Field grand challenge for thermal engineering
- Department of Mechanical and Mechatronics Engineering, University of Waterloo, Waterloo, ON, Canada
Introduction
According to Merriam-Webster Dictionary (Merriam-Webster, 2022), the word “thermal” means relating to or caused by heat or by changes in temperature, or being or involving a state of matter dependent upon temperature; the word “engineering” means the application of science and mathematics by which the properties of matter and the sources of energy in nature are made useful to people through the design, manufacture, and use of complex products. Therefore, thermal engineering deals with the transport and utilization of thermal energy (often referred to as heat in daily language) in the design, manufacture, and use of products. In thermodynamics (Cengel and MA, 2006), thermal energy represents the energy stored or contained within a system (or matter) in a microscopically disorganized manner, while heat denotes the energy transfer between systems in a microscopically disorganized manner. Since systems can be chosen in an arbitrary manner suitable for analysis for different analysts, in daily language thermal energy and heat are often mixed in an interchangeable fashion.
Heat or heat transfer can occur through a medium or in vacuum. It can occur through a medium with or without macroscopically observable motion, commonly referred to as convection and conduction, respectively. Thermal radiation can propagate most efficiently in vaccum, but it is also possible through a medium that might be solid or gas. Further, heat can be transferred, with or without chemical reaction during the transfer process, into or from other forms of energy, such as chemical, mechanical, electrical, and so on. Therefore, thermal engineering is multi-disciplinary, involving fluid flow, heat and mass transfer, chemical reaction, and properties of the medium through which heat transfer occurs.
The quantity and direction of heat transfer are governed by thermodynamics. The first law of thermodynamics states that the energy can be transferred or transformed into another form of energy, but the total quantity of energy remains the same (i.e., conserved), while the second law of thermodynamics dictates the direction of heat transfer from a higher temperature system (or region) to a lower temperature one, and the quality of energy is degraded during the energy transformation process. The degraded energy (often referred to as waste energy, commonly in the form of heat) is dumped into our environment, causing environmental damage if the resilient limit of the environment is exceeded. The impact on the environment arising from waste energy dumping can exhibit in many different forms, such as local and global environmental changes like global climate change arising from greenhouse gas emissions, and pollution of air, water, and soil through chemical pollutant emissions.
The earliest accounts of utilizing heat, or fire, dated back to thousands of years ago, when cooking of foods lifted humans from among the animals, a major step in the development and evolution of human civilization. Cooking and heating using biomass as fuel had been the main application lasting thousands of years until the invention of the steam engine in the Industrial Revolution in the 1800s. In fact, as early as in the first century, Hero’s engine was invented as illustrated in Figure 1 (IAPWS, 1997), considered the first recorded steam engine, although it had not been a practical source of power and not a direct predecessor of steam engines in the industrial age. Since Industrial Revolution, various power-producing devices, categorized as heat engines, provide the energy and power for the social and economic development of human society. What is common to heat engines is the need for a source and a sink of heat, between which the engine is operated such that heat is transferred from its source to the engine, the engine converts a portion of the heat into useful work to power devices such as locomotives, land vehicles, marine ships and airplanes, and the remaining amount of heat is rejected to the sink. The heat from the source is typically obtained from the combustion of hydrocarbon-based fuels, which can occur outside of the engine in what is called an external combustion engine, and inside the engine itself in what is called an internal combustion engine. The sink to which waste heat is rejected is typically our environment, and heat rejection is commonly achieved via either heat exchangers to the ambient atmosphere or river/lake/ocean, or through the exhaust of combustion products to the ambient. The second law of thermodynamics first rules out the continuous operation of heat engines with only one heat source, or complete conversion of heat from its source to useful work. This indicates that waste heat rejection to our environment is inevitable from the operation of heat engines that have less than 100% efficiency in the energy conversion process.
