Rediscovering wild food to diversify production across Australia's agricultural landscapes
- Centre for Tropical Water and Aquatic Ecosystem Research (TropWATER), James Cook University, Townsville, QLD, Australia
Conventional agriculture currently relies on the intensive and expansive growth of a small number of monocultures, this is both risky for food security and is causing substantial environmental degradation. Crops are typically grown far from their native origins, enduring climates, pests, and diseases that they have little evolutionary adaptation to. As a result, farming practices involve modifying the environment to suit the crop, often via practices including vegetation clearing, drainage, irrigation, tilling, and the application of fertilizers, pesticides, and herbicides. One avenue for improvement, however, is the diversification of monoculture agricultural systems with traditional foods native to the area. Native foods benefit from evolutionary history, enabling adaptation to local environmental conditions, reducing the need for environmental modifications and external inputs. Traditional use of native foods in Australia has a rich history, yet the commercial production of native foods remains small compared with conventional crops, such as wheat, barley and sugarcane. Identifying what native crops can grow where would be a first step in scoping potential native food industries and supporting farmers seeking to diversify their cropping. In this study, I modeled the potentially suitable distributions of 177 native food and forage species across Australia, given their climate and soil preferences. The coastal areas of Queensland's wet tropics, south-east Queensland, New South Wales, and Victoria were predicted to support the greatest diversity of native food and forage species (as high 80–120 species). These areas also correspond to the nation's most agriculturally intensive areas, including much of the Murray-Darling Basin, suggesting high potential for the diversification of existing intensive monocultures. Native crops with the most expansive potential distribution include Acacia trees, Maloga bean, bush plum, Emu apple, native millet, and bush tomatoes, with these crops largely being tolerant of vast areas of semi-arid conditions. In addition to greater food security, if diverse native cropping results in greater ecosystem service provisioning, through carbon storage, reduced water usage, reduced nutrient runoff, or greater habitat provision, then payment for ecosystem service schemes could also provide supplemental farm income.
Introduction
With the global population expected to reach nine billion inhabitants by 2050, global food demand is expected to increase by 35–56% by 2050 from 2010 levels (van Dijk et al., 2021). While it is estimated that current food production can meet this demand, challenges exist in ensuring food is adequately distributed to all populations, exacerbated by the climate change driven displacement of people and crops (Schmidhuber and Tubiello, 2007; Wheeler and von Braun, 2013). Achieving global food security is a monumental challenge, dependent on the availability of a diversity of quality foods that are accessible, usable, and stable (Mc Carthy et al., 2018; Kaseva et al., 2019; Barrett, 2021). In addition to removing food trade barriers (Wood et al., 2018; Kinnunen et al., 2020), developing agronomic knowledge on locally-suitable crops can help improve local food sovereignty and buffer against shocks in traded food (Altieri et al., 2012; Leventon and Laudan, 2017; Gliessman et al., 2019). Yet to date, improving food security has been largely reliant on the growth of a low diversity of monocultures. Despite over 50,000 potentially edible plant species globally, only an approximate 300 species are commercially cultivated (Jacques and Jacques, 2012; Massawe et al., 2016), with 90% of global caloric intake is fueled by just 30 crops (Hammer et al., 2003). The global reliance on a low diversity of monocultures puts food security ambitions at risk, particularly with a changing climate (Khoury et al., 2014), and is driving widespread decline in environmental conditions (Altieri, 2009; Stoate et al., 2009; Ramankutty et al., 2018).
With the global food system largely reliant on a small number of crops grown in vast monocultures, many crops are consequently grown well-beyond their natural range and ecosystems (Meyer et al., 2012; Milla et al., 2020). To accommodate this range expansion, landscapes have been heavily modified via the clearing of native vegetation, drainage, irrigation, and application of agrochemicals such as fertilisers, pesticides and herbicides (Altieri, 2009; Stoate et al., 2009; Ramankutty et al., 2018). Native ecosystems within these modified landscapes are often lost completely or disturbed (Dudley and Alexander, 2017). Aquatic systems, for example, face altered hydrology as vegetation coverage and drainage affects rainfall runoff patterns, eutrophication from the losses of fertilizer and livestock excretion, and sedimentation from eroding soils that have reduced vegetative protection (Tilman et al., 2001, 2002a; Awuchi et al., 2020; Gaugler et al., 2020). Terrestrial ecosystems and their species have also been lost or disturbed as the vegetative base of the food web is removed or altered (Tylianakis et al., 2008; Powers and Jetz, 2019). The low diversity of monoculture food systems puts global food security at risk as they have little resilience to climate disruptions (Khoury et al., 2014), pests and diseases (Grab et al., 2018; Ekroth et al., 2019), and nutrient limitations—all reinforcing the need for alteration of farming environments (Altieri, 2009; Crews et al., 2018). While engineering innovations may improve the efficiency of water and agrochemical applications (King, 2017; Shafi et al., 2019), they can be unreliable, resource and energy intensive, vulnerable to outages and extreme weather events, and are often cost-prohibitive to adopt (Tzounis et al., 2017; Shafi et al., 2019). In the USA and Canada, despite heavy investment in improving yields from fertilizer inputs, Hamilton et al. (2013) found no improvement in edible energy efficiency over the past two decades. Put simply, engineering will not alone solve the global issues brought about by mass monoculture. Jevon's Paradox presents an issue for engineering advances, which states that, in the long term, an increase in resource use efficiency will result in increased resource consumption rather than a decrease (Giampietro and Mayumi, 2018). Engineering advances may even exacerbate the ecological impacts of agriculture if farmers use the advances to expand industrial models of agriculture beyond the boundaries of their land's natural capacity (Woodhouse, 2010; Ceddia et al., 2013; Hamilton et al., 2013; Sears et al., 2018; Hamant, 2020).
Creating a sustainable food system will inevitably require the application of ecology to agricultural/food systems or “agroecology” (Gliessman, 2016; Wezel et al., 2020; Bezner Kerr et al., 2021). Rather than having agricultural practices combat natural environments, they will need to work with natural environments and grow a diversity of crops that thrive in those environments (Kremen et al., 2012; Massawe et al., 2016; Crews et al., 2018), as was largely practiced by many indigenous cultures for thousands of years (Singh and Singh, 2017; Suárez-Torres et al., 2017; Figueroa-Helland et al., 2018). Crop diversity can help increase resilience to external stressors, such as extreme weather events and pest and diseases, improve nutrient and water cycling, and help meet nutritional needs through provision a greater richness of phytochemicals (Provenza et al., 2015; Ijaz et al., 2019). Furthermore, using a diversity of crops can help increase the resilience of small-scale landholders (Aguilar-Støen et al., 2009; Meldrum et al., 2018; Ticktin et al., 2018).
In addition to having a diversity of crops, agricultural practices that promote, rather than degrade, the health of agroecosystems would need to be adopted, such as traditional practices that enabled sustained indigenous food production for thousands of years (Critchley et al., 1994; Altieri, 2004; Brookfield and Padoch, 2010), and those promoted through regenerative agriculture (Tilman et al., 2002b; LaCanne and Lundgren, 2018; Lal, 2020; Newton et al., 2020). While traditional agricultural practices are highly varied, some similarities in traditional agroecosystems often exist including: high species diversity; high structural diversity (temporally and spatially); efficient nutrient recycling; complex ecological networks; positive energy efficiency ratios with little to no fossil fuel input; and use of local plants and animals (Altieri, 2004; Gliessman, 2015). Within regenerative agriculture, the aim is to adopt practices that can help regenerate, rather than degrade, the health of agroecosystems and their connected ecosystems, and often include no or low tillage, crop diversity, ecologically-based pest and weed control, restoration of natural habitats, the incorporation of permanent vegetation (such as trees), adaptive multi-paddock grazing and using organic fertilisers (Table 1).
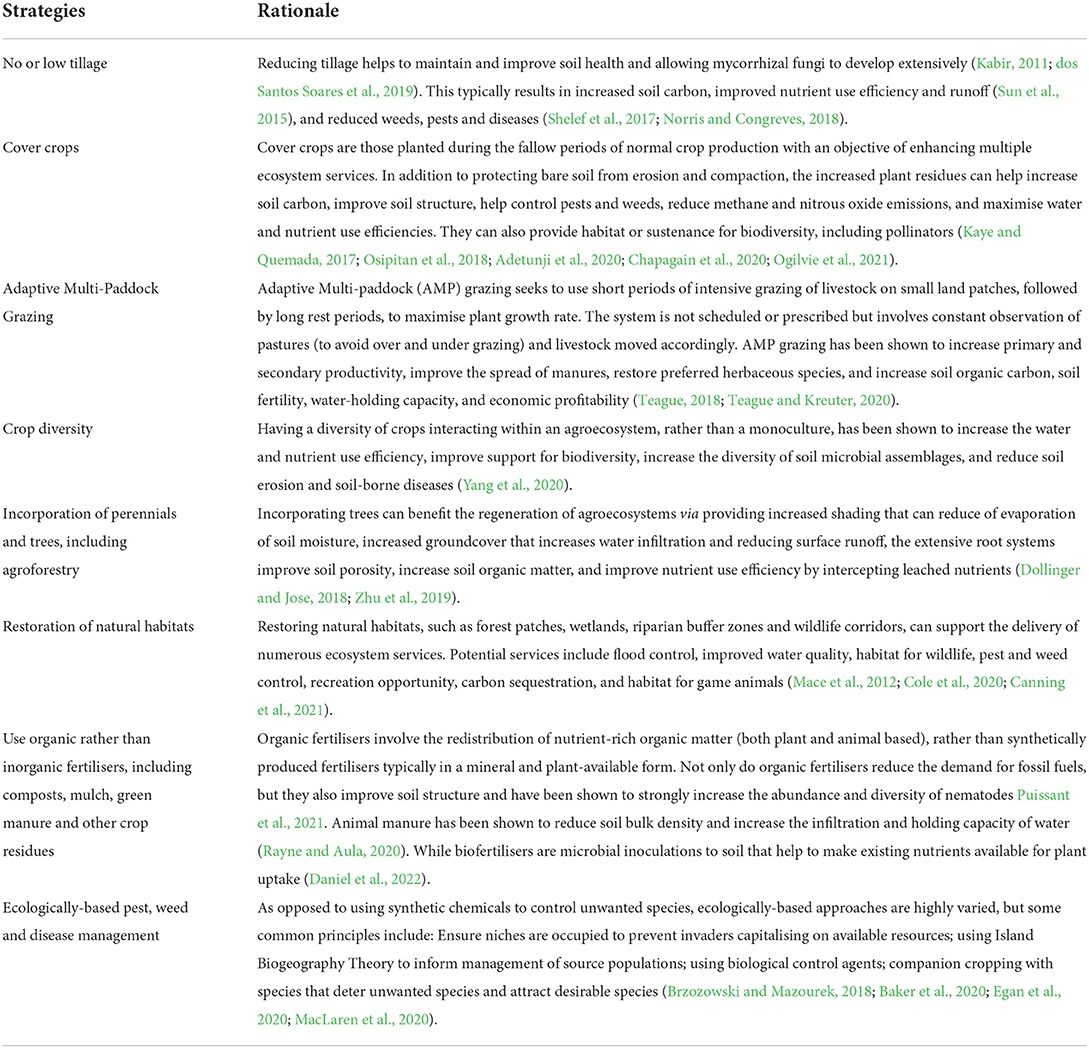
Table 1. Agricultural strategies commonly used in regenerating agroecosystems (LaCanne and Lundgren, 2018).
