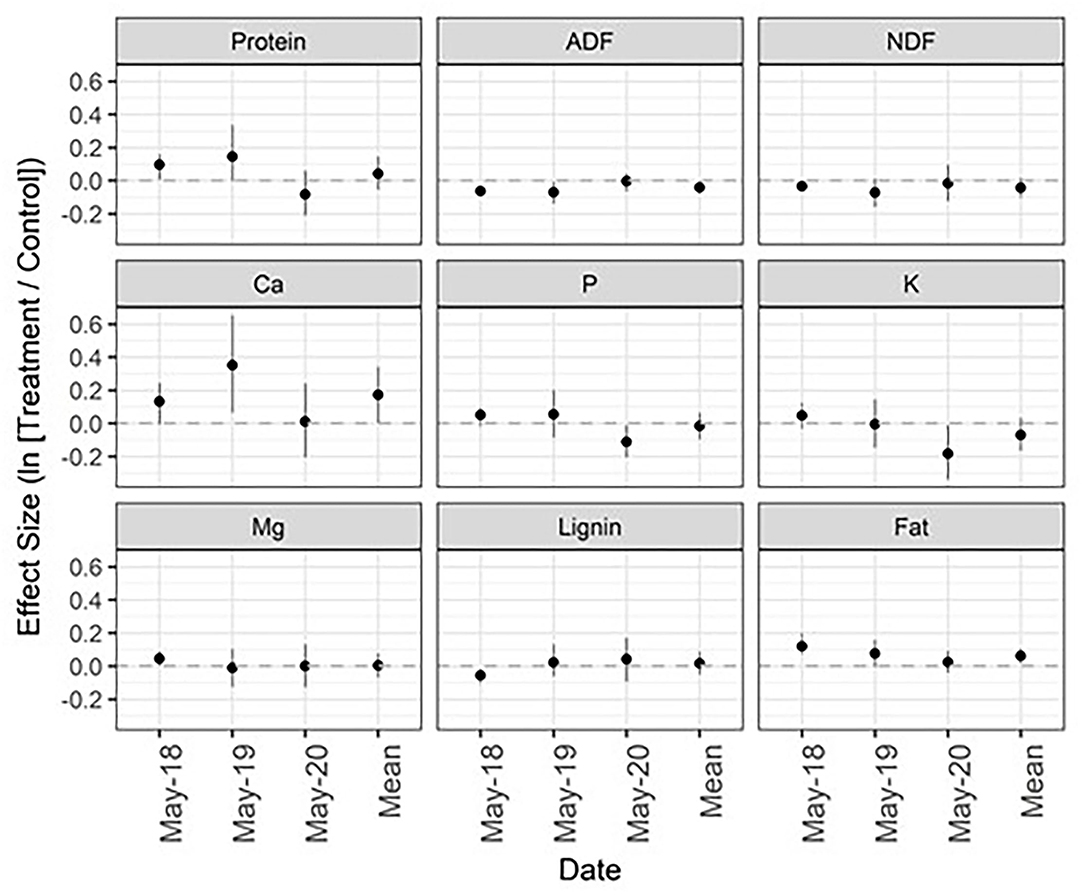
Figure 2. Mean effect size (natural log of the response ratio) and 95% confidence intervals for forage quality metrics across 3 years. The mean effect size is a product of all dates combined. Protein, crude protein; ADF, acid detergent fiber; NDF, neutral detergent fiber; Ca, calcium; P, phosphorus; K, potassium; Mg, magnesium. See Figure 1 caption for figure interpretation.
Microbial Biomass
Similar to forage, treatment effects on soil microbial communities varied by metric and sampling date. Total fungal biomass ranged from 291.1−1599.8 mg/kg dry soil and did not show sensitivity to treatment as evidenced by small mean effect sizes and narrow CIs overlapping zero (Figure 3; Appendix Table 4 in Supplementary Material). Active fungal biomass ranged from 1.1−265.5 mg/kg dry soil and showed the largest difference between treated and untreated sites over time, which can be seen in the comparatively large effect sizes that ranged on average from −0.4 to +0.6 across dates (Figure 3). While the data suggest treated sites likely had inherently less active fungal biomass than control sites prior to treatment application, in November 2018 and May 2020, active fungal biomass was on average 40.8% and 86.3% greater in treated compared to control plots (Figure 3). The CI in November 2019 included zero and was relatively wide (CI: −31.1, 232.0%), but in May 2020 the CI did not include zero (CI: 8.3, 222.2%), suggesting a significant difference between treatment and control. When averaged across all post-treatment sampling dates, total fungal biomass was 9.1% lower in treated sites (CI: −17.8, 0.4%) and active fungal biomass was 17.6% higher (CI: −10.1, 53.57%).
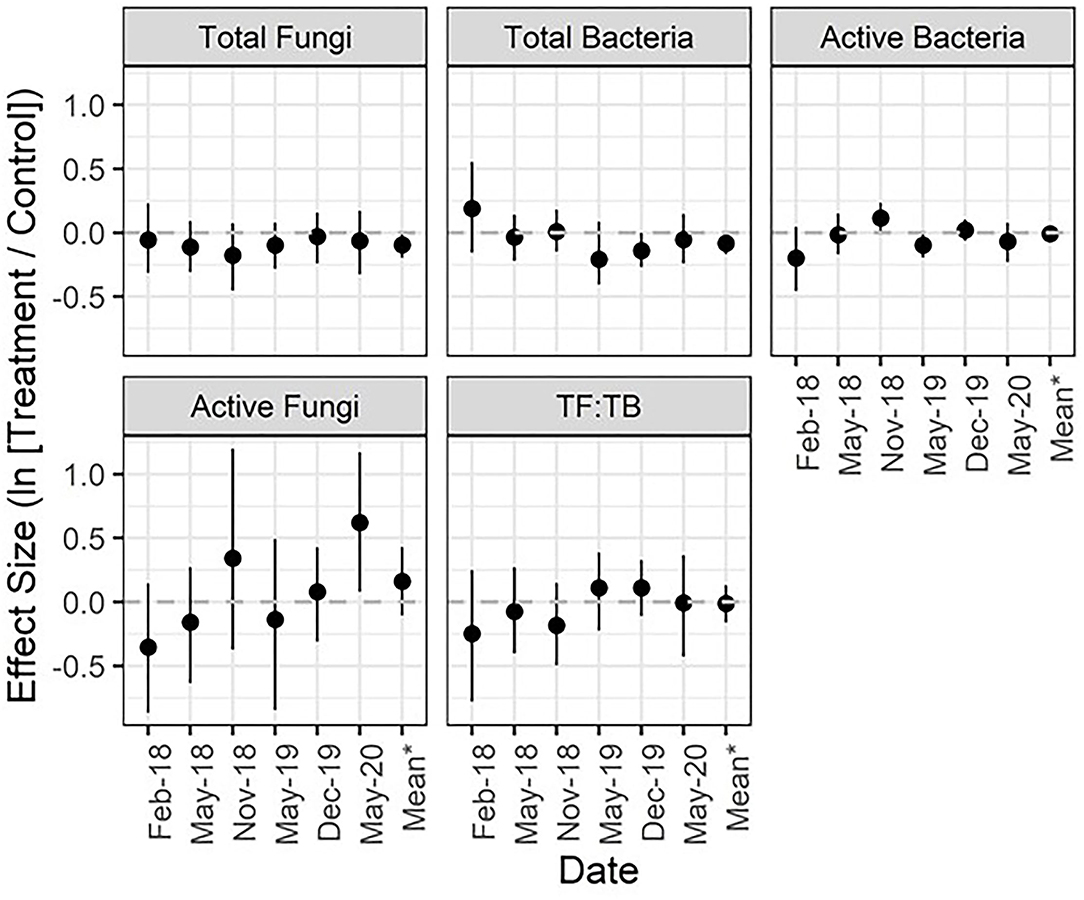
Figure 3. Mean effect size (natural log of the response ratio) and 95% confidence intervals for microbial biomass metrics across 3 years. *The mean effect size is a product of all dates post-application combined. TF:TB, ratio of total fungi to total bacteria. See Figure 1 caption for figure interpretation.