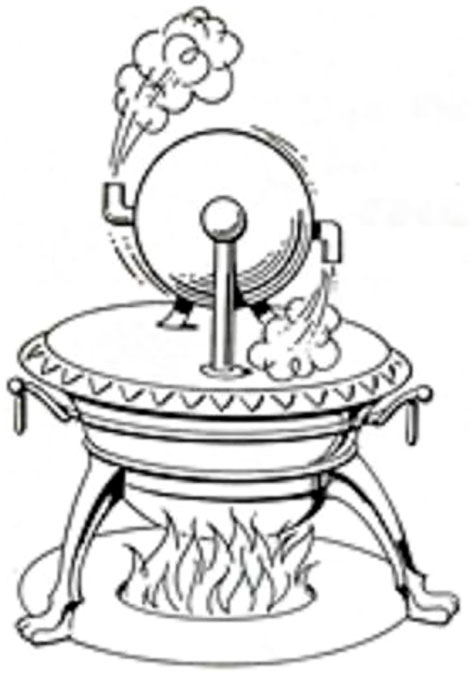
FIGURE 1. A schematic of Hero’s engine (IAPWS, 1997).
The first major account of environmental degradation due to the emissions arising from the combustion of hydrocarbon-based fuels was the Great Smog of London, which occurred in December 1952 in the form of severe air pollution mostly caused by the burning of coal (Stone, 2002). The smoke-like pollution was so toxic that it killed thousands of people, even reported to have choked cows to death in the fields; it reduced visibility so much that road, air, and rail transport was brought to a virtual standstill as well. This “Big Smoke” was a turning point in human civilization, and it made clear that the use of the combustion of fossil fuels was and still is a double-edged sword. Air pollution with severe smog has since been recognized for major metropolitans around the world, from Los Angeles to Beijing, New Delhi, and Mexico City. The recognition of the toxic chemical pollutants on the environment and health of humans and animals has been extended to the greenhouse gas emissions that cause the far more reaching effect of global climate change (IPCC, 2022), and global initiatives on the decarbonization of the energy sector have been on a considerable increase to moving into a new era of sustainable clean energy systems (The United nations Environment Programme, 2022), involving renewable energies and hydrogen fuel cells. The Lancet Commission on pollution and health (Landrigan et al., 2018) reports that “Pollution is the largest environmental cause of disease and premature death in the world today. Diseases caused by pollution were responsible for an estimated 9 million premature deaths in 2015–16% of all deaths worldwide—three times more deaths than from AIDS, tuberculosis, and malaria combined and 15 times more than from all wars and other forms of violence. In the most severely affected countries, pollution-related disease is responsible for more than one death in four.” The severe consequence related to the combustion of fossil fuels is recognized and it must be dealt with for the sustainability of our civilization. According to the recent multi-agency report coordinated by the World Meteorological Organization, United in Science (World Meteorological Organization, 2022), atmospheric greenhouse gas (GHG) concentration is reaching a historical record high, GHG emissions from fossil fuel combustion have exceeded the pre-pandemic levels, and national mitigation pledges for 2030 for lowering GHG emissions are insufficient, and would need to be four times higher to get on track to limit global warming to 2°C by the end of this century, and seven times higher to get on track to 1.5°C, the target of Paris Agreement (United Nations, 2015). A recent study (McKay et al., 2022) indicates that “even the Paris Agreement goal of limiting warming to well below 2°C and preferably 1.5°C is not safe” as this would risk crossing multiple climate tipping points; even global warming of 1°C, a threshold that has already passed, puts the world at risk by triggering some climate tipping points, leading to self-perpetuating changes in global climate. This further indicates the urgency of GHG emission reduction and the necessity of a fast transition to sustainable clean energy systems.
My own long career and interest in energy research date back to the fall of 1978 when I was admitted to Tianjin University for my undergraduate study, with a major in internal combustion engines. Tianjin University is the first Western-style university in China, and the internal combustion engine major ranks among the top both nationally and internationally—this major was a part of the Mechanical Engineering Department when I was admitted, then later on moved to the Engineering Thermophysics Department, which was renamed to the Thermal Engineering Department. After completing my Masters and PhD degrees at Northwestern University, Evanston, Illinois, United States, focusing on liquid fuel atomization and sprays, while also involved in a number of projects dealing with fluid flow, heat transfer, and combustion, I moved to Canada. In January 1992, I began my own academic career at the University of Victoria (UVic), on Vancouver Island, near Vancouver. While at UVic, I was among a large team of researchers working on a large fuel cell project sponsored by Ballard Power Systems Inc., the pioneer in modern fuel cell technology (Prater, 1990). Water and heat management remain critical issues for the scale-up and design of fuel cells for commercial applications. As one of the aftermaths arising from the 2008–2010 automotive industry crisis and as a means of combating climate change, the electrification of mobility and battery-electric vehicles became popular, opportunities arose for our research group to collaborate with the auto industry on the characterization and thermal management of various batteries, especially in the prevention of battery thermal runaway into fire hazards.
