- Neurological and Endocrine Toxicology Branch, Public Health and Integrated Toxicology Division, CPHEA/ORD, U.S. Environmental Protection Agency, Washington, NC, United States
Neuroelectrophysiology is an old science, dating to the 18th century when electrical activity in nerves was discovered. Such discoveries have led to a variety of neurophysiological techniques, ranging from basic neuroscience to clinical applications. These clinical applications allow assessment of complex neurological functions such as (but not limited to) sensory perception (vision, hearing, somatosensory function), and muscle function. The ability to use similar techniques in both humans and animal models increases the ability to perform mechanistic research to investigate neurological problems. Good animal to human homology of many neurophysiological systems facilitates interpretation of data to provide cause-effect linkages to epidemiological findings. Mechanistic cellular research to screen for toxicity often includes gaps between cellular and whole animal/person neurophysiological changes, preventing understanding of the complete function of the nervous system. Building Adverse Outcome Pathways (AOPs) will allow us to begin to identify brain regions, timelines, neurotransmitters, etc. that may be Key Events (KE) in the Adverse Outcomes (AO). This requires an integrated strategy, from in vitro to in vivo (and hypothesis generation, testing, revision). Scientists need to determine intermediate levels of nervous system organization that are related to an AO and work both upstream and downstream using mechanistic approaches. Possibly more than any other organ, the brain will require networks of pathways/AOPs to allow sufficient predictive accuracy. Advancements in neurobiological techniques should be incorporated into these AOP-base neurotoxicological assessments, including interactions between many regions of the brain simultaneously. Coupled with advancements in optogenetic manipulation, complex functions of the nervous system (such as acquisition, attention, sensory perception, etc.) can be examined in real time. The integration of neurophysiological changes with changes in gene/protein expression can begin to provide the mechanistic underpinnings for biological changes. Establishment of linkages between changes in cellular physiology and those at the level of the AO will allow construction of biological pathways (AOPs) and allow development of higher throughput assays to test for changes to critical physiological circuits. To allow mechanistic/predictive toxicology of the nervous system to be protective of human populations, neuroelectrophysiology has a critical role in our future.
Introduction
A proposal of future directions for application of neuroelectrophysiological techniques in toxicology must define some of the current and future problems facing the science of neurotoxicology. Currently (and in the foreseeable future), two major emphases in the field of neurotoxicology are: 1) mechanistic information and 2) human cognitive alterations (including diseases such as attention deficit hyperactivity disorder (ADHD), autism, learning disabilities, motor and sensory deficits, etc.). Both areas can be advanced through the systematic collection of targeted functional mechanistic data based on known or presumed biological pathways (Adverse Outcome Pathways (AOPs) in the field of neurotoxicology). Neurophysiology has the ability to bridge mechanistic data and behavioral changes—a critical linkage. As indicated below, the collection of mechanistic data to understand the function of the nervous system has been occurring for a long time.
Past
When considering future directions for neuroelectrophysiology as applied to neurotoxicology, it is beneficial to consider the historical progression of advancements and how the area of neurophysiology has contributed to the fields of neuroscience. Electrophysiology has provided a valuable basic understanding of nervous system function in neurobiology and neurotoxicology for centuries. The history of neurophysiology has been detailed in more exhaustive reviews and is only summarized here (Collura, 1993; Muley et al., 2009; Piccolino, 1998; Verkhratsky and Parpura, 2014). In the 1660’s, Dr. Jan Swammerdam dissected a frog leg and discovered that muscle fiber contraction could be induced by stimulation of nerve fibers (Muley et al., 2009; Verkhratsky et al., 2006). In 1791, Luigi Galvani published seminal work regarding nerve-muscle preparations in a frog, leading to the understanding of stimulus-response and muscle contractions (Galvani, 1791; Muley et al., 2009; Piccolino, 1998; Verkhratsky et al., 2006), and proposed that accumulation of positive and negative charges along the surface of muscles and nerve fibers resulted in “animal electricity”. Also using a nerve-muscle preparation, Leopoldo Nobili recorded the first evidence of the involvement of electrical activity (Nobili, 1828), although he apparently failed to appreciate the intrinsic biological origin. In about 1848, a crude recording of an action potential was made by Emile du Bois-Reymond (du Bois-Reymond, 1848). A few years later, a measure of nerve conduction involved in producing muscular contraction was made by Hermann Ludwig Ferdinand von Helmholtz in 1850 (Helmholtz, 1850a; Helmholtz, 1850b), and published with graphics in 1852 (Helmholtz, 1852). The first “true” measure of nerve conduction velocity (NCV) was published in 1868 by Julius Bernstein (Bernstein, 1868; Bernstein, 1871), who also verified that an action potential involved a charge movement which exceeded the resting membrane potential. Continuing the evolution of knowledge relating to nerve electrical activity, the involvement of ions (potassium) in nerve currents was proposed in 1912 (Bernstein, 1912). The theory of local circuits was proposed by Ludimar Hermann (Hermann, 1872; Hermann, 1873) postulating that a nerve contained a conductive “core”, an insulating sheath, and an external fluid medium, and that an electrical disturbance would result in nearby portions of the nerve completing current loops. However, it was Charles Overton who demonstrated that sodium ions were involved in the action potential overshoot (Overton, 1902). The electrical activity in single sensory fibers, and the encoding of stimulation intensity in muscles as the firing rate of the sensory fibers was reported in 1926 (Adrian, 1926; Adrian and Zotterman, 1926). This finding formed the basis for explaining how intensity could be encoded in the “all-or-none” triggering of what are today known as action potentials. The development of the voltage clamp technique allowed researchers to accurately monitor current flow across neuronal membranes (Cole, 1949; Marmont, 1949). The involvement of ionic sodium in action potentials was finally described by Hodgkin and Katz in 1949 using giant squid axons (Hodgkin and Katz, 1949), leading to the development of the Hodgkin-Huxley model for the ionic generation of action potentials (Hodgkin et al., 1952; Hodgkin and Huxley, 1952). The Hodgkin-Huxley model, coupled with the invention of the patch clamp technique in 1976 (Neher and Sakmann, 1976), allowed ionic flux thorough single channels to be studied. After approximately 185 years, the biophysical basis for the “animal electricity” proposed by Galvani was defined in detail.
While the above discoveries were obtained in peripheral nerve/muscle preparations, electrical activity in the brain was also being investigated. Early investigations involved brain stimulation and observing motor responses, which initiated the science of mapping the regions of differing brain function (Hitzig and Fritsch, 1870). In 1875 and 1877, Richard Canton recorded electrical activity from the brains of animals (Caton, 1875, Caton, 1877). These recordings involved Electroencephalogram (EEG) changes in sleep and awake states, as well as responses to auditory and somatosensory stimulation. These latter experiments may represent the first recordings of sensory evoked responses. The impact of peripheral stimulation on cortical desynchronization and recording responses localized on the cortex surface of animals was reported by Adolf Beck in 1890 (Beck, 1890; Coenen and Zayachkivska, 2013). Spontaneous human EEG recordings were first published by Hans Berger in 1929, documenting the importance of electrical activity in the human brain (Berger, 1929). In 1932, Dietsch introduced Fourier analysis of the EEG signal, a process that is still in use today to describe different EEG waveform bandwidths (Dietsch, 1932). The use of multichannel EEG recordings allowed description of spatial and temporal variations (Adrian and Matthews, 1934). As the techniques evolved, applications of EEG monitoring to localization of various brain waves was developed. An early medical application of human EEG involved localization of epileptic seizures, for subsequent surgical treatment (Jasper et al., 1940). These examples briefly show the valuable historical contribution of in vivo neurophysiology to the world of biology and medicine.
Present
Mechanistic Neuroelectrophysiology
Simultaneously with the advancement of knowledge of basic biological physiology, integration with pharmacology and toxicology was occurring. This, in turn, lead to investigations into the mechanism of action of various chemicals using neurophysiological techniques. In general, recordings can be made from single cells (allowing examination of cell action potentials, ion flux through channels) or field potentials from the extracellular space (that may represent activity from multiple neurons). Some basic descriptions of methods mentioned below and considerations in their application are summarized in Table 1.
Again, while the scope of this manuscript prevents exhaustive methodological and toxicological details, it is hoped the reader can use the examples provided as a basis for additional research. One of the first toxicological mechanistic studies was in 1857, when Claude Bernard used a neuromuscular preparation to show that curare interfered with neurotransmission at the level of the neuromuscular junction (Bernard, 1857; Gray, 1947). Mechanistic investigations can also be exemplified by proof of dichlorodiphenyltrichloroethane’s (DDT) effects on action potentials, which involved alterations at the level of sodium and potassium channels (Shanes, 1949; Narahashi and Yamasaki, 1960; Narahashi and Haas, 1967; Narahashi and Haas, 1968). Other investigators used in vitro preparations to demonstrate that mercury or lead altered presynaptic neurotransmitter release, presumably by interfering with calcium function (Manalis and Cooper, 1973; Manalis and Cooper, 1975; Kober and Cooper, 1976). In vivo neurophysiological measures of excitability and plasticity (indicated by population spikes, short- and long-term potentiation, kindling, etc … ) (Goddard et al., 1969; Lømo, 1971; Racine et al., 1972; Bliss and Lømo, 1973; Douglas and Goddard, 1975; Racine et al., 1983) opened the door for investigations related to network function and plasticity. Studies using these techniques illustrated the effect of lindane on GABA-mediated inhibition in the hippocampus (Joy and Albertson, 1985; Joy and Albertson, 1987; Joy et al., 1995). The effects of lindane, dieldrin, and endosulfan on increasing neuronal network excitability were also investigated using seizure models such as kindling (Joy et al., 1980; Joy et al., 1982; Gilbert, 1992, Gilbert, 1995; Gilbert and Mack, 1995). An increased decay of long-term potentiation was shown following long-term exposure to lead in drinking water (Gilbert and Mack, 1998). A paradigm known as paired-pulse inhibition was used to following treatment with cismethrin (Type I) or fenvalerate or deltamethrin (Type II) pyrethroids, and indicated changes in sodium channel kinetics supported the data better than changes in GABAergic function—providing a mechanistic basis for the altered network physiology (Gilbert et al., 1989). Although some authors have expressed limitations with respect to extrapolations to behavioral changes (Hölscher, 1997), neurophysiological phenomena such as long-term potentiation/depression have been used as models to study the neural plasticity associated with biological constructs such as learning and memory (Lynch, 2004; Nicoll, 2017; Abraham et al., 2019). In conjunction with methods discussed later in this paper, these types of studies addressed issues such as in vitro to in vivo extrapolation and the need to examine higher cognitive function, which are still pressing issues of concern in toxicology.
Evoked Potentials With Clinical Applications
While less mechanistic in nature than the above examples, other neurophysiological procedures, such as evoked potentials (EPs) have contributed to both the fields of neurobiology and toxicology. To record this type of neurophysiological response, a stimulus is presented to the test subject, and the time-locked signal of the nervous system is recorded (often involving signal averaging). As mentioned previously, this type of procedure may have first been used by Canton (Brazier, 1984; Caton, 1875; Caton, 1877). Evoked potentials have served as a neurological technique to characterize and help localize the neuroanatomical basis of neurotoxicity.
Assessment of changes in the peripheral nervous and muscular system has served to characterize the neurotoxicity of numerous chemicals. Examination of the nerve-muscle physiology can involve electromyographic (EMG) examination. These tests have been shown to be sensitive to several types of environmental toxicants or experimental manipulations, and many of these procedures have been described by others (Arezzo et al., 2011; Howard, 2013; McNeil et al., 2013) and will only be briefly described here. Perhaps the simplest test is of spontaneous EMG activity, which often increases in the presence of neurotoxicity or disease (Hnik et al., 1982; Ross and Lawhorn, 1990; Daube and Rubin, 2009). Stimulation of motor nerves allows recording of muscle action potentials (M-wave), which can be altered in the presence of neurotoxicity (Ross and Lawhorn, 1990). The elapsed time from the stimulus to the recorded neurophysiological response is known as the distal latency, and reflects the conduction velocity of the motor fibers in the stimulated nerve when the M-wave is recorded (Mallik and Weir, 2005). This measure has been shown to be a sensitive measure for intoxication with 2,5-hexanedione (Nachtman and Couri, 1984). A method known as single fiber jitter testing (voluntary or stimulation methods), measures the variability in neuromuscular transmission time between successive muscle action potentials (Stålberg and Sanders, 1981; Stålberg and Sonoo, 1994). Changes in neuromuscular jitter have been reported in mice after treatment with the organophosphates (OPs) mipafox or ecothiopate (Kelly et al., 1994). Decrements in muscle action potential amplitude after repetitive nerve stimulation (RNS) have been shown after treatment with the OPs dimethoate (Dongren et al., 1999), fenvalerate, or phoxim (Yang et al., 2001) or the depolarizing neuromuscular drug decamethonium (Finley et al., 2009). As reviewed by Le Quesne, changes in EMG responses have been shown following exposure to acrylamide, lead, organophosphates, or hexacarbons (Le Quesne, 1978; Hnik et al., 1982). Additional measures such as the F-wave (antidromic propagation along motor axons to the motor neuron cell, with subsequent firing and production of a small M-wave) (Panayiotopoulos and Chroni, 1996) and the H-reflex (stimulation of sensory fibers which then activate motor neurons at level of spinal cord, with subsequent firing of motor neurons and eliciting a M-Wave) (Cliffer et al., 1998b; Tucker et al., 2005) are also possible, although they may be technically challenging (Mattsson et al., 1984). The H-reflex may help disassociate sensory vs motor changes, due to the involvement of the sensory component, which should be absent/reduced in the M- or F-responses (Hamers et al., 1991; Cliffer et al., 1998a).
Often closely associated with electromyography is the assessment of peripheral nerve function (Arezzo et al., 2011). Nerve conduction velocity is the measure of the speed of action potential propagation along a nerve (stimulation and recording are along the nerve itself) and generally reflects the conduction speed of the largest diameter axons (Stålberg and Erdem, 2000). Changes in myelin will alter the normal saltatory conduction and change the NCV, whereas damage/death of the nerve will tend to alter the amplitude of the nerve action potential (Kimura, 1984; Hamers et al., 1991; Mattsson et al., 1992). Because the size and shape of a nerve action potential reflects the different constituent nerve fibers of the stimulated nerve (Gasser and Erlanger, 1927), changes in the distribution of conduction velocities may provide insight whether different types of nerve fibers are preferentially affected by a toxicant (Dorfman, 1984; Caccia et al., 1993; Ruijten et al., 1993). Examples of compounds that have been shown to alter nerve conduction and/or nerve action potentials include hexane (Howd et al., 1983; Rebert and Sorenson, 1983; Nylén et al., 1994), taxol (Cavaletti et al., 1995; Leandri et al., 2012), carbon disulfide (Herr et al., 1998), nitrile chemicals (Gagnaire and Marignac, 1999), hexachlorophene (DeJesus et al., 1978), and cisplatin (Rebert et al., 1984; Thompson et al., 1984; De Koning et al., 1987).