Total bacterial biomass ranged from 205.3−2893.6 mg/kg dry soil and the amount of bacterial biomass in treated compared to control plots decreased slightly after application of the microbial inoculant and alkali-extracted “humic” product. However, effect sizes were small and consistently overlaped zero. Active bacterial biomass ranged from 16.2−76.7 mg/kg dry soil and the largest difference between sites occurred pre-treatment in February of 2018 (−18.1%; CI: −36.6, 4.6%). Post-application, the effect sizes for active bacteria oscillated around zero and the CIs of all dates consistently crossed zero with the exception of November 2018, which showed a slight increase in active bacteria in treated compared to control plots (12.2%; CI: 0.11, 26.2%). The fungi:bacteria ratio varied from 0.2 to 3.8 across all sites and sampling dates, but did not show a strong or consistent response to treatment (Figure 3; Appendix Table 4 in Supplementary Material).
Total protozoa, flagellates, amoeba, and ciliates ranged from 1.2 × 107−6.8 × 108, 6.3 × 105−5.7 × 107, 7.1 × 106−6.7 × 108, and 0−1.5 × 106 individuals/kg dry soil, respectively. Ciliates were the most responsive to application of the microbial inoculant and alkali-extracted “humic” product, with treated sites harboring 131.4% (CI: 18.4, 343.7%) more ciliates than the control group in May 2020 (Figure 4; Appendix Table 5 in Supplementary Material). For all other protozoan groups and dates, the mean effect sizes were in general close to zero with relatively wide error bars that overlap zero, suggesting that treatment had a minimal impact.
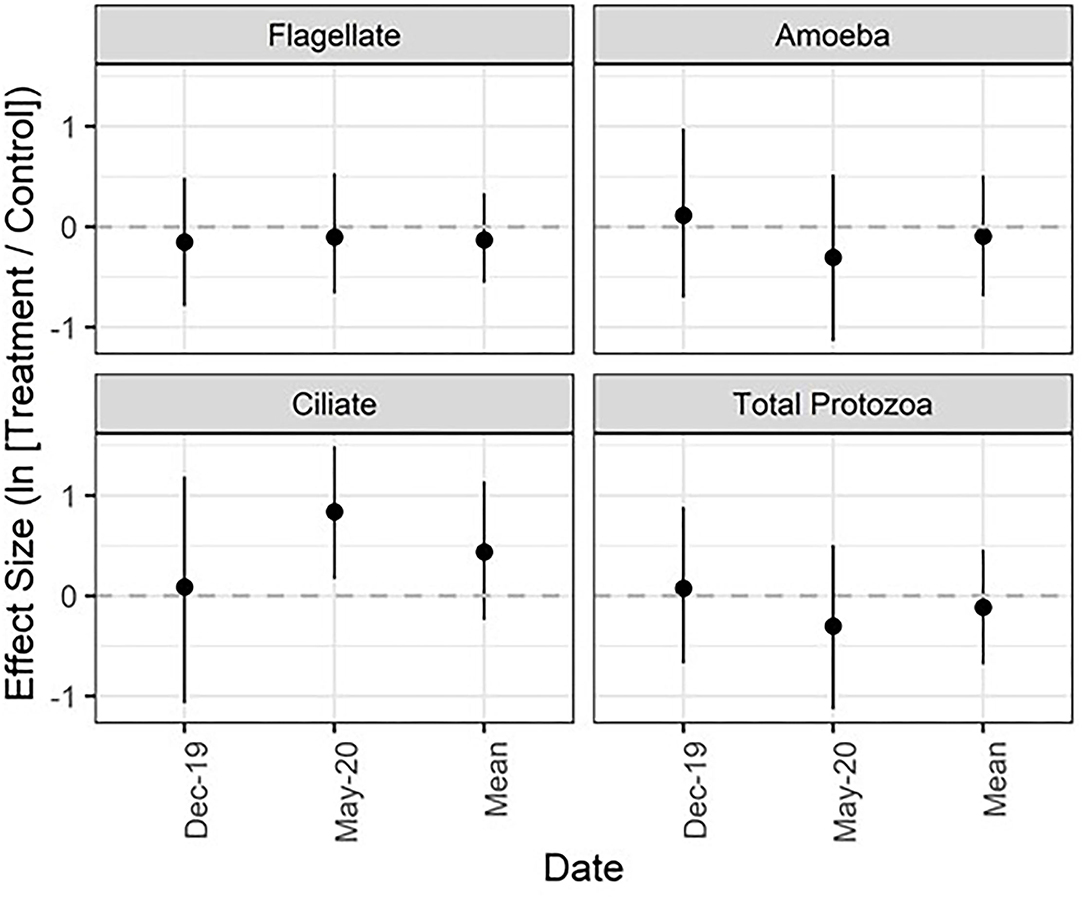
Figure 4. Mean effect size (natural log of the response ratio) and 95% confidence intervals for protozoa across 3 years. The mean effect size is a product of all dates combined. See Figure 1 caption for figure interpretation.
Microbial Community Structure
To provide a more detailed look at soil microbial community composition, we analyzed DNA amplicon sequences of the 16S rRNA gene and ITS1 region, which revealed that bacterial/archaeal and fungal community composition remained unaffected by treatment application at this level of resolution. This can be seen visually in the NMDS plots and the pairwise group differences between pre and post treatment application (Figures 5, 6). If treatment-induced differences existed, the distance between pre and post application would be larger for sites treated with the inoculant and alkali-extracted “humic” product than for sites left untreated. The Kruskal Wallis test confirmed that this was not the case and that pairwise bray-curtis distances between pre and post samples did not differ by treatment for bacteria/archaea (Pre-Time1: H = 0.02, P = 0.88; Pre-Time2: H = 0.46, P = 0.49) or fungi (Pre-Time1: H = 0.21, P = 0.65; Pre-Time2: H = 0.09, P = 0.76).