Enormous changes, both technologically and in attitudes, have occurred since I started my academic career in the field of energy and in particular thermal engineering. The focus was on increasing the energy conversion efficiency, to the reduction in chemical and GHG emissions, even if it means a sacrifice in energy efficiency, to decarbonization and electrification using renewable energies, and the storage of energy in the form of electric, chemical, electrochemical, etc. involving batteries and hydrogen fuel cells, among others. Significant technical progress ranges from fundamental energy materials to new devices and systems for the harvest of natural renewable energy resources such as solar, wind, hydro, biomass, geothermal, ocean wave, etc. The cost of electricity produced by solar photovoltaic panels and wind turbines has dropped dramatically and has become less than the cost of electricity produced by the traditional thermal power plants, making the possibility of green electricity for the electrification of mobility and heating/cooling sectors, for example. Enormous techno-economic progress has been achieved, and continues to be achieved, through advanced materials, optimal engineering design and automated volume manufacturing, complex characterization techniques, advanced real-time monitoring, sensing, diagnostic and prognostic devices and methods, and powerful computer modeling and simulations at different scales, from molecular dynamics simulations at the nanoscale, Lattice Boltzman methods at the microscale, to the computational fluid dynamics (CFD) analyses at the macroscale. From the viewpoint of energy conversion and power production, significant research focuses on the development and use of low carbon fuels such as natural gas, carbon-neutral fuels like biomass, biofuels and synthetic fuels, and non-carbon fuels including hydrogen and ammonia; advanced energy conversion techniques and devices such as hydrogen fuel cells, and internal combustion engines and gas turbines burning low- and carbon-neutral fuels as well as non-carbon fuels; carbon capture, utilization, and sequestration (CCUS), etc.
Opinions abound regarding the future direction of energy technology, and no energy technology is perfect as a consequence of the second law of thermodynamics that all energy processes involve energy degradation and energy loss that impact our environment in different but unfavorable manners. Dialogue, debate, and effective communication among the energy policymakers, industry, and academia will help to better understand the complex facets of energy and energy transition, and better shape the future directions; above all, it will help to better tackle the challenges accompanying energy use economically, environmentally and socially. As noted earlier, all energy processes would involve energy degradation into waste heat, hence all energy processes involve heat generation and transfer, or the scope of thermal engineering.
Thermal engineering is congenitally within mechanical engineering, but it is truly multidisciplinary, involving physics, chemistry, matters and materials as well as their property changes, materials science and engineering, chemical engineering, electrical and electronic engineering, process engineering, and mathematical modeling, among others. For example, the bottleneck to the miniaturization of IC (integrated circuits) chips is how to design such chips with effective transfer or dissipation of the enormous heat generated at a very small scale. Therefore, to manage the journal, it is necessary to divide it into sections, and surely there will be overlap among them as well. Frontiers in Thermal Engineering seeks to become a premier journal covering a complete range of relevant topics across the field of thermal science and engineering, including fundamental and basic research to applied and industry-focused engineering and technology development. A strong emphasis is given to applied aspects related to the design, development, demonstration, and implementation of components, devices, equipment, technologies, and systems involving thermal processes for the production, transmission, storage, utilization, conservation, and management of energy with improved energy efficiency and minimized energy footprint. The journal is particularly focused on addressing technological advances which support United Nations’ Sustainable Development Goal (SDG) #7: access to affordable, reliable, sustainable, and modern energy for all; and SDG#13: urgent action to combat climate change and its impacts, through disseminating and communicating scientific knowledge, impactful discoveries and technological innovation. At this moment, the following sections are covered with more to be introduced as they mature.