Stimulation of different sensory systems can be used to assess changes in somatosensory, auditory, and visual function (Dyer, 1985). Because the parts of the nervous system that generate these sensory EPs are generally known (Rebert, 1983; Mattsson and Albee, 1988; Herr and Boyes, 1995), the changes in EPs can help with neuroanatomical localization of altered physiological response, and can be integrated into a toxicological profile that includes targeted histopathological investigations (Mattsson et al., 1989a; Ross, 1989; Mattsson et al., 1990; Mattsson et al., 1992; Morgan et al., 2004; Arezzo et al., 2011). An example for the visual system is the electroretinogram (ERG). This response includes components reflecting activity at the levels of the photoreceptors, the bipolar-Müeller cells, and for pattern stimuli, may include ganglion cell components (Baker Jr et al., 1988; see; Herr and Boyes, 1995 for review; Heynen and Van Norren, 1985; Maffei and Fiorentini, 1986; Miura et al., 2009). As a second example, auditory stimulation allows recording of the brainstem auditory evoked response. The EP consists of a series of peaks that include physiological responses from the cochlear hair cells, the auditory nerve, cochlear nucleus, superior olivary complex lateral lemniscus, inferior colliculus, medial geniculate nucleus, and can include the auditory cortex (see Herr and Boyes, 1995; Mattsson et al., 1992 for reviews). The use of analogous neurophysiological techniques between laboratory animals and humans can assist in extrapolating effects across species (Hudnell et al., 1990; Benignus et al., 1991; Boyes, 1994). Additionally, test guidelines have been developed for using these techniques in a neurotoxicological setting (United States Environmental Protection Agency, 1998a; United States Environmental Protection Agency, 1998b). These guidelines cover the evaluation of peripheral nerve function, NCV, and sensory evoked potentials in toxicological studies for submission to the U.S. E.P.A., and can be adapted for acute, chronic, or developmental studies. These types of EP tests have been used to assess somatosensory alterations produced by dichloroacetylene (Albee et al., 1997), carbonyl sulfide (Herr et al., 2007), toluene or o-cresyl (Mattsson et al., 1989b), or hexane (Rebert and Sorenson, 1983). Changes in the auditory system have been shown following treatment with jet fuel coupled with noise exposure (Fechter et al., 2007), carbonyl sulfide (Morgan et al., 2004; Sills et al., 2004; Herr et al., 2007), chlordimeform or developmental glutamate (Janssen et al., 1983; Janssen et al., 1991), or polychlorinated biphenyls (Lilienthal et al., 2011; Poon et al., 2011). Changes in the ERG have been reported after exposure to methanol (Eells et al., 1996; Eells et al., 2000), cholinesterase inhibition (Jones et al., 1995), or lead (Fox and Rubinstein, 1989). Additionally, changes in the central nervous system function of the visual system (Boyes, 1992) have been shown following treatment with trichloroethylene (Boyes et al., 2005), carbon disulfide (Herr et al., 1992), 3,3′-iminodipropionitrile (Herr et al., 1995), and carbaryl or propoxur (Mwanza et al., 2008). The utility of these EP methods to detect, help localize the site of neurological dysfunction, and applicability to human neurology assures that such methods will continue to be applied in the future.
Neuroelectrophysiology and Cognitive Measures
Although largely in neuroscience and clinical settings, neurophysiological methods have also been used to study neural generators involved in cognitive processing of external stimuli using both methods based on recording EEG and/or evoked responses. The amount of literature regarding source localization (Grech et al., 2008; Asadzadeh et al., 2020) involving methods such as dipole source modeling (Wood, 1982; Koles, 1998), coherence measures (Nunez, 1995; Hoechstetter et al., 2004), neural networks (Abeyratne et al., 1991; Cui et al., 2019) and many other methods, is beyond the scope of this paper. However, such techniques (along with signal averaging) have been used to study associations between cognitive processing and neurophysiological responses. A negative peak recorded over the fronto-central regions of the brain at about 100 ms after stimulus delivery (N100) is proposed to be related to attentional processing, with the amplitude related to the salience of the stimuli (Haider et al., 1964; Hansen and Hillyard, 1980; Vogel and Luck, 2000). A negative potential at around 150 ms can be recorded after an incorrect response during tasks where the subject is required to identify a correct stimulus. It is recorded over the cingulate cortex and is thought to be a subconscious reflection of error monitoring (Falkenstein et al., 1991; Gehring et al., 1993; Carter et al., 1998). A negative potential recorded over the frontal cortex between 200–350 ms (N200) has been related to response inhibition, attention orientation, and error detection (Wijers et al., 1989; Jodo and Kayama, 1992). A well-studied positive potential occurring about 300 ms (P300) after a stimulus has been related to the “significance” of the stimulus, and has been related to constructs such as attention and working memory (Sutton et al., 1965; Verleger, 1988; Polich, 2007). These types of studies move the application of neurophysiology beyond neurotransmission and sensory perception, and into the realm of higher cognitive processing.
Future
Future directions for neurophysiology will incorporate the generation of data for functional changes as integrated into presumed, or known, biological pathways. Advances in in vitro high-throughput screening coupled with the recommendation of the National Research Council (NRC) (National.Research.Council, 2007; Krewski et al., 2010) has led to a resurgence in screening chemicals to rank and prioritize them for further testing. Critical to the success of this approach is the ability to relate the in vitro changes to adverse outcomes that are used for regulatory standards settings. Included in the realm of adverse outcomes are altered neurophysiology and changes in integrative functions such as cognitive abilities. Such a linkage can be incorporated into an AOP framework (Figure 1). Within this framework, neurophysiology plays a critical role in defining functional changes that can be related to both molecular/biochemical alterations, as well as behavioral changes in vivo.
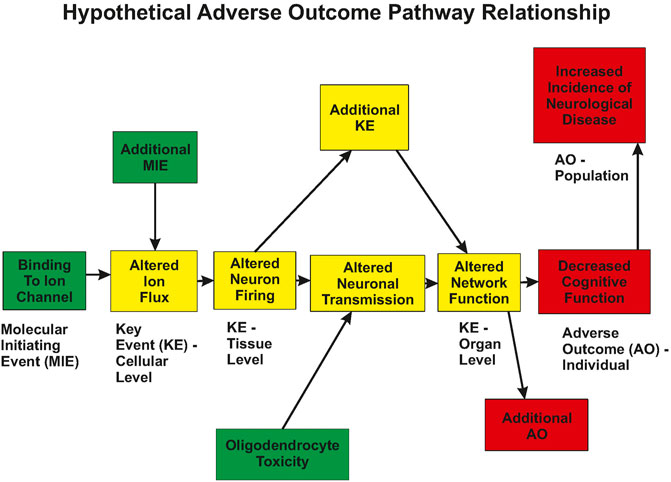
FIGURE 1. Hypothetical Adverse Outcome Pathway relationship. A xenobiotic interacts with biological tissue and results in a Molecular Initiating Event (MIE). This change in biology produces an alteration in a measurable Key Event (KE), which in turn, results in the subsequent change in additional Key Events. This progression leads to measurable changes at the cellular, tissue, and organ levels of biology. Note that multiple MIEs can impinge on a single KE, and KEs can interact in a network manner. Eventually, the biology is altered sufficiently to result in an Adverse Outcome (AO) that is of concern to society. Altered ion flux could be measured using patch clamp techniques, altered neuronal firing could be measured using multi-electrode arrays, altered network function could be measured using evoked potentials or EEG measures.
In Vitro Approaches
Several lines of research are already underway to use neurophysiological methods to screen for functional changes produced by toxicants. Examples include higher throughput patch clamp methods (Dunlop et al., 2008; Obergrussberger et al., 2016; Obergrussberger et al., 2018; Liu et al., 2019; Gao et al., 2020), in vitro cell culture multi-electrode arrays (MEA) (Mack et al., 2014; Zwartsen et al., 2018; Shafer, 2019), and use of alternative (non-mammalian) species such as zebrafish (Milan et al., 2006; Meyer et al., 2016), or Caenorhabditis elegans (C. elegans), (Richmond and Jorgensen, 1999; Goodman et al., 2012; Lockery et al., 2012). The use of MEAs has been proposed as a method to screen for seizurogenic potential of chemicals/drugs, and has been used in human tissue for epilepsy studies (Dossi et al., 2014; Meyer et al., 2016; Cho et al., 2017; Bradley and Strock, 2019; Fan et al., 2019). Additionally, MEAs have been proposed to have some utility in classifying possible mechanisms of actions of chemicals on the neuronal activity (Mack et al., 2014). Recently, MEA recordings have been used to provide in vitro evidence of altered neurophysiology in dorsal root ganglion cells (DRG) (Johnstone et al., 2020) based on in silico predictions (Melnikov et al., 2020). The continued development of the types of neurophysiological methods described above will allow scientists to detect and prioritize chemicals for further testing, based on changes in neuronal function, and to guide more targeted testing strategies with potential mechanistic information.
The future will require advancements using in vitro models to generate mechanistic data beyond screening applications. Applications using either human embryonic stem cells or induced pluripotent cells may allow better homology with human responses than rodent cells. Using cell culture conditions which allow development of electrically active three-dimensional (3D) cultures (Dingle et al., 2015; Sandström et al., 2017) may also help recapitulate the human nervous system, and such models have been proposed to study human neurodegenerative diseases (Jorfi et al., 2018; Faravelli et al., 2020). Such 3D models have been reported to have greater development of synaptic and ion transport mechanisms than two-dimensional cultures, suggesting that the neurons are in a higher state of maturation (Simão et al., 2018). In an example using electrical activity, the MEA activity of human neurospheres has been shown to be altered by methyl mercury, in the absence of changes in cell proliferation (Ylä-Outinen et al., 2010). Using a calcium flux measure, chemicals from multiple classes (drugs, flame retardants, industrial chemicals, poly-aromatic hydrocarbons, or pesticides) were tested in neurospheres and ranked for potency (Sirenko et al., 2019). Because this is a developing area of science, it is important to recognize the role and current limitations of electrophysiological measures in brain spheroids and brain-on-chip models (Forro et al., 2021). The technical issues such as planar vs. 3D electrodes, silicon electrodes, mesh electrodes, etc … are largely due to the relative recentness of this area of science. Many of these electrophysiological areas for advancement are similar as those for in vivo research (see below). All such in vitro models will require studies related to brain-related biology such as regional specific neural differences, hormonal influences on neuronal function, or gender-specific neuronal traits. Regional differences in neuronal composition may be assisted by bioprinting technologies using multiple cell types (see Zhuang et al., 2018 for review). However, the largest challenge will be inclusion of measures for cognitive function or emotion (Fritsche et al., 2018).
Although not restricted to electrophysiological preparations, methods will need to adequately not only model the diversity of neuronal cell types, but additional biological physiological functions will need to be included which can alter toxicokinetics for in vitro methods. While all areas of toxicology benefit from accurate modeling of chemical exposure, the blood-brain barrier (BBB) adds an additional level of complexity when considering the nervous system (Gumbleton and Audus, 2001; Bagchi et al., 2019). A developed BBB consists of endothelial cells connected by tight junctions, astrocytic projections, and the extracellular matrix, and can act as a barrier to passage of substances into the brain (Ward and Lamanna, 2004; van Der Helm et al., 2016). Additionally, transporter proteins such a p-glycoprotein can move substances out (or into) the brain (Banks, 1999). Inclusion of these additional cell types and proteins to in vitro or in silico models (Shityakov and Förster, 2018) will be required to improve dosimetry estimates. Advances in this area have included transwell systems (Stone et al., 2019) co-culture models, and microfluidic approaches using 3D cultures (Bagchi et al., 2019; Choi et al., 2020; Staicu et al., 2021). Such advances will need to be included in toxicity testing to closer reflect the biology of the in vivo situation.
In Vivo Neural Assessment
There have been some recent in vivo neurophysiological technique developments that allow assessment of both larger neurons and small sensory fibers and may provide some mechanistic insight into changes in peripheral motor or sensory nerve function. In contrast to traditional neurophysiological techniques, these methods use a series of nerve excitability tests that are translatable from humans to animal models. Several of the tests involve electrical conditioning pulses (some are 100 ms or longer) and track the stimulus intensity required to produce a criterion response in the nerve or muscle. The conditioning pulses activate of inactivate various ion channels, allowing some mechanistic interpretation of changes in neuronal responses (Bostock et al., 1998; Nodera and Kaji, 2006; Krishnan et al., 2008; Krishnan et al., 2009). These methods have been translated to both animal models and in vitro preparations (Maurer et al., 2007; Boërio et al., 2009; Mori et al., 2010; Nodera and Rutkove, 2012; Arnold et al., 2017), and have been begun to be used in a toxicological context. For example, the acute symptoms of treatment with oxaliplatin have been attributed to slowed inactivation of sodium channels (Heide et al., 2018), and changes in nerve excitability after Wallerian degeneration have been studied (Sawai et al., 2008). Importantly, these neurophysiological methods can be used repeatedly over time, allowing the onset and recovery of toxicological insult to be monitored (Nasu et al., 2014; Sung et al., 2014; Heide et al., 2018). Nerve excitability methods have also been used to assess small sensory fibers (Maurer et al., 2007; Howells et al., 2018), which are technically difficult to test (Bostock et al., 2003; George et al., 2007; Serra et al., 2010). Other investigators have used more traditional histopathological and neurophysiological techniques to assess small nerve fibers, using rectification of multiunit activity and binning the latencies into different ranges, to represent different conduction velocities (correlated with different sizes of nerves) (Zotova et al., 2008; Zotova and Arezzo, 2013). Recently, in vitro models for neurophysiological and histological assessment of rodent dorsal root ganglion or induced human motor nerve cells have been reported for assessment of chemotherapy-induced peripheral neuropathy with proposed expansion into environmental chemicals (Sharma et al., 2019; Anderson et al., 2021; Pollard et al., 2021). While these methods are not high throughput, continued development and application of such methods can start to bridge the mechanistic/functional gap in neurophysiological testing of sensory and motor fibers, which are known to be sensitive to toxicants (Le Quesne, 1978; London and Albers, 2007).
