- Crops Genetic and Biotechnology, AU Flakkebjerg, Department of Agroecology, Aarhus University, Slagelse, Denmark
Climate change threatens global food security, but the biggest impact will be in arid, low social-economic regions. To improve food security, new breeding technologies (NBTs) could be implemented for re-domestication of crop wild relatives (CWR). CWR harbor many beneficial traits, but it is difficult to incorporate these traits into conventional breeding programs. Thus, although genebanks hold significant collections of CWR, their potential has yet to be reached. Using barley as an example, we describe how using genebank collections, digital sequence information and NBTs, re-domesticated barley can be produced with improved characteristics, while retaining the resilience and adaptation of the original material. Lastly, we highlight some obstacles that need to be overcome for re-domesticates to be adopted.
1 Introduction
The importance of genebanks in contributing to global food security is stated in target 2.5 of the UN Sustainable Development Goals.1 Globally, there are more than 1,750 genebanks conserving plant genetic resources (FAO, 2009). These genetic resources represent improved cultivars, landraces, weedy types, genetic stocks, and wild plant species. Of these, it is the wild species that are particularly recognized as potentially holding the key to many of the challenges facing agriculture today, including climate change, population growth and loss of agricultural land. The reason for emphasizing the importance of crop wild relatives (CWR) is that modern breeding has focused on developing high-yielding varieties that perform consistently across disparate environments, resulting in a diversity bottleneck within the breeding pool and farmers’ crops lacking resistance to biotic and abiotic stresses (Louwaars, 2018; Zhang et al., 2018; Khoury et al., 2022). CWR are seen as a vital source of diversity that can be leveraged to confer resistance to these stresses (Dempewolf et al., 2017; El Haddad et al., 2021).
Identifying and then making use of the genes that contribute to the resilience/resistance of CWR however, is not a small task, even with the advent of high throughput phenomics and genomics. Phenotyping technologies may be used to screen CWR directly, followed by crossing of the CWR with a cultivar with a suitable genetic background and then selection, or conversely, CWR may be crossed with domesticated lines first, followed by evaluation of the F1; both strategies involve advancement and screening of multiple subsequent backcross generations (Dempewolf et al., 2017; Zhang et al., 2022). Identification of the specific genes or quantitative trait loci (QTL) conferring resistance or tolerance to the target trait(s) can speed up the process of ensuring relevant genes are introgressed through marker-assisted selection (Swamy and Sarla, 2008; Sharma et al., 2013; Migicovsky and Myles, 2017). There are some examples where this type of pre-breeding work has paid dividends. For example, in rice (Oryza sativa), introgression lines derived from O. rufipogon have been produced with planthopper resistance (Li et al., 2019) and bacterial blight resistance (Xing et al., 2021); and introgression lines derived from O. meridonalis and O. rufipogon have increased salinity tolerance (Wairich et al., 2021). Nonetheless, the pre-breeding pipeline can be long, and challenges include inter-species cross-incompatibility and linkage drag, whereby it is not just the target gene that is introgressed, but also other genes, which may negatively impact the performance of introgression lines. Two examples would be the rye-wheat introgressions, which can negatively affect the agricultural value of wheat (Johansson et al., 2020), and H. spontaneum introgression into cultivated barley (Hernandez et al., 2020). These introgressions can also be quite large, increasing the chance that unwanted genes with unwanted traits are inserted. The choice of elite line used as background can also impact the expression of introgressed genes (Zhang et al., 2022). Progress in incorporating CWR into breeding programs therefore remains slow and requires very significant investment of time and money.
Given these barriers to using CWR in breeding pipelines, in agreement with others (Bohra et al., 2022; Garland and Curry, 2022), we suggest an alternative approach: to re-domesticate progenitors of modern crop cultivars using gene editing techniques (Hanak et al., 2022). The aim would not be to produce plant ideotypes that closely resemble modern cultivars; rather, to tweak promising accessions of target CWR to meet some of our food security needs, while retaining their resilience and resistance. Once the technology has been optimized, it could be applied to multiple genotypes and made available to farmers in marginal lands, ensuring evolution in the target environment in response to local environmental pressures. Here, we outline why this could significantly contribute to food security, focusing on barley as an exemplar of the approach.
2 Breeding and re-domestication
The main crops used in agriculture were domesticated roughly 12,000 years BP, in particular, in the region known as the Fertile Crescent, where crops such as barley, wheat, chickpea and lentils were first domesticated (Zohary et al., 2012). For millennia, farmers used their own cultivars, later recognized as ‘landraces’, which were adapted to the local environment. However, in the 19th century, breeding started to become more popular. With the understanding of genetics and new breeding methods such as pedigree and backcross breeding, mutation breeding and single seed descent, together with extensive use of fertilizer, the green revolution started in the 20th century (Fernie and Yan, 2019; Hickey et al., 2019; Singer et al., 2021). Since then, more tools have been implemented: molecular marker assisted breeding, genome wide association studies to find new QTL and, more recently, speed-breeding and inclusion of CWR in breeding programs (Hickey et al., 2019; Lenaerts et al., 2019; Singer et al., 2021). Additionally, new approaches incorporating machine-learning have been applied to increase breeding success (Niazian and Niedbała, 2020; Van Dijk et al., 2021).
Breeding in general, is nonetheless, still a time-consuming process and breeding with CWR is troublesome. Many hurdles must be overcome to use CWR, including possible crossing-incompatibilities and the workload of pre-breeding, outcrossing and line fixation. New approaches could be re- and de novo domestication (Box 1) using genome editing methods (Brozynska et al., 2016; Van Tassel et al., 2020; Gasparini et al., 2021; Zhu and Zhu, 2021). Here, a CWR is changed only in specific genes responsible for domestication. The plants could then, theoretically, be directly used in the field or act as new material in breeding pipelines. This approach offers some advantages over classical breeding:
I. Plants created by re- or de novo domestication (RD/DND) would be adapted to the environment they were taken from and harbor beneficial traits. This could include pathogen resistance, but in particular, tolerance to abiotic stresses and used in regions facing previously unknown or rare stresses (Yu and Li, 2022).
II. Only a specific number of genetic changes in domestication genes are needed to RD barley (Table 1), as proposed by Haas et al. (2019), and shown for tomato (Abbo et al., 2014; Zsögön et al., 2017).
III. Until 2023, 20 cultivated and 4 wild barleys have been sequenced and assembled (Sato et al., 2021; Sakkour et al., 2022; Pan et al., 2023). This could be considered a limitation, however, the genes behind the beneficial traits in the CWR do not need to be known; they are inherent in the plant.
IV. RD could be significantly faster compared to normal breeding, perhaps 1–5 years for RD compared to up to 10 years for winter barley breeding, and/or accelerate the pre-breeding process for CWR (Fernie and Yan, 2019; Hickey et al., 2019; Lenaerts et al., 2019; Stockinger, 2021; Zhu and Zhu, 2021).
V. RD could help break linkage drag by mutating desired traits without the carryover of undesired traits coupled to the QTL (See 4.2 NUD1).
BOX 1. De novo- and re-domestication.
Domestication, from domesticus (lat. “belonging to the house”), describes the process of transitioning wild plants into the domus (lat. “home”). Domestication of barley dates to roughly 10,000 years BP (before present), and evidence links it to the Fertile crescent in the near east. Domestication of barley was accompanied by domestication of other crops: einkorn and emmer wheat, and legumes such as lentils. Recently, two terms around domestication have become common: ‘re-domestication’ (RD) and ‘de novo-domestication’ (DND). Here, we follow the definitions of Yu and Li (2022). Re-domestication describes the process of domestication of a CWR of a cultivated crop. In the scope of barley, Hordeum vulgare ssp. spontaneum is the CWR of Hordeum vulgare ssp. vulgare that we discuss. In contrast, DND describes the process of domestication of a plant that has not been domesticated previously. An example here would be Hordeum bulbosum, a perennial wild barley not yet domesticated or used in agriculture.
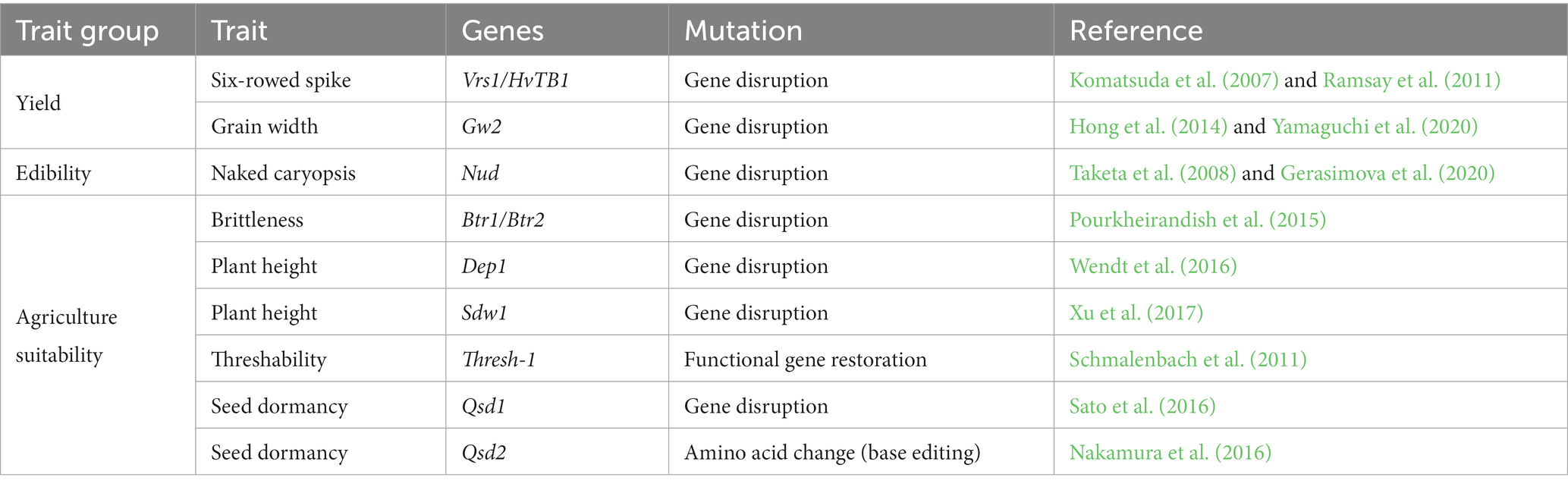
Table 1. Overview of the three important trait groups necessary to change in wild barley for re-domestication.
3 Why barley?
Barley, Hordeum vulgare subsp. vulgare (L.) K. Richt., is one of the major crops in terms of dry matter production worldwide (Verstegen et al., 2014), with more than 150 million tonnes harvested last year, and an estimated 142 million tonnes in 2023 (Foreign Agricultural Service, 2023). The main use of barley is feed for livestock and malt for beer production (Verstegen et al., 2014). In some African regions, however, barley is a staple food for people, providing carbohydrate, fiber and micronutrients (Langridge, 2018). Farmers in Africa and India are using barley as a dual-purpose plant, cutting the straw for animal feed early in the season and harvesting grain from the recovered plants at the end of the season (Sadreddine, 2016; Kumar et al., 2017). The barley grain has a high level of beta-glucan, which is associated with the maintenance or reduction of blood cholesterol and to reduction in post-prandial glycaemia (Harland, 2014).
Cultivated barley originates from its wild progenitor H. vulgare L. subsp. spontaneum (K.Koch) Thell. It was discovered and classified by Karl Koch as a species (H. spontaneum K. Koch), although modern taxonomy classifies H. spontaneum as a subspecies of H. vulgare (von Bothmer and Jacobsen, 1985; Zohary et al., 2012; Blattner, 2018). Barley has high adaptability and is known as ‘the last crop before the desert’.