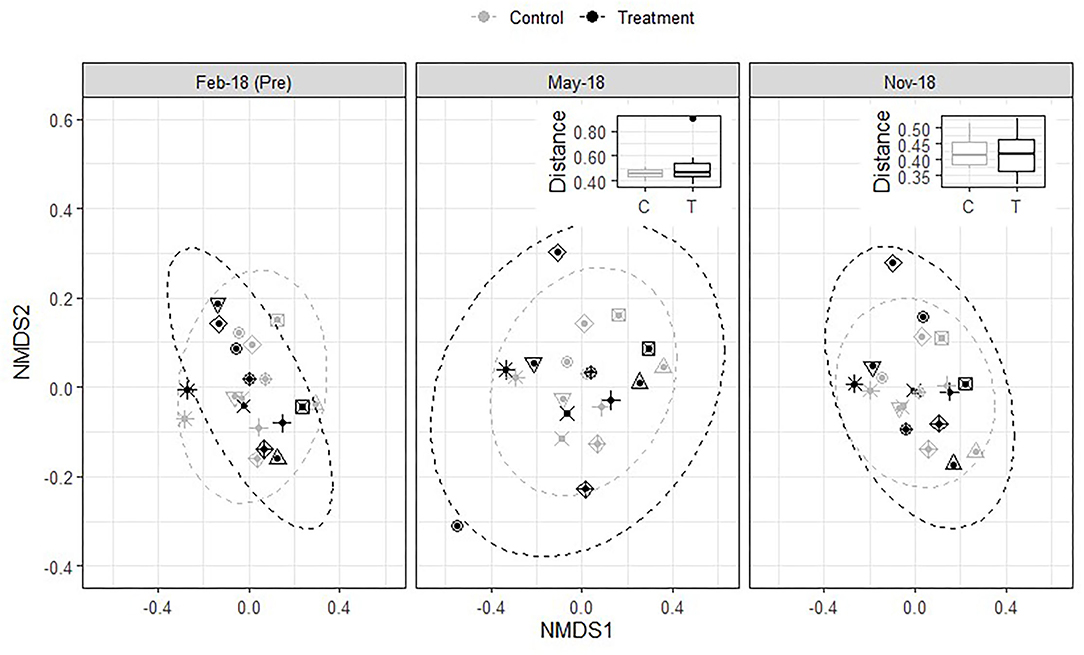
Figure 5. Influence of treatment on bacterial/archaeal community composition. Primary figure = Nonmetric multidimensional scaling (NMDS) plots of bray-curtis dissimilarity metric. Each symbol corresponds to a field, and each color corresponds to a treatment. Black = treatment with microbial inoculant and alkali-extracted “humic” product; Gray = untreated control. Points that are close to together represent samples with similar community composition, and the dashed oval represents 95% confidence intervals of sample ordination grouped by treatment. Inset = pairwise bray-curtis distance comparisons between March 2018 (pre application) and each subsequent sampling date.
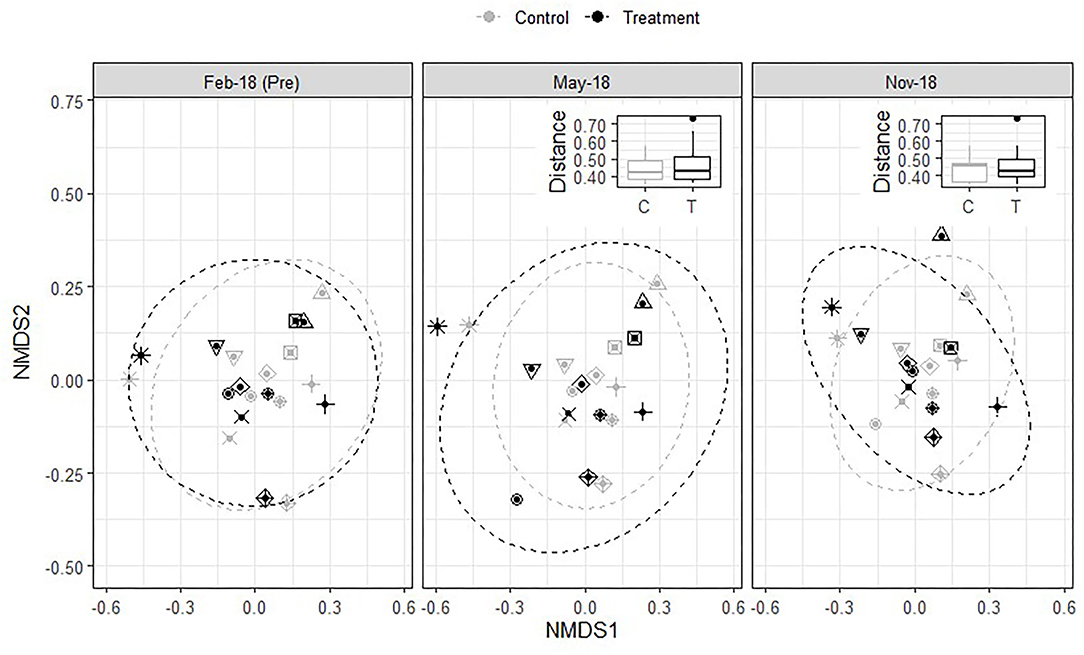
Figure 6. Influence of treatment on fungal community composition. See Figure 5 caption for figure interpretation.
Abiotic Soil Properties
In May 2020, we also assessed abiotic soil properties. General ranges of each property can be found in Appendix Table 6 in Supplementary Material. Although paired comparisons between the two treatments all included zero, the mean effect sizes and CIs provide evidence that calcium, magnesium, and the sum of base cations were more dissimilar between treatments than the other abiotic metrics (Figure 7; Appendix Table 6 in Supplementary Material). Specifically, average calcium, magnesium, and sum of base cation values were 11.4% (CI: −24.12, 1.71%), 13.6% (CI: −26.51, 1.79%), and 6.1% (CI: −11.75, 0.13%) lower in treated compared to control sites.