Network Connectivity
Another critical direction for neurophysiological investigations is the interrogation of network connectivity. Neurons, while affected by toxicants individually, function as part of an integrated network. For years, investigators have used in vitro approaches such as brain slices as a reductionist approach to study neuronal networks (Dingledine et al., 1980; Joy and Albertson, 1985; Joy and Albertson, 1987; Joy et al., 1989; Gilbert, 2004). Recently, technological advances have allowed patch clamp investigations in brain slices (including human slices) to look at network connectivity (Radnikow et al., 2011; Peng et al., 2019). Other investigators have used cell culture-based in vitro MEA to begin to look at formation of synaptic connectivity and network interactions between cells (Jimbo et al., 2003; Berdondini et al., 2006; Müller et al., 2015). Formation of functional networks on MEAs has been described (Erickson et al., 2008; Robinette et al., 2011), and the influence of chemicals to alter network-related endpoints such as coordinated bursting or synchronous firing has been investigated (Brown et al., 2016; Frank et al., 2018). A mechanistic hypothesis for synaptic plasticity in hippocampal neurons cultured on MEAs has been proposed to involve NMDA receptors and ERK1/2 signaling, along with gene transcription and protein synthesis, for maintenance of synchronous bursting for days (Arnold et al., 2005). The need for assessments of neuronal connectivity is critical in a developmental context, and can also be assessed using alternative species such as zebrafish (Miller et al., 2018). To truly assess the impact of chemicals on in vivo integrated neuronal function, it is essential to include network interactions as a future direction for both in vitro and in vivo work.
Assessment of complex neuronal function (such as cognitive or sensory alterations) using neurophysiological techniques will require incorporation of methods developed in basic neuroscience research with continued use and development of methods applicable to humans. As described earlier in this manuscript, neurophysiological methods to assess changes in sensory or cognitive processing in humans have been described (Hansen and Hillyard, 1980; Verleger, 1988; Wijers et al., 1989; Jodo and Kayama, 1992; Vogel and Luck, 2000; Polich, 2007). Assessment of sensory perception is likely to continue to rely on evoked response methods (as described above), with the inclusion of larger arrays of electrodes to allow assessment of changes in the topography of neural responses (Junghöfer et al., 2000; Robinson et al., 2017). Interrogation of complex sensory or cognitive processing will require assessment of networks of neurons and/or brain regions (Buzsáki, 2004; Urai et al., 2021). Neurophysiology is uniquely suited for recording neuronal responses in virtually “real time”, in contrast to the longer time periods usually required for in vivo imaging methods (D'Esposito et al., 1999; Sack and Linden, 2003; Trachtenberg et al., 2002). To adequately map neuronal network responses, an array of electrodes is necessary. In humans, these are typically surface electrodes and may be coupled with performance of a behavioral task (Bekker et al., 2004; Sänger et al., 2014). However, mechanistic studies to provide the biological basis for these responses will require animal models and often involve implanted electrodes.
The recording and interpretation of neuronal network activity in vivo is an ongoing effort in neuroscience. Advancements in electrode probe materials such as silicon (Senzai et al., 2019; Timme et al., 2021), carbon fiber (Kozai et al., 2012), or mesh probes that can be based on nanotechnology (Liu, 2015; Xie et al., 2015) have allowed long-term recordings of neuronal network responses. Multiple probes, each with multiple electrodes, allow sampling a three-dimensional array of neuronal activity. Multi-electrode methods allow assessment of the timing and correlation of firing between many neurons simultaneously in both anesthetized preparations and in alert and behaving animals. An eight probe array (with 64 recording sites) has been used to examine activity in the rat somatosensory and prefrontal cortex (Barthó et al., 2004). By examining the three-dimensional location of the neurons, the first movement of the autocorellogram, action potential waveform duration, and mean firing rates, the neurons were able to be classified as pyramidal cells or interneurons. A series of probes has been developed with over 5,000 recording sites, with 768 sites recorded simultaneously (Steinmetz et al., 2020) over an eight week period—allowing incorporation into future behavioral paradigms. Using a head-fixed preparation, about 30,000 neurons were recorded from 42 brain regions during multiple sessions of a visual discrimination task in mice. The regional brain responses to ipsilateral vs contralateral choices, and engagement timing were differentiated (Steinmetz et al., 2019). These multi-electrode methods allow assessment of cell outputs (action potentials) with great fidelity. However, it is much more difficult to measure the multiple inputs to a neuron’s dendrites and spines. One approach to looking for changes in cellular inputs can involve measuring the extracellular current flow using a technique known as current source density analysis (CSD) (Mitzdorf, 1985; Szymanski et al., 2009; Senzai et al., 2019). While CSD does not have widespread use in the neurotoxicology field, it has been used to show increased current flow into the supragranular layer of the auditory cortex in rats after an acute dose of salicylate (Stolzberg et al., 2012), indicating changes in intracortical microcircuits. Other investigators have used CSD analysis to show reduction in electrical sinks in the stratum moleculare and decreased distance from the peak inward current (sink) to the granule cell layer of the hippocampus, suggesting a loss of entorhinal afferents to the hippocampal outer molecular layer after 20 weeks of ethanol exposure (Abraham and Hunter, 1982). Therefore, application of multi-electrode analysis, coupled with CSD techniques can begin to assess both neuronal network circuitry and alterations in synaptic inputs (field potentials).
Cognitive Function
The assessment of changes in cognitive function produced by toxicants is an on-going challenge. Once again, principles and advances in neuroscience will have to be adapted to advance toxicological procedures. Knowing where and when to look for altered physiological responses will remain an important question. Combinations of technologies, such as EEG, magnetoencephalography, and fMRI, may be used to examine sources of cognitive responses (Min et al., 2020). The role of frequency-based assessment of EEG has been linked to communication between different brain regions, memory formation, and other cognitive processes in both human and animal models (Lachaux et al., 2012). In humans. decreased power in alpha and beta EEG frequencies, and reductions in P300 power, were found in high load working memory tasks (Chuang et al., 2019). However, investigations may also continue to rely on evoked responses to interrogate complex brain functions. As some examples, the P3 potential resulting from olfactory stimulation in humans was shown to be modulated by attention in the inferior frontal cortex, insula, and inferior temporal gyrus (Singh et al., 2019). Increased reaction time variability and a reduced amplitude of the P3 potential has been associated with increased ADHD Problems Scale scores on the Child Behavior Checklist (Liu et al., 2020). In rats, peak P2 was found to be related to target detection in an auditory go/no go task, but P3 was not altered (as frequently seen in humans). Increased low frequency power (1–7 Hz) was observed in the frontal cortex on hit trials, but 8–14 Hz power (alpha frequency range) was suppressed, compared to correct rejections (Nanda et al., 2020). These studies illustrate the power of neurophysiological techniques to study cognitive processing in real time, albeit with some differences between rodent models and humans, with an extensive range of cognitive processing remaining to be investigated.
Perturbation Methodology: Optogenetics
Using methods to perturb normal brain function in animal models will remain an important approach to uncover toxicological mechanisms. Traditional methods such as electrical stimulation, lesions, and pharmacological manipulations can still provide important mechanistic information (Nadler and Cuthbertson, 1980). A well-known caveat to lesion and electrical stimulation methods, is the non-specific nature of the technique. Not only are neuronal cell bodies affected, but so are axons of passage. Pharmacological manipulations can target specific types of neurons/receptors, etc … but may involve a relatively longer time scale of effects and may involve multiple brain regions (Cassaday et al., 1991; Singh et al., 1998; Berman et al., 2002).
Many of these limitations can be overcome with application of the relatively new technique of optogenetics, coupled with neurophysiology. Optogenetic tools allow targeting specific types of neurons with excitatory or inhibitory opsins, allowing neuron function to be controlled with light pulses in real time, and can be integrated with electrophysiology and neuroanatomical methods (Kim et al., 2017; Kuleshova, 2019). Optogenetic inhibition has been used to study the flow of neural network information from sensory to motor areas of the cortex in mice (Guo et al., 2014). Changes in EEG activity have also been examined using optogenetic techniques. For example, modulation of hippocampal theta activity by somatostatin positive, but not parvalbumin positive, GABAergic neurons in a mouse model of Alzheimer’s disease has been demonstrated (Chung et al., 2018). Optogenetic re-activation of dentate gyrus neurons that were first activated during fear conditioning, induced freezing behavior in a different environmental context—indicating that these specific neurons were contributing to the memory engram (Liu et al., 2012). Optogenetic stimulation of auditory presynaptic inputs to the lateral amygdala has been shown to serve as a conditioned stimulus (CS) during fear conditioning, substituting for an auditory stimulus. This study showed the importance of auditory inputs (traditional CS for fear training) to the lateral amygdala in fear memory formation (Kwon et al., 2014). The role of dopaminergic and GABAergic neurons in emotional reward (salience) during motivational behaviors has been dissected using optogenetic methods (Nieh et al., 2013). Closed-loop optogenetic techniques are under development that allow the brain’s neuronal activity to control the optogenetic stimulation of neurons, resulting in extremely naturalistic stimulation paradigms (Grosenick et al., 2015; Bolus et al., 2018). Additionally, optogenetic and neurophysiological techniques can be used in conjunction with other methods such as fMRI, voltage imaging, calcium imaging, and neurotransmitter release (Liang et al., 2015; Renault et al., 2015; Burmeister et al., 2018; Adam et al., 2019) to examine the mechanistic basis of changes produced by xenobiotics on complex brain network functions, such as learning and memory, in nearly real time. Application of these sorts of tools in a toxicological setting can provide valuable mechanistic information that is related to changes in behavior.
Conclusions
To adequately solve the problems facing neurotoxicology, the generation of mechanistic data to fill data gaps and allow the construction of AOP networks is needed. Understanding the biological pathways involved in toxicological alterations will enable the predictive validity of in vitro screens and the physiological relevance of omic-based changes produced by toxicants to be verified. This type of validity is essential for regulatory purposes and will increase the translational relevance to humans. Given the impetus to use in vitro methods as the basis for future risk assessments (National Research Council, 2007; Krewski et al., 2010), the benefit/cost of animal research needs to be considered. At the current time, there is insufficient scientific knowledge to adequately develop in vitro tests to adequately assess and protect higher cognitive functions. Thus, judicious and hypothesis-driven in vivo research to determine mechanistic key events in AOPs and Integrated Approaches to Testing and Assessments (Organisation for Economic Co-Operation and Development, 2016) will provide toxicological linkages for regulatory purposes and allow the development of batteries of in vitro assays to eventually replace the majority of animal testing. It is the change in brain function that is of concern to the public. Since the 1700’s, neurophysiology has been uniquely positioned to bridge the gap between mechanistic studies and in vivo alterations of the brain’s neuronal networks, helping to fulfill the promise of systems biology to protect human and ecological health.
Author Contributions
The author confirms being the sole contributor of this work and has approved it for publication.
Funding
This work was funded by the US EPA.
Author Disclaimer
The research described in this article has been reviewed by the Center for Public Health and Environmental Assessment, U.S. Environmental Protection Agency, and approved for publication. Approval does not signify that the contents necessarily reflect the views and policies of the Agency, nor does mention of trade names or commercial products constitute endorsement or recommendation for use.
Conflict of Interest
The author declares that the research was conducted in the absence of any commercial or financial relationships that could be construed as a potential conflict of interest.
Publisher’s Note
All claims expressed in this article are solely those of the authors and do not necessarily represent those of their affiliated organizations, or those of the publisher, the editors, and the reviewers. Any product that may be evaluated in this article, or claim that may be made by its manufacturer, is not guaranteed or endorsed by the publisher.
Acknowledgments
The author would like to thank William Boyes and Mary Gilbert for helpful discussions and critique of previous versions of this manuscript. The author would also like to acknowledge the support and encouragement provided by many colleagues both within the US EPA and the scientific community.
References
Abeyratne, U. R., Kinouchi, Y., Oki, H., Okada, J., Shichijo, F., and Matsumoto, K. (1991). Artificial Neural Networks for Source Localization in the Human Brain. Brain Topogr 4 (1), 3–21. doi:10.1007/BF01129661
Abraham, W. C., and Hunter, B. E. (1982). An Electrophysiological Analysis of Chronic Ethanol Neurotoxicity in the Dentate Gyrus: Distribution of Entorhinal Afferents. Exp. Brain Res. 47 (1), 61–68. doi:10.1007/BF00235887
Abraham, W. C., Jones, O. D., and Glanzman, D. L. (2019). Is Plasticity of Synapses the Mechanism of Long-Term Memory Storage? Npj Sci. Learn. 4 (1), 9. doi:10.1038/s41539-019-0048-y
Adam, Y., Kim, J. J., Lou, S., Zhao, Y., Xie, M. E., Brinks, D., et al. (2019). Voltage Imaging and Optogenetics Reveal Behaviour-dependent Changes in Hippocampal Dynamics. Nature 569 (7756), 413–417. doi:10.1038/s41586-019-1166-7
Adrian, E. D., and Matthews, B. H. C. (1934). The Interpretation of Potential Waves in the Cortex. J. Physiol. 81 (4), 440–471. doi:10.1113/jphysiol.1934.sp003147
Adrian, E. D. (1926). The Impulses Produced by Sensory Nerve Endings. J. Physiol. 61 (1), 49–72. doi:10.1113/jphysiol.1926.sp002273
Adrian, E. D., and Zotterman, Y. (1926). The Impulses Produced by Sensory Nerve Endings. J. Physiol. 61 (4), 465–483. doi:10.1113/jphysiol.1926.sp002308
Albee, R. R., Nitschke, K. D., Mattsson, J. L., and Stebbins, K. E. (1997). Dichloroacetylene: Effects on the Rat Trigeminal Nerve Somatosensory Evoked Potential. Neurotoxicology and Teratology 19 (1), 27–37. doi:10.1016/s0892-0362(96)00182-1
Anderson, W. A., Bosak, A., Hogberg, H. T., Hartung, T., and Moore, M. J. (2021). Advances in 3D Neuronal Microphysiological Systems: towards a Functional Nervous System on a Chip. In Vitro Cell.Dev.Biol.-Animal 57 (2), 191–206. doi:10.1007/s11626-020-00532-8
Arezzo, J. C., Litwak, M. S., and Zotova, E. G. (2011). Correlation and Dissociation of Electrophysiology and Histopathology in the Assessment of Toxic Neuropathy. Toxicol. Pathol. 39 (1), 46–51. doi:10.1177/0192623310390231
Arnold, F. J. L., Hofmann, F., Bengtson, C. P., Wittmann, M., Vanhoutte, P., and Bading, H. (2005). Microelectrode Array Recordings of Cultured Hippocampal Networks Reveal a Simple Model for Transcription and Protein Synthesis-dependent Plasticity. J. Physiol. 564 (1), 3–19. doi:10.1113/jphysiol.2004.077446
Arnold, R., Moldovan, M., Rosberg, M. R., Krishnan, A. V., Morris, R., and Krarup, C. (2017). Nerve Excitability in the Rat Forelimb: a Technique to Improve Translational Utility. J. Neurosci. Methods 275, 19–24. doi:10.1016/j.jneumeth.2016.10.013
Asadzadeh, S., Yousefi Rezaii, T., Beheshti, S., Delpak, A., and Meshgini, S. (2020). A Systematic Review of EEG Source Localization Techniques and Their Applications on Diagnosis of Brain Abnormalities. J. Neurosci. Methods 339, 108740. doi:10.1016/j.jneumeth.2020.108740
Bagchi, S., Chhibber, T., Lahooti, B., Verma, A., Borse, V., and Jayant, R. D. (2019). In-vitro Blood-Brain Barrier Models for Drug Screening and Permeation Studies: an Overview. Dddt 13, 3591–3605. doi:10.2147/dddt.s218708
Baker, C. L., Hess, R. R., Olsen, B. T., and Zrenner, E. (1988). Current Source Density Analysis of Linear and Non-linear Components of the Primate Electroretinogram. J. Physiol. 407 (1), 155–176. doi:10.1113/jphysiol.1988.sp017408
Banks, W. A. (1999). Physiology and Pathology of the Blood-Brain Barrier: Implications for Microbial Pathogenesis, Drug Delivery and Neurodegenerative Disorders. J. Neurovirol. 5 (6), 538–555. doi:10.3109/13550289909021284
Barthó, P., Hirase, H., Monconduit, L., Zugaro, M., Harris, K. D., and Buzsáki, G. (2004). Characterization of Neocortical Principal Cells and Interneurons by Network Interactions and Extracellular Features. J. Neurophysiol. 92 (1), 600–608. doi:10.1152/jn.01170.2003
Beck, A. (1890). Die Bestimmung der Localisation der Gehirn-und Ruckenmarksfunctionen vermittelst der elektrischen Erscheinungen. Centralblatt fur Physiologie 4, 473–476.