3.1 Wild barley: a highly resilient subspecies
Although modern cultivated barley is a highly adapted self-pollinator, the breeding pool has lacked allelic variation, in particular for use in challenging environments (Ellis et al., 2000; Dawson et al., 2015). In the last decade, breeders have addressed this bottleneck through the use of landraces (Kumar et al., 2020; Brbaklić et al., 2021; Hill et al., 2021). In contrast, wild barley, H. hordeum subsp. spontaneum, has not undergone years of selective breeding and therefore still has its allelic variation. Research in wild barley has identified some of the genetic basis of salinity and drought tolerance (Ebrahim et al., 2020; Khalil et al., 2021). Wild barley accessions from arid regions seem to be superior under drought stress (Hong et al., 2020; Pan et al., 2022; Wang et al., 2023). Transcriptome analysis of wild barley led to the discovery of several differentially expressed genes that are responsible for gas exchange and stomatal traits (Ashoub et al., 2018; Chen et al., 2019). Wu et al. (2022) found a wild barley polymorphis for increased β-amylase activity during drought stress in wild barley. While Shrestha et al. (2022) identified a new polymorphism in the proline accumulation pathway and Pan et al. (2022) found a polymorphism in the ROS scavenging pathway, both conferring increased drought stress. Nitrogen use efficiency (NUE) is increasingly important (Ekardt et al., 2018). Studies with wild barley suggest that it inherits improved NUE and could be a valuable source for new alleles (Shah et al., 2017, 2019; Zahn et al., 2020). Rehman et al. (2021) screened wild barley accessions and found several accessions that were less susceptible to common diseases (net blotch, scald, leaf rust and powdery mildew). Resistance QTL and/or genes have been identified against Puccinia graminis f. sp. tritici (wheat stem rust) and P. graminis f. sp. secalis (rye stem rust) (Sallam et al., 2017; Henningsen et al., 2021), while Pan et al. (2021) found a novel barley mosaic virus resistance QTL. In summary, wild barley holds many beneficial traits and genes that could contribute to solving the major challenges facing agriculture but could already be used today to address urgent crises for people in poorer, climate-stressed regions.
3.2 Barley genetic resources
Genetic diversity is the foundation of breeding; conversely, breeding resulted in a reduction in diversity, due to the narrow genetic pool used (Van De Wouw et al., 2010; Swarup et al., 2021). Wide adoption of modern cultivars, which perform to a satisfactory degree across different environments, further leads to genetic erosion within farmer fields, through the planting of monocultures over large areas of land and displacement of landraces (Khoury et al., 2022). Recognizing the importance of novel material in breeding programs, collections of germplasm started to be established in the late-19th century (Hay et al., 2021) and efforts continue to collect both cultivated and CWR germplasm (Eastwood et al., 2022; Khan et al., 2022).
Given the importance of barley as a crop, it is not surprising that it is one of the best represented crops in genebanks, at least in terms of number of accessions. Further reflecting its importance, it is included in Annex 1 of the International Treaty on Plant Genetic Resources for Food and Agriculture (FAO, 2010). According to FAO (2009), the largest collections of barley genetic resources were held by national genebanks in Canada, United States and Brazil, and by the International Center for Agricultural Research in the Dry Areas (ICARDA), together accounting for 27% of the 466,531 accessions held in genebanks worldwide. Genesys, an online aggregator database of genebank accessions (Genesys, 2022), currently has records for 277,913 Hordeum accessions (or 242,981 accessions using ‘barley’ as the search term). Most of these records (225,736) are cultivated barley (current GRIN taxon indicated as H. vulgare subsp. vulgare or H. vulgare), but apart from those not specified, the other taxon with the most records is H. vulgare subsp. spontaneum (12,904 accessions). Approximately 35% (4,490 accession records) of these are held by the Lieberman Germplasm Bank in Israel, with other genebanks with >1,000 accession records in Genesys being Embrapa in Brazil, ICARDA in Lebanon, the Nordic Genetic Resource Center in Sweden, and the National Small Grains Germplasm Research Facility in the USA. Only 7,001 of the accession records of H. vulgare subsp. spontaneum have georeferenced data for their original source location. Based on this data, accessions have been collected, in countries such as Jordan, Israel and Lebanon, countries that are recognized as having high rates of aridity, and where cultivation of crops might be difficult, especially if irrigation is not possible (Figure 1). Accessions of H. bulbosum, H. murinum (inc. subsp. leporinum) and H. marinum (inc. subsp. gussoneanum) originate from the same latitudinal range (approx. 25–45°N) but extend further west. The other wild species represented in Genesys is H. chilense which, based on records in Genesys, has only been collected in Chile. In addition to these barley CWR, there are a number of other wild Hordeum spp., many of which have been identified as under-represented in genebanks (Vincent et al., 2012; Lala et al., 2018), but which might ultimately be similarly important sources of genes and/or candidates for RD.
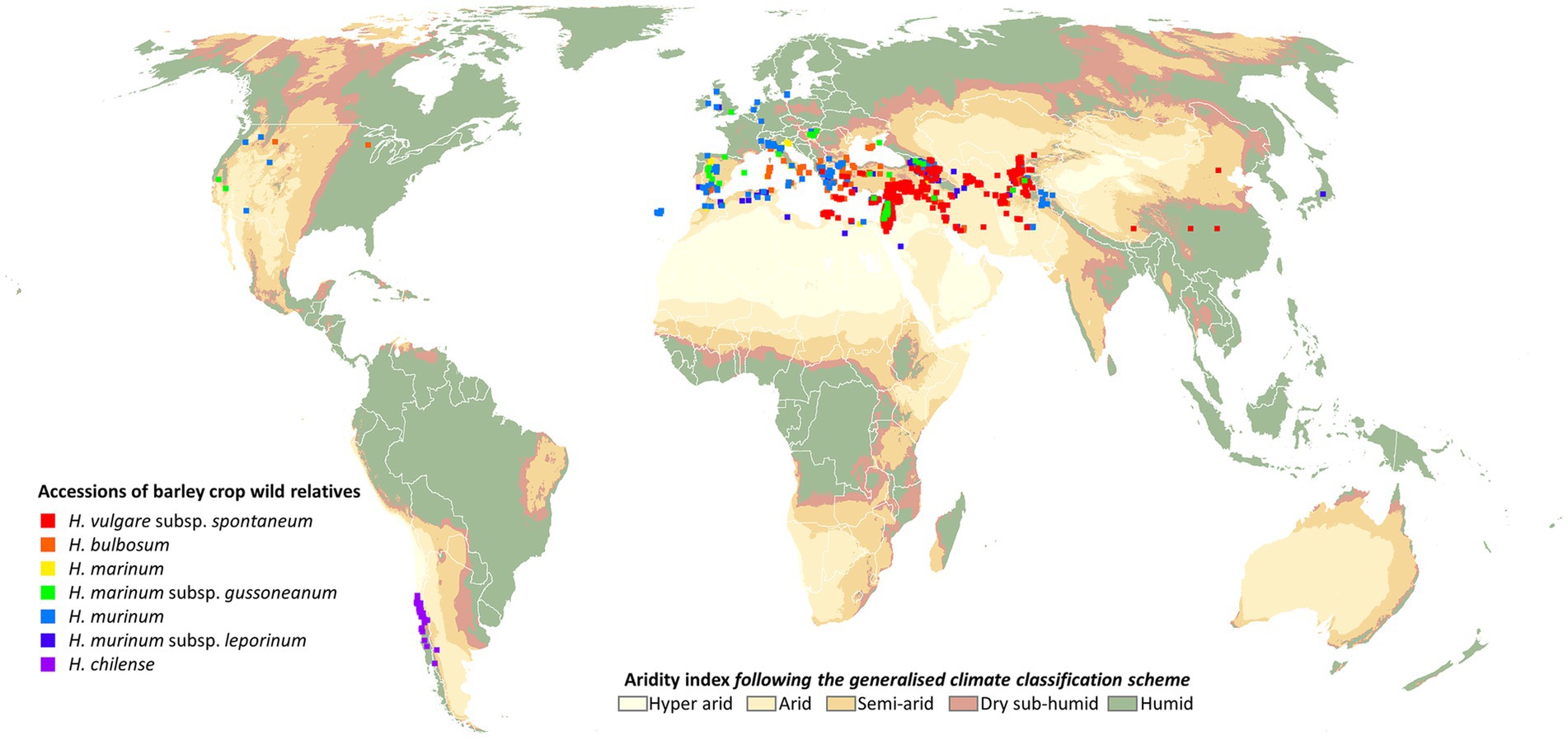
Figure 1. Overview of wild barley accessions listed in Genesys mapped to their origin on a global aridity index map (Zomer et al., 2022). Figure prepared by Andy Nelson, University of Twente, Netherlands.
4 Genome editing of target traits
Using digital sequence information and precise genome editing techniques such as CRISPR/Cas9, genes within CWR accessions can be specifically altered to the desired domesticated trait. The CRISPR/Cas9 toolkit facilitates the use of a small single-guide RNA containing a spacer, identical to the target region in the genome, guiding the enzyme complex to the target and inducing double strand breaks (Urnov et al., 2010; Gaj et al., 2013; Wei et al., 2013). Various tools then make the required change to the DNA in that region. For example, with base-editors, specific nucleotides can be changed to alter the transcribed amino acid (Gaudelli et al., 2017). PAM-less variants of Cas9 increase versatility and target selection (Walton et al., 2020; Ren et al., 2021). With prime editing, specific nucleotide changes at the protospacer region are possible (Anzalone et al., 2020). Twin prime editing targets larger regions enabling specific integrations into these regions (Anzalone et al., 2021). With multiplexing, several targets can be edited simultaneously, limited only by the plasmid size. However, new gRNA expression designs are increasing the possibilities (Xie et al., 2015).
CRISPR/Cas 9 mediated genome editing in barley is already well-established (Figure 2). Kapusi et al. (2017) induced deletions in the ENGase gene and Holme et al. (2017) knocked out phytase HvPAPhy_a. Use of CRISPR/Cas has also increased knowledge of lignin modifications (Lee et al., 2021), vitamin E biosynthesis (Zeng et al., 2020) and protein functions (Galli et al., 2022). Noteworthy is an approach by Panting et al. (2021) in which barley was modified for recombinant protein production. To RD barley from its progenitor, we conclude that four major traits would lead to a good foundation.