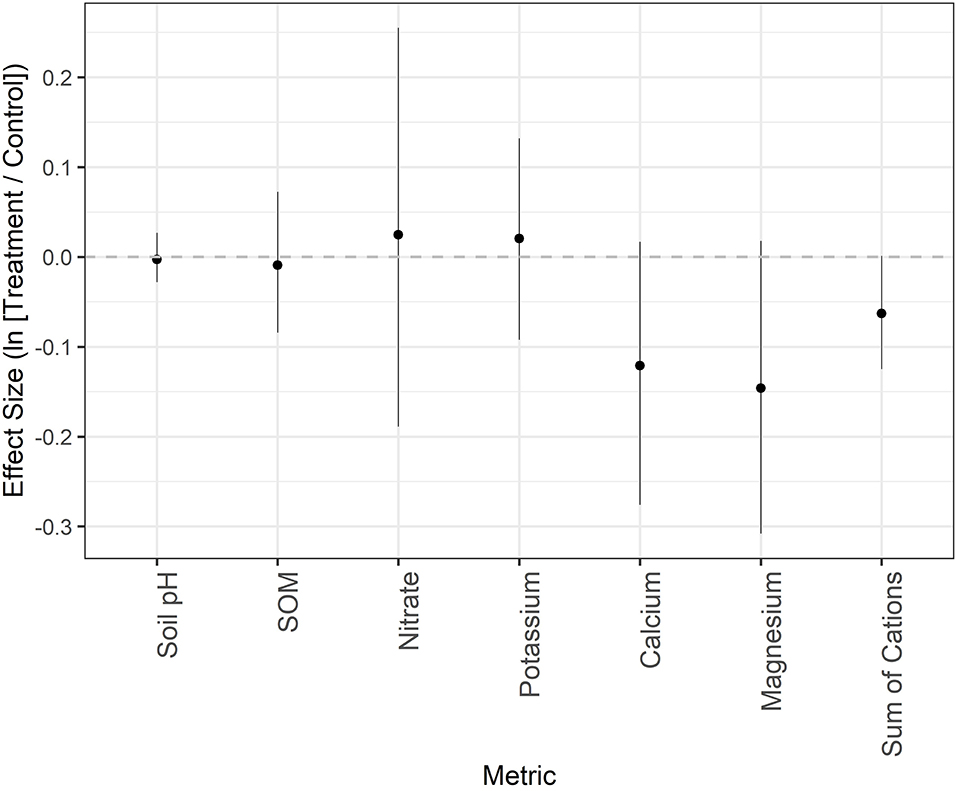
Figure 7. Mean effect size (natural log of the response ratio) and 95% confidence intervals for soil parameters from May 2020. SOM = soil organic matter; Sum of Cations = sum of the base cations.
For a more holistic and integrated evaluation of treatment effects on abiotic soil properties and other plant and microbial metrics collected in May 2020—the final sampling date in our study—we paired a PCA with a MRPP test of significance. The MRPP illustrated that, when all metrics were taken into account, treated and control sites did not differ significantly (effect size A = 0.03; P = 0.18). This also can be observed visually with the PCA, which shows considerable overlap between the two groups (Appendix Figure 5 in Supplementary Material).
Discussion
Understanding how commercially available biostimulant products, such as microbial inoculants and alkali-extracted “humic” substances, influence above and belowground dynamics on rangelands is important. This is especially true given increasing interest in these products to help manage rangelands regeneratively. In this study, we examined the combined effects of two such products that are currently on the market, and asked whether they influenced rangeland forage productivity and quality, soil microbial biomass and community composition, and abiotic soil parameters in Central Coastal California. While field studies such as this are limited in their ability to disentangle the chemical and biological mechanisms underpinning observed effects, they are useful for evaluating products under real-world conditions, assessing trade-offs, and generating a better understanding of the potential to reap economic and ecological benefits across varying agricultural production systems.
We found that foliar application of the commercial inoculant and alkali-extracted “humic” product consistently enhanced within-season forage production. Although microbial inoculant was applied in combination with the “humic” product at standardized rates throughout the experiment, at the beginning we followed the manufacturer's recommended application rates, which resulted in only a few fields receiving microbial inoculant in February 2018 (Appendix Table 1 in Supplementary Material). Given not every field received microbial inoculant as part of that first application, and that plant responses were observed soon after, it is likely the alkali-extracted “humic” product drove the observed response. Still, it is possible that introduction of the endophytic bacteria Bacillus subtilis or other microbial taxa in the inoculant contributed to plant growth promotion as well, particularly in the second year when all sites had received both products (Preininger et al., 2018).
Very few studies have measured how forage productivity changes in response to humic substances (Verlinden et al., 2007), and of those, most isolate one focal plant species (Churkova, 2013; Khaleda et al., 2017). Verlinden et al. (2010) found that applying a mixture of “humic” and “fulvic” acids increased initial grass production at four pastures dominated by Lolium multiflorum and L. perenne in northern Belgium; however, this effect attenuated after the first cut. The size of the initial response (i.e., the natural log of the response ratio) reported by Verlinden et al. (2010) ranged from 0.07−0.14, depending on the mode of application, which is considerably less than the mean effect sizes 0.51 in 2018 and 0.41 in 2019 from our study. Our work joins this small but growing list of others to illustrate that there is a potential role for these products to play when seeking to boost forage productivity of pasture and rangeland systems.
Assessing effect sizes is a critical aspect of interpreting the ecological and economic significance of treatment application for any outcome (e.g., Rinella and James, 2017). This is particularly true on agricultural lands, where decisions about how to allocate limited time and funding to achieve regenerative outcomes must be made carefully. When transformed to percentage change, forage production in treated sites was on average 66% (CI: 34, 104%) and 50% (CI: −0.6, 117%) higher than control sites in 2018 and 2019, respectively. This is equivalent to an average increase in forage production of 1,659 kg/ha and 1,314 kg/ha. This level of change between treatment and control is equivalent to what can be seen across growing seasons with different amounts of rainfall on the Central Coast (Becchetti et al., 2016). This shows the potential of the products to boost forage production, with possible benefits for livestock stocking density and carrying capacity. While the results need to be confirmed in other contexts before recommendation at scale, the promising nature warrants increased research and consideration for deploying this product in an adaptive management framework to help manage forage production on rangelands.
We found no evidence that background levels of SOM influenced how forage productivity responded to treatment application, despite a more than two-fold difference in SOM levels across sites. Soil organic matter provides myriad benefits including improved water retention and nutrient supply to plants (reviewed in Lal, 2009) as well as support of soil biodiversity (Murphy et al., 2011). Soils with higher SOM levels should therefore create conditions more conducive to plant growth and resiliency, all else equal. Oldfield et al. (2019) demonstrated this by comparing maize and wheat yields to soil organic carbon (SOC; a proxy for SOM) globally and finding that yields correlated positively with SOC until a threshold of 2% (Oldfield et al., 2019). A recent study by Kane et al. (2021) demonstrated that the benefits of SOM—namely benefits to available water capacity and cation exchange capacity—extend beyond this to help stabilize maize yields during stressful drought conditions. Because less optimal growing conditions are likely to enhance the benefits of microbial inoculation (Hart et al., 2018) and alkali-extracted “humic” substances (Olk et al., 2021), it is conceivable that sites with lower SOM levels would be in greater need and thus more responsive to application. However, we found no evidence for this to be the case, providing new information that at least in rangelands of Central Coastal California, this contextual parameter does not seem to moderate the impact of treatment application on peak growth. Other aspects of forage production, such as growing season length, are important in these systems from a livestock production standpoint, and it's possible that product application could interact with contextual factors like SOM to influence this and other parameters not captured here.