Bekker, E. M., Kenemans, J. L., and Verbaten, M. N. (2004). Electrophysiological Correlates of Attention, Inhibition, Sensitivity and Bias in a Continuous Performance Task. Clin. Neurophysiol. 115 (9), 2001–2013. doi:10.1016/j.clinph.2004.04.008
Benignus, V. A., Boyes, W. K., Hudnell, H. K., Frey, C. M., and Svendsgaard, D. J. (1991). Quantitative Methods for Cross-Species Mapping (CSM). Neurosci. Biobehavioral Rev. 15 (1), 165–171. doi:10.1016/s0149-7634(05)80110-1
Berdondini, L., Chiappalone, M., Van Der Wal, P. D., Imfeld, K., de Rooij, N. F., Koudelka-Hep, M., et al. (2006). A Microelectrode Array (MEA) Integrated with Clustering Structures for Investigating In Vitro Neurodynamics in Confined Interconnected Sub-populations of Neurons. Sensors Actuators B: Chem. 114 (1), 530–541. doi:10.1016/j.snb.2005.04.042
Berger, H. (1929). Über das Elektrenkephalogramm des Menschen. Archiv F. Psychiatrie 87 (1), 527–570. doi:10.1007/bf01797193
Berman, F. W., LePage, K. T., and Murray, T. F. (2002). Domoic Acid Neurotoxicity in Cultured Cerebellar Granule Neurons Is Controlled Preferentially by the NMDA Receptor Ca2+ Influx Pathway. Brain Res. 924 (1), 20–29. doi:10.1016/S0006-8993(01)03221-8
Bernard, C. (1857). Leçons sur les effets des substances toxiques et médicamenteuses. Paris: J.-B. Baillière et fils.
Bernstein, J. (1912). Historisches und Einleitung. Elektrobiologie. Braunschweig, Friedr: Vieweg und Sohn, 1–20. doi:10.1007/978-3-663-01627-4_1
Bernstein, J. (1868). Ueber den zeitlichen Verlauf der negativen Schwankung des Nervenstroms. Pflüger, Arch. 1 (1), 173–207. doi:10.1007/bf01640316
Bernstein, J. (1871). Untersuchungen über den Erregungsvorgang im Nerven-und Muskelsysteme. Heidelberg: Winter's Unisersitatsbuchhandlung.
Bliss, T. V. P., and Lømo, T. (1973). Long-lasting Potentiation of Synaptic Transmission in the Dentate Area of the Anaesthetized Rabbit Following Stimulation of the Perforant Path. J. Physiol. 232 (2), 331–356. doi:10.1113/jphysiol.1973.sp010273
Boërio, D., Greensmith, L., and Bostock, H. (2009). Excitability Properties of Motor Axons in the Maturing Mouse. J. Peripher. Nervous Syst. 14 (1), 45–53. doi:10.1111/j.1529-8027.2009.00205.x
Bolus, M. F., Willats, A. A., Whitmire, C. J., Rozell, C. J., and Stanley, G. B. (2018). Design Strategies for Dynamic Closed-Loop Optogenetic Neurocontrolin Vivo. J. Neural Eng. 15 (2), 026011. doi:10.1088/1741-2552/aaa506
Bostock, H., Campero, M., Serra, J., and Ochoa, J. (2003). Velocity Recovery Cycles of C Fibres Innervating Human Skin. J. Physiol. 553 (2), 649–663. doi:10.1113/jphysiol.2003.046342
Bostock, H., Cikurel, K., and Burke, D. (1998). Threshold Tracking Techniques in the Study of Human Peripheral Nerve. Muscle Nerve 21 (2), 137–158. doi:10.1002/(sici)1097-4598(199802)21:2<137:aid-mus1>3.0.co;2-c
Boyes, W. K. (1994). Rat and Human Sensory Evoked Potentials and the Predictability of Human Neurotoxicity from Rat Data. Neurotoxicology 15 (3), 569–578.
Boyes, W. K., Bercegeay, M., Krantz, T., Evans, M., Benignus, V., and Simmons, J. E. (2005). Momentary Brain Concentration of Trichloroethylene Predicts the Effects on Rat Visual Function. Toxicol. Sci. 87 (1), 187–196. doi:10.1093/toxsci/kfi242
Boyes, W. K. (1992). “Testing Visual System Toxicity Using Evoked Potential Technology,” in The Vulnerable Brain and Environmental Risks. Editors R. L. Isaacson, and K. F. Jensen (New York: Plenum Press), 193–222. doi:10.1007/978-1-4615-3326-9_9
Bradley, J. A., and Strock, C. J. (2019). Screening for Neurotoxicity with Microelectrode Array. Curr. Protoc. Toxicol. 79 (1), e67. doi:10.1002/cptx.67
Brazier, M. A. B. (1984). Pioneers in the Discovery of Evoked Potentials. Electroencephalography Clin. Neurophysiology/Evoked Potentials Section 59 (1), 2–8. doi:10.1016/0168-5597(84)90015-7
Brown, J. P., Hall, D., Frank, C. L., Wallace, K., Mundy, W. R., and Shafer, T. J. (2016). Editor's Highlight: Evaluation of a Microelectrode Array-Based Assay for Neural Network Ontogeny Using Training Set Chemicals. Toxicol. Sci. 154 (1), 126–139. doi:10.1093/toxsci/kfw147
Burmeister, J. J., Pomerleau, F., Quintero, J. E., Huettl, P., Ai, Y., Jakobsson, J., et al. (2018). In Vivo Electrochemical Studies of Optogenetic Control of Glutamate Signaling Measured Using Enzyme-Based Ceramic Microelectrode Arrays, Biochemical Approaches for Glutamatergic Neurotransmission. New York, NY: Humana Press, 327–351. doi:10.1007/978-1-4939-7228-9_11
Buzsáki, G. (2004). Large-scale Recording of Neuronal Ensembles. Nat. Neurosci. 7 (5), 446–451. doi:10.1038/nn1233
Caccia, M. R., Salvaggio, A., Dezuanni, E., Osio, M., Bevilacqua, M., Norbiato, G., et al. (1993). An Electrophysiological Method to Assess the Distribution of the Sensory Propagation Velocity of the Digital Nerve in normal and Diabetic Subjects. Electroencephalography Clin. Neurophysiology/Evoked Potentials Section 89 (2), 88–94. doi:10.1016/0168-5597(93)90089-8
Carter, C. S., Braver, T. S., Barch, D. M., Botvinick, M. M., Noll, D., and Cohen, J. D. (1998). Anterior Cingulate Cortex, Error Detection, and the Online Monitoring of Performance. Science 280 (5364), 747–749. doi:10.1126/science.280.5364.747
Cassaday, H., Hodges, H., and Gray, J. (1991). “The Effects of Pharmacological and Neurotoxic Manipulation of Serotonergic Activity on Latent Inhibition in the Rat: Implications for the Neural Basis of Acute Schizophrenia,” in Serotonin-related Psychiatric Syndrome: Clinical and Therapeutic Links. International Congress and Symposium Series. Editors G. Cassano, and H. Akiskal (Royal society of Medical Services Limited), 99–105.
Caton, R. (1877). Interim Report on Investigation of the Electric Currents of the Brain. Br. Med. J. 1, 62.
Cavaletti, G., Tredici, G., Braga, M., and Tazzari, S. (1995). Experimental Peripheral Neuropathy Induced in Adult Rats by Repeated Intraperitoneal Administration of Taxol. Exp. Neurol. 133 (1), 64–72. doi:10.1006/exnr.1995.1008
Cho, S.-J., Byun, D., Nam, T.-S., Choi, S.-Y., Lee, B.-G., Kim, M.-K., et al. (2017). A 3D-Printed Sensor for Monitoring Biosignals in Small Animals. J. Healthc. Eng. 2017 (1), 1–6. doi:10.1155/2017/9053764
Choi, J.-H., Santhosh, M., and Choi, J.-W. (2020). In Vitro blood–brain Barrier-Integrated Neurological Disorder Models Using a Microfluidic Device. Micromachines 11 (1), 21. doi:10.3390/mi11100905
Chuang, K.-Y., Chen, Y.-H., Balachandran, P., Liang, W.-K., and Juan, C.-H. (2019). Revealing the Electrophysiological Correlates of Working Memory-Load Effects in Symmetry Span Task with HHT Method. Front. Psychol. 10, 855. doi:10.3389/fpsyg.2019.00855
Chung, H., Park, K., Jang, H. J., Kohl, M. M., and Kwag, J., 2018. Optogenetic Activation of SST-Positive Interneurons Restores Hippocampal Theta Oscillation Impairment Induced by Soluble Amyloid Beta Oligomers In Vivo. bioRxiv, 465112.
Cliffer, K. D., Siuciak, J. A., Carson, S. R., Radley, H. E., Park, J. S., Lewis, D. R., et al. (1998a). Physiological Characterization of Taxol-Induced Large-Fiber Sensory Neuropathy in the Rat. Ann. Neurol. 43 (1), 46–55. doi:10.1002/ana.410430111
Cliffer, K. D., Tonra, J. R., Carson, S. R., Radley, H. E., Cavnor, C., Lindsay, R. M., et al. (1998b). Consistent Repeated M- and H-Wave Recording in the Hind Limb of Rats. Muscle Nerve 21 (11), 1405–1413. doi:10.1002/(sici)1097-4598(199811)21:11<1405:aid-mus7>3.0.co;2-d
Coenen, A., and Zayachkivska, O. (2013). Adolf Beck: A pioneer in Electroencephalography in between Richard. Adv. Cogn. Psychol. 9, 216–221. doi:10.2478/v10053-008-0148-3
Cole, K. S. (1949). Dynamic Electrical Characteristics of the Squid Axon Membrane. Arch. des Sci. Physiologiques 3 (2), 253–258.
Collura, T. F. (1993). History and Evolution of Electroencephalographic Instruments and Techniques. J. Clin. Neurophysiol. 10 (4), 476–504. doi:10.1097/00004691-199310000-00007
Cui, S., Duan, L., Gong, B., Qiao, Y., Xu, F., Chen, J., et al. (2019). EEG Source Localization Using Spatio-Temporal Neural Network. China Commun. 16 (7), 131–143. doi:10.23919/jcc.2019.07.011
D'Esposito, M., Zarahn, E., and Aguirre, G. K. (1999). Event-related Functional MRI: Implications for Cognitive Psychology. Psychol. Bull. 125 (1), 155–164. doi:10.1037/0033-2909.125.1.155
Daube, J. R., and Rubin, D. I. (2009). Needle Electromyography. Muscle Nerve 39 (2), 244–270. doi:10.1002/mus.21180
De Koning, P., Neijt, J. P., Jennekens, F. G. I., and Gispen, W. H. (1987). Evaluation of Cis-Diamminedichloroplatinum (II) (Cisplatin) Neurotoxicity in Rats. Toxicol. Appl. Pharmacol. 89 (1), 81–87. doi:10.1016/0041-008x(87)90178-5
DeJesus, C. P. V., Towfighi, J., and Snyder, D. R. (1978). Sural Nerve Conduction Study in the Rat: a New Technique for Studying Experimental Neuropathies. Muscle Nerve 1 (2), 162–167. doi:10.1002/mus.880010210
Dietsch, G. (1932). Fourier-analyse von elektrencephalogrammen des menschen. Pflügers Arch. 230 (1), 106–112. doi:10.1007/bf01751972
Dingle, Y.-T. L., Boutin, M. E., Chirila, A. M., Livi, L. L., Labriola, N. R., Jakubek, L. M., et al. (2015). Three-Dimensional Neural Spheroid Culture: AnIn VitroModel for Cortical Studies. Tissue Eng. C: Methods 21 (12), 1274–1283. doi:10.1089/ten.TEC.2015.0135
Dingledine, R., Dodd, J., and Kelly, J. S. (1980). The In Vitro Brain Slice as a Useful Neurophysiological Preparation for Intracellular Recording. J. Neurosci. Methods 2 (4), 323–362. doi:10.1016/0165-0270(80)90002-3
Dongren, Y., Tao, L., and Fengsheng, H. (1999). Electroneurophysiological Studies in Rats of Acute Dimethoate Poisoning. Toxicol. Lett. 107 (1-3), 249–254. doi:10.1016/s0378-4274(99)00054-5
Dorfman, L. J. (1984). The Distribution of Conduction Velocities (DCV) in Peripheral Nerves: a Review. Muscle Nerve 7 (1), 2–11. doi:10.1002/mus.880070103
Dossi, E., Blauwblomme, T., Nabbout, R., Huberfeld, G., and Rouach, N. (2014). Multi-electrode Array Recordings of Human Epileptic Postoperative Cortical Tissue. JoVE 92 (92), e51870. doi:10.3791/51870
Douglas, R. M., and Goddard, G. V. (1975). Long-term Potentiation of the Perforant Path-Granule Cell Synapse in the Rat hippocampus. Brain Res. 86 (2), 205–215. doi:10.1016/0006-8993(75)90697-6
Dunlop, J., Bowlby, M., Peri, R., Vasilyev, D., and Arias, R. (2008). High-throughput Electrophysiology: an Emerging Paradigm for Ion-Channel Screening and Physiology. Nat. Rev. Drug Discov. 7 (4), 358–368. doi:10.1038/nrd2552
Dyer, R. (1985). The Use of Sensory Evoked Potentials in Toxicology*1, *2. Fundam. Appl. Toxicol. 5 (1), 24–40. doi:10.1016/0272-0590(85)90048-X
Eells, J. T., Henry, M. M., Lewandowski, M. F., Seme, M. T., and Murray, T. G. (2000). Development and Characterization of a Rodent Model of Methanol-Induced Retinal and Optic Nerve Toxicity. Neurotoxicology 21 (3), 321–330.