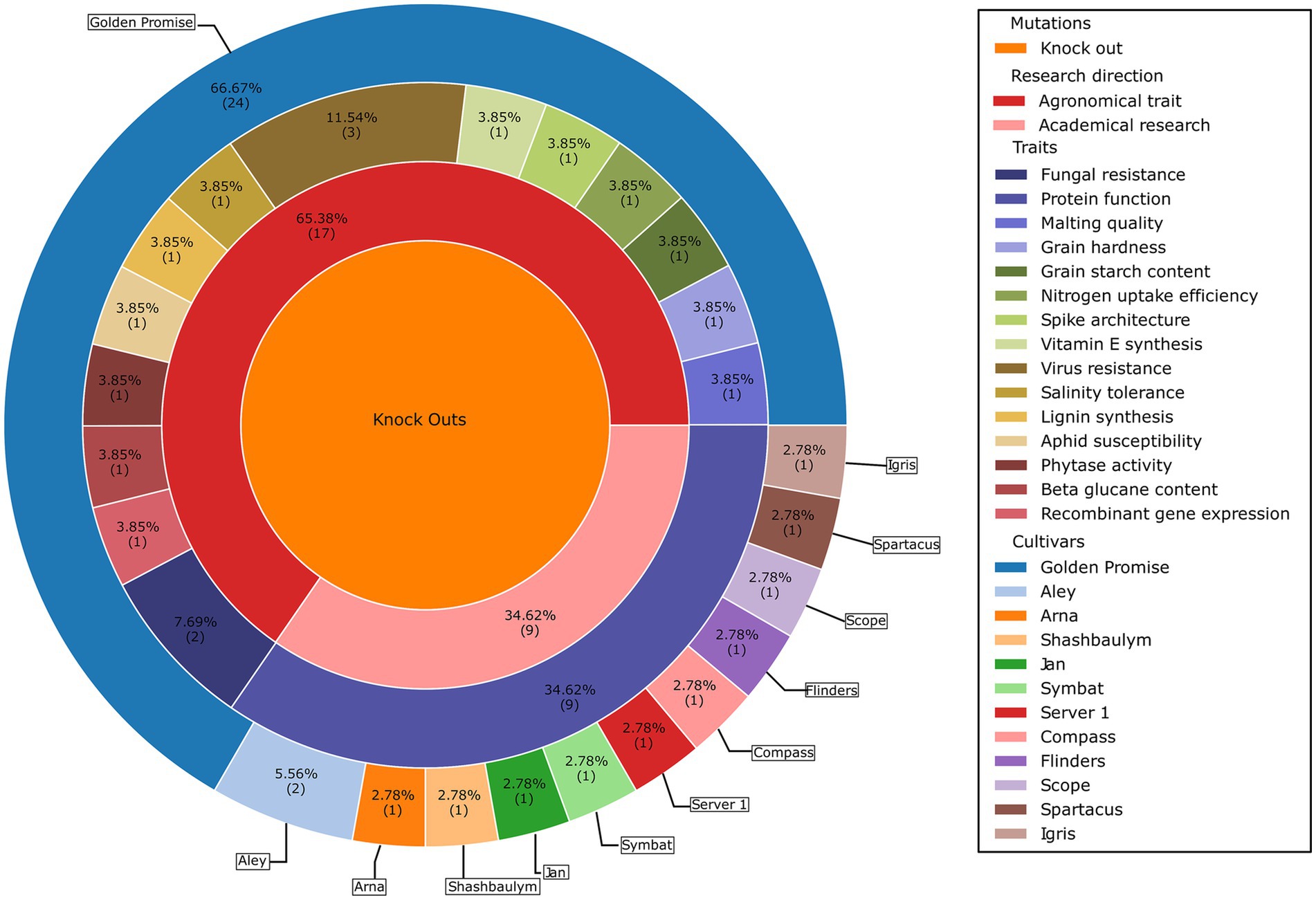
Figure 2. Current state of CRISPR/Cas9 mediated genome editing of traits in barley. Literature was taken from web of science with to the search terms limited to barley, genome editing and CRISPR/Cas9 (S1). All published literature were knockouts. The chart was made using python with the packages provided in the Supplementary data (Data Sheet 2) and Inkscape (Inkscape Project, 2020).
4.1 Yield
Differences in yield between cultivated and wild barley can be large. Yield is a polygenetic trait, which is not conferred by a single gene. Genes from different classes contribute to yield (Nadolska-Orczyk et al., 2017). Recently, Fernández-Calleja et al. (2021), summarized the interplay of major flowering time genes affecting yield in different ways, for example by affecting the number of spikelets or floret survival (Fernández-Calleja et al., 2021). Furthermore, new genes are constantly being found, which affect yield in different conditions (Thabet et al., 2020). It is unlikely, that single or multiplex editing will achieve similar results to nearly 10,000 years of breeding, but targeting a combination of genes could increase wild barley yield (Table 1). One important trait associated with yield is row architecture. Cultivated barley varieties are either 2- or 6-rowed; in 2-rowed barley, the two lateral spikelets either side of the central spikelet are sterile, while in 6-rowed barley, all spikelets are fertile. Two-rowed wild barleys could be modified to increase the quantity of seeds (Fukuyama et al., 1975; Lundqvist, 1997; Komatsuda et al., 2007). A knockout of Vrs1 and Vrs5 would be necessary (Komatsuda et al., 2007; Ramsay et al., 2011). An alternative approach to improve wild barley yield could be to increase grain size, by targeting Grain width 2; deletion of this gene in rice and transcript suppression in wheat resulted in bigger grains (Hong et al., 2014; Yamaguchi et al., 2020).
4.2 Edibility
Barley is the only member of the Poaceae family with a special botanical trait. Roughly 10 days after flowering, an adhesive coating forms around the caryopsis (Taketa et al., 2008). The majority of cultivated barley has this layer, since for malting, it is not disadvantageous (Taketa et al., 2004). However, for food production, the naked variant is preferred due to ease of processing and increased edibility. The genetic basis for the naked trait is a 17kbp deletion in the Nud locus (Taketa et al., 2008). Recently, CRISPR/Cas9-induced deletions in the Nud gene recreated the natural deletion phenotype (Gerasimova et al., 2020).
4.3 Agriculture suitability
Wild barley harbors various attributes limiting its use in agriculture. The most important change would be to remove the brittleness character of wild barley spikes by inducing a knockout in either the Btr1 or Btr2 gene (Pourkheirandish et al., 2015). This would impact the retention of grains on the spike, limiting seed dispersal and enabling harvest. The second important attribute is plant height. Tall plants are prone to lodging and hence, yield losses and declines in seed/grain quality (Rajkumara, 2008). Through mutagenesis and breeding of mutated alleles into cultivars, Dockter et al. (2014) created a dwarf phenotype. The erectoides-k mutation was the first mutation generated, however a candidate gene has not yet been identified (Gustafsson et al., 1977; Skov Kristensen et al., 2016). Two candidate genes where knockouts are conferring reduced plant height are Semidwarf1 (sdw1) and DEP1 (Wendt et al., 2016; Xu et al., 2017). Another trait is threshability. Threshability is conferred by the Thresh-1 locus, where a functional region enables easy removal of awns and breaking of the rachis (Schmalenbach et al., 2011). This trait will need a different approach; since a functional Thresh-1 is needed for threshability, restoration of this locus would be necessary, requiring either a homology directed repair approach or the new twin prime-editing approach. The presence/absence of seed dormancy is also an important trait in relation to agricultural suitability. In contrast to cultivated barley, wild barley seeds are highly dormant and long periods of after-ripening are needed for germination (Takeda and Hori, 2007). Two dormancy-related QTLs are described in the literature (Gong et al., 2014). Qsd1 confers an alanine aminotransferase (AlaAT); RNAi knock-down experiments confirmed its role in seed dormancy while sequence analysis revealed an amino acid change conferring the dormant phenotype (Sato et al., 2016). Alternatively, Qsd2 encodes for the mitogen activated map kinase kinase 3 (MKK3); this allele is found in Asian cultivars (Nakamura et al., 2016). A single amino acid change confers pre-harvest sprouting resistance (i.e., greater dormancy), preventing early germination (Nakamura et al., 2016). Since both candidates can be targeted with base editors, this makes them ideal targets for genome editing.
5 Legal obstacles
Producing gene-edited RD plant types is clearly feasible, but to have impact, seeds need to be made available to farmers in the target regions. This may be challenging due to laws related firstly, to genome-edited crops and secondly, to the distribution and sale of seeds (Palmgren et al., 2015).
5.1 Legislation around genome-edited crops
Genome-edited crops (GECs) are regulated differently around the globe, creating a complicated picture of where gene-edited RD plants might be used (Figure 3). Many countries take a cautionary approach, in part because of the public perception of genetically modified organisms (GMOs) which contain foreign DNA.
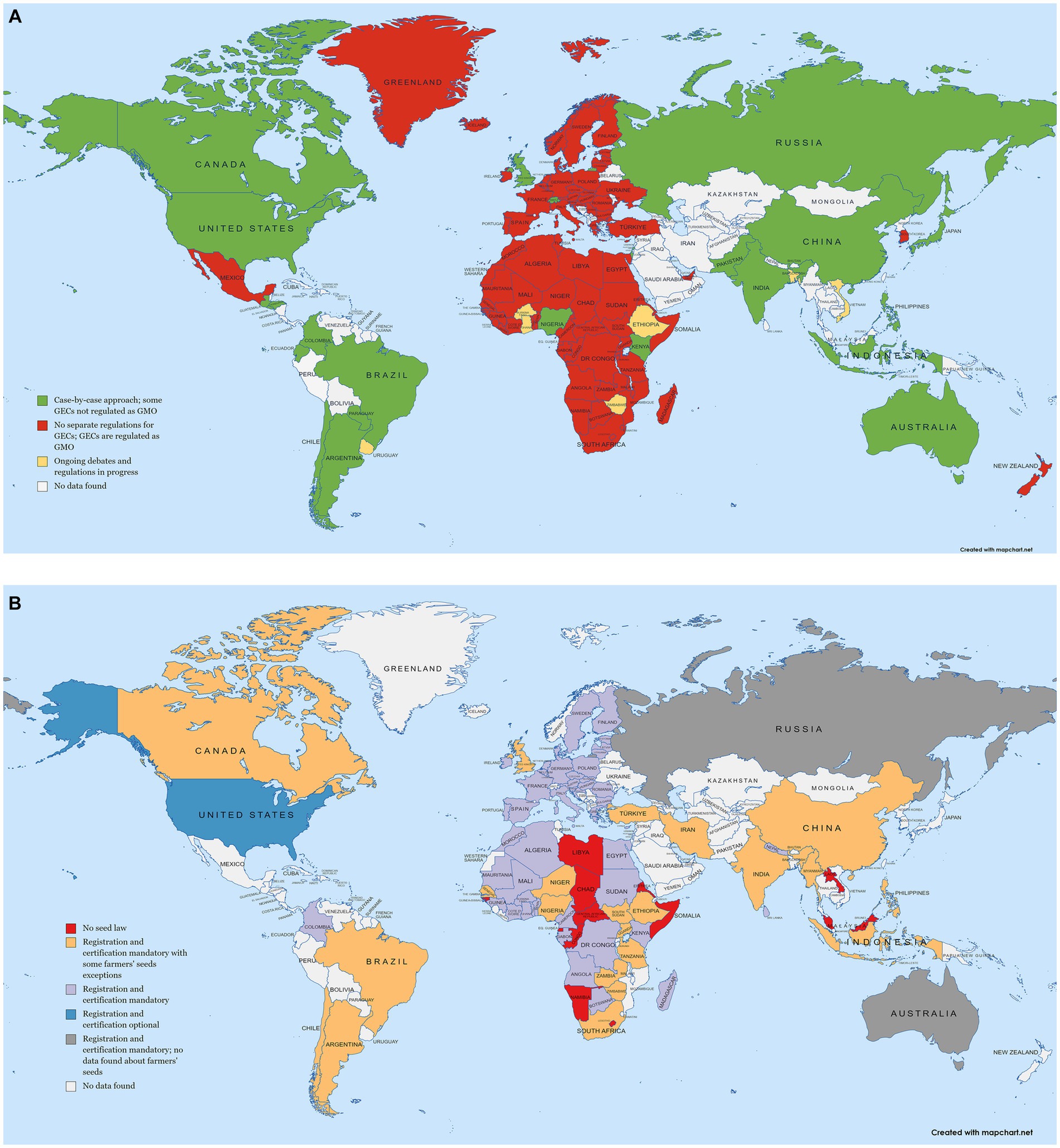
Figure 3. Overview of global regulations for (A) gene edited crops (GECs) and their classification; and (B) seed distribution systems and their requirements. The maps were generated using mapchart.net. References used for these maps can be found in the Supplementary File S2.
5.1.1 Case-by-case evaluation of GECs
Several countries generally accept GECs although there are still case-by-case evaluations of each product. The outcome then defines whether authorization is required, or a standard variety release procedure is applied (Whelan et al., 2020). Argentina was the first country with this type of legislative framework (Whelan et al., 2020). Other Latin American countries, e.g., Chile, Brazil and Colombia, followed Argentina’s approach (Schiemann et al., 2020). The United States, the current leader in NBT-derived plants (Parisi and Rodriguez-Cerezo, 2021), also takes this approach. The basis for this approach is that if plants obtained by NBTs contain a possibly naturally occurring mutation, they should be treated as conventionally generated plants (Hoffman, 2021). A recent new member of this type is Canada (Health Canada, 2022).