Diversification could occur by the incorporation of native plants, benefiting from evolution to local environmental conditions (Shelef et al., 2017; Singh and Singh, 2017; Ijaz et al., 2019). Being accustomed to the local environment, native plants have potential for use in no or low tillage strategies, which can maintain and improve soil health (Kabir, 2011; dos Santos Soares et al., 2019), consequently increase soil carbon, improving nutrient use efficiency, reduce runoff (Sun et al., 2015), and reducing weeds, pests and diseases (Shelef et al., 2017).
In Australia, the impacts of farming global staple crops are no different from the rest of the globe. Aquatic ecosystems, such as the Great Barrier Reef (a World Heritage Area) and the Murray-Darling River (the nation's largest freshwater ecosystem), are suffering from the resulting nutrient enrichment, hydrological change, and sedimentation (Pittock et al., 2011; Davis et al., 2016; Brodie et al., 2017). Australia does, however, have a rich history and diverse array of traditional foods or “bush tucker” arising from native plants that hold potential for diversifying agricultural landscapes with locally resilient species (Sultanbawa and Sultanbawa, 2016). For instance, many Australian native plants have evolved to survive and thrive with low phosphorus availability, with sclerophylly, hairy roots and associations with mycorrhizal fungi being common. Some native plants even respond negatively to the addition of phosphorus (Handreck, 1997). Beyond the environmental benefits, there are also potential human, social, cultural, and economic benefits. With respect to human health, an initial screening of 13 native foods found a high diversity of phytochemicals, and the majority having greater availability of minerals than blueberries (a well-recognized health food) (Konczak et al., 2009; Sommano et al., 2013; Richmond et al., 2019). Socially and culturally, the native foods sector also provides an opportunity for Indigenous Australians to use their traditional knowledge systems, including long-developed social and environmental management practices, to create new livelihoods and enterprises (Buchanan, 2014; Laurie, 2020; Jarvis et al., 2022).
Realizing Australia's native food potential, however, requires substantial knowledge gaps to be filled to inform financial decisions. Key gaps include understanding what species can grow where, growth and production rates, efficacy of propagation techniques, market and value-adding opportunities, industry structure and size, industry risks and limitations, potential Indigenous business models, and methods to best mobilise knowledge (Clarke, 2012; Jarvis et al., 2022).
In this study, I seek to inform the gap of what species can grow where, by using modelling to identify land parcels with soil and climate characteristics likely to support the growth of an array of bush tucker plants. This would enable farmers to identify what native crops could be supported within the natural capacity of their farming system, and help agricultural industries scope the production potential of different native crops.
Methods
Species data
A compilation of 177 terrestrial native plant species, excluding grasses, with known use potential for producing human foods or livestock forage was compiled from the literature (Dear et al., 2008; Konczak et al., 2009; Revell et al., 2013; Sultanbawa and Sultanbawa, 2016; Isaacs, 2019; Richmond et al., 2019). For all species identified (Supplementary Table S1), all observations (including location) from across Australia were extracted from the Atlas of Living Australia (ALA), yielding 647,180 records. ALA is a large database that collates sightings of animals from a wide range of organisations and contributors (Belbin and Williams, 2016). Given the likely differences in survey method and intensity among observers (Canning and Waltham, 2021), which could reduce the reliability of abundance data, this analysis only examined the presence of a species, rather than the abundance. Furthermore, surveys could not be used to indicate species absence. Nonetheless, the ALA dataset represents the most comprehensive observation dataset over the entire spatial extent and is, therefore, the best data available for this analysis.
Environmental variables
At all observation locations, statistics for 19 climate variables were extracted from the WorldClim2 database (Fick and Hijmans, 2017), and 12 soil variables from the Soil and Landscape Grid of Australia (SLGA; Table 1; Grundy et al., 2015; Viscarra Rossel et al., 2015).
WorldClim 2 provides 19 climate metrics for baseline conditions (Table 2), using long-term average between 1970 and 2000, from between 9,000 and 60,000 weather stations, that were then interpolated to provide global coverage at 1 km2 resolutions (Fick and Hijmans, 2017). Globally, the cross-validated correlations on baseline data were 0.86 for precipitation, 0.76 for wind speed, and ≥ 0.99 for temperature and humidity, though there is regional variation between models and parameters (Fick and Hijmans, 2017).
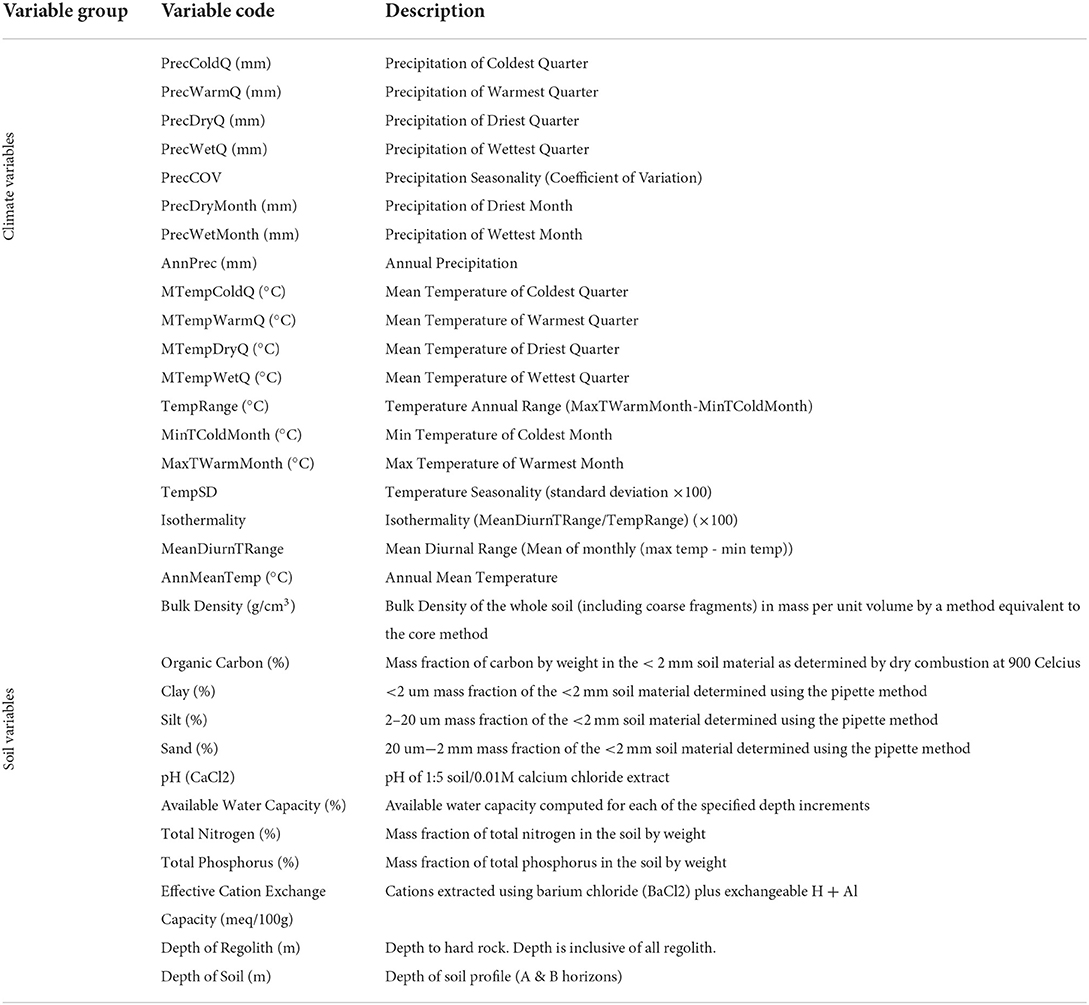
Table 2. The climatic variables (Fick and Hijmans, 2017) and the soil variables (Grundy et al., 2015) used in random forest modelling to predict the potential distributions of bush foods across Australia.
The SLGA provides Australia-wide coverage of 11 continuous soil attributes at 0.008 km2 (90 x 90 m) resolutions (Table 2), across regolith depths between 0 and 2 m (Grundy et al., 2015; Viscarra Rossel et al., 2015), with predictions conforming to the GlobalSoilMap specifications (Arrouays et al., 2014). For this study, only those for regolith depths between 30 and 60 cm were extracted. The SLGA three-dimensional soil maps were derived from spatial models informed by 281,202 soil profiles in the national soil visible–near infrared database (NSVNIRD) and 1,315 sites from the national soil visible–near infrared database (Viscarra Rossel et al., 2015). Across all attributes mapped, models between 30 and 70% of their total variation, with near-surface estimates typically having greater accuracy with more training data (Viscarra Rossel et al., 2015).
Random forest models
Random forests are a machine learning method that uses a collection of regression trees, whereby each tree is fitted to a bootstrapped sample (with replacement) and then validated on the out-of-bag sample (Breiman, 2001). Random forest predictions are the average of the predictions of each tree. Regression trees, and consequently random forests, work by partitioning observations at splits of predictors that minimise the sum of squares error. They have a high level of flexibility, can handle non-linear relationships and complex interactions, and do not require cross-validation or a separate testing dataset as each tree is constructed using a different bootstrap sample (Cutler et al., 2007; Hastie et al., 2009; Ellis et al., 2012).
