- 1US Environmental Protection Agency, Public Health Integrated Toxicology Division, Center for Public Health and Environmental Assessment, Research Triangle Park, NC, United States
- 2Oak Ridge Institute for Science Education, Oak Ridge, TN, United States
It is well known that the adult brain is protected from some infections and toxic molecules by the blood-brain and the blood-cerebrospinal fluid barriers. Contrary to the immense data collected in other fields, it is deeply entrenched in environmental toxicology that xenobiotics easily permeate the developing brain because these barriers are either absent or non-functional in the fetus and newborn. Here we review the cellular and physiological makeup of the brain barrier systems in multiple species, and discuss decades of experiments that show they possess functionality during embryogenesis. We next present case studies of two chemical classes, perfluoroalkyl substances (PFAS) and bisphenols, and discuss their potential to bypass the brain barriers. While there is evidence to suggest these pollutants may enter the developing and/or adult brain parenchyma, many studies suffer from confounding technical variables which complicates data interpretation. In the future, a more formal consideration of brain barrier biology could not only improve understanding of chemical toxicokinetics but could assist in prioritizing environmental xenobiotics for their neurotoxicity risk.
Introduction
Exposure to environmental contaminants during development may have persistent negative impacts on human health across the lifespan (Grandjean and Landrigan, 2006; Landrigan and Goldman, 2011; Grandjean and Landrigan, 2014). Recently, the link between early life exposures to pollutants and neurodevelopmental disorders (NDDs) has received more public attention (Diamanti-Kandarakis et al., 2009; Gore et al., 2014). The National Health Interview Survey, a survey of American civilian noninstitutionalized households, found approximately one in six children aged 3–17 were diagnosed with one of ten specified developmental disabilities (e.g., autism, attention-deficit/hyperactivity disorder, and learning disabilities) between 2009 and 2017 (Zablotsky et al., 2019). Between 2009–2011 and 2015–2017, there were also significant increases in the prevalence of all three NDDs in American children (Zablotsky et al., 2019). While these observed increases could be attributed to increased awareness in health care providers and parents, growing evidence suggests that exposure to environmental contaminants, in addition to genetic and psychosocial factors, may play an overlooked role in altering brain development (Grandjean and Landrigan, 2006; Axelrad et al., 2013; Grandjean and Landrigan, 2014; Landrigan et al., 2019).
Human neurodevelopment is an intricately choreographed process that begins 3 weeks post-conception and concludes in early adulthood (Stiles and Jernigan, 2010; Budday et al., 2015). This protracted maturation period poses unique challenges when attempting to understand NDDs, as there are complex and time-dependent sequences of chemical messages required for normal development (Diamanti-Kandarakis et al., 2009). For example, a NDD may be diagnosed long after perturbation of the causative developmental pathway (Diamanti-Kandarakis et al., 2009). Understanding these windows of susceptibility is especially challenging when trying to understand how environmental contaminants may influence the developing brain. Beginning as early as conception, a person could be exposed to a multitude of industrial chemicals that are used in products like children’s toys, food preparation and packaging, personal care products, and household agents (O'Shaughnessy et al., 2021). While having economic and consumer benefit, these chemicals are used ubiquitously in communities and associated with air pollution, electronic waste, flame retardants, plastics, and pesticides (Diamanti-Kandarakis et al., 2009; Gore et al., 2014; O'Shaughnessy et al., 2021). Although there are at least tens of thousands of manufactured compounds currently in the chemical universe, very few have undergone toxicological evaluation (Judson et al., 2009; Rayasam et al., 2022) and even fewer have undergone developmental neurotoxicity testing. Grandjean and Landrigan (2006) published a systematic review identifying over 100 industrial chemicals known to be neurotoxic to humans, five of which could be classified as developmental neurotoxicants (arsenic, lead, methylmercury, polychlorinated biphenyls, and toluene). Their 2014 report found six more developmental neurotoxicants (chlorpyrifos, dichlorodiphenyltrichloroethane, fluoride, manganese, polybrominated diphenyl ethers, and tetrachloroethylene) (Grandjean and Landrigan, 2014). Several other reviews and meta-analyses have found associations between industrialized chemicals and developmental neurotoxicity (Heyer and Meredith, 2017; Landrigan et al., 2019; Iqubal et al., 2020) both in population-based studies and animal models.