Advancements in cooling and heating
The cooling and heating (or heating in general) of built environments is directly related to the level of human comfort and quality of life. Heating is also essential for many industrial processes, including steel making, petrochemical, and chemical. Combined, heating represents almost half of global final energy consumption (IEA, 2021), and space and water heating accounts for over 40% of global energy consumption and related GHG emissions (IEA, 2021), because the heating needs are mostly met through the burning of fossil fuels at present. As such, decarbonization of heating is essential to achieve the target of net-zero GHG emissions by 2050; and the path to non-carbon heating includes electrification with renewable electricity via heat pumps and air-conditioning units, and the use of carbon-neutral and non-carbon fuels. Therefore, the “Advancements in Cooling and Heating” section will focus on fundamental and applied research involving refrigeration, heat pumps, thermal management, air-conditioning and psychrometry, environmentally friendly refrigerants, waste heat recovery and utilization, as well as heat storage using single-phase or phase-change materials. The emphasis is on new and emerging technologies as well as significant improvements to existing technologies. Aspects of heat and mass transfer that are related to cooling and heating are also integral parts of this section.
Combustion engineering
Combustion is perhaps the oldest discipline of science and technology that human civilization relies on, and is perhaps also among the least developed disciplines, as it involves many disciplines, including chemical thermodynamics, chemical kinetics, fluid flow, heat and mass transport, catalysis, etc. Improved understanding of combustion phenomena requires the development and maturity of others. Fire has been utilized for thousands of years, but the modern understanding of combustion phenomena began with Antoine Lavoisier’s pioneering work in the 18th century (Yount, 2008), for his discovery of the role oxygen plays in combustion, and his recognition and naming of oxygen (1778) and hydrogen (1783). The development of steam engines with external combustion led to the industrial revolution, the invention of internal combustion engines resulting in the economically important auto industry. Similarly, commercial aviation burgeons with gas turbine engines, and the space age began with rocket engines. Today an absolute majority of energy needs are still being met through the combustion of carbon-containing fuels, which is responsible for 87% of global GHG emissions (Ritchie et al., 2020). This requires the understanding of the mechanisms of formation for the chemical pollutants in the reaction zone, and the development of low- and zero-emission combustion devices and systems. The “Combustion Engineering” section will focus on the understanding of multi-physics and multi-scale combustion phenomena, employing advanced theoretical, experimental, and numerical techniques; the development of models of different complexities and accuracies for the design and operation of various combustion devices and systems; the adaptation of safe and efficient combustion devices and systems using carbon-neutral and carbon-free fuels including most importantly hydrogen and ammonia for zero emission applications; the development of oxy-combustion technologies facilitating carbon capture, utilization and sequestration; the energetic valorization of various organic waste streams generated daily for circular economy; and greening the power generation and propulsion systems for mobility applications, ranging from ground transport, to surface ships, aviation and space travel. The emphasis is also on the innovative new chemical energy conversion processes by better integrating pyrolysis, gasification, combustion and hydrothermal processes, and hybridization of processes including thermo-chemical conversion with electrochemical conversion, solar-thermal-chemical systems (such as solar fuels), waste to energy and to energy carrier systems, nuclear energy to hydrogen systems, to name a few. The main objective of this section is to archive the improved understanding, and interdisciplinary and integrative approaches to new designs and systems of combustion with reduced to zero emissions for climate change mitigation.
Heat engines
A heat engine derives heat from the combustion of a fuel with air at a high temperature T1, and converts a part of the heat into useful work (often in the form of power) while rejecting the remaining heat to the surrounding at a low temperature T2, hence it is also commonly referred to as 2T engine. The combustion process can occur outside of the engine itself in what is called external combustion engine, such as steam turbines and the Hero’s engine shown in Figure 1; for internal combustion engines the combustion occurs inside the engine itself, such as in gasoline and diesel engines which also involve a piston moving back and forward, hence known also as reciprocating engines. The working fluid inside the engine that absorbs and rejects heat can remain in a single gas phase, such as in gasoline and diesel engines as well as gas turbines, and it can also change phases between its liquid and vapor phases, such as in steam turbines and Organic Rankine Cycle (ORC). It is also possible to convert heat from a high temperature source at T1 into useful work, such as electric energy, without any moving parts in a thermoelectric power generator, while rejecting the remaining heat to a lower temperature source at T2.