In addition to boosting forage productivity, the microbial inoculant and alkali-extracted “humic” product improved some aspects related to forage quality—albeit to a lesser degree. Notably, concentrations of ADF were 4.0% lower in treated compared to untreated sites when averaged across all 3 years. Acid detergent fiber contains cellulose and lignin, and is inversely related to the digestibility and energy of forage (Colburn and Evans, 1967). While an increase in crude fiber has been observed in crops like corn and soybean (Kocira et al., 2019; Efthimiadou et al., 2020), a decrease in ADF with application of the inoculant and alkali-extracted “humic” product in this case suggests an improvement to forage quality for rangeland systems. Further confirming this, calcium was on average 18.8% higher and fat content was on average 6.4% higher in sites that were treated compared to those that were not. Fat is a potent source of energy and calcium serves as an essential plant nutrient, and increases in these metrics can be interpreted as indicating improved forage quality. Indeed, although beef cattle in California tend to be deficient in other minerals like manganese, zinc, and selenium (Davy et al., 2019), deficiencies in calcium can affect the livestock industry and so increased concentrations of this nutrient may be important in some cases. Although we cannot determine the mechanisms behind such a response, it's possible that the alkali-extracted “humic” product stimulated uptake of calcium (Olk et al., 2018), which is generally immobile in plants, and influenced metabolic pathways that regulate lipid, lignin, and cellulose production.
It is possible that other components of the alkali-extracted “humic” product contributed to the observed plant responses a well. Notably, the product contained trace amounts of nutrients extracted, in part, from the seaweed Ascophyllum nodosum. Considerable evidence suggests that very low doses of A. nodosum extract can stimulate plant growth and alleviate abiotic and biotic stressors (reviewed in Shukla et al., 2019). For instance, Jannin et al. (2013) found just 0.1% (vol/vol) of a commercial A. nodosum extract, AZAL5®, helped to stimulate nitrogen and sulfate uptake allowing for an increased growth of rapeseed (Brassica napus). Others have shown that extracts of A. nodosum regulate phytohormone biosynthesis (e.g., auxins, cytokinins, abscisic acid) and increase plant growth in that way as well (Craigie, 2011). While we are unable to disentangle the relative influence of product components in this study, our findings suggest that the application of the commercial products at hand have the potential to improve forage quality and some metrics of palatability in rangeland systems.
Unlike aboveground forage dynamics, we did not see a strong or consistent response to treatment application for soil microbial communities or abiotic soil parameters. Given that the microbial inoculant was applied as a foliar spray, it is perhaps not surprising that belowground shifts in microbial biomass, activity, or community composition were not apparent within the timeframe of our study. Microorganisms applied in this way are thought to improve plant growth, quality, and stress resilience by entering the plant via the stomata (Preininger et al., 2018). They are not likely to intercept and sufficiently interact with the soil when applied at low concentrations to sites that have considerable live or dead standing plant material, such as those included in this study. Similarly, because we were applying alkali-extracted “humic” products as a biostimulant, the application rates would be too low to influence SOM dynamics or nutrient availability via direct addition. However, as others have suggested (Olk et al., 2018), there is a potential for soil health metrics like microbial biomass and SOM to improve over time if plant productivity remains elevated, especially of root growth (Rasse et al., 2005; Conselvan et al., 2017). Given the importance of soils for sustaining ranch operations and public benefits like climate change mitigation (Bradford et al., 2019), the direct and indirect benefits of these management tools will be important to capture in the near- and long-term. This will help to inform expectations and decision-making for ranchers and policy-makers who are looking for new products to help build resiliency into landscapes.
Conclusion
There is increasing interest in using biostimulant products, such as microbial inoculants and alkali-extracted “humic” substances, to help manage rangelands regeneratively. Understanding how above and belowground dynamics on rangelands respond to these products is therefore important. In our study, we found that forage productivity and some metrics of forage quality responded positively to the foliar application of a commercial microbial inoculant and alkali-extracted “humic” product. These benefits were not mirrored by changes belowground in the microbial community or abiotic parameters, but also did not come at a cost to the measured parameters as well. Depending on the goals of using the products, this could be seen as a winning scenario and suggests microbial inoculants and alkali-extracted “humic” products could warrant attention as a potential tool for regenerative stewardship of rangelands. While our study derives from one ranch and therefore requires confirmation of its ubiquity, our results provide compelling new insights into the usefulness of this approach for managing rangeland productivity in California's Central Coast. As with any new management tool, we recommend ranchers interested in these products first apply to small areas in an adaptive management framework and then scale accordingly.
Data Availability Statement
The sequencing datasets presented in this study can be found in online repositories. The names of the repository/repositories and accession number(s) can be found at: NCBI [accession: PRJNA808421].
Author Contributions
CC, HS, and MB conceived and planned the experiment. HS, MB, and FS implemented the treatments. CC analyzed the data and wrote the first draft of the article. All authors carried out the experiment, interpretation of the results, contributed to the article, and approved the submitted version.
Funding
This work was supported by funding from the TomKat Ranch Educational Foundation.
Conflict of Interest
FS works for Regenerative Land Solutions, a distributor of Earthfort LLC products.
The remaining authors declare that the research was conducted in the absence of any commercial or financial relationships that could be construed as a potential conflict of interest.
Publisher's Note
All claims expressed in this article are solely those of the authors and do not necessarily represent those of their affiliated organizations, or those of the publisher, the editors and the reviewers. Any product that may be evaluated in this article, or claim that may be made by its manufacturer, is not guaranteed or endorsed by the publisher.
Acknowledgments
We thank many TomKat apprentices for their help collecting plant and soil samples, including Andrea Hatsukami, Justin Miller, Drake Swezey, Evan Watson, Dillon Gruber, Jessica Teresi, Alex Michel, Jose Ramirez Martinez, Mariana Zavala, Lana Jansen, and Celia Hoffman. We also thank Wendy Millet, Libby Porzig, Grant Ballard, and Suzane Rowland for support of the project and review of the manuscript.
Supplementary Material
The Supplementary Material for this article can be found online at: https://www.frontiersin.org/articles/10.3389/fsufs.2022.847096/full#supplementary-material
Footnotes
1. ^We have chosen to use the term "humic" due to its pervasiveness in scientific and management discourse, but use quotations throughout as a way to acknowledge the outdated nature of this terminology based on current understanding of soil organic matter dynamics (Lehman and Kleber, 2015).
References
Adesemoye, A. O., Torbert, H. A., and Kloepper, J. W. (2009). Plant growth-promoting rhizobacteria allow reduced application rates of chemical fertilizers. Microb. Ecol. 58, 921–929. doi: 10.1007/s00248-009-9531-y
Arora, N. K., Fatima, T., Mishra, I., and Verma, S. (2020). “Microbe-based inoculants: role in next green revolution,” in Environmental Concerns and Sustainable Development (Singpore: Springer), pp. 191–246.