Within the field of developmental neurotoxicology, a feature of the central nervous system that is often overlooked is the development and function of the brain barriers. These interfaces separate the brain from other bodily fluids (e.g., blood, cerebrospinal fluid), maintain homeostasis, and protect against toxic molecules and infection. However, there is a large data gap in determining whether/when industrial chemicals can cross into the brain, due in part to a common misconception that the developing brain has an immature and therefore “leaky” (i.e., permeable) barrier system. Many recent reports mention that neurotoxicants are easily transported into the young brain with little, if any, discussion as to what processes purportedly take place (see Ek et al., 2012 for review). Surprisingly, the mechanism of toxicity for even the best studied neurotoxicants is still not clear (Ek et al., 2012), which ultimately begets the question: how are environmental contaminants entering the young brain, if at all? Moreover, could exposure to some environmental contaminants alter brain barrier function? Alterations in blood- and cerebrospinal fluid-brain barrier integrity is now recognized as an important component of epilepsy (Baruah et al., 2019), and its dysfunction could contribute to disorders like autism (Kealy et al., 2020), attention-deficit/hyperactivity disorder (Medin et al., 2019), and schizophrenia (Najjar et al., 2017; Greene et al., 2018; Pollak et al., 2018; Kealy et al., 2020). Progress in brain barrier research has also shown that the adult barrier system is a target of environmental toxicants (Zheng et al., 2003; Zheng and Ghersi-Egea, 2020). Thus, it is possible that early-life exposure to some chemicals could lead to pathophysiological changes in brain barrier function, which could result in NDDs or other brain disorders. However, there is little work that investigates this hypothesis.
In this review, we briefly outline the rich, 200-year history of brain barrier biology. We next discuss the cellular and physiological properties that underlie the function of the brain barrier systems, and present evidence to show that the developing brain possesses functional blood and cerebrospinal fluid barriers. Finally, we critically review the neurotoxicological literature that may provide evidence of environmental contaminants crossing into brain tissue. While it is widely believed in environmental toxicology that the developing brain is more susceptible to neurotoxic insults than the adult due in part to inadequate brain barriers, there is a lack of evidence to support this assumption. Alternatively, there are convincing data that the barriers form and possess functionality during embryogenesis in both humans and experimental models. We challenge readers in the field to reevaluate their understanding of these fluid interfaces, as in many contexts the brain barriers will determine the neurotoxic potential of a chemical exposure.
History of the brain barriers
The physiological compartmentalization of the brain and spinal cord has been described for centuries and is widely accepted across scientific disciplines. Dating back to the late 1600s, the London physician Humphrey Ridley is likely one of the first to recognize and describe the brain’s ability to obstruct certain compounds from crossing in the adult brain (Ridley, 1695). Almost 200 years later, the brain’s ability to selectively filter substances was more formally established during an attempt to cure African sleeping sickness caused by the protozoa, trypanosomes (Bentivoglio and Kristensson, 2014). Trypanosomes readily cross into the central nervous system (Myburgh et al., 2013), eliciting agonizing neurological symptoms if left untreated. In the late 1800s, the medical researcher Paul Ehrlich attempted to kill the parasite with dyed antiprotozoal agents (trypanocides) to treat infection (Bentivoglio and Kristensson, 2014). Instead, he accidentally discovered that the trypanocides could not traverse into the brain tissue. Through subsequent studies in which both Ehrlich and his student Edwin E. Goldman injected dyes in rodents, it was revealed that the whole body was stained except for the brain and spinal cord (Ehrlich, 1885; Goldmann, 1909). Ehrlich initially believed this was due to the brain tissue lacking affinity for the dyes as opposed to a formal barrier, but this work nonetheless marked a seminal moment in neuroscience. To further their findings, Goldman conducted an additional study in which he injected trypan blue, another dye, directly into the brain and found that it was maintained within the neural tissue, suggesting that some type of boundary existed between the blood and brain (Goldmann, 1913). However, “barrier” was not used to describe the brain’s selectivity phenomenon until 1918 by Stern and Gautier (Stern and Gautier, 1918), although many publications wrongfully ascribe the term to Lewandowsky’s paper where he recounted the relative impermeability of the brain’s vasculature (Lewandowsky, 1900). These initial studies were pivotal in the recognition of the blood-brain barrier in the adult, and the traditional method of injecting dyes either intravenously or intraperitoneally in animal models to determine the functionality of the brain barriers is still in practice today. It has since been established that the adult brain is well-protected by several brain barriers through elegant ultrastructural, immunohistochemical, and functional studies.