Countries in Africa could perhaps benefit the most from RD plants and, in general, from cultivation of GECs, as recognized by the African Union Agenda 2063 (Buchholzer and Frommer, 2023). Nigeria and Kenya are two pioneer countries with regulatory guidelines for GECs based on the case-by-case approach (Buchholzer and Frommer, 2023). In fact, in Kenya, gene insertions from sexually compatible species are excluded from the regulation under the Biosafety Act for GMOs (National Biosafety Authority Kenya, 2022).
On the Asian continent India introduced separate regulations regarding GECs in 2022. GECs obtained with non-homologous end joining or homologous directed repair with a template carrying specific nucleotide substitutions (regarded as a different allele) and without foreign DNA in the final product are exempted from GMO regulations (Government of India, 2022). Pakistan follows a similar route (Jones et al., 2022). Since Pakistan shows a high population growth rate, NBTs could be highly relevant in providing food security (Jones et al., 2022). Indonesia and the Philippines have also announced to follow the product-based approach (Sprink et al., 2022; Buchholzer and Frommer, 2023). Additionally, Japan and Australia share the approach of de-regulating plants edited without insertions of extracellularly made DNA template (Mallapaty, 2019; Tsuda et al., 2019).
5.1.2 GECs are treated as GMOs
The most restrictive approach to GECs is to group them with GMOs. This is the approach currently taken by, for example, the European Union, New Zealand, and the majority of countries in Africa (Fritsche et al., 2018; Genetic Literacy Project, 2020; European Parliament, 2022). Authorization is required for releasing GMOs on the market or into the environment, which can only be granted following an assessment of the risks (European Comission, 2021). Strict rules about monitoring, labeling and traceability requirements are part of the authorization (European Comission, 2021). Recently, the current approach, regulating the method of cultivar generation has been challenged, with suggestions that focus should be placed on the effect of the targeted gene edit (Jouanin et al., 2018; Leopoldina, NADW, 2019; Dima and Inze, 2021; Dima et al., 2022). In April 2021, the EU Commission published a study on New Genomic Techniques (NGTs), which include CRISPR/Cas9 and other genome editing approaches, indicating that current regulations need to be updated (European Comission, 2021). The same report concludes that “It may not be justified to apply different levels of regulatory oversight to similar products with similar levels of risk, as is the case for plants conventionally bred and obtained from certain NGTs.”
5.1.3 Ongoing debate
In between both extremes of evaluating GECs as GMOs or not, many countries are yet to decide. Recently, Burkina Faso, Ethiopia, Ghana, and Zimbabwe began discussing GECs (Entine et al., 2021). Asian countries such as Vietnam or Bangladesh could prosper significantly from GECs. Legislation still needs to be established but draft documents are being developed (Sprink et al., 2022). New Zealand is also a member of the states where NBTs are under discussion. The current situation sees GECs still as GMOs (Environmental Protection Authority of New Zealand, 2013; Kershen, 2015; Fritsche et al., 2018). Recently, a shift toward a political discussion in favor of GECs could be seen (Rolleston, 2019; Allan, 2023).
The legal challenges to using GECs are a major hurdle for farmers. While many countries have already accepted this technology, some countries that could benefit the most are still hesitant to adapt. Especially, in regions where borders are not entirely controlled, neighboring countries’ laws have a big impact. The European Union could be a flagship region in this field, paving the way for future adoption of this technology. A shift toward the acceptance of GECs could hereby lead to a general global shift, including in developing countries. For now, the current situation prohibits not only the use of this technology, but also the incentive to develop it further. Nonetheless, as more and more countries accept GECs and RD CWRs, more regions will likely follow.
5.2 Seed laws and regulations
Seed systems consider all components involved in seed supply and usage. Two different systems are defined: formal and informal (or farmers’) systems (Louwaars, 2007). The farmers’ system involves local seed selection, production and distribution by farmers (Louwaars, 2007 Louwaars and Manicad, 2022). The formal system consists of components surrounding seed production and supply on a large scale, operated by private or public entities (Louwaars, 2007). Additionally, a third, semi-formal (community-based or quality declared) system is sometimes distinguished. This may involve registered farmers or farmers’ organizations producing quality declared seeds (QDS). QDS must meet certain standards, but requirements are less strict than those applied to certified seeds within a formal system (FAO, 2006; Ayenan et al., 2021). For a variety to be registered and certified, it must be distinct, uniform, and stable (DUS) and undergo value for cultivation and use testing (VCU testing). The test considers variety performance, focusing on yield, resistance to harmful organisms, behaviour in the physical environment, and quality traits (Liveseed, 2021).
Western European countries and the United States have the oldest institutional framework for germplasm (Wattnem, 2016). The basis of today’s seed legislation was founded in 1966 (Wattnem, 2016). According to the system, a plant variety must fulfill ‘distinct, uniform and stable’ (DUS) criteria and ‘value for cultivation and use’ (VCU) testing and to be registered (both nationally and/or in the European Common Catalog). This system guarantees the quality of released varieties, but inhibited the farmers’ system and biodiversity conservation (Wattnem, 2016). This was addressed with a new directive enabling use of “conservational varieties” (Bocci, 2009). The US seed legislation differs substantially from the European version, making seed registration, certification and testing optional in regional institutions (Winge, 2012). Hence, the law does not illegalize the farmers’ system, and local, non-homogenized varieties can be marketed. Certification of a variety is highly interesting for agricultural businesses due to the protection provided by the Plant Variety Protection Act (PVP), the Plant Patent Act or the Utility Patent Protection for Plants (Winston, 2008). These laws allow breeders to prevent other parties from selling, reproducing, importing, exporting, or stocking the registered variety. Additionally, other parties cannot use it for breeding of new varieties or hybrids. Those protective practices hugely influenced the seed system’s homogenization (Wattnem, 2016) and marginalized the role of farmers’ systems.
In developing countries, seed regulations mainly arose from pressure imposed by agricultural companies and trade organizations (Wattnem, 2016). Latin American seed regulations are heavily influenced by EU seed laws. Such legal frameworks benefit the formal seed system through registration and certification processes that risk the exclusion of smaller companies or farmers. Colombia as an example, prohibits any commercialization and sharing of seeds without a certificate (Jiménez et al., 2013). Seeds can be certified if they fulfill DUS criteria and pass VCU testing. Additionally, registration is mandatory and storing seeds other than from the plot approved by the Colombian National Agricultural Institute (ICA) is not allowed (Campesina, 2015; Santilli, 2015; Wattnem, 2016).
Most African countries have national regulations (African Union Commission, 2021). Additionally, seed regulations in Africa are organized regionally, by the Economic Community of West African States (ECOWAS), the South African Development Community (SADC) and the Common Market for Eastern and Southern Africa (COMESA) (African Union Commission, 2021). The main aspects covered by the regional regulations are variety release, seed certification, and phytosanitary control (African Union Commission, 2021). Some national laws are in complete harmony with the regional laws; in other countries, implementation and harmonization are in progress. The regional rules focus on regulating the formal seed system, leaving the farmers’ system regulated by national legislation. National laws differ in their regulations of the informal seed system. Although in most countries, a variety must be in the national catalog and/or certified or with the quality declaration, some national provisions exempt farmers’ seeds from those regulations (Figure 3).
In Asia, the seed system legislation varies among countries, but since establishing seed laws, countries have been pressured to adopt patent legislation, the PVP act and the seed certification system (Campesina, 2015). FAO analyzed seed systems in 20 Asian states; only Malaysia and Laos still lacked national seed laws (FAO, 2020). Some countries implemented the PVP Act in their laws entirely or parts of it, such as the Republic of Korea and China, respectively (FAO, 2020). China did not implement the PVP act completely, in order to strengthen their farmers’ role in the seed system (FAO, 2020). Additionally, Indonesia, the Philippines, Bhutan and Myanmar seed laws concern and include farmers’ rights (FAO, 2020). Some countries made it more difficult for small farmers with the necessity for DUS and VCU testing. Nepal and Sri Lanka have both as a requirement for nearly all crops, but in general, DUS is more prevalent (FAO, 2020). Certification is mandatory mainly for major cereals, roots and tubers (FAO, 2020). Apart from legal requirements, a problem highlighted in the report was ‘fake seeds’ (FAO, 2020).
Making VCU testing, DUS and certification mandatory creates problems for farmers. Responding to the implementation of restrictive laws, farmers protested, for example, in India after implementation of the PVP act. Subsequently, the Protection of Plant Varieties and Farmers’ Rights Act was passed, giving the rights back to farmers (Peschard and Randeria, 2020). The act is known to be one of the legislations with the most rights given to farmers (Peschard, 2014). In Indonesia, the introduction of seed laws led to a court trial of a farmer for illegally reproducing and distributing seeds (Campesina, 2015). However, the Constitutional Court decided that the seed law was unconstitutional and permission to collect, reproduce and distribute seeds is not required anymore (Campesina, 2015).
The seed system legislation is still limiting and creating difficulties for farmers. RD of barley and other GECs could potentially bring significant prosperity to the informal seed system. However, even if the regulations around GECs enable their cultivation, seed laws and patent acts prevent those crops’ seeds from being used, reproduced and distributed by farmers. Additionally, the technology for making GEC varieties is largely restricted to the formal seed sector; hence, their production would be monopolized. It would be crucial to loosen restrictive patent and seed laws. Additionally, NGOs or state-run research facilities can play a role in making GECs accessible to farmers.
6 Discussion
Clearly there is huge potential for RD and DND crops, and the proof-of-concepts in ground cherry, tomato and allotetraploid rice show some of the likely impact of NBTs (Lemmon et al., 2018; Li et al., 2018; Zsögön et al., 2018; Yu et al., 2021). With the various NBT toolkits that are now available, we can specifically alter the genomic DNA of an organism to produce desired traits. However, there are still gaps hindering the full realization of the potential of NBT, namely: (i) transformability and tissue culture regeneration of plants; (ii) screening material; (iii) connecting genebank accessions with genomic data from genotyping, omics, and phenotyping data.
i. Transformability is perhaps still the biggest hurdle for NBTs. To date, in barley, most of the research is done using ‘Golden Promise’ since it is well known for its transformability and tissue culture suitability. Despite extensive research into new transformation protocols, progress using wild barley or even other genotypes of cultivated barley has been slow (Orman-Ligeza et al., 2020; Choi et al., 2022; Tiwari et al., 2022; Wang et al., 2022). Some success has been achieved using the barley ovule system (Holme et al., 2008), but we still need to optimize this technique for wild barley.
ii. Screening for suitable material will be another challenge, not only for RD; it is also necessary for classical breeding. For RD, incorporating algorithm-based analyzes is possible. These machine- or deep-learning tools are already common in plant science (Van Dijk et al., 2021; Yan and Wang, 2022). Automated analysis of big data sets has enabled disease stress analysis, crop phenotyping and biochemical network analysis (Singh et al., 2018; Van Dijk et al., 2021; Zheng et al., 2021). Recently, Sandhu et al. (2021) were able to predict complex traits in breeding wheat (Sandhu et al., 2021). Van Hilten et al. (2021), were able to predict phenotypes from genetic variants. As noted by Zheng et al. (2021), another great opportunity could be the use of satellite or drone data. While these authors mainly focused on precision farming of strawberry, we suggest another possibility: screening for wild plants in rough environments via satellite pictures or drones, and evaluation of the acquired data via machine-learning. Once appropriate analytical methods have been developed, this approach could cover large areas of land and quickly identify new genetic resources.
iii. Genetic resources comprise not just the physical material which a genebank distributes, but also all the information that is collected on the material. The most basic information is the passport data, but more and more characterization and evaluation data are being collected for genebank accessions. Further, it is increasingly recognized that it is important that accession-level data is made accessible; this will also be important to realize the potential of CWR and NBTs. Relevant data includes characterization and phenotyping data, and digital sequence information for specific genes and/or partial or whole genomes, collected by researchers both within and outside the genebank. Tools are available to ensure linkages among databases and accessibility, for example, the use of digital object identifiers for germplasm as part of the global information system (FAO, 2023) and databases such as Germinate which maintain accession-level phenotypic data (Raubach et al., 2021). Nonetheless, there needs to be strong collaboration among scientists to ensure relevant data is collected and made available, and to avoid duplication of efforts and waste of resources.