Babiuk, L. A., and Paul, E. A. (1970). The use of fluorescein isothiocyanate in the determination of the bacterial biomass of grassland soil. Canad. J. Microbiol. 16, 57–62. doi: 10.1139/m70-011
Becchetti, T., George, M., McDougald, N., Dudley, D., Connor, M., Flavel, D., et al. (2016). Rangeland Management Series: Annual Range Forage Production. California: ANR Publication. doi: 10.3733/ucanr.8018
Bokulich, N. A., Kaehler, B. D., Rideout, J. R., Dillon, M., Bolyen, E., Knight, R., et al. (2018). Optimizing taxonomic classification of marker-gene amplicon sequences with QIIME 2's q2-feature-classifier plugin. Microbiome. 6, 1–17. doi: 10.1186/s40168-018-0470-z
Bolyen, E., Rideout, J. R., Dillon, M. R., Bokulich, N. A., Abnet, C. C., Al-Ghalith, G. A., et al. (2019). Reproducible, interactive, scalable and extensible microbiome data science using QIIME 2. Nat. Biotechnol. 37, 852–857. doi: 10.1038/s41587-019-0209-9
Bradford, M. A., Carey, C. J., Atwood, L., Bossio, D., Fenichel, E. P., Gennet, S., et al. (2019). Soil carbon science for policy and practice. Nat. Sustain. 2, 1070–1072. doi: 10.1038/s41893-019-0431-y
Brennan, E. B., and Acosta-Martinez, V. (2019). Cover crops and compost influence soil enzymes during 6 years of tillage-intensive, organic vegetable production. Soil Sci. Soc. Am. J. 83, 624–637. doi: 10.2136/sssaj2017.12.0412
Calvo, P., Nelson, L., and Kloepper, J. W. (2014). Agricultural uses of plant biostimulants. Plant Soil 383, 3–41. doi: 10.1007/s11104-014-2131-8
Chamizo, S., Rodríguez-Caballero, E., Cantón, Y., and De Philippis, R. (2018). Soil inoculation with cyanobacteria: reviewing its' potential for agriculture sustainability in Drylands. Agri. Res. Tech Open Access. J. 18, 10–19080. doi: 10.19080/ARTOAJ.2018.18.556046
Churkova, B. (2013). Influence of some bio-products on the biological and productive characteristics of bird's foot trefoil grown for forage. Biotechnol. Anim. Husb. 29, 123–132. doi: 10.2298/BAH1301123C
Colburn, M. W., and Evans, J. L. (1967). Chemical composition of the cell-wall constituent and acid detergent fiber fractions of forages. J. Dairy Sci. 50, 1130–1135. doi: 10.3168/jds.S0022-0302(67)87578-7
Conselvan, G. B., Pizzeghello, D., Francioso, O., Di Foggia, M., Nardi, S., and Carletti, P. (2017). Biostimulant activity of humic substances extracted from leonardites. Plant soil. 420, 119–134. doi: 10.1007/s11104-017-3373-z
Craigie, J. S. (2011). Seaweed extract stimuli in plant science and agriculture. J. Appl. Phycol. 23, 371–393. doi: 10.1007/s10811-010-9560-4
Darbyshire, J. F., Wheatley, R. E., Greaves, M. P., and Inkson, R. H. E. (1974). Rapid micromethod for estimating bacterial and protozoan populations in soil. Rev. Ecol. Biol. Sol. 11, 465–475.
Davy, J. S., Forero, L. C., Shapero, M. W., Rao, D. R., Becchetti, T. A., Koopman Rivers, C., et al. (2019). Mineral status of California beef cattle. Translational Animal Science, 3, 66–73. doi: 10.1093/tas/txy114
Del Buono, D. (2021). Can biostimulants be used to mitigate the effect of anthropogenic climate change on agriculture? it is time to respond. Sci. Tot. Environ. 751, 141763. doi: 10.1016/j.scitotenv.2020.141763
Dos Santos, B. D. M. S., de Sousa Antunes, L. F., Dos Santos, R. M., Santos, C. H. B., and Rigobelo, E. C. (2021). Humic substances in combination with plant growth-promoting bacteria as an alternative for sustainable agriculture. Front. Microbiol. 12, 53. doi: 10.3389/fmicb.2021.719653
Efthimiadou, A., Katsenios, N., Chanioti, S., Giannoglou, M., Djordjevic, N., and Katsaros, G. (2020). Effect of foliar and soil application of plant growth promoting bacteria on growth, physiology, yield and seed quality of maize under Mediterranean conditions. Scientific Rep. 10, 1–11. doi: 10.1038/s41598-020-78034-6
Gilbert, J. A., Meyer, F., Antonopoulos, D., Balaji, P., Brown, C. T., Brown, C. T., et al. (2010). Meeting report: the terabase metagenomics workshop and the vision of an Earth microbiome project. Standards Genom. Sci. 3, 243–248. doi: 10.4056/sigs.1433550
Godde, C. M., Boone, R. B., Ash, A. J., Waha, K., Sloat, L. L., Thornton, P. K., et al. (2020). Global rangeland production systems and livelihoods at threat under climate change and variability. Environ. Res. Lett. 15, 044021. doi: 10.1088/1748-9326/ab7395
Haider, G., Koyro, H. W., Azam, F., Steffens, D., Müller, C., and Kammann, C. (2015). Biochar but not humic acid product amendment affected maize yields via improving plant-soil moisture relations. Plant Soil. 395, 141–157. doi: 10.1007/s11104-014-2294-3
Halpern, M., Bar-Tal, A., Ofek, M., Minz, D., Muller, T., and Yermiyahu, U. (2015). The use of biostimulants for enhancing nutrient uptake. Adv. Agron. 130, 141–174. doi: 10.1016/bs.agron.2014.10.001
Hamdali, H., Hafidi, M., Virolle, M. J., and Ouhdouch, Y. (2008). Rock phosphate-solubilizing Actinomycetes: screening for plant growth-promoting activities. World J. Microbiol. Biotechnol. 24, 2565–2575. doi: 10.1007/s11274-008-9817-0
Hart, M. M., Antunes, P. M., Chaudhary, V. B., and Abbott, L. K. (2018). Fungal inoculants in the field: is the reward greater than the risk? Funct. Ecol. 32, 126–135. doi: 10.1111/1365-2435.12976
Hart, M. M., and Forsythe, J. A. (2012). Using arbuscular mycorrhizal fungi to improve the nutrient quality of crops; nutritional benefits in addition to phosphorus. Scientia Horticult. 148, 206–214. doi: 10.1016/j.scienta.2012.09.018
Hartz, T. K., and Bottoms, T. G. (2010). Humic substances generally ineffective in improving vegetable crop nutrient uptake or productivity. HortScience 45, 906–910. doi: 10.21273/HORTSCI.45.6.906
Henneman, C., Seavy, N. E., and Gardali, T. (2014). Restoring native perennial grasses by changing grazing practices in central coastal California. Ecol. Restorat. 32, 352–354. doi: 10.3368/er.32.4.352
Hiatt, C., Fernandez, D., and Potter, C. (2012). Measurements of fog water deposition on the California Central Coast. Atmosphere. Clim. Sci. 2, 525. doi: 10.4236/acs.2012.24047
Hubbard, R., and Lindsay, R. M. (2008). Why P values are not a useful measure of evidence in statistical significance testing. Theory Psychol. 18, 69–88. doi: 10.1177/0959354307086923
Ingham, E. R., and Klein, D. A. (1984). Soil fungi: relationships between hyphal activity and staining with fluorescein diacetate. Soil Biol. Biochemistr. 16, 273–278. doi: 10.1016/0038-0717(84)90014-2