Unlike the adult, the notion of fully functioning barrier system in the fetus, newborn, and child continues to be highly disputed amongst physicians, researchers, and governing agencies despite the mounting evidence collected over the last 100 years (Neuwelt et al., 2011; Ek et al., 2012; Saunders et al., 2018, 2019). Weed (1917) conducted one of the first developmental studies and demonstrated that the central nervous system is a closed compartment as early as gestational day nine in pig embryos (full term ∼115 days), as evidenced by restriction of his injected tracer (sodium ferrocyanide) to the cerebrospinal fluid (CSF). Wislocki (1920) injected trypan blue into the amniotic sacks of guinea pig embryos from mid-gestation to full term and noted that the dye stained tissues throughout the body except for the brain. Many dye studies repeatedly illustrated that dyes do not enter the developmentally immature brain as long as an appropriate amount of dye was used (e.g., Stern and Rapoport, 1928; Cohen and Davies, 1938; Gröntoft, 1954; Grazer and Clemente, 1957; Millen and Hess, 1958; Moos and Møllgård, 1993). Interestingly, one study was conducted in human fetuses as young as 10 weeks post conception (∼5 cm in length), and again the whole brain was not stained unless a state of hypoxia had occurred prior to collection (Gröntoft, 1954). The first papers to ostensibly provide support for a developmentally dysfunctional blood-brain barrier were conducted by Gerhard Behnsen, in which he injected excessive amounts of dye in mice (Behnsen, 1926, 1927). Penta (1932) also used large volumes of injected dye in newborn rabbits. Although the dye stained brain tissue which suggested the brain barriers were not functioning, most of the animals died from toxic effects of the dye. In response to Behnsen and others, Stern et al. (1929) underscored the concern of injecting too much dye in young animals, as superseding a dye’s binding capacity results in physiological instability, overt neurotoxicity, and eventual entry in the brain (see Saunders et al., 2015 for further review). So, although some studies report the functional immaturity of the brain barriers, these observations were later attributed to experimental artifact. Once technical parameters were adjusted, functionality could again be demonstrated during development across multiple species.
Despite these accepted biological principles, toxicology publications within the last 10 years continue to imply that the barrier systems during development are permeable due to their immaturity (see Grandjean and Landrigan 2014; Heyer and Meredith 2017; Rock and Patisaul 2018; Iqubal et al., 2020), and thus xenobiotics are assumed to enter the brain tissue without any, or potentially mis-cited, supporting evidence (see Ek et al., 2012 for review).
What constitutes the brain barriers?
The brain is the most complex organ in the body that regulates all physiological processes at some level. As such, it is ensconced by several protective barriers. While there are at least six barriers (Saunders et al., 2018), the focus of this review will be on the two barriers of most medical importance: the blood-brain barrier (BBB) and the blood-cerebrospinal fluid barrier (BCSFB). We will also be briefly discussing the transient fetal cerebrospinal fluid-brain barrier, which may have an important role during development.
The blood-brain barrier
Once absorbed in the bloodstream, a substance (e.g., xenobiotics, gases, nutrients) circulates through the vascular system. The vascular system is incredibly heterogenous in structure and function around the body and within organs (Aird, 2007a; b), with the capillaries and postcapillary venules of the central nervous system (CNS) being particularly unique (Daneman and Prat, 2015). The specialized blood vessels of the CNS are composed of tightly packed endothelial cells and, unlike most other vessels around the body, form a continuous non-fenestrated boundary. The lack of capillary pores prohibits diffusion of most substances. In turn, this isolates the circulating blood from the CNS, including the brain. Mammalian brains are highly vascularized and contain over 100 million vessel segments (Kirst et al., 2020); however, it is the cerebral capillaries (<10 μm diameter) (Tong et al., 2020) that constitute the BBB and corresponds to a surface area of 15–25 m2 in adult humans (Lauwers et al., 2008; Wong et al., 2013). The BBB is highly involved in the transfer of nutrients and drugs to the brain (Abbott et al., 2008; Wong et al., 2013) and acts as both a physical and metabolic barrier (Abbott, 2005; McCaffrey and Davis, 2012). Its series of cellular properties (discussed below) allow it to regulate brain homeostasis and serve as a gatekeeper between the circulating blood, brain interstitium, and parenchyma, by tightly regulating the exchange of blood constituents such as ions, glucose, hormones, and neurotransmitters (Stonestreet et al., 2006; Zlokovic, 2008; Daneman and Prat, 2015).
Cellular properties
The BBB is one of the biggest challenges in the pharmaceutical industry for its ability to prohibit 98% of drugs from crossing into the brain tissue (Pardridge, 2007), due in part to its cellular properties (Abbott et al., 2006; Abbott et al., 2010). The brain’s capillaries are lined with endothelial cells arranged as a modified simple squamous epithelium and are connected by three cell-cell junctions: tight junctions (i.e., occluding junctions), adherens junctions (i.e., zonula adherens), and gap junctions (i.e., septate junctions). Despite having three different cell junction types, the intercellular tight junctions form the seal between endothelial cells. These seals are created at the apical side (closest to blood) where tight junction transmembrane proteins laterally “stitch” two adjacent cells. This closes the intercellular space, averting paracellular diffusion of molecules from the blood into the brain parenchyma (see Tsukita et al., 2001 for review). The cellular barrier is one of the first lines of defense and manually blocks large molecules from entering the tissue (Figure 1).