Random Forests were used to model the probability of occurrence for all species (Supplementary Table S1) based on all the climatic and soil characteristics (Table 2), relative to a random background, using the “randomForest” function (trees=500) from the randomForest package in R (Liaw and Wiener, 2002; R Core Team, 2016). As the occurrence data used was presence-only, and could not indicate absence, an equal number of random background locations were used instead of absences, to achieve a balanced presence-background predictive model. Model performance was assessed by calculating the area under the receiver operating curve (AUC-ROC), calculated using the ‘auc’ function from the pROC package (Robin et al., 2011). According to Šimundić (2009), AUC-ROC between 0.7 and 0.8 indicate good diagnostic accuracy, while 0.8–0.9 indicates very good, and 0.9–1.0 indicates excellent accuracy. The “importance” function, from the randomForest package (Liaw and Wiener, 2002), then used to identify the globally important variables, which measure the decrease in Gini index from splitting on each variable, averaged over all trees.
Predicting species suitability across australia
Using the random forest models, the suitability of each species (where AUC-ROC >0.7) was predicted across the entirety of Australia using the soil and climate data extracted from the centroids of 18,827 polygons. Polygons were derived by intersect the 419 bio-subregion polygons, as mapped by the Interim Biogeographic Regionalisation for Australia (Thackway and Cresswell, 1995; Cummings and Hardy, 2000; IBRA, 2020), and soil order polygons according to the Australian Soil Classification (McKenzie et al., 2012). The IBRA (V7.0) classifies Australia's landscape into 89 bioregions and 419 subregions, based on climate, geology, landform, and native species presence (Thackway and Cresswell, 1995; Cummings and Hardy, 2000), and is endorsed by all levels of government for use under Australia's Strategy for the National Reserve System 2009–2030. IBRA is a landscape-scale classification, and the intersection with soil order provides a greater resolution.
Results
Of the initial 177 species identified, 150 species (225 taxonomic groups including subspecies and varieties) had sufficient observations, model accuracy and suitability predicted across Australia (Supplementary Table S2). In general, across all species models, the maximum temperature of the warmest month and quarter, precipitation of the driest month and quarter, and precipitation of the coldest quarter, were the most important climatic and soil variables, though substantial differences in variable importance were observed between species (Supplementary Table S2).
Across the nation, the diversity of potentially suitable native crops ranged from 0 to 120 for a given parcel (Figure 1). Areas with the climate and soil characteristics with potential for supporting the highest diversity of native crops include the coastal areas of New South Wales, Victoria and northern Tasmania, and in Queensland's Wet Tropics (Figure 1). While the central and mid-south areas of Western Australia had the lowest potential diversity of native crops.
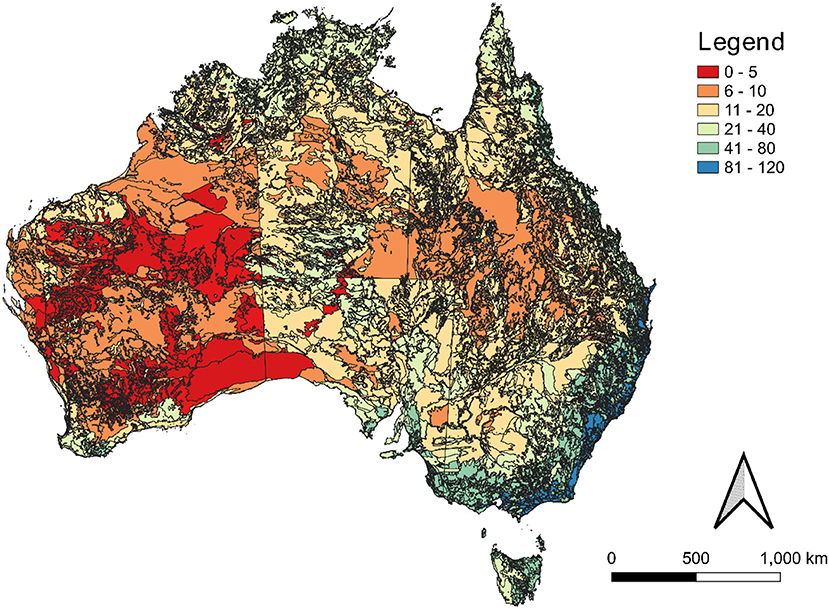
Figure 1. The potential number of native plant food and forage species predicted to be suitable across Australia, with locations delineated by soil order mapping and IBRA mapping (McKenzie et al., 2012; IBRA, 2020).
The native crops with the most expansive potential distribution include Acacia trees (Acacia spp.), Maloga bean (Vigna lanceolata), bush plum (Carissa spinarum), Emu apple (Owenia acidula), native millet (Panicum decompositum), and bush tomatoes (Solanum spp; Supplementary Table S2).
Of the six native crops most commonly used and in demand (Robbins, 2008), Lemon Myrtle (Backhousia citriodora) is predicted to be suitable along the east coast (Figure 1A), Native Citrus (Citrus spp.) across south-east Queensland and central New South Wales (Figure 1B), Davidson Plum (Davidsonia spp.) across northern coastal Queensland, coastal New South Wales and Tasmania (Figure 1C), Quandong (Santalum acuminatum) across southern areas of west Western Australia, South Australia and Victoria (Figure 1D), Bush Tomato (Solanum centrale) across central Western Australia and Northern Territory (Figure 1E), and Native Pepper (Tasmannia lanceolata) across southern New South Wales and Tasmania (Figure 1F).
Given the need to urgently reduce nutrient and sediment losses from the sugarcane-growing regions of the Great Barrier Reef catchment, I also compiled a list a species predicted to be widely suitable across the intensive sugarcane-growing areas of the Burdekin region (dry tropical climate) and the Ingham (wet tropical climate) regions (Table 3). For each species, I have indicated the type of edible produced, approximate forest strata position, approximate growth rate, and whether it is a legume, to help inform agroforestry, including syntropics, and farm planning. Within the Ingham region there were 32 widely suitable terrestrial bush foods, while there were 18 across the lower Burdekin region (Table 3).
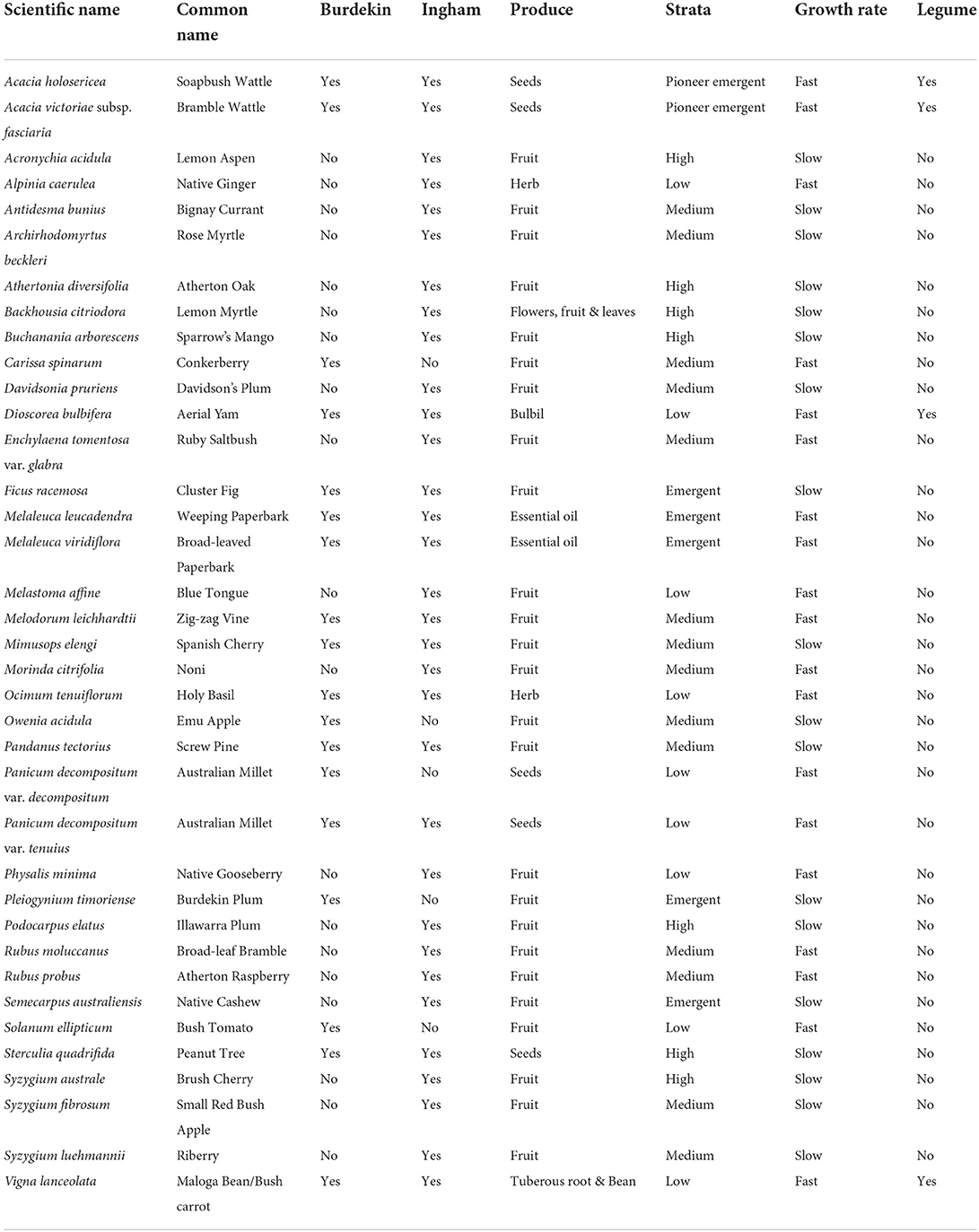
Table 3. Bush food species predicted to be widely suitable across the sugarcane-growing areas of the Burdekin (dry tropical climate) and Ingham (wet tropical climate) regions of the Great Barrier Reef catchment, along with the type of edible produce, approximate forest strata position, indicative growth rate, and whether the species is a legume.