The maximum energy efficiency of converting heat into work for a heat engine is limited by the Carnot efficiency as follows:
Therefore, possible approaches to increase engine efficiency are to increase the high temperature T1 and lower the low temperature T2. However, high temperature is limited by the materials for engine construction, developing materials with high temperature tolerance or coating the engine surface with insulation layers to prevent heat penetration into the engine parts are two possible material research. The low temperature T2 is limited by the ambient environment such as the temperature of atmospheric air, or river/lake/ocean water to which the waste heat is rejected. In reality, combustion of hydrocarbon fuels in air can yield T1 in the order of 2200 K, while the ambient temperature T2 is in the order of 300 K, thus the Carnot efficiency can be as high as over 86%. A practical heat engine is typically lower than half of this Carnot efficiency due to the heat lost to engine cooling fluid to maintain engine part temperature at a temperature with sufficient mechanical strength, and the heat contained in the exhaust stream. Therefore, recovery and utilization of waste heat from both the cooling and exhaust streams is an effective method to increase practical heat engine efficiency.
Historically the first modern internal combustion engine was invented back in 1804 by Isaac de Rivaz with a patent granted in Paris on 30 January 1807 (Patent Number 731); and he made “the world’s first internal combustion engine powered automobile” in 1808 (Eckermann, 2001). His internal combustion engine used hydrogen as fuel with electric ignition. However, today’s mainstay of internal combustion engines use petrol oils as fuel, and the liquid fuel is typically injected through an atomizer into the combustion chamber to disperse the liquid fuel into small droplets that vaporize quickly to form combustible fuel vapor-air mixture before combustion. Fuel injection and air management are important for engine performance and emission formation during the combustion process. Aftertreatment systems are important to reduce the emissions at the tailpipe to acceptable levels stipulated by regulation. To meet the net-zero emission target, carbon-neutral fuels like bio and synthetic fuels and carbon-free fuels such as hydrogen and ammonia are being considered as a replacement fuel. However, due to the changes in the fuel’s physical and chemical properties, the engine needs to be modified or designed completely new to optimize its performance and operation. For example, biofuels often have higher viscosity and surface tension, leading to difficulty in atomization, significant changes in the fuel injection and combustion process are required. Further, for the carbon-free fuel of hydrogen, an alternative electrochemical approach to energy conversion via fuel cells is gaining momentum with significant technical progress (Prater, 1990; Li, 2006), instead of burning hydrogen that presents many challenges for engine design and operation.
Heat transfer mechanisms and applications
Heat can be transferred in a number of manners, mostly categorized into conduction when heat transfer is mainly due to the random motion of the microscopic particles of the medium through which heat is transported; convection when heat is transferred mainly due to the macroscopically observable fluid motion; and thermal radiation when heat propagates in the form of electromagnetic waves. This categorization might be misleading, because in practical applications all these three modes of heat transfer are likely to occur simultaneously, commonly referred to as combined modes of heat transfer, and the interaction between each mode tends to make the phenomena and analysis complex and challenging. For example, the atmospheric air motion coupled with ocean currents and absorption, reflection, and transmission of radiation from the Sun and the Earth makes challenging the accurate prediction of local weather beyond 5 days, and global climate changes.
Thermal radiation is also unique in the sense that it can propagate through a medium (solid, liquid, or gas) or without a medium (that is, vacuum), and with wavelength dependence. In thermos-economic analysis, exergy or exergy efficiency is often used as an indicator of thermal system performance (Dincer and Rosen, 2007). Exergy for conduction and convection heat transfer can easily be expressed in terms of the amount of heat transferred and the temperature at which heat transfer occurs between the system and its reference surroundings, and exergy for radiation has also been treated in a similar manner (Petela, 1964; Candau, 2003). Since exergy represents the amount of useful energy the system contains that can be converted into work, and since solar photovoltaic cells can convert solar radiation with a suitable wavelength into electric energy, this also implies that the exergy of thermal radiation is wavelength dependent as well (Rodriguez et al., 2022). Further challenges to radiation heat transfer analysis arise from its propagation through an absorbing, reflecting, and transmitting medium that also involves other modes of heat transfer. Therefore, this section is mainly focused on the fundamental and applied research on all aspects of radiative and combined heat transfer, and the studies concerning phenomena and processes involving heat conduction, convection, and radiative transfer in the most general cases of semi-transparent dispersed media of different natures.