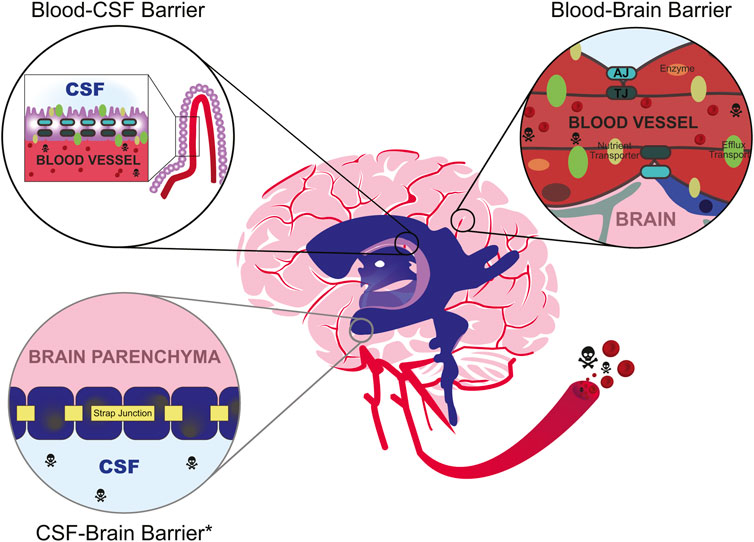
FIGURE 1. Environmental toxicants (skull and crossbones) commonly enter the bloodstream after inhalation, ingestion, or dermal exposure. While in circulation, they may eventually enter the cerebrovascular system, which is partly composed of the brain barrier systems such as the blood-cerebrospinal fluid (CSF) barrier (top left) and the blood-brain barrier (right). The anatomical location of the blood-CSF barrier occurs within the ventricles (purple) at the choroid plexus (lilac), while the blood-brain barrier occurs at the cerebral capillaries (red). The CSF-brain barrier occurs at the level of the cells lining the brain’s ventricles. Depending on the developmental stage, these cells may be progenitors of the ventricular epithelium (developmental) or primarily ependymal cells (mature). All depicted barriers mechanically obstruct the passage of compounds through either tight junctions (“TJ”, dark green hashes) at the choroid plexus epithelial cells and within endothelial cells, or strap junctions (yellow) at the ventricular epithelial cells. Strap junctions are functionally similar to tight junctions, but they are only present during development (denoted by light gray circle, asterisk, *). As the animal ages, they are replaced by adherens junctions (“AJ”, teal). At the blood barriers, other adhesion molecules (e.g., JAM-A/B/C) and cell junction types (e.g., adherens, teal; gap, not pictured) also help maintain attachment between cells. Select compounds may enter the brain through specialized receptor-mediated transporters (e.g., GLUT1, LAT1; olive ovals). Otherwise, unwanted substrates may be metabolized through metabolic enzymes (orange ovals) and/or discharged back into the bloodstream through efflux transporter proteins (e.g., p-glycoprotein, Pgp; multidrug resistance proteins, MRP; green ovals). The blood-brain barrier is also known to dynamically operate as a system called the neurovascular unit, which includes the coordinated function of additional cellular components like pericytes (blue) and astrocytes (gray). It is important to note that the specific composition of the cell structures varies between the blood-CSF barrier, the blood-brain barrier, and the CSF-brain barrier.
Tight junction proteins include claudins, occludin, zonula occludens, and junctional adhesion molecules. The claudin family of proteins is recognized as the essential component of paracellular barriers due to experimentation with knockout mice (Saitou et al., 2000) and physiological studies (Furuse et al., 1998) (see Tsukita et al., 2019 for review). The claudin family has 27 identified members (Gunzel and Yu, 2013), 17 of which are present in the human BBB (Berndt et al., 2019). Claudin-5 is the best-studied and appears to be the only tight junction protein whose importance is agreed upon unanimously (Morita et al., 1999; Ohtsuki et al., 2007; Greene et al., 2018). Experimentation with claudin-5 knockout mice (Cld5−/−) revealed that homozygous null mutants had morphologically intact tight junctions yet died within hours of being born. The BBB of the affected mice lost its ability to obstruct small molecules from passing into the tissue, as demonstrated by primary amine-reactive biotinylated reagent (443 Da) crossing into the brain parenchyma (Figures 2A–Bʹʹ) (Nitta et al., 2003). In addition, a viral knockdown mouse model against claudin-5 demonstrated that a reduction in claudin-5 induced BBB disruption as characterized by increased amounts of biotin (600 Da) and fibrinogen (340 kDa) in brain tissue; these mice also experienced seizures and behavioral changes (Greene et al., 2018). Other important proteins help constitute the BBB including connexins and endothelial immunoglobulin-like cell adhesion molecules (Zhao et al., 2018). Crosstalk between these aforementioned molecules, amongst others, helps maintain the integrity of the BBB.