Discussion
Modelling of bush foods
Diversifying agricultural landscapes with native foods provides an avenue to improve the resilience and ecological values of contemporary agricultural systems dominated by monocultures. Here I modelled the potential suitability of land parcels across Australia to support 177 native crops, with the majority of species (150) having good to excellent diagnostic accuracy. The probability of suitability for most species was primarily influenced the maximum temperatures and rainfall. While it is not surprising that these climatic variables are influential in predicting diversity, it may also be possible that other factors are driving the patterns, such as energetic and landscape characteristics, which appear visually correlative (Venevsky and Veneskaia, 2003), and evolutionary histories (Martin, 2006; Cowling et al., 2015). Nonetheless, the present study focused on identifying potentially suitable land parcels for native crops, not the drivers of diversity, and the high performance of the models permits land identification. While the land parcels presented here are large-scale, the models can also be used to predict at higher resolutions, such as the farm-scale. It is also likely that this study has not encapsulated all potential native foods, with traditional knowledge systems likely to inform gaps. Furthermore, most work scoping native shrubs for livestock forage has focused on southern Australia, with northern Australia requiring substantially more work (Dear et al., 2008; Monjardino et al., 2010; Revell et al., 2013). As knowledge on additional species, and new observations arise, the modelling framework applied here should be updated in time to include additional the information. Further work should also be undertaken to scope the use of native plants for fibres, and the use of native aquatic plants.
National-scale diversity patterns
Essentially all the major agricultural regions that currently support intensive monoculture systems were suitable for supporting between 20 and 120 species, with the coastal areas of Queensland's wet tropics, south-east Queensland, New South Wales, and Victoria having the most versatile land parcels. While the inland desert areas of Western Australia were predicted to have the least potential diversity.
Those with the most expansive potential distributions are also drought resilient species, such as Acacias, native millet, and bush tomatoes, and are found across areas of central Australia. With the two most expansive groups, Acacia and Maloga bean, also both being legumes and can support nitrogen fixation. While much of central Australia is arid or semi-arid, vegetation present in these areas can be traced back to when central Australia was humid and supported rainforest, with the few species remaining being those that could tolerate or adapt to dry climates and infertile soils (Martin, 2006). As 70% of Australia is arid or semi-arid with soils of poor fertility, species that can survive in dry climates and mitigate nutrient deficiencies, have a much greater potential range than those dependent on regular rainfall and fertile soils (Stafford Smith and Morton, 1990).
Murray-darling basin
The Murray-Darling Basin has experienced substantial hydrological alterations to support cropping and survival through dry periods (Leblanc et al., 2012), with large water demands causing not only declines in freshwater ecological health, but major social and cultural impacts, including the loss of livelihoods for indigenous communities, as well as public resources lost to buying back water for environmental flows (Davies et al., 2010; Connell and Grafton, 2011). Within the Murray-Darling basin, there was a high diversity of potential native crops predicted, meaning there is also large opportunity for native crop integration to be a strong measure towards improving the Basin's freshwater ecosystems, which has been given little consideration to date (Pittock et al., 2011). Vegetation native to the Basin have been shown to be highly resilient to prolonged extreme drought (Capon and Reid, 2016). Consequently, a landscape dominated by a diversity of crops native to the basin are unlikely to demand the same volume of water as existing crops (Miers, 2004; Ryder et al., 2008; Sultanbawa and Sultanbawa, 2016), allowing more natural hydrological regimes to improve river health (Pittock et al., 2011).
Furthermore, five of the six native foods identified by Robbins (2008) that are already commercially grown, with developed agronomy, in demand and with growth potential, are also suitable within the Basin. These include Lemon Myrtle, Native Citrus, Davidson Plum, Quandong (a tart-tasting, dry textured fruit), and Native Pepper (Figure 2). However, focussing solely on these five crops may lead to non-native monocultures simply being replaced by native monocultures that are devoid of the benefits of multi-species crops and do little to improve the environmental woes of the present agricultural system. Instead, these crops should be grown in combination with other native crops suitable within the same land parcel, which will require further investigations into the design of native agroforestry regimes.
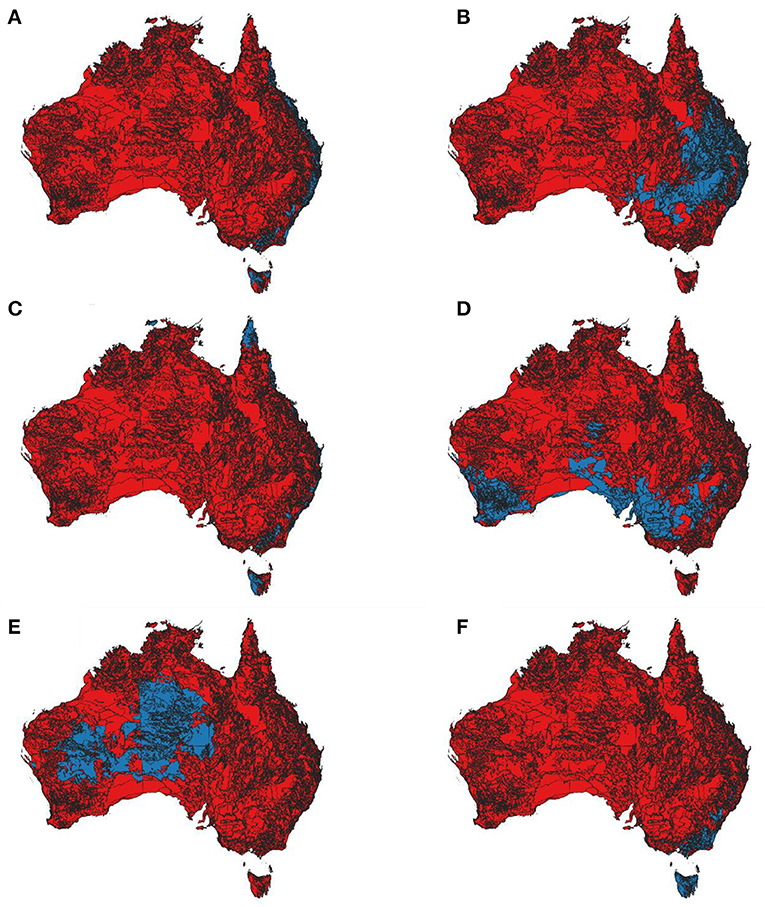
Figure 2. Predicted suitability of the six native crops most used and in demand (Robbins, 2008). These include (A) Lemon Myrtle (Backhousia citriodora); (B) Native Citrus (Citrus spp.); (C) Davidson Plum (Davidsonia spp.); (D) Quandong (Santalum acuminatum); (E) Bush Tomato (Solanum centrale), and (F) Native Pepper (Tasmannia lanceolata). Blue indicated the suitable environmental range, while red indicates the unsuitable range, with locations delineated by soil order mapping and IBRA mapping (McKenzie et al., 2012; IBRA, 2020).
Great Barrier Reef region
In the Great Barrier Reef region, the Queensland Government has a target to reduce dissolved inorganic nitrogen (DIN) and sediment losses to the reef by 60% and 25% respectively by 2025 to protect reef health (Reef 2050 Water Quality Improvement Plan). Despite only occupying 1.3% of the catchment area, DIN from sugarcane fertiliser losses accounts for 42% of the DIN exported to the reef (McCloskey et al., 2021). Transitioning to multi-species, multi-story agroforestry growing bush foods could be one option for reducing these losses. As an example, in Table 3 I showcase the bush foods predicted to be widely suitable across the intensive sugarcane growing areas of the Ingham (wet tropics) and Burdekin (dry tropics) regions, for which 32 and 18 species were identified, respectively. I also emphasise the word transition, as for many a large-scale transition from sugarcane to a multi-species native food cropping system, that at present has little industry and market, would be unfeasible. The transition could begin with small-scale trials where native foods are incorporated within existing sugarcane systems, either intercropped or grown during the rotation's fallow. A suitable intercrop would be low growing to prevent shading sugarcane, fast growing to recover from sugarcane harvesting damage every 12–18 months, and easily harvested in a way that does not damage sugarcane. Options to explore could include aerial yam, blue tongue, holy basil, and Australian millet. During the fallow period, maloga bean/bush carrot could be grown as a leguminous cash crop. Not only would a maloga bean rotation fix nitrogen for the next sugarcane crop, thereby reducing fertiliser demands, but it could potentially also reduce the pressure of lesion nematodes. Recent trials within the region found that leguminous rotation crops significantly reduced Lesion nematodes (Pratylenchus zeae) relative to continuous sugarcane cropping (Haplin et al., 2020). If, however, a new agroecological system was to be developed with agroforestry, rather than an intercropped-sugarcane system, then a greater diversity of species could be incorporated as species across all strata and growth rates could be included.
The need to identify suitable crop combinations
In developing successful agroforestry regimes, it will be necessary to further investigate the within-forest ecological requirements (e.g., light, and mutualistic relationships), growth rates and nutrient demands. To further assist with planning suitable regimes, growth models could be incorporated into an easy-to-use modelling platform, similar to the Agroforestry Design Tool in the USA (Elevitch and Logan, 2021). Understanding the strategies each species uses to survive in their local environment and potential multi-species crop assemblages will also be necessary for successful cultivation. As the plants are derived from natural ecosystems, it is likely that many will be co-dependent on other species. For example, Quandongs are hemi-parasitic, relying on a host plant, for water and nutrients. In the natural environment, they tend to rely on nitrogen fixing trees, such as Acacia and Casuarina, and usually have multiple hosts simultaneously (Australian National Botanic Gardens and Centre for Australian National Biodiversity Research, 2012). Failure to identify dependencies and suitable grafting techniques could result in a failure to thrive in a farmed environment. However, by growing Quandongs with Acacia, then not only can native foods be sourced from both species, but the nitrogen-fixation by mycorrhizal bacteria in Acacia can help minimize dependence on fertilizers. This is an example of just one simple multi-species synergy, with many more potential combinations possible. Combinations will need to be specific to the location and objectives as not all species are suitable at all locations. Attempts to grow crops out of their suitable habitat will likely result in crop failure and reliance on environmental alterations and external inputs (Ryder et al., 2008; Sultanbawa and Sultanbawa, 2016). While this is no small task, traditional knowledge by indigenous who have a long history of cultivating native foods can provide a foundation to build from Bliege Bird et al. (2008), Gerritsen (2010), Paterson (2018).