Micro- and nano-scale heat transfer
Conventional heat transfer, as discussed so far in this article, involves the transfer of thermal energy when the continuum assumption is valid; and it is modeled with the conservation of energy coupled with Fourier’s law for conduction. On the other hand, when the distance over which heat transfer occurs is comparable with or shorter than the characteristic length scales (mean free path length) of the system or/and the time over which heat transfer occurs is comparable with or shorter than the characteristic time scales (relaxation time) of the system, continuum model breaks because new phenomena that are important appear (Volz, 2007). Commonly, micro- and nano-scale heat transfer is referred to as the study of thermal energy transfer when the continuum model breaks down, although what matters here is the relative length and time scales involved rather than the absolute length scales. Understanding the heat transfer at micro- and nano-length scales has significant practical applications, such as thermal management of electronic, optical, and optoelectronic devices, development of novel micro- and nano-scale materials and systems, evaluation of thermal properties and performance of ultra-fine materials and modern miniaturized systems, and development of new materials with different thermal transport properties for energy conversion and utilization.
Thermal science and energy systems
Thermal science deals with heat and energy, or energy conversion involving heat and/or the changes in the thermal properties of the systems. It aims at achieving the maximum system performance and novel devices/sub-systems that enhance the performance and safety of the overall system. Clearly, this involves thermodynamics and heat transfer considering the interactions between physical and chemical processes. Thermal science is used in many practical applications, mostly related to energy systems for power generation and propulsion. Hence, the “Thermal Science and Energy Systems” section focuses on new ideas, measurement techniques, and findings in the field of heat and mass transfer and/or fluid flow, and technologies in the thermal engineering field, including novel energy materials, waste to energy, thermal energy storage technologies, energy measurement and management technologies, sustainability of energy systems, etc. Emphasis is placed on low-carbon environmental protection, climate change mitigation, and UN sustainable development goals.
Thermal system design
Thermal systems involve the storage and transfer of heat, and heat storage can be accomplished with the temperature change of the storage medium with or without phase change. The processes involved are largely governed by the principles of thermodynamics, heat transfer, and fluid mechanics. They arise in a wide range of practical applications, such as manufacturing, materials processing, power generation and propulsion, energy conversion, transportation, and built environment control. The design of thermal systems requires an integrated approach, and is often implemented through open-ended problems and design projects (Jaluria and Kulacki, 2018). Changes in design conditions (such as flow rates, inlet temperatures, and pressures) and component geometry (such as lengths, shapes, and configurations) on the system performance can be analyzed through modeling and simulation, as well as experimentation. This “Thermal System Design” section focuses on basic research and applied science across all aspects of process design and system integration for components, devices, equipment, and systems related to thermal engineering. Emphasis will be placed on the innovation, research, development, and demonstration of computer-aided process design methods and system-wide analysis for thermal systems.
Author contributions
XL completed this article.
Acknowledgments
XL gratefully acknowledges the Natural Sciences and Engineering Research Council of Canada and other federal and provincial funding agencies for their unwavering financial support of his research over the years of his academic career.
Conflict of interest
The author declares that the research was conducted in the absence of any commercial or financial relationships that could be construed as a potential conflict of interest.
Publisher’s note
All claims expressed in this article are solely those of the authors and do not necessarily represent those of their affiliated organizations, or those of the publisher, the editors and the reviewers. Any product that may be evaluated in this article, or claim that may be made by its manufacturer, is not guaranteed or endorsed by the publisher.
References
Candau, Y. (2003). On the exergy of radiation. Sol. Energy 75, 241–247. doi:10.1016/j.solener.2003.07.012
Cengel, Y. A., and MA, B. (2006). Thermodynamics, an engineering approach. 5th ed. New York: McGraw-Hill.