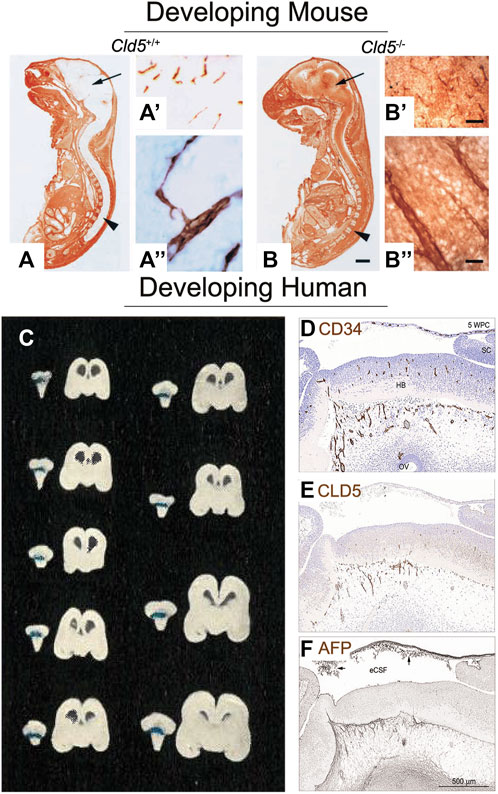
FIGURE 2. Tight junctions of the blood-brain barrier are present and functional as soon as the brain is vascularized. (A) Sagittal frozen section of a gestational day (GD) 18.5 wildtype mouse fetus (Cld5+/+) demonstrate functionality of the blood-brain barrier. Primary amine-reactive biotinylated reagent (443 Da, visualized by brown staining) was excluded from the brain parenchyma (arrow) and the spinal cord (arrowhead). (A′) Biotinylated reagent (brown) is only visible within the brain’s blood vessels, as visualized at low and (A′′) high magnification. This demonstrates that this 443 Da molecule does not cross the BBB, and hence shows its activity (B) However, in the Cld5 knockout mouse (Cld5−/−), tight junctions are impaired and biotinylated reagent is visible as brown staining throughout the brain and spinal cord. Extraversion of biotinylated reagent into the brain parenchyma is detectable at (B′) low and (B′′) high magnification. This shows that even during fetal life, tight junctions are present in the brain and actively occlude substances. Scale bars: (A,B) 2 mm; (A′) and (B′) 40 μm; (A′′) and (B′′) 10 μm. Reproduced from Nitta et al., 2003, which was originally published in Journal of Cell Biology under a Creative Commons Attribution 4.0 International License (CC-BY) (Nitta et al., 2003). (C) Coronal sections of nine human fetuses aged approximately 16 weeks post conception (WPC; 14 cm) to 29 WPC (30 cm) show that trypan blue dye does not permeate the brain tissue after cardiac perfusion, demonstrating an active BBB. Note that blue staining is visible at the choroid plexus of the hind brain, but not in the brain parenchyma. Reproduced from Gröntoft, 1954, which was originally published in Acta pathologica et microbiologica Scandinavica. Permissions were obtained from Wiley (Gröntoft, 1954). (D) At 5 WPC, consecutive sagittal sections of the human embryonic brain show overlapping staining for blood vessels (CD34; brown staining) and (E) tight junctions (CLD5, brown staining). This shows that tight junctions are likely colocalizing with the brain’s vasculature to form the BBB. (F) Functionality of the barrier is observed by the lack of endogenous alpha fetoprotein (AFP, 70 kDa) in the forebrain tissue (brown staining). Abbreviations: FB, forebrain; HB, hindbrain; SC, spinal cord, Scale bar, 100 μm. Reproduced from © Møllgård et al., 2017, which was originally published in Scientific Reports under a Creative Commons Attribution 4.0 International License (CC-BY) (Møllgård et al., 2017).
The gatekeeping properties of the BBB extend beyond its cell junctions. The BBB dynamically operates as a system: endothelial cells are surrounded by a basement membrane, pericytes, smooth muscle cells, and astrocyte end-feet, forming what is recognized as the neurovascular unit (NVU) (Neuwelt et al., 2011) (Figure 1, also see Saili et al., 2017). The basement membrane is a 20–200 nm (Timpl, 1989) non-cellular matrix between the endothelial cells and astrocytic endfeet, and provides stability to the BBB and blocks blood constituents from traversing (Thomsen et al., 2017). Embedded within the basement membrane are pericytes. Pericytes are required for BBB formation in the fetus (Daneman et al., 2010) and are thought to regulate astrocytes, the basement membrane, blood vessel stability, and blood flow (Armulik et al., 2010; Winkler et al., 2011). Astrocytes are also thought to significantly contribute to, but not induce the formation of the NVU (Abbott et al., 2006; Saunders et al., 2014). In the adult brain, astrocytic endfeet encircle cerebral capillaries, providing an additional barrier for xenobiotics. The astrocytic end-foot is approximately 20 nm away from the capillaries (Paulson and Newman, 1987) and invested in more than 98% of their surfaces (Caley and Maxwell, 1970; Johanson, 1980). Additionally, circulating blood cells such as polymorphonuclear cells, lymphocytes, and monocytes along the vascular lumen are considered components of the NVU (Neuwelt et al., 2011). In all, the interrelationships between the components of the NVU are incredibly intimate, and the regulation of the molecular and cellular events is complex and tightly regulated.