Jannin, L., Arkoun, M., Etienne, P., Laîn,é, P., Goux, D., Garnica, M., et al. (2013). Brassica napus growth is promoted by Ascophyllum nodosum (L.) Le Jol seaweed extract: microarray analysis and physiological characterization of N, C, and S metabolisms. J. Plant Growth Regulat. 32, 31–52. doi: 10.1007/s00344-012-9273-9
Jindo, K., Olivares, F. L., Malcher, D. J. D. P., Sánchez-Monedero, M. A., Kempenaar, C., and Canellas, L. P. (2020). From lab to field: role of humic substances under open-field and greenhouse conditions as biostimulant and biocontrol agent. Front. Plant Sci. 11, 426. doi: 10.3389/fpls.2020.00426
Justi, M., Morais, E. G., and Silva, C. A. (2019). Fulvic acid in foliar spray is more effective than humic acid via soil in improving coffee seedlings growth. Archiv. Agron. Soil Sci. 65, 1969–1983. doi: 10.1080/03650340.2019.1584396
Kaminsky, L. M., Trexler, R. V., Malik, R. J., Hockett, K. L., and Bell, T. H. (2019). The inherent conflicts in developing soil microbial inoculants. Trends Biotechnol. 37, 140–151. doi: 10.1016/j.tibtech.2018.11.011
Kane, D. A., Bradford, M. A., Fuller, E., Oldfield, E. E., and Wood, S. A. (2021). Soil organic matter protects US maize yields and lowers crop insurance payouts under drought. Environ. Res. Lett. 16, 044018. doi: 10.1088/1748-9326/abe492
Kassambara, A., and Mundt, F. (2020). Package ‘factoextra’. Extract and visualize the results of multivariate data analyses. R package version 1.0.7. Available online at: https://CRAN.R-project.org/package=factoextra
Khaleda, L., Kim, M. G., Jeon, J. R., Cha, J. Y., and Kim, W. Y. (2017). Foliar application of humic acid or a mixture of catechol and vanillic acid enhanced growth and productivity of alfalfa. J. Korean Soc. Grassland Forage Sci. 37, 248–253. doi: 10.5333/KGFS.2017.37.3.248
Khatoon, Z., Huang, S., Rafique, M., Fakhar, A., Kamran, M. A., and Santoyo, G. (2020). Unlocking the potential of plant growth-promoting rhizobacteria on soil health and the sustainability of agricultural systems. J. Environ. Manage. 273, 111118. doi: 10.1016/j.jenvman.2020.111118
Kocira, S., Szparaga, A., Kocira, A., Czerwińska, E., Depo, K., Erlichowska, B., et al. (2019). Effect of applying a biostimulant containing seaweed and amino acids on the content of fiber fractions in three soybean cultivars. Legume Res. Int. J. 42, 341–347. doi: 10.18805/LR-412
Kong, Z., Hart, M., and Liu, H. (2018). Paving the way from the lab to the field: using synthetic microbial consortia to produce high-quality crops. Front. Plant Sci. 9, 1467. doi: 10.3389/fpls.2018.01467
Koziol, L., and Bever, J. D. (2017). The missing link in grassland restoration: arbuscular mycorrhizal fungi inoculation increases plant diversity and accelerates succession. J. Appl. Ecol. 54, 1301–1309. doi: 10.1111/1365-2664.12843
Lal, R. (2009). Challenges and opportunities in soil organic matter research. Euro. J. Soil Sci. 60, 158–169. doi: 10.1111/j.1365-2389.2008.01114.x
Lal, R. (2020). Regenerative agriculture for food and climate. J. Soil Water Conservat. 75, 123A-124A. doi: 10.2489/jswc.2020.0620A
Lehman, J., and Kleber, M. (2015). The contentious nature of soil organic matter. Nature 528, 60–68. doi: 10.1038/nature16069
Lodge, D. J., and Ingham, E. R. (1991). A comparison of agar film techniques for estimating fungal biovolumes in litter and soil. Agricult. Ecosyst. Environ. 34, 131–144. doi: 10.1016/0167-8809(91)90101-3
Maltz, M. R., and Treseder, K. K. (2015). Sources of inocula influence mycorrhizal colonization of plants in restoration projects: a meta-analysis. Restorat. Ecol. 23, 625–634. doi: 10.1111/rec.12231
Mangiafico, S. (2021). Companion: Functions to support extension education program evaluation. R package version 2.4.1. Available online at: https://CRAN.R-project.org/package=rcompanion
Mao, J., Olk, D. C., Chen, N., Dinnes, D. L., and Chappell, M. (2013). “Chemical properties of humic and fulvic acid products and their ores of origin,” in Functions of Natural Organic Matter in Changing Environment (Dordrecht: Springer), pp. 1067–1070.
Marotz, L., Schwartz, T., Thompson, L., Humphrey, G., Gogul, G., and Gaffney, J. (2018) Earth Microbiome Project (EMP) high throughput (HTP) DNA extraction protocol. Version 18, 07112018. doi: 10.17504/protocols.io.pdmdi46.
Mayer, J., Scheid, S., Widmer, F., Fließbach, A., and Oberholzer, H. R. (2010). How effective are ‘Effective microorganisms® (EM)’? results from a field study in temperate climate. Appl. Soil Ecol. 46, 230–239. doi: 10.1016/j.apsoil.2010.08.007
Mielke, P. W. Jr., Berry, K. J., and Brier, G. W. (1981). Application of multi-response permutation procedures for examining seasonal changes in monthly mean sea-level pressure patterns. Monthly Weather Rev. 109, 120–126.
Murphy, D. V., Cookson, W. R., Braimbridge, M., Marschner, P., Jones, D. L., Stockdale, E. A., et al. (2011). Relationships between soil organic matter and the soil microbial biomass (size, functional diversity, and community structure) in crop and pasture systems in a semi-arid environment. Soil Res. 49, 582–594. doi: 10.1071/SR11203
Nardi, S., Pizzeghello, D., Muscolo, A., and Vianello, A. (2002). Physiological effects of humic substances on higher plants. Soil Biol. Biochemistr. 34, 1527–1536. doi: 10.1016/S0038-0717(02)00174-8
Newton, P., Civita, N., Frankel-Goldwater, L., Bartel, K., and Johns, C. (2020). What is regenerative agriculture? a review of scholar and practitioner definitions based on processes and outcomes. Front. Sustain. Food Syst. 4, 194. doi: 10.3389/fsufs.2020.577723
Nilsson, R. H., Larsson, K. H., Taylor, A. F., Bengtsson-Palme, J., Jeppesen, T. S., Schigel, D., et al. (2019). The UNITE database for molecular identification of fungi: handling dark taxa and parallel taxonomic. Nucleic Acids Res. 47, D259–D264. doi: 10.1093/nar/gky1022
Oksanen, J., Blanchet, F. G., Friendly, M., Kindt, R., Legendre, P., McGlinn, D., et al. (2020). Vegan: Community ecology package. R package version 2.5-7. Available online at: https://CRAN.R-project.org/package=vegan
Oldfield, E. E., Bradford, M. A., and Wood, S. A. (2019). Global meta-analysis of the relationship between soil organic matter and crop yields. Soil. 5, 15–32. doi: 10.5194/soil-5-15-2019
Olk, D. C., Dinnes, D. L., Callaway, C., and Raske, M. (2013). “On-farm evaluation of a alkali-extracted product in Iowa (US) maize production,” in Functions of Natural Organic Matter in Changing Environment (Dordrecht: Springer), pp. 1047–1050.