Dincer, I., and Rosen, M. A. (2007). Exergy as a driver for achieving sustainability. Int. J. Green Energy 1, 1–19. doi:10.1081/GE-120027881
Eckermann, E. (2001). World history of the automobile. Warrendale, PA: Society of Automotive Engineers. ISBN 0-7680-0800-X.
IAPWS (1997). Thermodynamic properties of water and steam. London: International Association for the Properties of Water and Steam IAPWS.
IEA (2021). Renewables 2021 analysis and forecast to 2026. Available at: https://www.iea.org/reports/renewables-2021.
IPCC 2022. The intergovernmental panel on climate change (IPCC) and its various assessment reports. Available at: https://cop23.unfccc.int/topics/science/workstreams/cooperation-with-the-ipcc. Accessed on September 1, 2022.
Jaluria, Y. (2018). Design of thermal systems. In Handbook of thermal science and engineering F. A. Kulacki (Editor). Germany: Springer. doi:10.1007/978-3-319-26695-4_67
Landrigan, P. J., Fuller, R., Acosta, N. J. R., Adeyi, O., Arnold, R., Basu, N. N., et al. (2018). The Lancet Commission on pollution and health. Lancet 391, 462–512. doi:10.1016/S0140-6736(17)32345-0
McKay, D. I. A., Staal, A., Abrams, J. F., Winkelmann, R., Sakschewski, B., Loriani, S., et al. (2022). Exceeding 1.5°C global warming could trigger multiple climate tipping points. Science 377, eabn7950. doi:10.1126/science.abn7950
Merriam-Webster 2022. Merriam-webster. Available at: https://www.merriam-webster.com/. Accessed on September 1, 2022.
Petela, R. (1964). Exergy of heat radiation. J. Heat. Transf. 86 (2), 187–192. doi:10.1115/1.3687092
Prater, K. (1990). The Renaissance of the solid polymer fuel cell. J. Power Sources 29, 239–250. doi:10.1016/0378-7753(90)80023-7
Ritchie, H., Roser, M., and Rosado, P. (2020) Energy. Published online at OurWorldInData.org. Retrieved from: https://ourworldindata.org/energy [Online Resource].
Rodriguez, E., Cardemil, J. M., Starke, A. R., and Escobar, R. (2022). Modelling the exergy of solar radiation: A review. Energies 15 (4), 1477. doi:10.3390/en15041477
Stone, R. (2002). Counting the cost of london’s killer smog. Science 13, 2106–2107. doi:10.1126/SCIENCE.298.5601.2106B
The United nations Environment Programme 2022. (UNEP) and its various reports. Available at: https://www.unep.org/. Accessed on September 1, 2022.
United Nations 2015. The Paris agreement. Available at: https://unfccc.int/sites/default/files/english_paris_agreement.pdf.
Volz, S. (Editor) (2007). Microscale and nanoscale heat transfer, topics appl. Physics (Berlin Heidelberg: Springer-Verlag), 107. doi:10.1007/11767862
World Meteorological Organization 2022. United in Science: We are heading in the wrong direction. Available at: https://public.wmo.int/en/media/press-release/united-science-we-are-heading-wrong-direction 13 September 2022.
Keywords: Energy, Thermal Energy, Thermal Engineering, Thermodynamics, Fluid Flow, Heat and Mass Transfer, Combustion, Emissions
Citation: Li X (2022) Field grand challenge for thermal engineering. Front. Therm. Eng. 2:1045838. doi: 10.3389/fther.2022.1045838
Received: 16 September 2022; Accepted: 20 September 2022;
Published: 20 October 2022.
Edited and Reviewed by:
Zhibin Yu, University of Glasgow, United KingdomCopyright © 2022 Li. This is an open-access article distributed under the terms of the Creative Commons Attribution License (CC BY). The use, distribution or reproduction in other forums is permitted, provided the original author(s) and the copyright owner(s) are credited and that the original publication in this journal is cited, in accordance with accepted academic practice. No use, distribution or reproduction is permitted which does not comply with these terms.
*Correspondence: Xianguo Li, x6li@uwaterloo.ca