Indigenous business opportunities
Indigenous traditional knowledge also provides an opportunity for the development of culturally appropriate and Indigenous-led business and supply chain models within the emerging bush foods sector (Jarvis et al., 2022). Traditional knowledge often constitutes substantial Indigenous cultural and intellectual property (ICIP), developed and passed on from generation to generation. While the use of Indigenous knowledge is vital for sector development, the protection, use and accessibility of ICIP needs to be balanced with benefits derived from using the ICIP commercially. Managing ICIP challenges may be done within existing intellectual property legislation but will also need to consider the place-based nature of ICIP (Jarvis et al., 2022). IP Australia, an Australian Government entity, seek to protect the Indigenous Knowledge of Aboriginal and Torres Strait Islanders (IP Australia, 2021), and suggest four actions that need advancing: (1) Establishing an Indigenous Advisory Panel to provide a formalised Indigenous voice to IP Australia; (2) Enhance the trademarks and designs systems to prevent rights being granted over IK in circumstances that Aboriginal or Torres Strait Islander people or communities consider is inappropriate, unfair or offensive; (3) Introduce new requirements to declare the source of Indigenous Knowledge used in new innovations; and (4) Introduce labelling schemes that distinguish authentic Aboriginal or Torres Strait Islander goods (IP Australia, 2021). Researchers should also aim to ensure that partnering with Indigenous does not simply result in the extraction of Indigenous Knowledge but is collaborative, and that all partners co-design, co-conduct, co-govern, and co-author research projects (Maclean et al., 2021).
Ecosystem service markets for supplemental income
One of the largest barriers for new entrants into Australia's native foods market is the lack of developed industries and heightened financial risk. One way to potentially hedge bets and reduce risk would be by also diversifying income streams. Given that diverse agroforestry regimes can also deliver substantial ecosystem services (the benefits nature provides to humans) when compared with conventional agriculture (Idol et al., 2011; Torralba et al., 2016; Kay et al., 2019), then native agroforestry farms could also consider designs that have the potential to attract payments for providing ecosystem services (PES) (Farley and Costanza, 2010; Rodríguez-Ortega et al., 2018; Salzman et al., 2018; Canning et al., 2021). Across Australia, the most prevalent PES is the Carbon Emissions Reduction Fund, which makes payments to farmers for the generation of carbon credits (representing the storage or reduction of carbon). Credits are derived from the adoption of new agronomic practices and systems, including increasing soil carbon, above-ground vegetation, and reducing livestock emissions, beyond that expected from baseline farming conditions. For example, a case study in Northern Territory, demonstrated that in the top 1m of soil, grassland sites stored approximately 25 t C/ha, while sites with trees remaining (tropical open forest savannah) stored between 30 and 70 t C/ha (Chen et al., 2005; Australian Government Chief Scientist, 2009). Excluding the aboveground carbon storage, the difference of 5-45 t C/ha, at an approximate carbon trading price of $19 AUD/t C (June 2021 quarter, based on Australian Carbon Credit Unit trading), corresponds to an additional value between $95-855 AUD 2021 at the open savannah sites than the grassland sites (Australian Government Clean Energy Regulator, 2021). This represents a substantial increase in value, particularly when applied across large cattle stations (>1,000–10,000 ha). In the Great Barrier Reef catchment, schemes are emerging that fund farmers for adopting practices that reduce nutrient runoff or provide habitat for Cassowaries (Casuarius spp.) (Eco-Markets Australia, 2020; Terrain Natural Resource Management, 2020). In the Murray-Darling Basin, markets exist to fund farmers for the better management of water and supporting flow regulation (Wheeler et al., 2014; Settre et al., 2019). While PES opportunities are increasing, maximising participation will require easy access, low administration and assessment burden, clear assessment methodologies, strong guidance on permanence requirements, and the ability to bundle payments from multiple services (Farley and Costanza, 2010; Rodríguez-Ortega et al., 2018; Salzman et al., 2018; Canning et al., 2021). Payments for ecosystem services not only helps to diversify income and improve financial stability, but they help support farming systems that advance, rather than erode, progress towards critical environmental aspirations.
Conclusion
Conventional agriculture in Australia, like many parts of the world, is dominated by extensive monocultures is a key driver of biodiversity loss, climate change, alteration of hydrological cycles, and the sedimentation and eutrophication of aquatic ecosystems. Here I used models of environmental suitability for 177 native bush foods to scope the potential for a diverse bush foods sectors underpinned by diverse native agroforestry. Fortunately, the regions potentially supporting the greatest richness of bush food species are also those currently most at-stress from intensive monocultured agriculture, such as the Murray-Darling basin and the Great Barrier Reef catchment, meaning there is ample scope in those regions to transform the agricultural landscapes with a diversity of native foods using regenerative practices. This form of agriculture has the potential to support new Indigenous-led business models and attract supplemental income from the provision of ecosystem services. Although further work is required to better understand crop combinations, cultivation methods and to develop markets, a stark change in current agriculture is needed, and the potential benefits for humanity and the ecosystems we are part of are vast.
Data availability statement
The original contributions presented in the study are included in the article/Supplementary material, further inquiries can be directed to the corresponding author/s.
Author contributions
AC conceived the idea, carried out the analysis, and wrote the manuscript.
Acknowledgments
The author thanks Marnie Prickett for her feedback on a draft version of this manuscript, and the two reviewers for their constructive feedback.
Conflict of interest
The author declares that the research was conducted in the absence of any commercial or financial relationships that could be construed as a potential conflict of interest.
Publisher's note
All claims expressed in this article are solely those of the authors and do not necessarily represent those of their affiliated organizations, or those of the publisher, the editors and the reviewers. Any product that may be evaluated in this article, or claim that may be made by its manufacturer, is not guaranteed or endorsed by the publisher.
Supplementary material
The Supplementary Material for this article can be found online at: https://www.frontiersin.org/articles/10.3389/fsufs.2022.865580/full#supplementary-material
References
Adetunji, A. T., Ncube, B., Mulidzi, R., and Lewu, F. B. (2020). Management impact and benefit of cover crops on soil quality: a review. Soil. Tillage. Res 204, 104717. doi: 10.1016/j.still.2020.104717
Aguilar-Støen, M., Moe, S. R., and Camargo-Ricalde, S. L. (2009). Home gardens sustain crop diversity and improve farm resilience in Candelaria Loxicha, Oaxaca, Mexico. Hum. Ecol. 37, 55–77. doi: 10.1007/s10745-008-9197-y
Altieri, M. A. (2004). Linking ecologists and traditional farmers in the search for sustainable agriculture. Front. Ecol. Environ. 2, 35–42. doi: 10.1890/1540-9295(2004)0020035:LEATFI2.0.CO;2
Altieri, M. A. (2009). The Ecological Impacts of Large-Scale Agrofuel Monoculture Production Systems in the Americas: Bull. Sci. Technol. Soc. 29, 236–244. doi: 10.1177/0270467609333728
Altieri, M. A., Funes-Monzote, F. R., and Petersen, P. (2012). Agroecologically efficient agricultural systems for smallholder farmers: contributions to food sovereignty. Agron. Sustain. Dev. 32, 1–13. doi: 10.1007/s13593-011-0065-6
Arrouays, D., Grundy, M. G., Hartemink, A. E., Hempel, J. W., Heuvelink, G. B. M., Hong, S. Y., et al. (2014). GlobalSoilMap: Toward a Fine-Resolution Global Grid of Soil Properties. Adv. Agron. 125, 93–134. doi: 10.1016/B978-0-12-800137–0.00003-0
Australian Government Chief Scientist (2009). Which plants store more carbon in Australia: forests or grasses? | Chief Scientist. Available online at: https://www.chiefscientist.gov.au/2009/12/which-plants-store-more-carbon-in-australia-forests-or-grasses (accessed January 29, 2022).
Australian Government Clean Energy Regulator (2021). Quarterly Carbon Market Report: June Quarter 2021. Canberra, Australia. Available online at: http://www.cleanenergyregulator.gov.au/DocumentAssets/Documents/Quarterly%20Carbon%20Market%20Report%20-%20June%20Quarter%202021.pdf (accessed January 29, 2022).
Australian National Botanic Gardens and Centre for Australian National Biodiversity Research (2012). Santalum acuminatum—Growing. Native Plants. Canberra, ACT: Australian Government.