Olk, D. C., Dinnes, D. L., Scoresby, J. R., Callaway, C. R., and Darlington, J. W. (2018). Alkali-extracted products in agriculture: potential benefits and research challenges—a review. Journal of Soils and Sediments, 18, 2881–2891. doi: 10.1007/s11368-018-1916-4
Olk, D. C., Dinnes, D. L., Scoresby, J. R., Darlington, J. W., Hurburgh, C. R., and Rippke, G. R. (2021). Maize Growth and Grain Yield Responses to a Micronized Alkali-extracted product Across Soil Types and Annual Weather Patterns in Central Iowa, United States. Front. Plant Sci. 12, 838. doi: 10.3389/fpls.2021.672078
Perkins, L. B., and Hatfield, G. (2016). Can commercial soil microbial treatments remediate plant-soil feedbacks to improve restoration seedling performance? Restorat. Ecol. 24, 194–201. doi: 10.1111/rec.12302
Preininger, C., Sauer, U., Bejarano, A., and Berninger, T. (2018). Concepts and applications of foliar spray for microbial inoculants. Appl. Microbiol. Biotechnol. 102, 7265–7282. doi: 10.1007/s00253-018-9173-4
Quast, C., Pruesse, E., Yilmaz, P., Gerken, J., Schweer, T., and Yarza, P. (2013) The SILVA ribosomal RNA gene database project: improved data processing web-based tools. Nucl. Acids Res. 41, D590–D596. 10.1093/nar/gks1219.
Rasse, D. P., Rumpel, C., and Dignac, M. F. (2005). Is soil carbon mostly root carbon? Mechan. Specific Stabilisat. 269, 341–356. doi: 10.1007/s11104-004-0907-y
Rinella, M. J., and James, J. J. (2017). A modelling framework for improving plant establishment during ecological restoration. Ecologic. Modell. 361, 177–183. doi: 10.1016/j.ecolmodel.2017.08.005
Rivers, A. R., Weber, K. C., Gardner, T. G., Liu, S., and Armstrong, S. D. (2018). ITSxpress: Software to rapidly trim internally transcribed spacer sequences with quality scores for marker gene analysis. F 1000 Research 7, 25. doi: 10.12688/f1000research.15704.1
Robertson, G. P., Coleman, D. C., Sollins, P., and Bledsoe, C. S. (1999). Standard Soil Methods for Long-term Ecological Research. New York, NY: Oxford University Press.
Rose, M. T., Patti, A. F., Little, K. R., Brown, A. L., Jackson, W. R., and Cavagnaro, T. R. (2014). A meta-analysis and review of plant-growth response to humic substances: practical implications for agriculture. Adv. Agron. 124, 37–89. doi: 10.1016/B978-0-12-800138-7.00002-4
Shah, Z. H., Rehman, H. M., Akhtar, T., Alsamadany, H., Hamooh, B. T., Mujtaba, T., et al. (2018). Humic substances: Determining potential molecular regulatory processes in plants. Front. Plant Sci. 9, 263. doi: 10.3389/fpls.2018.00263
Shukla, P. S., Mantin, E. G., Adil, M., Bajpai, S., Critchley, A. T., and Prithiviraj, B. (2019). Ascophyllum nodosum-based biostimulants: Sustainable applications in agriculture for the stimulation of plant growth, stress tolerance, and disease management. Front. Plant Sci. 10, 655. doi: 10.3389/fpls.2019.00655
Smith, D. P., and Peay, K. G. (2014). Sequence depth, not PCR replication, improves ecological inference from next generation DNA sequencing. PLoS ONE 9, e90234. doi: 10.1371/journal.pone.0090234
Verlinden, G., Coussens, T., De Vliegher, A., Baert, G., and Haesaert, G. (2010). Effect of humic substances on nutrient uptake by herbage and on production and nutritive value of herbage from sown grass pastures. Grass Forage Sci. 65, 133–144. doi: 10.1111/j.1365-2494.2009.00726.x
Verlinden, G., Mertens, J., Pycke, B., Debersaques, F., Bries, J., Baert, G., et al. (2007). “Addition of humic substances improves yield and nutrient uptake efficiency of grasslands. In Permanent and temporary grassland: plant, environment and economym” in Proceedings of the 14th Symposium of the European Grassland Federation (Ghent: Belgian Society for Grassland and Forage Crops), pp. 142–145.
Verlinden, G., Pycke, B., Mertens, J., Debersaques, F., Verheyen, K., Baert, G., et al. (2009). Application of humic substances results in consistent increases in crop yield and nutrient uptake. J. Plant Nutri. 32, 1407–1426. doi: 10.1080/01904160903092630
Walters, W., Hyde, E. R., Berg-Lyons, D., Ackermann, G., Humphrey, G., Parada, A., et al. (2016). Improved bacterial 16S rRNA gene (V4 and V4-5) and fungal internal transcribed spacer marker gene primers for microbial community surveys. mSystems 1, e00009-15. doi: 10.1128/mSystems.00009-15
Ward, R. C. (2020). Ward Guide. Available online at: https://www.wardlab.com/resources/ward-guide/ (accessed February 16, 2022).
Weiss, W. P., and Hall, M. B. (2020). Laboratory methods for evaluating forage quality. Forages: Sci. Grassland Agricult, 2, 659–672. doi: 10.1002/9781119436669.ch36
Wickham, H. (2016). ggplot2: Elegant Graphics for Data Analysis. New York, NY: Springer-Verlag. doi: 10.1007/978-0-387-98141-3
Keywords: biostimulant, bacteria, DNA amplicon sequencing, fungi, forage quality, microbial biomass, protozoa, soil
Citation: Carey CJ, Strohm H, Smith F and Biaggi M (2022) Foliar Application of a Microbial Inoculant and Alkali-Extracted Humic Product Boosts Forage Productivity and Quality on a Central Coast California Rangeland. Front. Sustain. Food Syst. 6:847096. doi: 10.3389/fsufs.2022.847096
Received: 01 January 2022; Accepted: 28 February 2022;
Published: 06 April 2022.
Edited by:
Henry Yabbey Sintim, The University of Georgia, United StatesReviewed by:
Utkarsh Manohar Bitla, National Institute of Abiotic Stress Management (ICAR), IndiaElizabeth Temitope Alori, Landmark University, Nigeria
Khurram Shahzad, Lasbela University of Agriculture, Water and Marine Sciences, Pakistan
Nana Kusi, Virginia State University, United States
Copyright © 2022 Carey, Strohm, Smith and Biaggi. This is an open-access article distributed under the terms of the Creative Commons Attribution License (CC BY). The use, distribution or reproduction in other forums is permitted, provided the original author(s) and the copyright owner(s) are credited and that the original publication in this journal is cited, in accordance with accepted academic practice. No use, distribution or reproduction is permitted which does not comply with these terms.
*Correspondence: Chelsea J. Carey, Y2NhcmV5QHBvaW50Ymx1ZS5vcmc=