Flux-flow dynamics
Blood constituents were previously thought to pass through cerebrospinal fluid first before entering the brain (Pardridge, 2016), but it is now understood that compounds can flow directly from blood vessels into the brain parenchyma. To transfer from blood to cerebral tissue, compounds need to pass through both the luminal (facing blood) and the abluminal (facing brain interstitial fluid) membranes of endothelial cells. Tight junctions deter paracellular movement (see “Cellular properties”), thus intracellular systems along the barrier are important drivers of compound disposition in the CNS. Transporters embedded within endothelial cells control the influx of nutrients and the efflux of wastes, toxicants, and drugs. It is estimated that 10–15% of all proteins in the NVU are transporters (Enerson and Drewes, 2006), and the list of identified transporters at the BBB continues to grow (Tachikawa et al., 2014). Two main categories of transporters have been established: efflux transporters (i.e., ATP-driven membrane transporters) and influx transporters (i.e., receptor-mediated transporters). Efflux transporters from the ATP-binding cassette (ABC) superfamily, including P-glycoprotein (Pgp; ABCB1, mdr1), multidrug resistance proteins (MRP; ABCC family) and breast cancer resistance protein (BCRP; ABCG2), are generally localized to the luminal surface (International Transporter et al., 2010; Morris et al., 2017) and mediate nonspecific transport of hydrophobic compounds. Pgp, MRP1, and BCRP have been the best studied ABC-transporters as they preclude pharmaceutical drug access to brain tissue. Alterations in expression of efflux transporters appear clinically important as diseases associated with inflammation and oxidative stress (e.g., bacterial meningoencephalitis, Parkinson’s, Alzheimer’s, etc.) interfere with these transporters (see Roberts and Goralski 2008 for review) (Loscher and Potschka, 2005; Neuwelt et al., 2011). Mechanisms of efflux transport is a highly active area of research to aid in the development of CNS therapeutics. Receptor-mediated transporters also facilitate the transport of specific nutrients across the BBB and have been another target for new drug therapeutics (Trojan horse paradigm) (Pardridge, 2006). Unlike efflux pumps, receptor-mediated transporters are highly specific. Many belong to the solute carrier (SLC) superfamily which is composed of ion channels, exchangers, and passive transporter families.
The speed of blood flow within the blood vessels is also critical for transport. Cerebral blood flow, in concert with the permeability of the compound, directly relate to the rate of CNS penetration (Reichel, 2015). For example, gases such as carbon dioxide, oxygen, and volatile anesthetics diffuse rapidly into the brain and are limited primarily by the cerebral blood flow rate. Cerebral blood flow can be altered through vasodilation and vasoconstriction (Takano et al., 2006; Iadecola and Nedergaard, 2007) and varies in a spatiotemporal and psychosomatic manner around brain (Fenstermacher et al., 1991). However, cerebral blood flow can be largely variable, illustrating the difficulty to define precise reference values. Irrespective of the documented variabilities, blood flow is required to necessitate the transport of substrates to and from the brain.
The blood-cerebrospinal fluid barrier
With a surface area of 0.02 m2 in humans (Dohrmann and Bucy, 1970), the blood-cerebrospinal fluid barrier (BCSFB) is the next largest brain gatekeeper and also plays a role in xenobiotic transport (Lin, 2008; Strazielle and Ghersi-Egea, 2016). Like the BBB, the BCSFB exhibits low paracellular permeability and expresses multiple transporters that aid in the flux-flow dynamics. It is anatomically located around the brain’s cavities at the epithelial layer of the choroid plexus. The choroid plexus is within each of the brain ventricles: the lateral, third, and fourth ventricles. The choroid plexus epithelial cells are responsible for producing most of the cerebrospinal fluid (CSF), the colorless body fluid covering the brain and spinal cord. Adult human CSF volume is estimated to be approximately 150 ml, with choroid plexus epithelial cells secreting around 240–450 ml per day (Sakka et al., 2011). This allows the CSF to turn over three to four times daily, which has important physiological implications such as acting as a waste removal system and a convoy for a variety of compounds to the brain tissue.
Cellular properties
The BCSFB is composed of a single layer of specialized cuboidal epithelial cells nested between blood vessels and the CSF, separating the dense network of vasculature from the ventricular system. These epithelial cells contain cilia and microvilli, which force the CSF to circulate from the brain ventricles to the brain stem. Beneath the layer of choroid plexus epithelial cells is an inner core of stromal cells surrounding relatively large capillary blood vessels. Like the BBB, the morphological basis of the BCSFB is at the level of tight junctions. However, the BCSFB tight junctions bind epithelial cells rather than the blood vessel endothelial cells (Figure 1). Unlike the endothelial cells of the BBB, the endothelium within the BCSFB is relatively thinner and fenestrated, allowing for regulated transfer of materials from blood to the interstitial fluid (see “Flux-flow dynamics”). Tight junction proteins in the BCSFB also include the claudins and occludin like the BBB, but the composition is distinct. The role of each tight junction protein has yet to be appropriately defined, but it is believed that claudin-1 and claudin-3 are the prominent transmembrane proteins (Wolburg et al., 2001; Wolburg et al., 2009), and other members of the claudin family are differentially expressed compared to BBB vasculature (Redzic, 2011). Claudin-2 appears specific to the BCSFB and aids in paracellular water movement, and thus is critical to secretion of the CSF (see Bauer et al., 2014 for further review).