With progress in these fields, we can think about the future of plant breeding and achieving global food security, through RD, as discussed here for the CWR Hordeum vulgare subsp. spontaneum, but also for less closely related CWR. DND of Hordeum bulbosum could create a perennial barley suited to long-term sustainable cultivation. However, this will require significant research investment to develop transformation protocols. We are already at the start of what some are referring to as Breeding 4.0, combining genome editing with the use of algorithms to predict outcomes (Kuriakose et al., 2020; Shen et al., 2022; Zhao et al., 2022). Further ahead, in Breeding 5.0, the specific de-novo design of genes and metabolic pathways could be possible (Kuriakose et al., 2020). Thus, we can start to imagine creating plant types suited to diverse extreme environments, from regions with high aridity, to those with high levels of heavy metals or salt or other pollutants, to the challenges of for example, low gravity and/or high radiation in space. RD of highly resilient CWR may be the best starting point for producing varieties that are uniquely adapted.
Author contributions
TH: Conceptualization, Investigation, Visualization, Writing – original draft, Writing – review & editing. JJ: Conceptualization, Investigation, Visualization, Writing – original draft, Writing – review & editing. FH: Supervision, Writing – original draft, Writing – review & editing, Conceptualization, Investigation, Visualization. HB-P: Conceptualization, Funding acquisition, Project administration, Supervision, Writing – review & editing.
Funding
The author(s) declare financial support was received for the research, authorship, and/or publication of this article. This study was part of the Novo Nordisk Foundation project NovoCrops “NNF19OC0056580” and of the BarleyMicroBreed project funded through the European Union’s Horizon research and innovation program under grant agreement No 101060057.
Acknowledgments
We would like to thank Khaled Abulaila (National Center for Agricultural Research and Extension, Jordan), Vania Azevedo (International Potato Center, Peru), Hrannar Smári Hilmarsson (Agricultural University of Iceland), Mohammad Khajeh-Hosseini (Ferdowsi University of Mashhad, Iran), Norma Manrique (Alliance of Bioversity and CIAT, Colombia), Seid Hussein Muhie (Wollo University, Ethiopia), A. Naci Onus (Akdeniz University Agricultural Fakültesi Antalya, Turkey) and R. K. Singh (International Center for Biosaline Agriculture) for information on laws around genetically modified organisms.
Conflict of interest
The authors declare that the research was conducted in the absence of any commercial or financial relationships that could be construed as a potential conflict of interest.
Publisher’s note
All claims expressed in this article are solely those of the authors and do not necessarily represent those of their affiliated organizations, or those of the publisher, the editors and the reviewers. Any product that may be evaluated in this article, or claim that may be made by its manufacturer, is not guaranteed or endorsed by the publisher.
Supplementary material
The Supplementary material for this article can be found online at: https://www.frontiersin.org/articles/10.3389/fsufs.2023.1331577/full#supplementary-material
Footnotes
References
Abbo, S., Van-Oss, R. P., Gopher, A., Saranga, Y., Ofner, I., and Peleg, Z. (2014). Plant domesftication versus crop evolution: a conceptual framework for cereals and grain legumes. Trends Plant Sci. 19, 351–360. doi: 10.1016/j.tplants.2013.12.002
African Union Commission. (2021). The seed sector in Africa–Status Report and Ten-year Action Plan (2020–2030): A summary. Addis Ababa: African Union Commission.
Allan, A. (2023). Climate change makes it high time to grow GMOs.. Auckland: University of Auckland,Waipaqa Taumata Rau.
Anzalone, A. V., Gao, X. D., Podracky, C. J., Nelson, A. T., Koblan, L. W., Raguram, A., et al. (2021). Programmable deletion, replacement, integration and inversion of large DNA sequences with twin prime editing. Nat. Biotechnol. 40, 731–740. doi: 10.1038/s41587-021-01133-w
Anzalone, A. V., Koblan, L. W., and Liu, D. R. (2020). Genome editing with CRISPR-Cas nucleases, base editors, transposases and prime editors. Nat. Biotechnol. 38, 824–844. doi: 10.1038/s41587-020-0561-9
Ashoub, A., Müller, N., Jiménez-Gómez, J. M., and Brüggemann, W. (2018). Prominent alterations of wild barley leaf transcriptome in response to individual and combined drought acclimation and heat shock conditions. Physiol. Plant. 163, 18–29. doi: 10.1111/ppl.12667
Ayenan, M. A. T., Aglinglo, L. A., Zohoungbogbo, H. P. F., N'Danikou, S., Honfoga, J., Dinssa, F. F., et al. (2021). Seed Systems of Traditional African Vegetables in eastern Africa: A systematic review. Front. Sustain. Food Syst. 5:909. doi: 10.3389/fsufs.2021.689909
Blattner, F. R. (2018). “Taxonomy of the genus Hordeum and barley (Hordeum vulgare)” in The barley genome (Berlin: Springer International Publishing), 11–23.
Bocci, R. (2009). Seed legislation and agrobiodiversity: conservation varieties. J. Agric. Environ. Int. Dev. 103, 31–49. doi: 10.12895/jaeid.20091/2.23
Bohra, A., Kilian, B., Sivasankar, S., Caccamo, M., Mba, C., McCouch, S. R., et al. (2022). Reap the crop wild relatives for breeding future crops. Trends Biotechnol. 40, 412–431. doi: 10.1016/j.tibtech.2021.08.009
Brbaklić, L., Trkulja, D., Mikić, S., Mirosavljević, M., Momčilović, V., Dudić, B., et al. (2021). Genetic diversity and population structure of Serbian barley (Hordeum vulgare L.) collection during a 40-year long breeding period. Agronomy 11:118. doi: 10.3390/agronomy11010118
Brozynska, M., Furtado, A., and Henry, R. J. (2016). Genomics of crop wild relatives: expanding the gene pool for crop improvement. Plant Biotechnol. J. 14, 1070–1085. doi: 10.1111/pbi.12454
Buchholzer, M., and Frommer, W. B. (2023). An increasing number of countries regulate genome editing in crops. New Phytol. 237, 12–15. doi: 10.1111/nph.18333
Chen, G., Wang, Y., Wang, X., Yang, Q., Quan, X., Zeng, J., et al. (2019). Leaf epidermis transcriptome reveals drought-induced hormonal signaling for stomatal regulation in wild barley. Plant Growth Regul. 87, 39–54. doi: 10.1007/s10725-018-0450-0
Choi, S.-W., Kumaishi, K., Motohashi, R., Enoki, H., Chacuttayapong, W., Takamizo, T., et al. (2022). Oxicam-type nonsteroidal anti-inflammatory drugs enhance Agrobacterium -mediated transient transformation in plants. Plant Biotechnol. 39, 323–327. doi: 10.5511/plantbiotechnology.22.0312a
Dawson, I. K., Russell, J., Powell, W., Steffenson, B., Thomas, W. T. B., and Waugh, R. (2015). Barley: a translational model for adaptation to climate change. New Phytol. 206, 913–931. doi: 10.1111/nph.13266
Dempewolf, H., Baute, G., Anderson, J., Kilian, B., Smith, C., and Guarino, L. (2017). Past and future use of wild relatives in crop breeding. Crop Sci. 57, 1070–1082. doi: 10.2135/cropsci2016.10.0885
Dima, O., Heyvaert, Y., and Inze, D. (2022). Interactive database of genome editing applications in crops and future policy making in the European Union. Trends Plant Sci. 27, 746–748. doi: 10.1016/j.tplants.2022.05.002
Dima, O., and Inze, D. (2021). The role of scientists in policy making for more sustainable agriculture. Curr. Biol. 31, R218–R220. doi: 10.1016/j.cub.2021.01.090
Dockter, C., Gruszka, D., Braumann, I., Druka, A., Druka, I., Franckowiak, J., et al. (2014). Induced variations in brassinosteroid genes define barley height and sturdiness, and expand the green revolution genetic toolkit. Plant Physiol. 166, 1912–1927. doi: 10.1104/pp.114.250738
Eastwood, R. J., Tambam, B. B., Aboagye, L. M., Akparov, Z. I., Aladele, S. E., Allen, R., et al. (2022). Adapting agriculture to climate change: A synopsis of coordinated National Crop Wild Relative Seed Collecting Programs across five continents. Plan. Theory 11:1840. doi: 10.3390/plants11141840
Ebrahim, F., Arzani, A., Rahimmalek, M., Sun, D., and Peng, J. (2020). Salinity tolerance of wild barley Hordeum vulgare ssp. spontaneum. Plant Breed. 139, 304–316. doi: 10.1111/pbr.12770
Ekardt, F., Wieding, J., Garske, B., and Stubenrauch, J. (2018). Agriculture-related climate policies - law and governance issues on the European and global level. Carbon Clim. Law Rev. 12, 316–331. doi: 10.21552/cclr/2018/4/7
El Haddad, N., Sanchez-Garcia, M., Visioni, A., Jilal, A., El Amil, R., Sall, A. T., et al. (2021). Crop wild relatives crosses: multi-location assessment in durum wheat, barley, and lentil. Agronomy 11:2283. doi: 10.3390/agronomy11112283
Ellis, R. P., Forster, B. P., Robinson, D., Handley, L. L., Gordon, D. C., Russell, J. R., et al. (2000). Wild barley: a source of genes for crop improvement in the 21st century? J. Exp. Bot. 51, 9–17. doi: 10.1093/jexbot/51.342.9
Entine, J., Felipe, M. S. S., Groenewald, J. H., Kershen, D. L., Lema, M., McHughen, A., et al. (2021). Regulatory approaches for genome edited agricultural plants in select countries and jurisdictions around the world. Transgenic Res. 30, 551–584. doi: 10.1007/s11248-021-00257-8
Environmental Protection Authority of New Zealand (2013). Decision. New Zealand: Environmental Protection Authority.
European Comission. (2021). Study on the status of new genomic techniques under union law and in light of the court of justice ruling in case C-528/16. Brussels: European Comission.
European Parliament. (2022). Genome-edited crops and 21st century food system challenges. Brussels: European Parliament.
FAO. (2009). International Treaty on Plant Genetic Resources for Food and Agriculture.Rome, Italy: FAO.
FAO. (2010). The second report on the state of the World’s plant genetic resources for food and agriculture. Rome: World Summit on Food Security Rome.
FAO. (2020). Status of seed legislation and policies in the Asia-Pacific region, Bangkok. Rome, Italy: FAO.
FAO (2023). The Global Information System for Plant Genetic Ressources for Food and Agriculture (GLIS). Food and Agriculture Organization of the United Nations. Available at: https://ssl.fao.org/glis/ (Accessed January 02, 2023).
Fernández-Calleja, M., Casas, A. M., and Igartua, E. (2021). Major flowering time genes of barley: allelic diversity, effects, and comparison with wheat. Theor. Appl. Genet. 134, 1867–1897. doi: 10.1007/s00122-021-03824-z
Fernie, A. R., and Yan, J. (2019). De novo domestication: an alternative route toward new crops for the future. Mol. Plant 12, 615–631. doi: 10.1016/j.molp.2019.03.016
Foreign Agricultural Service. (2023). World barley production, consumption, and stocks: Foreign Agricultural Service: Production, supply and distribution. Washington, DC: United States Department of Agriculture.
Fritsche, S., Poovaiah, C., MacRae, E., and Thorlby, G. (2018). A New Zealand perspective on the application and regulation of gene editing. Front. Plant Sci. 9:1323. doi: 10.3389/fpls.2018.01323
Fukuyama, T., Hayashi, J., and Takahashi, R. (1975). Genetic and linkage studies of the five types of induced ‘six-row’ mutants. Barley Genet. Newsl. 5, 12–13.