Eells, J. T., Salzman, M. M., Lewandowski, M. F., and Murray, T. G. (1996). Formate-induced Alterations in Retinal Function in Methanol-Intoxicated Rats. Toxicol. Appl. Pharmacol. 140 (1), 58–69. doi:10.1006/taap.1996.0197
Erickson, J., Tooker, A., Tai, Y.-C., and Pine, J. (2008). Caged Neuron MEA: A System for Long-Term Investigation of Cultured Neural Network Connectivity. J. Neurosci. Methods 175 (1), 1–16. doi:10.1016/j.jneumeth.2008.07.023
Falkenstein, M., Hohnsbein, J., Hoormann, J., and Blanke, L. (1991). Effects of Crossmodal Divided Attention on Late ERP Components. II. Error Processing in Choice Reaction Tasks. Electroencephalography Clin. Neurophysiol. 78 (6), 447–455. doi:10.1016/0013-4694(91)90062-9
Fan, J., Thalody, G., Kwagh, J., Burnett, E., Shi, H., Lewen, G., et al. (2019). Assessing Seizure Liability Using Multi-Electrode Arrays (MEA). Toxicol. Vitro 55, 93–100. doi:10.1016/j.tiv.2018.12.001
Faravelli, I., Costamagna, G., Tamanini, S., and Corti, S. (2020). Back to the Origins: Human Brain Organoids to Investigate Neurodegeneration. Brain Res. 1727, 146561. doi:10.1016/j.brainres.2019.146561
Fechter, L. D., Gearhart, C., Fulton, S., Campbell, J., Fisher, J., Na, K., et al. (2007). JP-8 Jet Fuel Can Promote Auditory Impairment Resulting from Subsequent Noise Exposure in Rats. Toxicol. Sci. 98 (2), 510–525. doi:10.1093/toxsci/kfm101
Finley, D. B., Wang, X., Graff, J. E., and Herr, D. W. (2009). Single Fiber Electromyographic Jitter and Detection of Acute Changes in Neuromuscular Function in Young and Adult Rats. J. Pharmacol. Toxicol. Methods 59 (2), 108–119. doi:10.1016/j.vascn.2009.02.001
Forro, C., Caron, D., Angotzi, G., Gallo, V., Berdondini, L., Santoro, F., et al. (2021). Electrophysiology Read-Out Tools for Brain-On-Chip Biotechnology. Micromachines 12 (2), 124. doi:10.3390/mi12020124
Fox, D. A., and Rubinstein, S. D. (1989). Age-related Changes in Retinal Sensitivity, Rhodopsin Content and Rod Outer Segment Length in Hooded Rats Following Low-Level lead Exposure during Development. Exp. Eye Res. 48 (2), 237–249. doi:10.1016/s0014-4835(89)80073-9
Frank, C. L., Brown, J. P., Wallace, K., Wambaugh, J. F., Shah, I., and Shafer, T. J. (2018). Defining Toxicological Tipping Points in Neuronal Network Development. Toxicol. Appl. Pharmacol. 354, 81–93. doi:10.1016/j.taap.2018.01.017
Fritsche, E., Barenys, M., Klose, J., Masjosthusmann, S., Nimtz, L., Schmuck, M., et al. (2018). Current Availability of Stem Cell-Based In Vitro Methods for Developmental Neurotoxicity (DNT) Testing. Toxicol. Sci. 165 (1), 21–30. doi:10.1093/toxsci/kfy178
Gagnaire, F., and Marignac, B. (1999). Electrophysiological Deficiency in Peripheral Nerve Induced by Treatment for 12 Weeks with 2-Butenenitrile, 3-Butenenitrile, Cis-2-Pentenenitrile and 3, 3′-Iminodipropionitrile in Rats. Pharmacol. Toxicol. 84 (6), 247–254. doi:10.1111/j.1600-0773.1999.tb01490.x
Galvani, L. (1791). De Viribus Electricitatis in Motu Musculari. Commentarius. De Bonoiensi Scientiarum Artium Intituo Atque Academie Commentarii 7, 363–418.
Gao, J., Zhang, H., Xiong, P., Yan, X., Liao, C., and Jiang, G. (2020). Application of Electrophysiological Technique in Toxicological Study: from Manual to Automated Patch-Clamp Recording. Trac Trends Anal. Chem. 133, 116082. doi:10.1016/j.trac.2020.116082
Gasser, H. S., and Erlanger, J. (1927). The Rôle Played by the Sizes of the Constituent Fibers of a Nerve Trunk in Determining the Form of its Action Potential Wave. Am. J. Physiology-Legacy Content 80 (3), 522–547. doi:10.1152/ajplegacy.1927.80.3.522
Gehring, W. J., Goss, B., Coles, M. G. H., Meyer, D. E., and Donchin, E. (1993). A Neural System for Error Detection and Compensation. Psychol. Sci. 4 (6), 385–390. doi:10.1111/j.1467-9280.1993.tb00586.x
George, A., Serra, J., Navarro, X., and Bostock, H. (2007). Velocity Recovery Cycles of Single C Fibres Innervating Rat Skin. J. Physiol. 578 (1), 213–232. doi:10.1113/jphysiol.2006.116129
Gilbert, M. E., Mack, C. M., and Crofton, K. M. (1989). Pyrethroids and Enhanced Inhibition in the hippocampus of the Rat. Brain Res. 477 (1-2), 314–321. doi:10.1016/0006-8993(89)91420-0
Gilbert, M. E. (1992). A Characterization of Chemical Kindling with the Pesticide Endosulfan. Neurotoxicology and Teratology 14 (2), 151–158. doi:10.1016/0892-0362(92)90063-g
Gilbert, M. E. (2004). Alterations in Synaptic Transmission and Plasticity in Area CA1 of Adult hippocampus Following Developmental Hypothyroidism. Developmental Brain Res. 148 (1), 11–18. doi:10.1016/j.devbrainres.2003.09.018
Gilbert, M. E., and Mack, C. M. (1998). Chronic lead Exposure Accelerates Decay of Long-Term Potentiation in Rat Dentate Gyrus In Vivo. Brain Res. 789 (1), 139–149. doi:10.1016/s0006-8993(97)01517-5
Gilbert, M. E., and Mack, C. M. (1995). Seizure Thresholds in Kindled Animals Are Reduced by the Pesticides Lindane and Endosulfan. Neurotoxicology and teratology 17 (2), 143–150. doi:10.1016/0892-0362(94)00065-l
Gilbert, M. E. (1995). Repeated Exposure to Lindane Leads to Behavioral Sensitization and Facilitates Electrical Kindling. Neurotoxicology and Teratology 17 (2), 131–141. doi:10.1016/0892-0362(94)00064-k
Goddard, G. V., McIntyre, D. C., and Leech, C. K. (1969). A Permanent Change in Brain Function Resulting from Daily Electrical Stimulation. Exp. Neurol. 25 (3), 295–330. doi:10.1016/0014-4886(69)90128-9
Goodman, M. B., Lindsay, T. H., Lockery, S. R., and Richmond, J. E. (2012). Electrophysiological Methods for Caenorhabditis elegans Neurobiology. Methods Cel Biol. 107, 409–436. doi:10.1016/B978-0-12-394620-1.00014-X
Gray, T. C. (1947). The Use of D-Tubocurarine Chloride in Anaesthesia. Ann. R. Coll. Surg. Engl. 1 (4), 191–203.
Grech, R., Cassar, T., Muscat, J., Camilleri, K. P., Fabri, S. G., Zervakis, M., et al. (2008). Review on Solving the Inverse Problem in EEG Source Analysis. J. Neuroengineering Rehabil. 5 (1), 1–33. doi:10.1186/1743-0003-5-25
Grosenick, L., Marshel, J. H., and Deisseroth, K. (2015). Closed-loop and Activity-Guided Optogenetic Control. Neuron 86 (1), 106–139. doi:10.1016/j.neuron.2015.03.034
Gumbleton, M., and Audus, K. L. (2001). Progress and Limitations in the Use of In Vitro Cell Cultures to Serve as a Permeability Screen for the Blood-Brain Barrier. J. Pharm. Sci. 90 (11), 1681–1698. doi:10.1002/jps.1119
Guo, Z. V., Li, N., Huber, D., Ophir, E., Gutnisky, D., Ting, J. T., et al. (2014). Flow of Cortical Activity Underlying a Tactile Decision in Mice. Neuron 81 (1), 179–194. doi:10.1016/j.neuron.2013.10.020
Haider, M., Spong, P., and Lindsley, D. B. (1964). Attention, Vigilance, and Cortical Evoked-Potentials in Humans. Science 145 (3628), 180–182. doi:10.1126/science.145.3628.180
Hamers, F., van der Hoop, R. G., Steerenburg, P. A., Neijt, J. P., and Gispen, W. H. (1991). Putative Neurotrophic Factors in the protection of Cisplatin-Induced Peripheral Neuropathy in Rats. Toxicol. Appl. Pharmacol. 111 (3), 514–522. doi:10.1016/0041-008x(91)90255-d
Hansen, J. C., and Hillyard, S. A. (1980). Endogenous Brain Potentials Associated with Selective Auditory Attention. Electroencephalogr Clin. Neurophysiol. 49 (3-4), 277–290. doi:10.1016/0013-4694(80)90222-9
Heide, R., Bostock, H., Ventzel, L., Grafe, P., Bergmans, J., Fuglsang-Frederiksen, A., et al. (2018). Axonal Excitability Changes and Acute Symptoms of Oxaliplatin Treatment: In Vivo Evidence for Slowed Sodium Channel Inactivation. Clin. Neurophysiol. 129 (3), 694–706. doi:10.1016/j.clinph.2017.11.015
Helmholtz, H. (1852). Messungen über fortpflanzungsgeschwindigkeit der reizung in den nerven-zweite reihe. Arch. Anat. Physiol. Wiss Med., 199–216.
Helmholtz, H. (1850a). Note sur la vitesse de propagation de l’agent nerveux dans les nerfs rachidiens. CR Acadademy Sci. (Paris) 30, 204–206.
Helmholtz, H. (1850b). Über die Fortpflanzungsgeschwindigkeit der Nervenreizung [On the speed of nerve conduction]. Archiv für Anatomie, Physiologie und wissenschaftliche Medicin, 71–73.
Hermann, L. (1872). Ueber eine Wirkung galvanischer Ströme auf Muskeln und Nerven. Pflüger, Arch. 5 (1), 223–275. doi:10.1007/bf01675805
Hermann, L. (1873). Weitere Untersuchungen über den Electrotonus, insbesondere über die Erstreckung desselben auf die intramusculären Nervenenden. Pflüger, Arch. 7 (1), 301–322. doi:10.1007/bf01613330
Herr, D., Boyes, W. K., and Dyer, R. S. (1992). Alterations in Rat Flash and Pattern Reversal Evoked Potentials after Acute or Repeated Administration of Carbon Disulfide (CS2)*1, *2. Fundam. Appl. Toxicol. 18 (3), 328–342. doi:10.1016/0272-0590(92)90131-z
Herr, D., King, D., Barone, S., and Crofton, K. M. (1995). Alterations in Flash Evoked Potentials (FEPs) in Rats Produced by 3,3′-iminodipropionitrile (IDPN),. Neurotoxicology and Teratology 17 (6), 645–656. doi:10.1016/0892-0362(95)02007-1
Herr, D. W., Vo, K. T., Morgan, D. L., and Sills, R. C. (1998). Carbon Disulfide Neurotoxicity in Rats: VI. Electrophysiological Examination of Caudal Tail Nerve Compound Action Potentials and Nerve Conduction Velocity. Neurotoxicology 19 (1), 129–146.
Herr, D. W., and Boyes, W. K. (1995). “Electrophysiological Analysis of Complex Brain Systems,” in Neurotoxicology. Approaches and Methods. Editors L. W. Chang, and W. SlikkerJr. (New York: Academic Press), 205–221. doi:10.1016/b978-012168055-8/50013-3
Herr, D. W., Graff, J. E., Moser, V. C., Crofton, K. M., Little, P. B., Morgan, D. L., et al. (2007). Inhalational Exposure to Carbonyl Sulfide Produces Altered Brainstem Auditory and Somatosensory-Evoked Potentials in Fischer 344N Rats. Toxicol. Sci. 95 (1), 118–135. doi:10.1093/toxsci/kfl146
Heynen, H., and Van Norren, D. (1985). Origin of the Electroretinogram in the Intact Macaque Eye-II. Vis. Res. 25 (5), 709–715. doi:10.1016/0042-6989(85)90177-4
Hitzig, E., and Fritsch, G. (1870). Über die elektrische Erregbarkeit des Grosshirns. Arch. Anat. Physiol., 300–332.