Awuchi, C. G., Awuchi, C. G., Ukpe, A. E., Asoegwu, C. R., Uyo, C. N., Ngoka, K. E., et al. (2020). Environmental impacts of food and agricultural production: a systematic review. Eur. Acad. Res 8, 1120–1135. Available online at: https://euacademic.org/UploadArticle/4384.pdf
Baker, B. P., Green, T. A., and Loker, A. J. (2020). Biological control and integrated pest management in organic and conventional systems. Biol. Control 140, 104095. doi: 10.1016/j.biocontrol.2019.104095
Barrett, C. B. (2021). Overcoming global food security challenges through science and solidarity. Am. J. Agric. Econ 103, 422–447. doi: 10.1111/ajae.12160
Belbin, L., and Williams, K. J. (2016). Towards a national bio-environmental data facility: experiences from the Atlas of Living Australia. Int. J. Geogr. Inf. Sci. 30, 108–125. doi: 10.1080/13658816.2015.1077962
Bezner Kerr, R., Madsen, S., Stüber, M., Liebert, J., Enloe, S., Borghino, N., et al. (2021). Can agroecology improve food security and nutrition? A review. Glob. Food. Sec 29, 100540. doi: 10.1016/j.gfs.2021.100540
Bliege Bird, R., Bird, D. W., Codding, B. F., Parker, C. H., and Jones, J. H. (2008). The “fire stick farming” hypothesis: Australian Aboriginal foraging strategies, biodiversity, and anthropogenic fire mosaics. Proc. Natl. Acad. Sci. 105, 14796–14801. doi: 10.1073/pnas.0804757105
Brodie, J. E., Lewis, S. E., Collier, C. J., Wooldridge, S., Bainbridge, Z. T., Waterhouse, J., et al. (2017). Setting ecologically relevant targets for river pollutant loads to meet marine water quality requirements for the Great Barrier Reef, Australia: a preliminary methodology and analysis. Ocean. Coast. Manag. 143, 136–147. doi: 10.1016/j.ocecoaman.2016.09.028
Brookfield, H., and Padoch, C. (2010). Appreciating agrodiversity: a look at the dynamism and diversity of indigenous farming practices. Environment 36, 6–45. doi: 10.1080/00139157.1994.9929164
Brzozowski, L., and Mazourek, M. (2018). A Sustainable agricultural future relies on the transition to organic agroecological pest management. Sustainability 10, 2023. doi: 10.3390/su10062023
Buchanan, G. (2014). Hybrid economy research in remote Indigenous Australia: seeing and supporting the customary in community food economies. Local Environ. 19, 10–32. doi: 10.1080/13549839.2013.787973
Canning, A., and Waltham, N. (2021). Ecological impact assessment of climate change and habitat loss on wetland vertebrate assemblages of the Great Barrier Reef catchment and the influence of survey bias. Ecol Evol 11, 5422–5254. doi: 10.1002/ece3.7412
Canning, A. D., Jarvis, D., Costanza, R., Hasan, S., Smart, J., Finisdore, J., et al. (2021). Financial incentives for large-scale wetland restoration: beyond markets to common asset trusts. One. Earth 4, 937–950. doi: 10.1016/j.oneear.2021.06.006
Capon, S. J., and Reid, M. A. (2016). Vegetation resilience to mega-drought along a typical floodplain gradient of the southern Murray-Darling Basin, Australia. J. Veg. Sci. 27, 926–937. doi: 10.1111/jvs.12426
Ceddia, M. G., Sedlacek, S., Bardsley, N. O., and Gomez-y-Paloma, S. (2013). Sustainable agricultural intensification or Jevons paradox? The role of public governance in tropical South America. Global. Environ. Change 23, 1052–1063. doi: 10.1016/j.gloenvcha.2013.07.005
Chapagain, T., Lee, E. A., and Raizada, M. N. (2020). The potential of multi-species mixtures to diversify cover crop benefits. Sustainability 12, 2058. doi: 10.3390/su12052058
Chen, X., Hutley, L. B., and Eamus, D. (2005). Soil organic carbon content at a range of north Australian tropical savannas with contrasting site histories. Plant Soil 268, 161–171. doi: 10.1007/s11104-004-0249-9
Cole, L. J., Stockan, J., and Helliwell, R. (2020). Managing riparian buffer strips to optimise ecosystem services: a review. Agric. Ecosyst. Environ 296, 106891. doi: 10.1016/j.agee.2020.106891
Connell, D., and Grafton, R. Q. (2011). Water reform in the Murray-Darling Basin. Water. Resour. Res 47. doi: 10.1029/2010WR009820
Cowling, R. M., Potts, A. J., Bradshaw, P. L., Colville, J., Arianoutsou, M., Ferrier, S., et al. (2015). Variation in plant diversity in mediterranean-climate ecosystems: the role of climatic and topographical stability. J. Biogeogr. 42, 552–564. doi: 10.1111/jbi.12429
Crews, T. E., Carton, W., and Olsson, L. (2018). Is the future of agriculture perennial? Imperatives and opportunities to reinvent agriculture by shifting from annual monocultures to perennial polycultures. Global Sustain. 1. doi: 10.1017/sus.2018.11
Critchley, W. R. S., Reij, C., and Willcocks, T. J. (1994). Indigenous soil and water conservation: a review of the state of knowledge and prospects for building on traditions. Land. Degrad. Dev. 5, 293–314. doi: 10.1002/ldr.3400050406
Cummings, B., and Hardy, A. (2000). Revision of the Interim Biogeographic Regionalisation for Australia. (IBRA) and Development of Version. 5, 1. Canberra, Australia.
Cutler, D. R., Edwards, T. C. Jr, Beard, K. H., Cutler, A., Hess, K. T., Gibson, J., et al. (2007). Random forests for classification in ecology. Ecology 88, 2783–2792. doi: 10.1890/07-0539.1
Daniel, A. I., Fadaka, A. O., Gokul, A., Bakare, O. O., Aina, O., Fisher, S., et al. (2022). Biofertilizer: the future of food security and food safety. Microorganisms 10, 1220. doi: 10.3390/microorganisms10061220
Davies, P. E., Harris, J. H., Hillman, T. J., Walker, K. F., Davies, P. E., Harris, J. H., et al. (2010). The Sustainable Rivers Audit: assessing river ecosystem health in the Murray–Darling Basin, Australia. Mar. Freshw. Res. 61, 764–777. doi: 10.1071/MF09043
Davis, A. M., Pearson, R. G., Brodie, J. E., Butler, B., Davis, A. M., Pearson, R. G., et al. (2016). Review and conceptual models of agricultural impacts and water quality in waterways of the Great Barrier Reef catchment area. Mar. Freshw. Res 68, 1–19. doi: 10.1071/MF15301
Dear, B. S., Ewing, M. A., Dear, B. S., and Ewing, M. A. (2008). The search for new pasture plants to achieve more sustainable production systems in southern Australia. Aust. J. Exp. Agric 48, 387–396. doi: 10.1071/EA07105
Dollinger, J., and Jose, S. (2018). Agroforestry for soil health. Agrofor. Syst. 92, 213–219. doi: 10.1007/s10457-018-0223-9
dos Santos Soares, D., Ramos, M. L. G., Marchão, R. L., Maciel, G. A., de Oliveira, A. D., Malaquias, J. V., et al. (2019). How diversity of crop residues in long-term no-tillage systems affect chemical and microbiological soil properties. Soil. Tillage. Res 194, 104316. doi: 10.1016/j.still.2019.104316
Dudley, N., and Alexander, S. (2017). Agriculture and biodiversity: a review. Biodiversity 18, 45–49. doi: 10.1080/14888386.2017.1351892
Eco-Markets Australia (2020). Reef Credit as Market-Based Incentive Mechanism - Eco-Markets Australia. Available online at: https://eco-markets.org.au/reef-credits/ (accessed January 24, 2022).
Egan, P. A., Dicks, L. v., Hokkanen, H. M. T., and Stenberg, J. A. (2020). Delivering Integrated Pest and Pollinator Management (IPPM). Trends. Plant. Sci. 25, 577–589. doi: 10.1016/j.tplants.2020.01.006
Ekroth, A. K. E., Rafaluk-Mohr, C., and King, K. C. (2019). Host genetic diversity limits parasite success beyond agricultural systems: a meta-analysis. Proc. Royal Soc. B 286, 20191811. doi: 10.1098/rspb.2019.1811
Elevitch, C., and Logan, N. (2021). Agroforestry Design Tool—AgroforestryX. Available online at: http://agroforestryX.com
Ellis, N., Smith, S. J., Pitcher, C. R., Roland Pitcher, C., and Pitcher, C. R. (2012). Gradient forests: calculating importance gradients on physical predictors. Ecology 93, 156–168. doi: 10.1890/11-0252.1
Farley, J., and Costanza, R. (2010). Payments for ecosystem services: From local to global. Ecol. Econ. 69, 2060–2068. doi: 10.1016/j.ecolecon.2010.06.010
Fick, S. E., and Hijmans, R. J. (2017). WorldClim 2: new 1-km spatial resolution climate surfaces for global land areas. Int. J. Climatol. 37, 4302–4315. doi: 10.1002/joc.5086
Figueroa-Helland, L., Thomas, C., and Aguilera, A. P. (2018). Decolonizing food systems: food sovereignty, indigenous revitalization, and agroecology as counter-hegemonic movements. Perspect. Global. Dev. Technol. 17, 173–201. doi: 10.1163/15691497-12341473
Gaugler, T., Stoeckl, S., and Rathgeber, A. W. (2020). Global climate impacts of agriculture: a meta-regression analysis of food production. J. Clean. Prod 276, 122575. doi: 10.1016/j.jclepro.2020.122575
Gerritsen, R. (2010). Evidence for Indigenous Australian Agriculture. Austr. Sci. 31, 35–37. Available online at: https://search.informit.org/doi/10.3316/ielapa.201007053
Giampietro, M., and Mayumi, K. (2018). Unraveling the complexity of the Jevons Paradox: The link between innovation, efficiency, and sustainability. Front. Energy. Res 6, 26. doi: 10.3389/fenrg.2018.00026
Gliessman, S. (2015). Agroecology: The Ecology of Sustainable Food Systems. 3rd ed. Boca Raton, USA: CRC Press.
Gliessman, S. (2016). Transforming food systems with agroecology. Agroecol. Sustain. Food. Syst. 40, 187–189. doi: 10.1080/21683565.2015.1130765
Gliessman, S., Friedmann, H., and Howard, H. P. (2019). “Agroecology and Food Sovereignty,” in The Political Economy of Food, IDS Bulletin, eds. J. Harris, M. Anderson, C. Clement, and N. Nisbett. (Brighton,. UK: Institute of Development Studies), 91–110.
Grab, H., Danforth, B., Poveda, K., and Loeb, G. (2018). Landscape simplification reduces classical biological control and crop yield. Ecol. Appl. 28, 348–355. doi: 10.1002/eap.1651
Grundy, M. J., Rossel, R. A. V., Searle, R. D., Wilson, P. L., Chen, C., Gregory, L. J., et al. (2015). Soil and Landscape Grid of Australia. Soil. Res. 53, 835–844. doi: 10.1071/SR15191
Hamant, O. (2020). Plant scientists can't ignore Jevons paradox anymore. Nat. Plants 6, 720–722. doi: 10.1038/s41477-020-0722-3
Hamilton, A., Balogh, S. B., Maxwell, A., and Hall, C. A. S. (2013). Efficiency of edible agriculture in Canada and the U.S. over the past three and four decades. Energies, 6, 1764–1793. doi: 10.3390/en6031764
Hammer, K., Arrowsmith, N., and Gladis, T. (2003). Agrobiodiversity with emphasis on plant genetic resources. Naturwissenschaften 90, 241–250. doi: 10.1007/s00114-003-0433-4
Handreck, K. A. (1997). Phosphorus requirements of Australian native plants. Soil Res. 35, 241–290. doi: 10.1071/S96060
Haplin, N., Rehbein, B., and Marshall, A. (2020). “Fallow management trial—Bundaberg,” in Sugarcane Field Trial Compendium: A. Collection of Research Trial Reports from 2012-2019, ed. Queensland Government Department of Agriculture and Fisheries (Brisbane, Australia: State of Queensland).
Hastie, T., Tibshirani, R., and Friedman, J. (2009). “Random forests,” in The Elements of Statistical Learning (Berlin, Germany: Springer), 587–604.