Gaj, T., Gersbach, C. A., and Barbas, C. F. (2013). ZFN, TALEN, and CRISPR/Cas-based methods for genome engineering. Trends Biotechnol. 31, 397–405. doi: 10.1016/j.tibtech.2013.04.004
Galli, M., Martiny, E., Imani, J., Kumar, N., Koch, A., Steinbrenner, J., et al. (2022). CRISPR/SpCas9-mediated double knockout of barley Microrchidia MORC1 and MORC6a reveals their strong involvement in plant immunity, transcriptional gene silencing and plant growth. Plant Biotechnol. J. 20, 89–102. doi: 10.1111/pbi.13697
Garland, S., and Curry, H. A. (2022). Turning promise into practice: crop biotechnology for increasing genetic diversity and climate resilience. PLoS Biol. 20:e3001716. doi: 10.1371/journal.pbio.3001716
Gasparini, K., Moreira, J. D. R., Peres, L. E. P., and Zsogon, A. (2021). De novo domestication of wild species to create crops with increased resilience and nutritional value. Curr. Opin. Plant Biol. 60:102006. doi: 10.1016/j.pbi.2021.102006
Gaudelli, N. M., Komor, A. C., Rees, H. A., Packer, M. S., Badran, A. H., Bryson, D. I., et al. (2017). Programmable base editing of A•T to G•C in genomic DNA without DNA cleavage. Nature 551, 464–471. doi: 10.1038/nature24644
Genesys. (2022). Available at: https://www.genesys-pgr.org. (Accessed November 29, 2022).
Genetic Literacy Project. (2020). Global gene editing regulation tracker and index. Available at: https://crispr-gene-editing-regs-tracker.geneticliteracyproject.org/ (Accessed March 16, 2023).
Gerasimova, S. V., Hertig, C., Korotkova, A. M., Kolosovskaya, E. V., Otto, I., Hiekel, S., et al. (2020). Conversion of hulled into naked barley by Cas endonuclease-mediated knockout of the NUD gene. BMC Plant Biol. 20:255. doi: 10.1186/s12870-020-02454-9
Gong, X., Li, C., Zhou, M., Bonnardeaux, Y., and Yan, G. (2014). Seed dormancy in barley is dictated by genetics, environments and their interactions. Euphytica 197, 355–368. doi: 10.1007/s10681-014-1072-x
Government of India. (2022). Office Memorandum: Guidelines for the Safety Assessment of Genome Edited Plants. Government of India.
Gustafsson, Å., Ekman, G., and Dormling, I. (1977). Effects of the Pallas gene in barley: phene analysis, overdominance, variability. Hereditas 86, 251–266. doi: 10.1111/j.1601-5223.1977.tb01235.x
Haas, M., Schreiber, M., and Mascher, M. (2019). Domestication and crop evolution of wheat and barley: genes, genomics, and future directions. J. Integr. Plant Biol. 61, 204–225. doi: 10.1111/jipb.12737
Hanak, T., Madsen, C. K., and Brinch-Pedersen, H. (2022). Genome editing-accelerated re-domestication (GEaReD)–A new major direction in plant breeding. Biotechnol. J. 17:545. doi: 10.1002/biot.202100545
Harland, J. (2014). “2 - authorised EU health claims for barley and oat beta-glucans” in Foods, nutrients and food ingredients with authorised EU health claims. ed. M. J. Sadler (Sawston, United Kingdom: Woodhead Publishing), 25–45.
Hay, F. R., Whitehouse, K. J., Ellis, R. H., Sackville Hamilton, N. R., Lusty, C., Ndjiondjop, M. N., et al. (2021). CGIAR genebank viability data reveal inconsistencies in seed collection management. Glob. Food Sec. 30:100557. doi: 10.1016/j.gfs.2021.100557
Health Canada. (2022). Guidelines for the safety assessment of novel foods, Appendix 1: Health Canada guidance on the novelty interpretation of products of plant breeding. Health Canada.
Henningsen, E., Sallam, A. H., Matny, O., Szinyei, T., Figueroa, M., and Steffenson, B. J. (2021). Rpg7: A new gene for stem rust resistance from Hordeum vulgare ssp. spontaneum. Phytopathology 111, 548–558. doi: 10.1094/phyto-08-20-0325-r
Hernandez, J., Meints, B., and Hayes, P. (2020). Introgression breeding in barley: perspectives and case studies. Front. Plant Sci. 11:761. doi: 10.3389/fpls.2020.00761
Hickey, L. T., Hafeez, N. A., Robinson, H., Jackson, S. A., Leal-Bertioli, S. C. M., Tester, M., et al. (2019). Breeding crops to feed 10 billion. Nat. Biotechnol. 37, 744–754. doi: 10.1038/s41587-019-0152-9
Hill, C. B., Angessa, T. T., Zhang, X. Q., Chen, K., Zhou, G., Tan, C., et al. (2021). A global barley panel revealing genomic signatures of breeding in modern Australian cultivars. Plant J. 106, 419–434. doi: 10.1111/tpj.15173
Hoffman, N. E. (2021). Revisions to USDA biotechnology regulations: the SECURE rule. Proc. Natl. Acad. Sci. U. S. A. 118:1118. doi: 10.1073/pnas.2004841118
Holme, I. B., Brinch-Pedersen, H., Lange, M., and Holm, P. B. (2008). Transformation of different barley (Hordeum vulgare L.) cultivars by Agrobacterium tumefaciens infection of in vitro cultured ovules. Plant Cell Rep. 27, 1833–1840. doi: 10.1007/s00299-008-0605-y
Holme, I. B., Wendt, T., Gil-Humanes, J., Deleuran, L. C., Starker, C. G., Voytas, D. F., et al. (2017). Evaluation of the mature grain phytase candidate HvPAPhy_a gene in barley (Hordeum vulgare L.) using CRISPR/Cas9 and TALENs. Plant Mol. Biol. 95, 111–121. doi: 10.1007/s11103-017-0640-6
Hong, Y., Chen, L., Du, L. P., Su, Z., Wang, J., Ye, X., et al. (2014). Transcript suppression of TaGW2 increased grain width and weight in bread wheat. Funct. Integr. Genomics 14, 341–349. doi: 10.1007/s10142-014-0380-5
Hong, Y., Ni, S. J., and Zhang, G. P. (2020). Transcriptome and metabolome analysis reveals regulatory networks and key genes controlling barley malting quality in responses to drought stress. Plant Physiol. Biochem. 152, 1–11. doi: 10.1016/j.plaphy.2020.04.029
Jiménez, M. J. O., Uribe, J. F. C., and Almansa, J. (2013). Reflexiones EN torno A la importancia de la biodiversidad Y A los efectos del régimen colombiano de semillas. Compendium 16, 49–69.
Johansson, E., Henriksson, T., Prieto-Linde, M. L., Andersson, S., Ashraf, R., and Rahmatov, M. (2020). Diverse wheat-alien introgression lines as a basis for durable resistance and quality characteristics in bread wheat. Front. Plant Sci. 11:1067. doi: 10.3389/fpls.2020.01067
Jones, M. G. K., Fosu-Nyarko, J., Iqbal, S., Adeel, M., Romero-Aldemita, R., Arujanan, M., et al. (2022). Enabling trade in gene-edited produce in Asia and Australasia: the developing regulatory landscape and future perspectives. Plants (Basel) 11:2538. doi: 10.3390/plants11192538
Jouanin, A., Boyd, L., Visser, R. G. F., and Smulders, M. J. M. (2018). Development of wheat with Hypoimmunogenic gluten obstructed by the gene editing policy in Europe. Front. Plant Sci. 9:1523. doi: 10.3389/fpls.2018.01523
Kapusi, E., Corcuera-Gómez, M., Melnik, S., and Stoger, E. (2017). Heritable genomic fragment deletions and small Indels in the putative ENGase gene induced by CRISPR/Cas9 in barley. Front. Plant Sci. 8:540. doi: 10.3389/fpls.2017.00540
Kershen, D. L. (2015). Sustainability Council of new Zealand Trust v. the environmental protection authority: gene editing technologies and the law. GM Crops Food 6, 216–222. doi: 10.1080/21645698.2015.1122859
Khalil, S. R., Ashoub, A., Hussein, B. A., Brüggemann, W., Hussein, E. H., and Tawfik, M. S. (2021). Physiological and molecular studies on wild barley (Hordeum spontaneum) under salt stress. Plant Arch. 20, 9669–9681.
Khan, M. A., Altaf, S., Shafi, S., Bhat, B. A., Dar, W. A., Parry, F. A., et al. (2022). Exploration, collection and characterization of kala zeera (Bunium persicum Boiss. Fedtsch.) germplasm from northwestern Himalayas. Plant Genet. Resour. 20, 62–65. doi: 10.1017/s1479262122000028
Khoury, C. K., Brush, S., Costich, D. E., Curry, H. A., Haan, S., Engels, J. M. M., et al. (2022). Crop genetic erosion: understanding and responding to loss of crop diversity. New Phytol. 233, 84–118. doi: 10.1111/nph.17733
Komatsuda, T., Pourkheirandish, M., He, C., Azhaguvel, P., Kanamori, H., Perovic, D., et al. (2007). Six-rowed barley originated from a mutation in a homeodomain-leucine zipper I-class homeobox gene. Proc. Natl. Acad. Sci. U. S. A. 104, 1424–1429. doi: 10.1073/pnas.0608580104
Kumar, M., Singh, B., Jain, A., and Dhaka, A. (2017). Dual purpose barley–an effective solution for fodder scarcity in semi-arid region–A review. Forage Res. 42, 211–217.
Kumar, A., Verma, R. P. S., Singh, A., Kumar Sharma, H., and Devi, G. (2020). Barley landraces: ecological heritage for edaphic stress adaptations and sustainable production. Environ. Sustain. Indic. 6:100035. doi: 10.1016/j.indic.2020.100035
Kuriakose, S. V., Pushker, R., and Hyde, E. M. (2020). “Data-driven decisions for accelerated plant breeding” in Accelerated plant breeding, volume 1: cereal crops. eds. S. S. Gosal and S. H. Wani (Cham: Springer International Publishing), 89–119.
Lala, S., Amri, A., and Maxted, N. (2018). Towards the conservation of crop wild relative diversity in North Africa: checklist, prioritisation and inventory. Genet. Resour. Crop. Evol. 65, 113–124. doi: 10.1007/s10722-017-0513-5
Langridge, P. (2018). “Economic and academic importance of barley” in The barley genome. eds. N. Stein and G. J. Muehlbauer (Cham: Springer International Publishing), 1–10.
Lee, J. H., Won, H. J., Tran, H. N. P., Lee, S. M., Kim, H. Y., and Jung, J. H. (2021). Improving lignocellulosic biofuel production by CRISPR/Cas9-mediated lignin modification in barley. GCB Bioenergy 13, 742–752. doi: 10.1111/gcbb.12808
Lemmon, Z. H., Reem, N. T., Dalrymple, J., Soyk, S., Swartwood, K. E., Rodriguez-Leal, D., et al. (2018). Rapid improvement of domestication traits in an orphan crop by genome editing. Nat. Plants 4, 766–770. doi: 10.1038/s41477-018-0259-x
Lenaerts, B., Collard, B. C. Y., and Demont, M. (2019). Review: improving global food security through accelerated plant breeding. Plant Sci. 287:110207. doi: 10.1016/j.plantsci.2019.110207
Leopoldina, NADW. (2019). Towards a scientifically justified, differentiated regulation of genome edited plants in the EU. Köthen, Germany: Druckhaus Köthen GmbH & Co.
Li, Z., Xue, Y., Zhou, H., Li, Y., Usman, B., Jiao, X., et al. (2019). High-resolution mapping and breeding application of a novel brown planthopper resistance gene derived from wild rice (Oryza. Rufipogon Griff). Rice 12:41. doi: 10.1186/s12284-019-0289-7
Li, T., Yang, X., Yu, Y., Si, X., Zhai, X., Zhang, H., et al. (2018). Domestication of wild tomato is accelerated by genome editing. Nat. Biotechnol. 36, 1160–1163. doi: 10.1038/nbt.4273
Liveseed. (2021). Guidelines for adapted DUS and VCU testing of organic varieties. Available at: https://www.liveseed.eu/wp-content/uploads/2021/02/D2.4-LIVESEED-Guidelines-for-adapted-DUS-and-VCU-testing-of-organic-varietie.pdf.–(Accessed February 2023).