Hnik, P., Vejsada, R., and Kasicki, S. (1982). EMG Changes in Rat Hind Limb Muscles Following Bilateral Deafferentation. Pflugers Arch. 395 (3), 182–185. doi:10.1007/BF00584806
Hodgkin, A. L., and Huxley, A. F. (1952). A Quantitative Description of Membrane Current and its Application to Conduction and Excitation in Nerve. J. Physiol. 117 (4), 500–544. doi:10.1113/jphysiol.1952.sp004764
Hodgkin, A. L., Huxley, A. F., and Katz, B. (1952). Measurement of Current‐voltage Relations in the Membrane of the Giant Axon of Loligo. J. Physiol. 116 (4), 424–448. doi:10.1113/jphysiol.1952.sp004716
Hodgkin, A. L., and Katz, B. (1949). The Effect of Sodium Ions on the Electrical Activity of the Giant Axon of the Squid. J. Physiol. 108 (1), 37–77. doi:10.1113/jphysiol.1949.sp004310
Hoechstetter, K., Bornfleth, H., Weckesser, D., Ille, N., Berg, P., and Scherg, M. (2003). BESA Source Coherence: a New Method to Study Cortical Oscillatory Coupling. Brain Topogr 16 (4), 233–238. doi:10.1023/b:brat.0000032857.55223.5d
Hölscher, C. (1997). Long-term Potentiation: a Good Model for Learning and Memory? Prog. Neuro-Psychopharmacology Biol. Psychiatry 21 (1), 47–68. doi:10.1016/s0278-5846(96)00159-5
Howard, J. F. (2013). Electrodiagnosis of Disorders of Neuromuscular Transmission. Phys. Med. Rehabil. Clin. North America 24 (1), 169–192. doi:10.1016/j.pmr.2012.08.013
Howd, R. A., Rebert, C. S., Dickinson, J., and Pryor, G. T. (1983). A Comparison of the Rates of Development of Functional Hexane Neuropathy in Weanling and Young Adult Rats. Neurobehav Toxicol. Teratol 5 (1), 63–68.
Howells, J., Bostock, H., Park, S. B., Kiernan, M. C., and Burke, D. (2018). Tracking Small Sensory Nerve Action Potentials in Human Axonal Excitability Studies. J. Neurosci. Methods 298, 45–53. doi:10.1016/j.jneumeth.2018.02.003
Hudnell, H. K., Boyes, W. K., and Otto, D. A. (1990). Rat and Human Visual-Evoked Potentials Recorded under Comparable Conditions: a Preliminary Analysis to Address the Issue of Predicting Human Neurotoxic Effects from Rat Data. Neurotoxicology and Teratology 12 (4), 391–398. doi:10.1016/0892-0362(90)90059-l
Janssen, R., Boyes, W. K., and Dyer, R. S. (1983). Effects of Chlordimeform on the Brainstem Auditory Evoked Response in Rats. Dev. Toxicol. Environ. Sci. 11, 533–536.
Janssen, R., Schweitzer, L., and Jensen, K. F. (1991). Glutamate Neurotoxicity in the Developing Rat Cochlea: Physiological and Morphological Approaches. Brain Res. 552 (2), 255–264. doi:10.1016/0006-8993(91)90090-i
Jasper, H. H., Kershman, J., and Elvidge, A. (1940). Electroencephalographic Studies of Injury to the Head. Arch. Neurpsych 44 (2), 328–350. doi:10.1001/archneurpsyc.1940.02280080088005
Jimbo, Y., Kasai, N., Torimitsu, K., Tateno, T., and Robinson, H. P. C. (2003). A System for MEA-Based Multisite Stimulation. IEEE Trans. Biomed. Eng. 50 (2), 241–248. doi:10.1109/TBME.2002.805470
Jodo, E., and Kayama, Y. (1992). Relation of a Negative ERP Component to Response Inhibition in a Go/No-Go Task. Electroencephalography Clin. Neurophysiol. 82 (6), 477–482. doi:10.1016/0013-4694(92)90054-l
Johnstone, A. F. M., Mack, C. M., Valdez, M. C., Shafer, T. J., LoPachin, R. M., Herr, D. W., et al. (2020). Acute In Vitro Effects on Embryonic Rat Dorsal Root Ganglion (DRG) Cultures by In Silico Predicted Neurotoxic Chemicals: Evaluations on Cytotoxicity, Neurite Length, and Neurophysiology. Toxicol. Vitro 69, 104989. doi:10.1016/j.tiv.2020.104989
Jones, R. D., Hamilton, B. F., and Dass, P. D. (1995). The Effects of Physostigmine on the Electroretinogram in the Beagle Dog. Vet. Res. Commun. 19 (2), 135–147. doi:10.1007/BF01839280
Jorfi, M., D'Avanzo, C., Kim, D. Y., and Irimia, D. (2018). Three-Dimensional Models of the Human Brain Development and Diseases. Adv. Healthc. Mater. 7 (1), 1700723. doi:10.1002/adhm.201700723
Joy, R. M., and Albertson, T. E. (1985). Effects of Lindane on Excitation and Inhibition Evoked in Dentate Gyrus by Perforant Path Stimulation. Neurobehav Toxicol. Teratol 7 (1), 1–8.
Joy, R. M., and Albertson, T. E. (1987). Interactions of Lindane with Synaptically Mediated Inhibition and Facilitation in the Dentate Gyrus. Neurotoxicology 8 (4), 529–542.
Joy, R. M., Stark, L. G., and Albertson, T. E. (1982). Proconvulsant Effects of Lindane: Enhancement of Amygdaloid Kindling in the Rat. Neurobehav Toxicol. Teratol 4 (3), 347–354.
Joy, R. M., Stark, L. G., Peterson, S. L., Bowyer, J. F., and Albertson, T. E. (1980). The Kindled Seizure: Production of and Modification by Dieldrin in Rats. Neurobehav Toxicol. 2 (2), 117–124.
Joy, R. M., Walby, W. F., Stark, L. G., and Albertson, T. E. (1995). Lindane Blocks GABAA-Mediated Inhibition and Modulates Pyramidal Cell Excitability in the Rat Hippocampal Slice. Neurotoxicology 16 (2), 217–228.
Joy, R. M., Albertson, T. E., and Ray, D. E. (1989). Type I and Type II Pyrethroids Increase Inhibition in the Hippocampal Dentate Gyrus of the Rat. Toxicol. Appl. Pharmacol. 98 (3), 398–412. doi:10.1016/0041-008x(89)90169-5
Junghöfer, M., Elbert, T., Tucker, D. M., and Rockstroh, B. (2000). Statistical Control of Artifacts in Dense Array EEG/MEG Studies. Psychophysiology 37 (4), 523–532.
Kelly, S. S., Mutch, E., Williams, F. M., and Blain, P. G. (1994). Electrophysiological and Biochemical Effects Following Single Doses of Organophosphates in the Mouse. Arch. Toxicol. 68 (7), 459–466. doi:10.1007/s002040050097
Kim, C. K., Adhikari, A., and Deisseroth, K. (2017). Integration of Optogenetics with Complementary Methodologies in Systems Neuroscience. Nat. Rev. Neurosci. 18 (4), 222–235. doi:10.1038/nrn.2017.15
Kimura, J. (1984). Principles and Pitfalls of Nerve Conduction Studies. Ann. Neurol. 16 (4), 415–429. doi:10.1002/ana.410160402
Kober, T. E., and Cooper, G. P. (1976). Lead Competitively Inhibits Calcium-dependent Synaptic Transmission in the Bullfrog Sympathetic Ganglion. Nature 262 (5570), 704–705. doi:10.1038/262704a0
Koles, Z. J. (1998). Trends in EEG Source Localization. Electroencephalography Clin. Neurophysiol. 106 (2), 127–137. doi:10.1016/s0013-4694(97)00115-6
Kozai, T. D. Y., Langhals, N. B., Patel, P. R., Deng, X., Zhang, H., Smith, K. L., et al. (2012). Ultrasmall Implantable Composite Microelectrodes with Bioactive Surfaces for Chronic Neural Interfaces. Nat. Mater 11 (12), 1065–1073. doi:10.1038/nmat3468
Krewski, D., Acosta, D., Andersen, M., Anderson, H., Bailar, J. C., Boekelheide, K., et al. (2010). Toxicity Testing in the 21st century: a Vision and a Strategy. J. Toxicol. Environ. Health B 13 (2-4), 51–138. doi:10.1080/10937404.2010.483176
Krishnan, A. V., Lin, C. S.-Y., Park, S. B., and Kiernan, M. C. (2008). Assessment of Nerve Excitability in Toxic and Metabolic Neuropathies. J. Peripher. Nerv Syst. 13 (1), 7–26. doi:10.1111/j.1529-8027.2008.00155.x
Krishnan, A. V., Lin, C. S.-Y., Park, S. B., and Kiernan, M. C. (2009). Axonal Ion Channels from Bench to Bedside: a Translational Neuroscience Perspective. Prog. Neurobiol. 89 (3), 288–313. doi:10.1016/j.pneurobio.2009.08.002
Kuleshova, E. P. (2019). Optogenetics - New Potentials for Electrophysiology. Neurosci. Behav. Physi 49 (2), 169–177. doi:10.1007/s11055-019-00711-5
Kwon, J.-T., Nakajima, R., Kim, H.-S., Jeong, Y., Augustine, G. J., and Han, J.-H. (2014). Optogenetic Activation of Presynaptic Inputs in Lateral Amygdala Forms Associative Fear Memory. Learn. Mem. 21 (11), 627–633. doi:10.1101/lm.035816.114
Lachaux, J.-P., Axmacher, N., Mormann, F., Halgren, E., and Crone, N. E. (2012). High-frequency Neural Activity and Human Cognition: Past, Present and Possible Future of Intracranial EEG Research. Prog. Neurobiol. 98 (3), 279–301. doi:10.1016/j.pneurobio.2012.06.008
Le Quesne, P. M. (1978). Clinical Expression of Neurotoxic Injury and Diagnostic Use of Electromyography. Environ. Health Perspect. 26, 89–95. doi:10.1289/ehp.782689
Leandri, M., Ghignotti, M., Emionite, L., Leandri, S., and Cilli, M. (2012). Electrophysiological Features of the Mouse Tail Nerves and Their Changes in Chemotherapy Induced Peripheral Neuropathy (CIPN). J. Neurosci. Methods 209 (2), 403–409. doi:10.1016/j.jneumeth.2012.07.005
Liang, Z., Watson, G. D. R., Alloway, K. D., Lee, G., Neuberger, T., and Zhang, N. (2015). Mapping the Functional Network of Medial Prefrontal Cortex by Combining Optogenetics and fMRI in Awake Rats. Neuroimage 117, 114–123. doi:10.1016/j.neuroimage.2015.05.036
Lilienthal, H., Heikkinen, P., Andersson, P. L., van der Ven, L. T. M., and Viluksela, M. (2011). Auditory Effects of Developmental Exposure to Purity-Controlled Polychlorinated Biphenyls (PCB52 and PCB180) in Rats. Toxicol. Sci. 122 (1), 100–111. doi:10.1093/toxsci/kfr077
Liu, C., Li, T., and Chen, J. (2019). Role of High‐Throughput Electrophysiology in Drug Discovery. Curr. Protoc. Pharmacol. 87 (1), e69. doi:10.1002/cpph.69
Liu, J., Fu, T.-M., Cheng, Z., Hong, G., Zhou, T., Jin, L., et al. (2015). Syringe-injectable Electronics. Nat. Nanotech 10 (7), 629–636. doi:10.1038/nnano.2015.115
Liu, X., Ramirez, S., Pang, P. T., Puryear, C. B., Govindarajan, A., Deisseroth, K., et al. (2012). Optogenetic Stimulation of a Hippocampal Engram Activates Fear Memory Recall. Nature 484 (7394), 381–385. doi:10.1038/nature11028
Liu, Y., Hanna, G. L., Hanna, B. S., Rough, H. E., Arnold, P. D., and Gehring, W. J. (2020). Behavioral and Electrophysiological Correlates of Performance Monitoring and Development in Children and Adolescents with Attention-Deficit/hyperactivity Disorder. Brain Sci. 10 (2), 79. doi:10.3390/brainsci10020079
Lockery, S. R., Hulme, S. E., Roberts, W. M., Robinson, K. J., Laromaine, A., Lindsay, T. H., et al. (2012). A Microfluidic Device for Whole-Animal Drug Screening Using Electrophysiological Measures in the Nematode C. elegans. Lab. Chip 12 (12), 2211–2220. doi:10.1039/c2lc00001f
Lømo, T. (1971). Patterns of Activation in a Monosynaptic Cortical Pathway: the Perforant Path Input to the Dentate Area of the Hippocampal Formation. Exp. Brain Res. 12 (1), 18–45.
London, Z., and Albers, J. W. (2007). Toxic Neuropathies Associated with Pharmaceutic and Industrial Agents. Neurol. Clin. 25 (1), 257–276. doi:10.1016/j.ncl.2006.10.001
Lynch, M. A. (2004). Long-term Potentiation and Memory. Physiol. Rev. 84 (1), 87–136. doi:10.1152/physrev.00014.2003
Mack, C. M., Lin, B. J., Turner, J. D., Johnstone, A. F. M., Burgoon, L. D., and Shafer, T. J. (2014). Burst and Principal Components Analyses of MEA Data for 16 Chemicals Describe at Least Three Effects Classes. Neurotoxicology 40, 75–85. doi:10.1016/j.neuro.2013.11.008
Maffei, L., and Fiorentini, A. (1986). Generator Sources of the Pattern ERG in Man and Animals. Front. Clin. Neurosci. 3, 101–116.
Mallik, A., and Weir, A. (2005). Nerve Conduction Studies: Essentials and Pitfalls in Practice. J. Neurol. Neurosurg. Psychiatry 76 (Suppl. 2), ii23–ii31. doi:10.1136/jnnp.2005.069138
Manalis, R. S., and Cooper, G. P. (1975). Evoked Transmitter Release Increased by Inorganic Mercury at Frog Neuromuscular junction. Nature 257 (5528), 690–691. doi:10.1038/257690a0
Manalis, R. S., and Cooper, G. P. (1973). Presynaptic and Postsynaptic Effects of lead at the Frog Neuromuscular junction. Nature 243 (5406), 354–356. doi:10.1038/243354a0
Marmont, G. (1949). Studies on the Axon Membrane. I. A New Method. J. Cel. Comp. Physiol. 34 (3), 351–382. doi:10.1002/jcp.1030340303
Mattsson, J., Albee, R. R., and Brandt, L. M. (1984). H-reflex Waveform and Latency Variability in Rats. Fundam. Appl. Toxicol. 4 (6), 944–948. doi:10.1016/0272-0590(84)90232-x
Mattsson, J., and Albee, R. (1988). Sensory Evoked Potentials in Neurotoxicology. Neurotoxicology and Teratology 10 (5), 435–443. doi:10.1016/0892-0362(88)90005-0
Mattsson, J. L., Albee, R. R., and Eisenbrandt, D. L. (1989a). Neurological Approach to Neurotoxicological Evaluation in Laboratory Animals. J. Am. Coll. Toxicol. 8 (2), 271–286. doi:10.3109/10915818909019552
Mattsson, J. L., Albee, R. R., and Gorzinski, S. J. (1989b). Similarities of Toluene and O-Cresol Neuroexcitation in Rats. Neurotoxicology and Teratology 11 (1), 71–75. doi:10.1016/0892-0362(89)90088-3
Mattsson, J. L., Boyes, W. K., and Ross, J. F. (1992). “Incorporating Evoked Potentials into Neurotoxicity Test Schemes,” in Neurotoxicology. Editors H. Tilson, and C. Mitchell (New York: Raven Press), 125–145.