IBRA (2020). Interim Biogeographic Regionalisation for Australia (Subregions) v. 7 (IBRA). Canberra, Australia.
Idol, T., Haggar, J., and Cox, L. (2011). Ecosystem Services from Smallholder Forestry and Agroforestry in the Tropics (Berlin, Germany: Springer), 209–270.
Ijaz, M., Nawaz, A., Ul-Allah, S., Rizwan, M. S., Ullah, A., Hussain, M., et al. (2019). “Crop diversification and food security,” in Agronomic Crops, ed. M. Hasanuzzaman. (Singapore:. Springer), 607–621.
Isaacs, J. (2019). Bush Food: Aboriginal Food and Herbal Medicine. Sydney, Australia: New Holland Publishers.
Jacques, P. J., and Jacques, J. R. (2012). Monocropping cultures into ruin: the loss of food varieties and cultural diversity. Sustainability 4, 2970–2997. doi: 10.3390/su4112970
Jarvis, D., Maclean, K., and Woodward, E. (2022). The Australian Indigenous-led bush products sector: Insights from the literature and recommendations for the future. Ambio 51, 226–240. doi: 10.1007/s13280-021-01542-w
Kabir, Z. (2011). Tillage or no-tillage: impact on mycorrhizae. Can. J. Plant Sci. 85, 23–29. doi: 10.4141/P03-160
Kaseva, J., Himanen, S. J., and Kahiluoto, H. (2019). Managing diversity for food system resilience. Adv. Food Secur. Sustain. 4, 1–32. doi: 10.1016/bs.af2s.2019.07.001
Kay, S., Graves, A., Palma, J. H. N., Moreno, G., Roces-Díaz, J. v., and Aviron, S., et al. (2019). Agroforestry is paying off—Economic evaluation of ecosystem services in European landscapes with and without agroforestry systems. Ecosyst. Serv 36, 100896. doi: 10.1016/j.ecoser.2019.100896
Kaye, J. P., and Quemada, M. (2017). Using cover crops to mitigate and adapt to climate change. A review. Agron. Sustain. Dev. 37, 1–17. doi: 10.1007/s13593-016-0410-x
Khoury, C. K., Bjorkman, A. D., Dempewolf, H., Ramirez-Villegas, J., Guarino, L., Jarvis, A., et al. (2014). Increasing homogeneity in global food supplies and the implications for food security. Proc. Natl. Acad. Sci. U. S. A 111, 4001–4006. doi: 10.1073/pnas.1313490111
Kinnunen, P., Guillaume, J. H. A., Taka, M., D'Odorico, P., Siebert, S., Puma, M. J., et al. (2020). Local food crop production can fulfil demand for less than one-third of the population. Nat. Food 1, 229–237. doi: 10.1038/s43016-020-0060-7
Konczak, I., Dunstan, M., Aguas, P., Roulfe, P., and Pavan, A. (2009). Health Benefits of Australian Native Foods. Canberra, Australia.
Kremen, C., Iles, A., and Bacon, C. (2012). Diversified farming systems: an agroecological, systems-based alternative to modern industrial agriculture. Ecol. Soc. 17. doi: 10.5751/ES-05103-170444
LaCanne, C. E., and Lundgren, J. G. (2018). Regenerative agriculture: Merging farming and natural resource conservation profitably. PeerJ. 2018, e4428. doi: 10.7717/peerj.4428
Lal, R. (2020). Regenerative agriculture for food and climate. J. Soil Water Conserv. 75, 123A−124A. doi: 10.2489/jswc.2020.0620A
Leblanc, M., Tweed, S., van Dijk, A., and Timbal, B. (2012). A review of historic and future hydrological changes in the Murray-Darling Basin. Glob. Planet Change 80–81, 226–246. doi: 10.1016/j.gloplacha.2011.10.012
Leventon, J., and Laudan, J. (2017). Local food sovereignty for global food security? Highlighting interplay challenges. Geoforum 85, 23–26. doi: 10.1016/j.geoforum.2017.07.002
Mace, G. M., Norris, K., and Fitter, A. H. (2012). Biodiversity and ecosystem services: a multilayered relationship. Trends. Ecol. Evol 27, 19–26. doi: 10.1016/j.tree.2011.08.006
MacLaren, C., Storkey, J., Menegat, A., Metcalfe, H., and Dehnen-Schmutz, K. (2020). An ecological future for weed science to sustain crop production and the environment. A review. Agron. Sustain. Dev. 40, 1–29. doi: 10.1007/s13593-020-00631-6
Maclean, K., Woodward, E., Jarvis, D., Turpin, G., Rowland, D., Rist, P., et al. (2021). Decolonising knowledge co-production: examining the role of positionality and partnerships to support Indigenous-led bush product enterprises in northern Australia. Sustain. Sci. 17, 333–350. doi: 10.1007/s11625-021-00973-4
Martin, H. (2006). (2006). Cenozoic climatic change and the development of the arid vegetation in Australia. J. Arid. Environ. 66, 533–563. doi: 10.1016/j.jaridenv.2006.01.009
Massawe, F., Mayes, S., and Cheng, A. (2016). Crop diversity: an unexploited treasure trove for food security. Trends. Plant. Sci 21, 365–368. doi: 10.1016/j.tplants.2016.02.006
Mc Carthy, U., Uysal, I., Badia-Melis, R., Mercier, S., O'Donnell, C., Ktenioudaki, A., et al. (2018). Global food security—issues, challenges and technological solutions. Trends. Food. Sci. Technol 77, 11–20. doi: 10.1016/j.tifs.2018.05.002
McCloskey, G. L., Baheerathan, R., Dougall, C., Ellis, R., Bennett, F. R., Waters, D., et al. (2021). Modelled estimates of dissolved inorganic nitrogen exported to the Great Barrier Reef lagoon. Mar. Pollut. Bull 171, 112655. doi: 10.1016/j.marpolbul.2021.112655
McKenzie, N., Jacquier, D., Maschmedt, D., Griffin, E., and Brough, D. (2012). The Australian Soil Resource Information System (ASRIS) Technical Specifications. Revised Version 1, 6. >Canberra, Australia.
Meldrum, G., Mijatović, D., Rojas, W., Flores, J., Pinto, M., Mamani, G., et al. (2018). Climate change and crop diversity: farmers' perceptions and adaptation on the Bolivian Altiplano. Environ. Dev. Sustain. 20, 703–730. doi: 10.1007/s10668-016-9906-4
Meyer, R. S., Duval, A. E., and Jensen, H. R. (2012). Patterns and processes in crop domestication: an historical review and quantitative analysis of 203 global food crops. New. Phytol. 196, 29–48. doi: 10.1111/j.1469-8137.2012.04253.x
Miers, G. (2004). Cultivation and Sustainable Wild Harvest of Bushfoods by Aboriginal Communities in Central Australia. Canberra, Australia.
Milla, R., Rey, U., Carlos, J., and Mayfield, M. (2020). Crop Origins and Phylo Food: a database and a phylogenetic tree to stimulate comparative analyses on the origins of food crops. Global Ecol. Biogeogr. 29, 606–614. doi: 10.1111/geb.13057
Monjardino, M., Revell, D., and Pannell, D. J. (2010). The potential contribution of forage shrubs to economic returns and environmental management in Australian dryland agricultural systems. Agric. Syst 103, 187–197. doi: 10.1016/j.agsy.2009.12.007
Newton, P., Civita, N., Frankel-Goldwater, L., Bartel, K., and Johns, C. (2020). What Is Regenerative Agriculture? A Review of Scholar and Practitioner Definitions Based on Processes and Outcomes. Front. Sustain. Food. Syst 4, 194. doi: 10.3389/fsufs.2020.577723
Norris, C. E., and Congreves, K. A. (2018). Alternative management practices improve soil health indices in intensive vegetable cropping systems: a review. Front. Environ. Sci 6, 50. doi: 10.3389/fenvs.2018.00050
Ogilvie, C. M., Ashiq, W., Vasava, H. B., and Biswas, A. (2021). Quantifying root-soil interactions in cover crop systems: a review. Agriculture 11, 218. doi: 10.3390/agriculture11030218
Osipitan, O. A., Dille, J. A., Assefa, Y., and Knezevic, S. Z. (2018). Cover crop for early season weed suppression in crops: systematic review and meta-analysis. Agron. J 110, 2211–2221. doi: 10.2134/agronj2017.12.0752
Paterson, A. (2018). Once were foragers: The archaeology of agrarian Australia and the fate of Aboriginal land management. Quaternary. Int. 489, 4–16. doi: 10.1016/j.quaint.2016.12.047
Pittock, J., Finlayson, C. M., Pittock, J., and Finlayson, C. M. (2011). Australia's Murray–Darling Basin: freshwater ecosystem conservation options in an era of climate change. Mar. Freshw. Res 62, 232–243. doi: 10.1071/MF09319
Powers, R. P., and Jetz, W. (2019). Global habitat loss and extinction risk of terrestrial vertebrates under future land-use-change scenarios. Nat. Clim. Change 9, 323–329. doi: 10.1038/s41558-019-0406-z
Provenza, F. D., Meuret, M., and Gregorini, P. (2015). Our landscapes, our livestock, ourselves: restoring broken linkages among plants, herbivores, and humans with diets that nourish and satiate. Appetite 95, 500–519. doi: 10.1016/j.appet.2015.08.004
Puissant, J., Villenave, C., Chauvin, C., Plassard, C., Blanchart, E., Trap, J., et al. (2021). Quantification of the global impact of agricultural practices on soil nematodes: a meta-analysis. Soil. Biol. Biochem 161, 108383. doi: 10.1016/j.soilbio.2021.108383
R Core Team (2016). R: A Language and Environment for Statistical Computing. Vienna: R Foundation for Statistical Computing.