Louwaars, N. (2007). Seeds of confusion - the impact of policies on seed system, PhD. Netherlands, Wageningen University.
Louwaars, N. P. (2018). Plant breeding and diversity: A troubled relationship? Euphytica 214:114. doi: 10.1007/s10681-018-2192-5
Louwaars, N. P., and Manicad, G. (2022). Seed systems resilience–an overview. Seeds 1, 340–356. doi: 10.3390/seeds1040028
Lundqvist, U. (1997). New and revised descriptions of barley genes. Barley Genet. Newslett. 26, 22–516.
Mallapaty, S. (2019). Australian gene-editing rules adopt 'middle ground'. Nature. doi: 10.1038/d41586-019-01282-8
Migicovsky, Z., and Myles, S. (2017). Exploiting wild relatives for genomics-assisted breeding of perennial crops. Front. Plant Sci. 8:460. doi: 10.3389/fpls.2017.00460
Nadolska-Orczyk, A., Rajchel, I. K., Orczyk, W., and Gasparis, S. (2017). Major genes determining yield-related traits in wheat and barley. Theor. Appl. Genet. 130, 1081–1098. doi: 10.1007/s00122-017-2880-x
Nakamura, S., Pourkheirandish, M., Morishige, H., Kubo, Y., Nakamura, M., Ichimura, K., et al. (2016). Mitogen-activated protein kinase kinase 3 regulates seed dormancy in barley. Curr. Biol. 26, 775–781. doi: 10.1016/j.cub.2016.01.024
National Biosafety Authority Kenya. (2022). Guidelines for determining the regulatory process of genome editing techniques in Kenya. Kenya: National Biosafety Authority Kenya
Niazian, M., and Niedbała, G. (2020). Machine learning for plant breeding and biotechnology. Agriculture 10:436. doi: 10.3390/agriculture10100436
Orman-Ligeza, B., Harwood, W., Hedley, P. E., Hinchcliffe, A., Macaulay, M., Uauy, C., et al. (2020). TRA1: A locus responsible for controlling Agrobacterium-mediated transformability in barley. Front. Plant Sci. 11:355. doi: 10.3389/fpls.2020.00355
Palmgren, M. G., Edenbrandt, A. K., Vedel, S. E., Andersen, M. M., Landes, X., Østerberg, J. T., et al. (2015). Are we ready for back-to-nature crop breeding? Trends Plant Sci. 20, 155–164. doi: 10.1016/j.tplants.2014.11.003
Pan, R., Buitrago, S., Feng, Z., Abou-Elwafa, S. F., Xu, L., Li, C., et al. (2022). HvbZIP21, a novel transcription factor from wild barley confers drought tolerance by modulating ROS scavenging. Front. Plant Sci. 13:459. doi: 10.3389/fpls.2022.878459
Pan, R., Hu, H., Xiao, Y., Xu, L., Xu, Y., Ouyang, K., et al. (2023). High-quality wild barley genome assemblies and annotation with Nanopore long reads and hi-C sequencing data. Sci. Data 10:535. doi: 10.1038/s41597-023-02434-2
Pan, Y., Zhu, J., Hong, Y., Zhang, M., Lv, C., Guo, B., et al. (2021). Identification of novel QTL contributing to barley yellow mosaic resistance in wild barley (Hordeum vulgare spp. spontaneum). BMC Plant Biol. 21:560. doi: 10.1186/s12870-021-03321-x
Panting, M., Holme, I. B., Björnsson, J. M., Zhong, Y., and Brinch-Pedersen, H. (2021). CRISPR/Cas9 and transgene verification of gene involvement in unfolded protein response and recombinant protein production in barley grain. Front. Plant Sci. 12:755788. doi: 10.3389/fpls.2021.755788
Parisi, C., and Rodriguez-Cerezo, E. (2021). Current and future market applications of new genomic techniques. Luxemburg: European Commission.
Peschard, K. (2014). Farmers' rights and food sovereignty: critical insights from India. J. Peasant Stud. 41, 1085–1108. doi: 10.1080/03066150.2014.937338
Peschard, K., and Randeria, S. (2020). 'Keeping seeds in our hands': the rise of seed activism. J. Peasant Stud. 47, 613–647. doi: 10.1080/03066150.2020.1753705
Pourkheirandish, M., Hensel, G., Kilian, B., Senthil, N., Chen, G., Sameri, M., et al. (2015). Evolution of the grain dispersal system in barley. Cells 162, 527–539. doi: 10.1016/j.cell.2015.07.002
Ramsay, L., Comadran, J., Druka, A., Marshall, D. F., Thomas, W. T., Macaulay, M., et al. (2011). Intermedium-C, a modifier of lateral spikelet fertility in barley, is an ortholog of the maize domestication gene teosinte branched 1. Nat. Genet. 43, 169–172. doi: 10.1038/ng.745
Raubach, S., Kilian, B., Dreher, K., Amri, A., Bassi, F. M., Boukar, O., et al. (2021). From bits to bites: advancement of the germinate platform to support prebreeding informatics for crop wild relatives. Crop Sci. 61, 1538–1566. doi: 10.1002/csc2.20248
Rehman, S., Amouzoune, M., Hiddar, H., Aberkane, H., Benkirane, R., Filali-Maltouf, A., et al. (2021). Traits discovery in Hordeum vulgare sbsp. spontaneum accessions and in lines derived from interspecific crosses with wild Hordeum species for enhancing barley breeding efforts. Crop Sci. 61, 219–233. doi: 10.1002/csc2.20360
Ren, Q., Sretenovic, S., Liu, S., Tang, X., Huang, L., He, Y., et al. (2021). PAM-less plant genome editing using a CRISPR–SpRY toolbox. Nat. Plants 7, 25–33. doi: 10.1038/s41477-020-00827-4
Rolleston, W. (2019). Changing GM policy will be good for the environment and carbon zero. Wellington, New Zealand: Stuff.
Sadreddine, B. (2016). Yield and quality of dual-purpose barley and triticale in a semi-arid environment in Tunisia. Afr. J. Agric. Res. 11, 2730–2735. doi: 10.5897/AJAR2016.10803
Sakkour, A., Mascher, M., Himmelbach, A., Haberer, G., Lux, T., Spannagl, M., et al. (2022). Chromosome-scale assembly of barley cv. ‘Haruna Nijo’ as a resource for barley genetics. DNA Res. 29:1. doi: 10.1093/dnares/dsac001
Sallam, A. H., Tyagi, P., Brown-Guedira, G., Muehlbauer, G. J., Hulse, A., and Steffenson, B. J. (2017). Genome-wide association mapping of stem rust resistance in Hordeum vulgare subsp. spontaneum. G3 7, 3491–3507. doi: 10.1534/g3.117.300222
Sandhu, K. S., Lozada, D. N., Zhang, Z., Pumphrey, M. O., and Carter, A. H. (2021). Deep learning for predicting complex traits in spring wheat breeding program. Front. Plant Sci. 11:613325. doi: 10.3389/fpls.2020.613325
Sato, K., Mascher, M., Himmelbach, A., Haberer, G., Spannagl, M., and Stein, N. (2021). Chromosome-scale assembly of wild barley accession “OUH602”. G3 11:244. doi: 10.1093/g3journal/jkab244
Sato, K., Yamane, M., Yamaji, N., Kanamori, H., Tagiri, A., Schwerdt, J. G., et al. (2016). Alanine aminotransferase controls seed dormancy in barley. Nat. Commun. 7:11625. doi: 10.1038/ncomms11625
Schiemann, J., Robienski, J., Schleissing, S., Spok, A., Sprink, T., and Wilhelm, R. A. (2020). Editorial: plant genome editing - policies and governance. Front. Plant Sci. 11:284. doi: 10.3389/fpls.2020.00284
Schmalenbach, I., March, T. J., Bringezu, T., Waugh, R., and Pillen, K. (2011). High-resolution genotyping of wild barley introgression lines and fine-mapping of the Threshability locus thresh-1 using the Illumina GoldenGate assay. G3 (Bethesda) 1, 187–196. doi: 10.1534/g3.111.000182
Shah, J. M., Asgher, Z., Zeng, J., Quan, X., Ali, E., Shamsi, I. H., et al. (2017). Growth and physiological characterization of low nitrogen responses in Tibetan wild barley (Hordeum spontaneum) and cultivated barley (Hordeum vulgare). J. Plant Nutr. 40, 861–868. doi: 10.1080/01904167.2016.1262405
Shah, J. M., Muntaha, S. T., Ali, E., Khan, A. A., Zaidi, S. H. R., Shahzad, A. N., et al. (2019). Comparative study of the genetic basis of nitrogen use efficiency in wild and cultivated barley. Physiol. Mol. Biol. Plants 25, 1435–1444. doi: 10.1007/s12298-019-00714-z
Sharma, S., Upadhyaya, H., Varshney, R., and Gowda, C. (2013). Pre-breeding for diversification of primary gene pool and genetic enhancement of grain legumes. Front. Plant Sci. 4:309. doi: 10.3389/fpls.2013.00309
Shen, Y., Zhou, G., Liang, C., and Tian, Z. (2022). Omics-based interdisciplinarity is accelerating plant breeding. Curr. Opin. Plant Biol. 66:102167. doi: 10.1016/j.pbi.2021.102167
Shrestha, A., Fendel, A., Nguyen, T. H., Adebabay, A., Kullik, A. S., Benndorf, J., et al. (2022). Natural diversity uncovers P5CS1 regulation and its role in drought stress tolerance and yield sustainability in barley. Plant Cell Environ. 45, 3523–3536. doi: 10.1111/pce.14445
Singer, S. D., Laurie, J. D., Bilichak, A., Kumar, S., and Singh, J. (2021). Genetic variation and unintended risk in the context of old and new breeding techniques. Crit. Rev. Plant Sci. 40, 68–108. doi: 10.1080/07352689.2021.1883826
Singh, A. K., Ganapathysubramanian, B., Sarkar, S., and Singh, A. (2018). Deep learning for plant stress phenotyping: trends and future perspectives. Trends Plant Sci. 23, 883–898. doi: 10.1016/j.tplants.2018.07.004
Skov Kristensen, P., Dockter, C., Lundqvist, U., Lu, Q., Gregersen, P. L., Thordal-Christensen, H., et al. (2016). Genetic mapping of the barley lodging resistance locus Erectoides-k. Plant Breed. 135, 420–428. doi: 10.1111/pbr.12377
Sprink, T., Wilhelm, R., and Hartung, F. (2022). Genome editing around the globe: an update on policies and perceptions. Plant Physiol. 190, 1579–1587. doi: 10.1093/plphys/kiac359
Stockinger, E. J. (2021). The breeding of winter-hardy malting barley. Plan. Theory 10:1415. doi: 10.3390/plants10071415
Swamy, B. P. M., and Sarla, N. (2008). Yield-enhancing quantitative trait loci (QTLs) from wild species. Biotechnol. Adv. 26, 106–120. doi: 10.1016/j.biotechadv.2007.09.005
Swarup, S., Cargill, E. J., Crosby, K., Flagel, L., Kniskern, J., and Glenn, K. C. (2021). Genetic diversity is indispensable for plant breeding to improve crops. Crop Sci. 61, 839–852. doi: 10.1002/csc2.20377
Takeda, K., and Hori, K. (2007). Geographical differentiation and diallel analysis of seed dormancy in barley. Euphytica 153, 249–256. doi: 10.1007/s10681-006-9260-y
Taketa, S., Amano, S., Tsujino, Y., Sato, T., Saisho, D., Kakeda, K., et al. (2008). Barley grain with adhering hulls is controlled by an ERF family transcription factor gene regulating a lipid biosynthesis pathway. Proc. Natl. Acad. Sci. U. S. A. 105, 4062–4067. doi: 10.1073/pnas.0711034105
Taketa, S., Kikuchi, S., Awayama, T., Yamamoto, S., Ichii, M., and Kawasaki, S. (2004). Monophyletic origin of naked barley inferred from molecular analyses of a marker closely linked to the naked caryopsis gene (nud). Theor. Appl. Genet. 108, 1236–1242. doi: 10.1007/s00122-003-1560-1
Thabet, S. G., Moursi, Y. S., Karam, M. A., Börner, A., and Alqudah, A. M. (2020). Natural variation uncovers candidate genes for barley spikelet number and grain yield under drought stress. Genes 11:533. doi: 10.3390/genes11050533
Tiwari, M., Gautam, N., Indoliya, Y., Kidwai, M., Mishra, A. K., and Chakrabarty, D. (2022). A tau class GST, OsGSTU5, interacts with VirE2 and modulates the Agrobacterium-mediated transformation in rice. Plant Cell Rep. 41, 873–891. doi: 10.1007/s00299-021-02824-z
Tsuda, M., Watanabe, K. N., and Ohsawa, R. (2019). Regulatory status of genome-edited organisms under the Japanese Cartagena act. Front. Bioeng. Biotechnol. 7:387. doi: 10.3389/fbioe.2019.00387
Urnov, F. D., Rebar, E. J., Holmes, M. C., Zhang, H. S., and Gregory, P. D. (2010). Genome editing with engineered zinc finger nucleases. Nat. Rev. Genet. 11, 636–646. doi: 10.1038/nrg2842
Van De Wouw, M., Van Hintum, T., Kik, C., Van Treuren, R., and Visser, B. (2010). Genetic diversity trends in twentieth century crop cultivars: a meta analysis. Theor. Appl. Genet. 120, 1241–1252. doi: 10.1007/s00122-009-1252-6
Van Dijk, A. D. J., Kootstra, G., Kruijer, W., and De Ridder, D. (2021). Machine learning in plant science and plant breeding. iScience 24:101890. doi: 10.1016/j.isci.2020.101890
Van Hilten, A., Kushner, S. A., Kayser, M., Ikram, M. A., Adams, H. H. H., Klaver, C. C. W., et al. (2021). GenNet framework: interpretable deep learning for predicting phenotypes from genetic data. Commun. Biol. 4:1094. doi: 10.1038/s42003-021-02622-z
Van Tassel, D. L., Tesdell, O., Schlautman, B., Rubin, M. J., DeHaan, L. R., Crews, T. E., et al. (2020). New food crop domestication in the age of gene editing: genetic, agronomic and cultural change remain co-evolutionarily entangled. Front. Plant Sci. 11:789. doi: 10.3389/fpls.2020.00789
Verstegen, H., Köneke, O., Korzun, V., and Von Broock, R. (2014). “The world importance of barley and challenges to further improvements” in Biotechnological approaches to barley improvement. eds. J. Kumlehn and N. Stein (Berlin, Heidelberg: Springer Berlin Heidelberg), 3–19.