Flux-flow dynamics
The choroid plexus is also highly vascularized. However, the relatively relaxed cellular properties of its endothelial cells transform the flux-flow dynamics of the choroid plexus from rigid to “leaky” as compared to the BBB (Solár et al., 2020). The composition of the CSF is nonetheless controlled, but the decreased resistance across this cellular barrier allows for plasma solutes to traverse more easily (Redzic, 2011). The choroid plexus produces CSF at a rate of ∼0.29 μl/min/g in human adults (about 1.03–3.00 μl/min/g brain in rodent) (Oshio et al., 2005; de Lange, 2013). This high production rate results in a fast turnover half-life (40–100 min in rat versus 170 min in humans) (Friden et al., 2009), which allows the brain to quickly clear and deliver compounds to brain regions that are in contact with the CSF (e.g., ventricular epithelium) (Johanson et al., 2008). CSF turnover is highly important in the pharmaceutical industry as it affects the drug concentration in the CSF, as well as drug diffusion across various brain compartments (de Lange, 2013). For example, slower flowing CSF results in reduced clearance of substances from the brain and can result in accumulation of potentially toxic molecules; this phenomenon is known as the “sink effect” (Johanson et al., 2008). Because the CSF flow rate is much slower in younger animals, the sink effect was once thought to indicate greater permeability in the developing brain (Saunders et al., 2014).
The transient CSF-brain barrier
The CSF-brain barrier is not as well studied compared to the blood barriers, but it appears to have at least some implications for normal brain development and its dysfunction is associated with NDDs (Stolp et al., 2011; Stolp et al., 2013). Several studies have also suggested that the presence of the CSF-brain barrier provides increased protection in the fetal brain as compared to the adult (Cavanagh and Warren, 1985; Fossan et al., 1985; Møllgård et al., 1987; Whish et al., 2015).
Junctions between the cells lining the ventricular system of the developing brain have been identified since the 1960s in animal models and humans (Tennyson and Pappas, 1962; Duckett, 1968) (Figure 1), but Møllgård et al., 1987 was the first to describe the mechanical structure as a “strap” junction using electron microscopy in sheep fetuses. Strap junctions are described as modified tight junctions but differ in both their ultrastructure morphology (Møllgård et al., 1987) and genetic makeup (Whish et al., 2015); however, the function of strap junctions remains similar to tight junctions. Mammalian animal studies have demonstrated that strap junctions also restrict the passage of molecules from the CSF to the brain tissue (Fossan et al., 1985; Whish et al., 2015). Interestingly, the permeability of the barrier appears to become less restrictive over the course of development. Whish et al. (2015) demonstrated molecule diffusion across the barrier in mice embryos was restricted against the smallest molecules (286 Da). The permeability appeared to increase with age in the mice of the same study, with noted extravasation of the author’s largest tracer (70 kDa) in the adult (Whish et al., 2015). The authors noted that the increase in CSF-brain permeability correlate with the disappearance of the strap junctions at later stages of development. As animals age, strap junctions are replaced by gap and adherens junctions (Møllgård et al., 1987), but it is not clear when this switch takes place. One limitation of the study is the limited number of animals used, with one time point only having one representative pup, and lack of diversification of litters. Notwithstanding, the current evidence suggests that the fetal brain may be more restrictive than the adult brain with respects to the CSF-brain barrier.
Comparing the barriers
It is clear that the barriers are important for protecting the brain and maintaining its delicate homeostasis in both the developing and mature brain. Considering every brain cell is within approximately 25 µm from a capillary vessel (Abbott et al., 2010), the BBB protects the entirety of the brain parenchyma. In contrast, the responsibilities of the BCSFB lie at the more anatomically restricted location of the brain’s ventricles and at the pial surface. Within the ventricles, both the BCSFB and the CSF-brain barrier exist in the developing brain. The BCSFB prevents substrates from entering the CSF, while the CSF-brain barrier obstructs substrates in the CSF from freely entering cells of the ventricular epithelium. All three barriers mechanically block the passage of substrates through either their tight junctions (BBB, BCSFB) or strap junctions (CSF-brain). The BBB and BCSFB also have the ability to efflux compounds back into the bloodstream. The BCSFB shares many of the same transporters as the BBB (Redzic, 2011); however, transporter expression at each barrier is distinct (Ek et al., 2010; Morris et al., 2017; Huttunen et al., 2022).