Ramankutty, N., Mehrabi, Z., Waha, K., Jarvis, L., Kremen, C., Herrero, M., et al. (2018). Trends in global agricultural land use: implications for environmental health and food security. Ann. Rev. Plant Biol. 69, 789–815. doi: 10.1146/annurev-arplant-042817-040256
Rayne, N., and Aula, L. (2020). Livestock manure and the impacts on soil health: a review. Soil. Syst. 4, 64. doi: 10.3390/soilsystems4040064
Revell, D. K., Norman, H. C., Vercoe, P. E., Phillips, N., Toovey, A., Bickell, S., et al. (2013). Australian perennial shrub species add value to the feed base of grazing livestock in low- to medium-rainfall zones. Anim. Prod. Sci 53, 1221–1230. doi: 10.1071/AN13238
Richmond, R., Bowyer, M., and Vuong, Q. (2019). Australian native fruits: Potential uses as functional food ingredients. J. Funct. Foods 62, 103547. doi: 10.1016/j.jff.2019.103547
Robbins, J. (2008). The New Crop Industries Handbook—Native Foods. Canberra, Australia: Australian Government Rural Industries Research and Development Corporation.
Robin, X., Turck, N., Hainard, A., Tiberti, N., Lisacek, F., Sanchez, J. C., et al. (2011). pROC: An open-source package for R and S+ to analyze and compare ROC curves. BMC. Bioinform. 12, 1–8. doi: 10.1186/1471-2105-12-77
Rodríguez-Ortega, T., Olaizola, A. M., and Bernués, A. (2018). A novel management-based system of payments for ecosystem services for targeted agri-environmental policy. Ecosyst. Serv 34, 74–84. doi: 10.1016/j.ecoser.2018.09.007
Ryder, M., Latham, Y., and Hawke, B. (2008). Cultivation and Harvest Quality of native Food Crops. Canberra, Australia: Rural Industries Research and Development Corporation.
Salzman, J., Bennett, G., Carroll, N., Goldstein, A., and Jenkins, M. (2018). The global status and trends of Payments for Ecosystem Services. Nat. Sustain 1, 136–144. doi: 10.1038/s41893-018-0033-0
Schmidhuber, J., and Tubiello, F. N. (2007). Global food security under climate change. Proc. Natl. Acad. Sci. U. S. A 104, 19703–19708. doi: 10.1073/pnas.0701976104
Sears, L., Caparelli, J., Lee, C., Pan, D., Strandberg, G., Vuu, L., et al. (2018). Jevons' Paradox and efficient irrigation technology. Sustainability 10, 1590. doi: 10.3390/su10051590
Settre, C. M., Connor, J. D., and Wheeler, S. A. (2019). Emerging water and carbon market opportunities for environmental water and climate regulation ecosystem service provision. J. Hydrol. (Amst) 578, 124077. doi: 10.1016/j.jhydrol.2019.124077
Shafi, U., Mumtaz, R., García-Nieto, J., Hassan, S. A., Zaidi, S. A. R., Iqbal, N., et al. (2019). Precision agriculture techniques and practices: from considerations to applications. Sensors 19, 3796. doi: 10.3390/s19173796
Shelef, O., Weisberg, P. J., and Provenza, F. D. (2017). The value of native plants and local production in an era of global agriculture. Front. Plant. Sci 8, 2069. doi: 10.3389/fpls.2017.02069
Singh, R., and Singh, G. S. (2017). Traditional agriculture: a climate-smart approach for sustainable food production. Ener. Ecol. Environ. 2, 296–316. doi: 10.1007/s40974-017-0074-7
Sommano, S., Caffin, N., and Kerven, G. (2013). Screening for antioxidant activity, phenolic content, and flavonoids from australian native food Plants. Int. J. Food Prop. 16, 1394–1406. doi: 10.1080/10942912.2011.580485
Stafford Smith, D. M., and Morton, S. R. (1990). A framework for the ecology of arid Australia. J. Arid. Environ 18, 255–278. doi: 10.1016/S0140-1963(18)30837-1
Stoate, C., Báldi, A., Beja, P., Boatman, N. D., Herzon, I., van Doorn, A., et al. (2009). Ecological impacts of early 21st century agricultural change in Europe—A review. J. Environ. Manage 91, 22–46. doi: 10.1016/j.jenvman.2009.07.005
Suárez-Torres, J., Suárez-López, J. R., López-Paredes, D., Morocho, H., Cachiguango-Cachiguango, L. E., Dellai, W., et al. (2017). Agroecology and health: lessons from indigenous populations. Curr. Environ. Health. Rep 4, 244–251. doi: 10.1007/s40572-017-0146-z
Sultanbawa, Y., and Sultanbawa, F. (2016). Australian Native Plants: Cultivation and Uses in the Health and Food. 1st ed. Boca Raton, FL: CRC Press.
Sun, Y., Zeng, Y., Shi, Q., Pan, X., and Huang, S. (2015). No-tillage controls on runoff: a meta-analysis. Soil. Tillage. Res. 153, 1–6. doi: 10.1016/j.still.2015.04.007
Teague, R., and Kreuter, U. (2020). Managing Grazing to Restore Soil Health, Ecosystem Function, and Ecosystem Services. Front. Sustain. Food. Syst. 4, 157. doi: 10.3389/fsufs.2020.534187
Teague, W. R. (2018). Forages and pastures symposium: cover crops in livestock production: whole-system approach: Managing grazing to restore soil health and farm livelihoods. J. Anim. Sci. 96, 1519–1530. doi: 10.1093/jas/skx060
Terrain Natural Resource Management (2020). Cassowary Credits Scheme: New Ecosystem Market. Available online at: https://terrain.org.au/cassowary-credits-scheme/ (accessed January 24, 2022).
Thackway, R., and Cresswell, I. (1995). An Interim Biogeographic Regionalisation for Australia. Canberra, Australia.
Ticktin, T., Quazi, S., Dacks, R., Tora, M., McGuigan, A., Hastings, Z., et al. (2018). Linkages between measures of biodiversity and community resilience in Pacific Island agroforests. Conserv. Biol. 32, 1085–1095. doi: 10.1111/cobi.13152
Tilman, D., Cassman, K. G., Matson, P. A., Naylor, R., and Polasky, S. (2002a). Agricultural sustainability and intensive production practices. Nature 418, 671–677. doi: 10.1038/nature01014
Tilman, D., Fargione, J., Wolff, B., D'Antonio, C., Dobson, A., Howarth, R., et al. (2001). Forecasting agriculturally driven global environmental change. Science 292, 281–284. doi: 10.1126/science.1057544
Tilman, D., Cassman, K. G., Matson, P. A., Naylor, R., and Polasky, S. (2002b). Agricultural sustainability and intensive production practices. Nature 418, 671–677.
Torralba, M., Fagerholm, N., Burgess, P. J., Moreno, G., and Plieninger, T. (2016). Do European agroforestry systems enhance biodiversity and ecosystem services? A meta-analysis. Agric. Ecosyst. Environ. 230, 150–161. doi: 10.1016/j.agee.2016.06.002
Tylianakis, J. M., Didham, R. K., Bascompte, J., and Wardle, D. A. (2008). Global change and species interactions in terrestrial ecosystems. Ecol. Lett 11, 1351–1363. doi: 10.1111/j.1461-0248.2008.01250.x
Tzounis, A., Katsoulas, N., Bartzanas, T., and Kittas, C. (2017). Internet of Things in agriculture, recent advances and future challenges. Biosyst. Eng. 164, 31–48. doi: 10.1016/j.biosystemseng.2017.09.007
van Dijk, M., Morley, T., Rau, M. L., and Saghai, Y. (2021). A meta-analysis of projected global food demand and population at risk of hunger for the period 2010–2050. Nat. Food 2, 494–501. doi: 10.1038/s43016-021-00322-9
Venevsky, S., and Veneskaia, I. (2003). Large-scale energetic and landscape factors of vegetation diversity. Ecol. Lett 6, 1004–1016. doi: 10.1046/j.1461-0248.2003.00527.x
Viscarra Rossel, R. A., Chen, C., Grundy, M. J., Searle, R., Clifford, D., Campbell, P. H., et al. (2015). The Australian three-dimensional soil grid: Australia's contribution to the GlobalSoilMap project. Soil. Res. 53, 845–864. doi: 10.1071/SR14366
Wezel, A., Herren, B. G., Kerr, R. B., Barrios, E., Gonçalves, A. L. R., Sinclair, F., et al. (2020). Agroecological principles and elements and their implications for transitioning to sustainable food systems. A review. Agron. Sustain. Dev. 40, 1–13. doi: 10.1007/s13593-020-00646-z
Wheeler, S., Loch, A., Zuo, A., and Bjornlund, H. (2014). Reviewing the adoption and impact of water markets in the Murray–Darling Basin, Australia. J. Hydrol. (Amst) 518, 28–41. doi: 10.1016/j.jhydrol.2013.09.019
Wheeler, T., and von Braun, J. (2013). Climate change impacts on global food security. Science 341, 508–513. doi: 10.1126/science.1239402
Wood, S. A., Smith, M. R., Fanzo, J., Remans, R., and Defries, R. S. (2018). Trade and the equitability of global food nutrient distribution. Nat. Sustain. 1, 34–37. doi: 10.1038/s41893-017-0008-6
Woodhouse, P. (2010). Beyond industrial agriculture? Some questions about farm size, productivity and sustainability. Jof. Agr. Change 10, 437–453. doi: 10.1111/j.1471-0366.2010.00278.x
Yang, T., Siddique, K. H. M., and Liu, K. (2020). Cropping systems in agriculture and their impact on soil health-A review. Glob. Ecol. Conserv 23, e01118. doi: 10.1016/j.gecco.2020.e01118
Keywords: traditional foods, ecosystem service, regenerative agriculture, polyculture agriculture, bush tucker, native food plants
Citation: Canning AD (2022) Rediscovering wild food to diversify production across Australia's agricultural landscapes. Front. Sustain. Food Syst. 6:865580. doi: 10.3389/fsufs.2022.865580
Received: 30 January 2022; Accepted: 17 October 2022;
Published: 31 October 2022.
Edited by:
Helda Morales, The South Border College (ECOSUR), MexicoReviewed by:
Stewart Diemont, SUNY College of Environmental Science and Forestry, United StatesNathan Einbinder, University of Plymouth, United Kingdom
Copyright © 2022 Canning. This is an open-access article distributed under the terms of the Creative Commons Attribution License (CC BY). The use, distribution or reproduction in other forums is permitted, provided the original author(s) and the copyright owner(s) are credited and that the original publication in this journal is cited, in accordance with accepted academic practice. No use, distribution or reproduction is permitted which does not comply with these terms.
*Correspondence: Adam D. Canning, adam.canning@jcu.edu.au
†ORCID: Adam D. Canning orcid.org/0000-0001-8813-2240