Mattsson, J. L., Eisenbrandt, D. L., and Albee, R. R. (1990). Screening for Neurotoxicity: Complementarity of Functional and Morphologic Techniques. Toxicol. Pathol. 18 (11 Pt 2), 115–127. doi:10.1177/019262339001800117
Maurer, K., Bostock, H., and Koltzenburg, M. (2007). A Rat In Vitro Model for the Measurement of Multiple Excitability Properties of Cutaneous Axons. Clin. Neurophysiol. 118 (11), 2404–2412. doi:10.1016/j.clinph.2007.08.009
McNeil, C. J., Butler, J. E., Taylor, J. L., and Gandevia, S. C. (2013). Testing the Excitability of Human Motoneurons. Front. Hum. Neurosci. 7, 152. doi:10.3389/fnhum.2013.00152
Melnikov, F., Geohagen, B. C., Gavin, T., LoPachin, R. M., Anastas, P. T., Coish, P., et al. (2020). Application of the Hard and Soft, Acids and Bases (HSAB) Theory as a Method to Predict Cumulative Neurotoxicity. Neurotoxicology 79, 95–103. doi:10.1016/j.neuro.2020.04.009
Meyer, M., Dhamne, S. C., LaCoursiere, C. M., Tambunan, D., Poduri, A., and Rotenberg, A. (2016). Microarray Noninvasive Neuronal Seizure Recordings from Intact Larval Zebrafish. PLoS One 11 (6), e0156498. doi:10.1371/journal.pone.0156498
Milan, D. J., Jones, I. L., Ellinor, P. T., and MacRae, C. A. (2006). In Vivo recording of Adult Zebrafish Electrocardiogram and Assessment of Drug-Induced QT Prolongation. Am. J. Physiology-Heart Circulatory Physiol. 291 (1), H269–H273. doi:10.1152/ajpheart.00960.2005
Miller, G. W., Chandrasekaran, V., Yaghoobi, B., and Lein, P. J. (2018). Opportunities and Challenges for Using the Zebrafish to Study Neuronal Connectivity as an Endpoint of Developmental Neurotoxicity. Neurotoxicology 67, 102–111. doi:10.1016/j.neuro.2018.04.016
Min, B.-K., Hämäläinen, M. S., and Pantazis, D. (2020). New Cognitive Neurotechnology Facilitates Studies of Cortical-Subcortical Interactions. Trends Biotechnol. 38 (9), 952–962. doi:10.1016/j.tibtech.2020.03.003
Mitzdorf, U. (1985). Current Source-Density Method and Application in Cat Cerebral Cortex: Investigation of Evoked Potentials and EEG Phenomena. Physiol. Rev. 65 (1), 37–100. doi:10.1152/physrev.1985.65.1.37
Miura, G., Wang, M. H., Ivers, K. M., and Frishman, L. J. (2009). Retinal Pathway Origins of the Pattern ERG of the Mouse. Exp. Eye Res. 89 (1), 49–62. doi:10.1016/j.exer.2009.02.009
Morgan, D. L., Little, P. B., Herr, D. W., Moser, V. C., Collins, B., Herbert, R., et al. (2004). Neurotoxicity of Carbonyl Sulfide in F344 Rats Following Inhalation Exposure for up to 12 Weeks. Toxicol. Appl. Pharmacol. 200 (2), 131–145. doi:10.1016/j.taap.2004.04.013
Mori, A., Nodera, H., Shibuta, Y., Okita, T., Bostock, H., and Kaji, R. (2010). Threshold-dependent Effects on Peripheral Nerve In Vivo Excitability Properties in the Rat. Neurosci. Lett. 468 (3), 248–253. doi:10.1016/j.neulet.2009.11.006
Muley, N., Jain, G., Singh, J. N., and Sharma, S. S. (2009). Historical Events in Electrophysiology. Curr. Res. Inf. Pharmaceuticals Sci. (Crips) 10 (1), 2–8.
Müller, J., Ballini, M., Livi, P., Chen, Y., Radivojevic, M., Shadmani, A., et al. (2015). High-resolution CMOS MEA Platform to Study Neurons at Subcellular, Cellular, and Network Levels. Lab. Chip 15 (13), 2767–2780. doi:10.1039/c5lc00133a
Mwanza, J.-C., Finley, D., Spivey, C. L., Graff, J. E., and Herr, D. W. (2008). Depression of the Photic after Discharge of Flash Evoked Potentials by Physostigmine, Carbaryl and Propoxur, and the Relationship to Inhibition of Brain Cholinesterase. Neurotoxicology 29 (1), 87–100. doi:10.1016/j.neuro.2007.09.004
Nachtman, J. P., and Couri, D. (1984). An Electrophysiological Study of 2-hexanone and 2,5-hexanedione Neurotoxicity in Rats. Toxicol. Lett. 23 (2), 141–145. doi:10.1016/0378-4274(84)90118-8
Nanda, P., Morris, A., Kelemen, J., Yang, J., and Wiest, M. C. (2020). Evoked Frontal and Parietal Field Potential Signatures of Target Detection and Response Inhibition in Rats Performing an Equiprobable Auditory Go/no-Go Task. Eneuro 7 (1). doi:10.1523/ENEURO.0055-19.2019
Narahashi, T., and Haas, H. G. (1967). DDT: Interaction with Nerve Membrane Conductance Changes. Science 157 (3795), 1438–1440. doi:10.1126/science.157.3795.1438
Narahashi, T., and Haas, H. G. (1968). Interaction of DDT with the Components of Lobster Nerve Membrane Conductance. J. Gen. Physiol. 51 (2), 177–198. doi:10.1085/jgp.51.2.177
Narahashi, T., and Yamasaki, T. (1960). Mechanism of Increase in Negative After-Potential by Dicophanum (DDT) in the Giant Axons of the Cockroach*. J. Physiol. 152 (1), 122–140. doi:10.1113/jphysiol.1960.sp006475
Nasu, S., Misawa, S., Nakaseko, C., Shibuya, K., Isose, S., Sekiguchi, Y., et al. (2014). Bortezomib-induced Neuropathy: Axonal Membrane Depolarization Precedes Development of Neuropathy. Clin. Neurophysiol. 125 (2), 381–387. doi:10.1016/j.clinph.2013.07.014
National.Research.Council, (2007). Toxicity Testing in the 21st Century: A Vision and a Strategy. Washington, D.C.: National Academies Press, 0309109922.
Neher, E., and Sakmann, B. (1976). Single-channel Currents Recorded from Membrane of Denervated Frog Muscle Fibres. Nature 260 (5554), 799–802. doi:10.1038/260799a0
Nicoll, R. A. (2017). A Brief History of Long-Term Potentiation. Neuron 93 (2), 281–290. doi:10.1016/j.neuron.2016.12.015
Nieh, E. H., Kim, S.-Y., Namburi, P., and Tye, K. M. (2013). Optogenetic Dissection of Neural Circuits Underlying Emotional Valence and Motivated Behaviors. Brain Res. 1511, 73–92. doi:10.1016/j.brainres.2012.11.001
Nobili, L. (1828). Comparaison entre les deux galvanometres les plus sensibles, la grenouille et le moltiplicateur a deux aiguilles, suivie de quelques resultats noveaux. Ann. de Chim. de Physique 38, 225–245.
Nodera, H., and Kaji, R. (2006). Nerve Excitability Testing and its Clinical Application to Neuromuscular Diseases. Clin. Neurophysiol. 117 (9), 1902–1916. doi:10.1016/j.clinph.2006.01.018
Nodera, H., and Rutkove, S. B. (2012). Accommodation to Hyperpolarizing Currents: Differences between Motor and Sensory Nerves in Mice. Neurosci. Lett. 518 (2), 111–116. doi:10.1016/j.neulet.2012.04.065
Nylén, P., Hagman, M., and Johnson, A.-C. (1994). Function of the Auditory and Visual Systems, and of Peripheral Nerve, in Rats after Long-Term Combined Exposure to N-Hexane and Methylated Benzene Derivatives. I. Toluene. Pharmacol. Toxicol. 74 (2), 116–123. doi:10.1111/j.1600-0773.1994.tb01085.x
Obergrussberger, A., Bru ggemann, A., Goetze, T. A., Rapedius, M., Haarmann, C., Rinke, I., et al. (2016). Automated Patch Clamp Meets High-Throughput Screening: 384 Cells Recorded in Parallel on a Planar Patch Clamp Module. J. Lab. Automation 21 (6), 779–793. doi:10.1177/2211068215623209
Obergrussberger, A., Goetze, T. A., Brinkwirth, N., Becker, N., Friis, S., Rapedius, M., et al. (2018). An Update on the Advancing High-Throughput Screening Techniques for Patch Clamp-Based Ion Channel Screens: Implications for Drug Discovery. Expert Opin. Drug Discov. 13 (3), 269–277. doi:10.1080/17460441.2018.1428555
Organisation for Economic Co-Operation and Development (2016). Guidance Document for the Use of Adverse Outcome Pathways in Developing Integrated Approaches to Testing and Assessment (IATA), Series on Testing & Assessment. Paris, France: OECD Publishing.
Overton, E. (1902). Beiträge zur allgemeinen Muskel- und Nervenphysiologie. Pflüger, Arch. 92, 346–386. doi:10.1007/bf01659816
Panayiotopoulos, C. P., and Chroni, E. (1996). F-waves in Clinical Neurophysiology: a Review, Methodological Issues and Overall Value in Peripheral Neuropathies. Electroencephalography Clin. Neurophysiology/Electromyography Mot. Control. 101 (5), 365–374. doi:10.1016/0924-980x(96)95635-0
Peng, Y., Mittermaier, F. X., Planert, H., Schneider, U. C., Alle, H., and Geiger, J. R. P. (2019). High-throughput Microcircuit Analysis of Individual Human Brains through Next-Generation Multineuron Patch-Clamp. Elife 8, e48178. doi:10.7554/eLife.48178
Piccolino, M. (1998). Animal Electricity and the Birth of Electrophysiology: the Legacy of Luigi Galvani. Brain Res. Bull. 46 (5), 381–407. doi:10.1016/s0361-9230(98)00026-4
Polich, J. (2007). Updating P300: an Integrative Theory of P3a and P3b. Clin. Neurophysiol. 118 (10), 2128–2148. doi:10.1016/j.clinph.2007.04.019
Pollard, K. J., Bolon, B., and Moore, M. J. (2021). Comparative Analysis of Chemotherapy-Induced Peripheral Neuropathy in Bioengineered Sensory Nerve Tissue Distinguishes Mechanistic Differences in Early-Stage Vincristine-, Cisplatin-, and Paclitaxel-Induced Nerve Damage. Toxicol. Sci. 180 (1), 76–88. doi:10.1093/toxsci/kfaa186
Poon, E., Powers, B. E., McAlonan, R. M., Ferguson, D. C., and Schantz, S. L. (2011). Effects of Developmental Exposure to Polychlorinated Biphenyls And/or Polybrominated Diphenyl Ethers on Cochlear Function. Toxicol. Sci. 124 (1), 161–168. doi:10.1093/toxsci/kfr214
Racine, R. J., Gartner, J. G., and McIntyre Burnham, W. (1972). Epileptiform Activity and Neural Plasticity in Limbic Structures. Brain Res. 47 (1), 262–268. doi:10.1016/0006-8993(72)90268-5
Racine, R. J., Milgram, N. W., and Hafner, S. (1983). Long-term Potentiation Phenomena in the Rat Limbic Forebrain. Brain Res. 260 (2), 217–231. doi:10.1016/0006-8993(83)90676-5
Radnikow, G., Günter, R. H., Marx, M., and Feldmeyer, D. (2011). “Morpho-functional Mapping of Cortical Networks in Brain Slice Preparations Using Paired Electrophysiological Recordings,” in Neuronal Network Analysis. Neuromethods. Editors T. Fellin, and M. Halassa (New YorK, N.Y.: Humana Press), 405–431. 978-1-61779-632-6978-1-61779-633-3. doi:10.1007/7657_2011_14
Rebert, C. S. (1983). Multisensory Evoked Potentials in Experimental and Applied Neurotoxicology. Neurobehav Toxicol. Teratol 5 (6), 659–671.
Rebert, C. S., and Sorenson, S. S. (1983). Concentration-related Effects of Hexane on Evoked Responses from Brain and Peripheral Nerve of the Rat. Neurobehav Toxicol. Teratol 5 (1), 69–76.