Vincent, H., Von Bothmer, R., Knüpffer, H., Amri, A., Konopka, J., and Maxted, N. (2012). Genetic gap analysis of wild Hordeum taxa. Plant Genet. Resour. 10, 242–253. doi: 10.1017/S1479262112000317
Von Bothmer, R., and Jacobsen, N. (1985). Origin, taxonomy, and related species. Barley 26, 19–56. doi: 10.2134/agronmonogr26.c2
Wairich, A., Wember, L. S., Gassama, L. J., Wu, L. B., Murugaiyan, V., Ricachenevsky, F. K., et al. (2021). Salt resistance of interspecific crosses of domesticated and wild rice species. J. Plant Nutr. Soil Sci. 184, 492–507. doi: 10.1002/jpln.202100068
Walton, R. T., Christie, K. A., Whittaker, M. N., and Kleinstiver, B. P. (2020). Unconstrained genome targeting with near-PAMless engineered CRISPR-Cas9 variants. Science 368, 290–296. doi: 10.1126/science.aba8853
Wang, Y., Chen, G., Zeng, F., Han, Z., Qiu, C. W., Zeng, M., et al. (2023). Molecular evidence for adaptive evolution of drought tolerance in wild cereals. New Phytol. 237, 497–514. doi: 10.1111/nph.18560
Wang, K., Shi, L., Liang, X., Zhao, P., Wang, W., Liu, J., et al. (2022). The gene TaWOX5 overcomes genotype dependency in wheat genetic transformation. Nat. Plants 8, 110–117. doi: 10.1038/s41477-021-01085-8
Wattnem, T. (2016). Seed laws, certification and standardization: outlawing informal seed systems in the global south. J. Peasant Stud. 43, 850–867. doi: 10.1080/03066150.2015.1130702
Wei, C., Liu, J., Yu, Z., Zhang, B., Gao, G., and Jiao, R. (2013). TALEN or Cas9 – rapid, efficient and specific choices for genome modifications. J. Genet. Genomics 40, 281–289. doi: 10.1016/j.jgg.2013.03.013
Wendt, T., Holme, I., Dockter, C., Preuß, A., Thomas, W., Druka, A., et al. (2016). HvDep1 is a positive regulator of culm elongation and grain size in barley and impacts yield in an environment-dependent manner. PLoS One 11:e0168924. doi: 10.1371/journal.pone.0168924
Whelan, A. I., Gutti, P., and Lema, M. A. (2020). Gene editing regulation and innovation economics. Front. Bioeng. Biotechnol. 8:303. doi: 10.3389/fbioe.2020.00303
Winge, T. (2012). A guide to EU legislation on the Marketing of Seed and Plant Propagating Material in the context of agricultural biodiversity. Norway: Fridtjof Nansen Institute.
Winston, E. I. (2008). What if seeds were not patentable? 321. Michigan: Michigan State Law Review, pp. 321–344.
Wu, X., Yue, W., Cai, K., Wang, H., Zeng, F., and Wang, J. (2022). Single-nucleotide polymorphisms in Bmy1 intron III alleles conferring the genotypic variations in β-amylase activity under drought stress between Tibetan wild and cultivated barley. Agronomy 12:1737. doi: 10.3390/agronomy12081737
Xie, K., Minkenberg, B., and Yang, Y. (2015). Boosting CRISPR/Cas9 multiplex editing capability with the endogenous tRNA-processing system. Proc. Natl. Acad. Sci. 112, 3570–3575. doi: 10.1073/pnas.1420294112
Xing, J., Zhang, D., Yin, F., Zhong, Q., Wang, B., Xiao, S., et al. (2021). Identification and fine-mapping of a new bacterial blight resistance gene, Xa47(t), in G252, an introgression line of Yuanjiang common wild Rice (Oryza rufipogon). Plant Dis. 105, 4106–4112. doi: 10.1094/pdis-05-21-0939-re
Xu, Y., Jia, Q., Zhou, G., Zhang, X.-Q., Angessa, T., Broughton, S., et al. (2017). Characterization of the sdw1 semi-dwarf gene in barley. BMC Plant Biol. 17, 11–10. doi: 10.1186/s12870-016-0964-4
Yamaguchi, K., Yamamoto, T., Segami, S., Horikawa, M., Chaya, G., Kitano, H., et al. (2020). gw2 mutation increases grain width and culm thickness in rice (Oryza sativa L.). Breed. Sci. 70, 456–461. doi: 10.1270/jsbbs.20018
Yan, J., and Wang, X. (2022). Machine learning bridges omics sciences and plant breeding. Trends Plant Sci. 28, 199–210. doi: 10.1016/j.tplants.2022.08.018
Yu, H., and Li, J. (2022). Breeding future crops to feed the world through de novo domestication. Nat. Commun. 13:1171. doi: 10.1038/s41467-022-28732-8
Yu, H., Lin, T., Meng, X., Du, H., Zhang, J., Liu, G., et al. (2021). A route to de novo domestication of wild allotetraploid rice. Cells 184, 1156–1170.e14. doi: 10.1016/j.cell.2021.01.013
Zahn, S., Koblenz, B., Christen, O., Pillen, K., and Maurer, A. (2020). Evaluation of wild barley introgression lines for agronomic traits related to nitrogen fertilization. Euphytica 216:39. doi: 10.1007/s10681-020-2571-6
Zeng, Z., Han, N., Liu, C., Buerte, B., Zhou, C., Chen, J., et al. (2020). Functional dissection of HGGT and HPT in barley vitamin E biosynthesis via CRISPR/Cas9-enabled genome editing. Ann. Bot. 126, 929–942. doi: 10.1093/aob/mcaa115
Zhang, H., Li, Y., and Zhu, J.-K. (2018). Developing naturally stress-resistant crops for a sustainable agriculture. Nat. Plants 4, 989–996. doi: 10.1038/s41477-018-0309-4
Zhang, B., Ma, L., Wu, B., Xing, Y., and Qiu, X. (2022). Introgression lines: valuable resources for functional genomics research and breeding in Rice (Oryza sativa L.). Frontiers. Plant Sci. 13:863789. doi: 10.3389/fpls.2022.863789
Zhao, X., Pan, S., Liu, Z., Han, Y., Zhang, Q., and Wang, K. (2022). Intelligent upgrading of plant breeding: decision support tools in the golden seed breeding cloud platform. Comput. Electron. Agric. 194:106672. doi: 10.1016/j.compag.2021.106672
Zheng, C., Abd-Elrahman, A., and Whitaker, V. (2021). Remote sensing and machine learning in crop phenotyping and management, with an emphasis on applications in strawberry farming. Remote Sens. 13:531. doi: 10.3390/rs13030531
Zhu, X.-G., and Zhu, J.-K. (2021). Precision genome editing heralds rapid de novo domestication for new crops. Cells 184, 1133–1134. doi: 10.1016/j.cell.2021.02.004
Zohary, D., Hopf, M., and Weiss, E. (2012). Domestication of plants in the Old World: The origin and spread of domesticated plants in Southwest Asia, Europe, and the Mediterranean Basin. Oxford: Oxford University Press on Demand.
Zomer, R. J., Xu, J., and Trabucco, A. (2022). Version 3 of the global aridity index and potential evapotranspiration database. Sci. Data 9:409. doi: 10.1038/s41597-022-01493-1
Zsögön, A., Čermák, T., Naves, E. R., Notini, M. M., Edel, K. H., Weinl, S., et al. (2018). De novo domestication of wild tomato using genome editing. Nat. Biotechnol. 36, 1211–1216. doi: 10.1038/nbt.4272
Keywords: crop wild relatives, re-domestication, food security, genebank accessions, genome editing regulations, new breeding techniques
Citation: Hanak T, Janjić J, Hay FR and Brinch-Pedersen H (2023) Genome editing to re-domesticate and accelerate use of barley crop wild relatives. Front. Sustain. Food Syst. 7:1331577. doi: 10.3389/fsufs.2023.1331577
Edited by:
Moslem Bahmankar, Agricultural Research, Education and Extension Organization (AREEO), IranReviewed by:
Giovanna Frugis, National Research Council (CNR), ItalyRasoul Sadeghi-Majd, University of Tehran, Iran
Mehdi Soltani Howyzeh, Islamic Azad University, Ahvaz, Iran
Mohsen Sarhangi, Seed and Plant Improvement Institute, Iran
Copyright © 2023 Hanak, Janjić, Hay and Brinch-Pedersen. This is an open-access article distributed under the terms of the Creative Commons Attribution License (CC BY). The use, distribution or reproduction in other forums is permitted, provided the original author(s) and the copyright owner(s) are credited and that the original publication in this journal is cited, in accordance with accepted academic practice. No use, distribution or reproduction is permitted which does not comply with these terms.
*Correspondence: Tobias Hanak, dG9iaWFzLmhhbmFrQGFncm8uYXUuZGs=
†These authors have contributed equally to this work and share first authorship