Rebert, C. S., Pryor, G. T., and Frick, M. S. (1984). Effects of Vincristine, Maytansine, Andcis-Platinum on Behavioral and Electrophysiological Indices of Neurotoxicity in the Rat. J. Appl. Toxicol. 4 (6), 330–338. doi:10.1002/jat.2550040610
Renault, R., Sukenik, N., Descroix, S., Malaquin, L., Viovy, J.-L., Peyrin, J.-M., et al. (2015). Combining Microfluidics, Optogenetics and Calcium Imaging to Study Neuronal Communication In Vitro. PLoS One 10 (4), e0120680. doi:10.1371/journal.pone.0120680
Richmond, J. E., and Jorgensen, E. M. (1999). One GABA and Two Acetylcholine Receptors Function at the C. elegans Neuromuscular junction. Nat. Neurosci. 2 (9), 791–797. doi:10.1038/12160
Robinette, B. L., Harrill, J. A., Mundy, W. R., and Shafer, T. J. (2011). In Vitro assessment of Developmental Neurotoxicity: Use of Microelectrode Arrays to Measure Functional Changes in Neuronal Network Ontogeny. Front. Neuroeng 4, 1. doi:10.3389/fneng.2011.00001
Robinson, A. K., Venkatesh, P., Boring, M. J., Tarr, M. J., Grover, P., and Behrmann, M. (2017). Very High Density EEG Elucidates Spatiotemporal Aspects of Early Visual Processing. Sci. Rep. 7 (1), 1–11. doi:10.1038/s41598-017-16377-3
Ross, J. F. (1989). Applications of Electrophysiology in a Neurotoxicity Battery. Toxicol. Ind. Health 5 (2), 221–230. doi:10.1177/074823378900500207
Ross, J. F., and Lawhorn, G. T. (1990). ZPT-related Distal Axonopathy: Behavioral and Electrophysiologic Correlates in Rats. Neurotoxicology and Teratology 12 (2), 153–159. doi:10.1016/0892-0362(90)90128-y
Ruijten, M. W. M. M., Sallé, H. J. A., and Kingma, R. (1993). Comparison of Two Techniques to Measure the Motor Nerve Conduction Velocity Distribution. Electroencephalography Clin. Neurophysiology/Evoked Potentials Section 89 (6), 375–381. doi:10.1016/0168-5597(93)90110-b
Sack, A. T., and Linden, D. E. J. (2003). Combining Transcranial Magnetic Stimulation and Functional Imaging in Cognitive Brain Research: Possibilities and Limitations. Brain Res. Rev. 43 (1), 41–56. doi:10.1016/s0165-0173(03)00191-7
Sandström, J., Eggermann, E., Charvet, I., Roux, A., Toni, N., Greggio, C., et al. (2017). Development and Characterization of a Human Embryonic Stem Cell-Derived 3D Neural Tissue Model for Neurotoxicity Testing. Toxicol. Vitro 38, 124–135. doi:10.1016/j.tiv.2016.10.001
Sawai, S., Kanai, K., Nakata, M., Hiraga, A., Misawa, S., Isose, S., et al. (2008). Changes in Excitability Properties Associated with Axonal Regeneration in Human Neuropathy and Mouse Wallerian Degeneration. Clin. Neurophysiol. 119 (5), 1097–1105. doi:10.1016/j.clinph.2008.01.022
Sã¤nger, J., Bechtold, L., Schoofs, D., Blaszkewicz, M., and Wascher, E. (2014). The Influence of Acute Stress on Attention Mechanisms and its Electrophysiological Correlates. Front. Behav. Neurosci. 8, 353. doi:10.3389/fnbeh.2014.00353
Senzai, Y., Fernandez-Ruiz, A., and Buzsáki, G. (2019). Layer-specific Physiological Features and Interlaminar Interactions in the Primary Visual Cortex of the Mouse. Neuron 101 (3), 500–513. doi:10.1016/j.neuron.2018.12.009
Serra, J., Bostock, H., and Navarro, X. (2010). Microneurography in Rats: a Minimally Invasive Method to Record Single C-Fiber Action Potentials from Peripheral Nerves In Vivo. Neurosci. Lett. 470 (3), 168–174. doi:10.1016/j.neulet.2009.09.061
Shafer, T. J. (2019). Application of Microelectrode Array Approaches to Neurotoxicity Testing and Screening. Vitro Neuronal Networks 22, 275–297. doi:10.1007/978-3-030-11135-9_12
Shanes, A. M. (1949). Electrical Phenomena in Nerve. J. Gen. Physiol. 33 (1), 75–102. doi:10.1085/jgp.33.1.75
Sharma, A. D., McCoy, L., Jacobs, E., Willey, H., Behn, J. Q., Nguyen, H., et al. (2019). Engineering a 3D Functional Human Peripheral Nerve In Vitro Using the Nerve-On-A-Chip Platform. Sci. Rep. 9 (1), 1–12. doi:10.1038/s41598-019-45407-5
Shityakov, S., and Förster, C. Y. (2018). Computational Simulation and Modeling of the Blood-Brain Barrier Pathology. Histochem. Cel Biol 149 (5), 451–459. doi:10.1007/s00418-018-1665-x
Sills, R. C., Morgan, D. L., Herr, D. W., Little, P. B., George, N. M., Thai Vu Ton, T. V., et al. (2004). Contribution of Magnetic Resonance Microscopy in the 12-week Neurotoxicity Evaluation of Carbonyl Sulfide in Fischer 344 Rats. Toxicol. Pathol. 32 (5), 501–510. doi:10.1080/01926230490493918
Simão, D., Silva, M. M., Terrasso, A. P., Arez, F., Sousa, M. F. Q., Mehrjardi, N. Z., et al. (2018). Recapitulation of Human Neural Microenvironment Signatures in iPSC-Derived NPC 3D Differentiation. Stem Cel Rep. 11 (2), 552–564. doi:10.1016/j.stemcr.2018.06.020
Singh, A. K., Touhara, K., and Okamoto, M. (2019). Electrophysiological Correlates of Top-Down Attentional Modulation in Olfaction. Sci. Rep. 9 (1), 4953. doi:10.1038/s41598-019-41319-6
Singh, G., Avasthi, G., Khurana, D., Whig, J., and Mahajan, R. (1998). Neurophysiological Monitoring of Pharmacological Manipulation in Acute Organophosphate (OP) Poisoning. The Effects of Pralidoxime, Magnesium Sulphate and Pancuronium. Electroencephalography Clin. Neurophysiol. 107 (2), 140–148. doi:10.1016/s0013-4694(98)00053-4
Sirenko, O., Parham, F., Dea, S., Sodhi, N., Biesmans, S., Mora-Castilla, S., et al. (2019). Functional and Mechanistic Neurotoxicity Profiling Using Human iPSC-Derived Neural 3D Cultures. Toxicol. Sci. 167 (1), 58–76. doi:10.1093/toxsci/kfy218
Staicu, C. E., Jipa, F., Axente, E., Radu, M., Radu, B. M., and Sima, F. (2021). Lab-on-a-Chip Platforms as Tools for Drug Screening in Neuropathologies Associated with Blood-Brain Barrier Alterations. Biomolecules 11 (6), 916. doi:10.3390/biom11060916
Stålberg, E., and Sanders, D. B. (1981). “Electrophysiological Tests of Neuromuscular Transmission,” in Clinical Neurophysiology. Editors E. Stålberg, and R. Young (London: Butterworths), 88–116.
Stålberg, E., and Sonoo, M. (1994). Assessment of Variability in the Shape of the Motor Unit Action Potential, the "jiggle," at Consecutive Discharges. Muscle & Nerve 17, 1135–1144.
Steinmetz, N. A., Aydin, C., Lebedeva, A., Okun, M., Pachitariu, M., Bauza, M., et al. (2020). Neuropixels 2.0: A Miniaturized High-Density Probe for Stable, Long-Term Brain Recordings. bioRxiv 1027, 358291.
Steinmetz, N. A., Zatka-Haas, P., Carandini, M., and Harris, K. D. (2019). Distributed Coding of Choice, Action and Engagement across the Mouse Brain. Nature 576 (7786), 266–273. doi:10.1038/s41586-019-1787-x
Stolzberg, D., Chrostowski, M., Salvi, R. J., and Allman, B. L. (2012). Intracortical Circuits Amplify Sound-Evoked Activity in Primary Auditory Cortex Following Systemic Injection of Salicylate in the Rat. J. Neurophysiol. 108 (1), 200–214. doi:10.1152/jn.00946.2011
Stone, N. L., England, T. J., and O’Sullivan, S. E. (2019). A Novel Transwell Blood Brain Barrier Model Using Primary Human Cells. Front. Cel. Neurosci. 13, 230. doi:10.3389/fncel.2019.00230
Sung, J.-Y., Tani, J., Park, S. B., Kiernan, M. C., and Lin, C. S.-Y. (2014). Early Identification of 'acute-Onset' Chronic Inflammatory Demyelinating Polyneuropathy. Brain 137 (8), 2155–2163. doi:10.1093/brain/awu158
Sutton, S., Braren, M., Zubin, J., and John, E. R. (1965). Evoked-potential Correlates of Stimulus Uncertainty. Science 150 (3700), 1187–1188. doi:10.1126/science.150.3700.1187
Szymanski, F. D., Garcia-Lazaro, J. A., and Schnupp, J. W. H. (2009). Current Source Density Profiles of Stimulus-specific Adaptation in Rat Auditory Cortex. J. Neurophysiol. 102 (3), 1483–1490. doi:10.1152/jn.00240.2009
Thompson, S. W., Davis, L. E., Kornfeld, M., Hilgers, R. D., and Standefer, J. C. (1984). Cisplatin Neuropathy. Clinical, Electrophysiologic, Morphologic, and Toxicologic Studies. Cancer 54 (7), 1269–1275. doi:10.1002/1097-0142(19841001)54:7<1269:aid-cncr2820540707>3.0.co;2-9
Timme, N., Ma, B., Linsenbardt, D. N., Cornwell, E., Galbari, T., and Lapish, C., 2021. Compulsive Drinking Is Associated with Neural Activity Patterns Reflecting Diminished Behavioral Control and Enhanced Seeking Representations in Dorsal Medial Prefrontal Cortex. bioRxiv.
Trachtenberg, J. T., Chen, B. E., Knott, G. W., Feng, G., Sanes, J. R., Welker, E., et al. (2002). Long-term In Vivo Imaging of Experience-dependent Synaptic Plasticity in Adult Cortex. Nature 420 (6917), 788–794. doi:10.1038/nature01273
Tucker, K. J., Tuncer, M., and Türker, K. S. (2005). A Review of the H-Reflex and M-Wave in the Human Triceps Surae. Hum. Movement Sci. 24 (5-6), 667–688. doi:10.1016/j.humov.2005.09.010
United States Environmental Protection Agency, (1998a). “Health Effects Test Guidelines. OPPTS 870.6850. Peripheral Nerve Function,” in Office of Prevention. Editor P. A. T.S (Washington, D.C., 1–9.
United States Environmental Protection Agency, (1998b). “Health Effects Test Guidelines. OPPTS 870.6855. Neurophysiology: Sensory Evoked Potentials,” in Office of Prevention. Editor P. A. T.S (Washington, D.C., 1–14.
Urai, A. E., Doiron, B., Leifer, A. M., and Churchland, A. K., 2021. Large-scale Neural Recordings Call for New Insights to Link Brain and Behavior. arXiv: 2103.14662.
van Der Helm, M. W., Van Der Meer, A. D., Eijkel, J. C. T., van den Berg, A., and Segerink, L. I. (2016). Microfluidic Organ-On-Chip Technology for Blood-Brain Barrier Research. Tissue Barriers 4 (1), e1142493. doi:10.1080/21688370.2016.1142493
Verkhratsky, A., Krishtal, O. A., and Petersen, O. H. (2006). From Galvani to Patch Clamp: the Development of Electrophysiology. Pflugers Arch. - Eur. J. Physiol. 453 (3), 233–247. doi:10.1007/s00424-006-0169-z
Verkhratsky, A., and Parpura, V. (2014). History of Electrophysiology and the Patch Clamp, Patch-Clamp Methods and Protocols. Springer, 1–19. doi:10.1007/978-1-4939-1096-0_1
Verleger, R. (1988). Event-related Potentials and Cognition: A Critique of the Context Updating Hypothesis and an Alternative Interpretation of P3. Behav. Brain Sci. 11 (3), 343–356. doi:10.1017/s0140525x00058015
Victor Nadler, J., and Cuthbertson, G. J. (1980). Kainic Acid Neurotoxicity toward Hippocampal Formation: Dependence on Specific Excitatory Pathways. Brain Res. 195 (1), 47–56. doi:10.1016/0006-8993(80)90865-3
Vogel, E. K., and Luck, S. J. (2000). The Visual N1 Component as an index of a Discrimination Process. Psychophysiology 37 (2), 190–203. doi:10.1111/1469-8986.3720190
Ward, N. L., and Lamanna, J. C. (2004). The Neurovascular Unit and its Growth Factors: Coordinated Response in the Vascular and Nervous Systems. Neurol. Res. 26 (8), 870–883. doi:10.1179/016164104x3798
Wijers, A. A., Otten, L. J., Feenstra, S., Mulder, G., and Mulder, L. J. M. (1989). Brain Potentials during Selective Attention, Memory Search, and Mental Rotation. Psychophysiology 26 (4), 452–467. doi:10.1111/j.1469-8986.1989.tb01951.x
Wood, C. C. (1982). Application of Dipole Localization Methods to Source Identification of Human Evoked Potentials. Ann. NY Acad. Sci. 388 (1), 139–155. doi:10.1111/j.1749-6632.1982.tb50789.x
Xie, C., Liu, J., Fu, T.-M., Dai, X., Zhou, W., and Lieber, C. M. (2015). Three-dimensional Macroporous Nanoelectronic Networks as Minimally Invasive Brain Probes. Nat. Mater 14 (12), 1286–1292. doi:10.1038/Nmat4427
Yang, D., He, F., and Li, T. (2001). Repetitive Nerve Stimulation and Stimulation Single Fiber Electromyography Studies in Rats Intoxicated with Single or Mixed Insecticides. Toxicology 161 (1-2), 111–116. doi:10.1016/s0300-483x(01)00339-0
Ylä-Outinen, L., Heikkilä, J., Skottman, H., Suuronen, R., Äänismaa, R., and Narkilahti, S. (2010). Human Cell-Based Micro Electrode Array Platform for Studying Neurotoxicity. Front. Neuroengineering 3, 111.
Zhuang, P., Sun, A. X., An, J., Chua, C. K., and Chew, S. Y. (2018). 3D Neural Tissue Models: From Spheroids to Bioprinting. Biomaterials 154, 113–133. doi:10.1016/j.biomaterials.2017.10.002
Zotova, E. G., and Arezzo, J. C. (2013). Non-Invasive Evaluation of Nerve Conduction in Small Diameter Fibers in the Rat. Physiol. J. 2013, 254789. doi:10.1155/2013/254789
Zotova, E. G., Schaumburg, H. H., Raine, C. S., Cannella, B., Tar, M., Melman, A., et al. (2008). Effects of Hyperglycemia on Rat Cavernous Nerve Axons: a Functional and Ultrastructural Study. Exp. Neurol. 213 (2), 439–447. doi:10.1016/j.expneurol.2008.07.009
Keywords: neurotoxicololgy, neurophysiology, adverse outcome pathway, neural networks, mechanistic
Citation: Herr DW (2021) The Future of Neurotoxicology: A Neuroelectrophysiological Viewpoint. Front. Toxicology 3:729788. doi: 10.3389/ftox.2021.729788
Received: 23 June 2021; Accepted: 29 November 2021;
Published: 14 December 2021.
Edited by:
Deborah A. Cory-Slechta, University of Rochester, United StatesReviewed by:
Marcia Ratner, Boston University, United StatesGemma Calamandrei, National Institute of Health (ISS), Italy
Fabien Gosselet, Université d'Artois, France
Copyright © 2021 Herr. This is an open-access article distributed under the terms of the Creative Commons Attribution License (CC BY). The use, distribution or reproduction in other forums is permitted, provided the original author(s) and the copyright owner(s) are credited and that the original publication in this journal is cited, in accordance with accepted academic practice. No use, distribution or reproduction is permitted which does not comply with these terms.
*Correspondence: David W. Herr, SGVyci5kYXZpZEBlcGEuZ292