The BBB and BCSFB also have different anatomical locations of their tight junctions. The tight junctions are localized to the luminal surface of the blood vessels of the BBB and the luminal surface of the choroid plexus of the BCSFB. However, the blood vessels invaginating the BCSFB do not contain tight junctions, permitting for the passage of macromolecules into the extracellular space bordering the choroidal epithelium (Brightman, 1968). In a non-disease state, molecules with a diameter of ∼12 nm may diffuse through the capillary pores into the extracellular space (Sarin, 2010). Molecules such as sucrose, inulin, albumin, and IgG do not cross the BBB but can slowly cross the choroid plexus (see Pardridge 2016 for review). As such, BCSFB is considered to exhibit low paracellular permeability in comparison to the BBB that exhibits near zero. The differences in permeability are also attributed to physical processes. Blood flow at the BCSFB is significantly faster than at the BBB, indicating more likely contact with blood-bound substrates. Despite the argument that the BCSFB is considered “leaky,” the BBB is propounded to be the major interface of concern. This is because the capillaries of the BBB come into contact with nearly every brain cell, whereas the CSF only contacts certain brain regions (Figure 1). This key difference is recognized in drug development. Therapeutics that enter the CSF are not often considered fully efficacious as they cannot permeate the brain tissue uniformly, and instead are preferentially delivered to cells that contact the CSF (Pardridge, 2016).
The developmental ontogeny of the barriers in animal models and humans
To directly compare animal models to humans, we will be using the terminology gestational day (GD) for animals and weeks post conception (WPC) for humans. Therefore, we have adjusted some of the published animal data to standardize that GD 0 indicates the first day of gestation, or the observed sperm positive day for rodents. However, some authors did not define what they considered the sperm positive date (e.g., GD 0, GD 0.5, or GD 1). Therefore, the discussed time points in this section may be within one gestational day for rodents. Any human data that used the terminology of “weeks pregnant” were translated to WPC assuming a 28-day menstrual cycle (e.g., 40 weeks pregnant is 38 weeks post conception). It is also important to note that the patterning of certain anatomical structures varies between species as it most likely relates to the length of gestation and brain growth (Dziegielewska et al., 2001). We will be focusing on data from mice, rats, and humans, which have average gestations of 19 days, 21 days, and 40 weeks, respectively.
Mammalian brain formation begins during embryogenesis, which is closely followed by its vascularization to allow for the tissue to receive the needed oxygen and nutrients. In rodent models, immunohistochemical stains show angiogenesis in the cortical brain structures starting at GD 11 for mice (Sturrock, 1979; Daneman et al., 2009) and GD 12 for rats (Daneman et al., 2010). This process in the human brain is believed to occur between 5 and 7 WPC (Allsopp and Gamble, 1979; Raybaud, 2010; Møllgård et al., 2017) as evidenced by immunohistochemical (Møllgård et al., 2017) and light and electron microscopy observations (Allsopp and Gamble, 1979). The choroid plexus develops after vascularization, and can be seen as early as GD 11 in mouse (Sturrock, 1979; Lun et al., 2015), GD 12 in rats (Dziegielewska et al., 2001), and 7 WPC in humans (Dziegielewska et al., 2001; Møllgård et al., 2017). These developmental time points remain fairly consistent throughout the literature; however, the formation and functionality of the barrier systems remains a point of contention between environmental toxicology and basic science.
The morphological basis of the barriers occurs at the level of cell junction complexes known as tight junctions (see “Cellular properties”). Immunohistochemical studies have suggested that BBB endothelial cells express tight junction proteins (e.g., occludin, claudin-5, and ZO-1) on the same day that angiogenesis begins in rats and mice (Daneman et al., 2010). It also appears that tight junctions are present as soon as blood vessels penetrate the human embryonic brain (Møllgård et al., 2017). Ultrastructural studies have also shown tight junctions in cerebral endothelial cells at the earliest age studied in mice (Bauer et al., 1993), rats (Donahue and Pappas, 1961), and humans (Møllgård and Saunders, 1975, 1986). Microscopy studies have repeatedly demonstrated that the developing brain barriers appear similar to the adult in multiple species (Bohr and Møllgård, 1974; Bradbury, 1979; Bauer et al., 1993; Bertossi et al., 1999; Virgintino et al., 2004). Additionally, efflux transporters (e.g., p-glycoprotein, breast cancer resistant protein, and multidrug resistant proteins), another critical component of barrier function, are detected via immunohistochemical staining and/or gene expression as early as GD 10.5–13 in mice (Qin and Sato, 1995; Tachikawa et al., 2005; Ek et al., 2010), GD 13 in rats (Daneman et al., 2010; Ek et al., 2010), and 5 WPC in humans (Møllgård et al., 2017). It should be noted that the expression of these transporters changes throughout development and are not identical to the adult. In some instances, the embryo/fetus will have higher expression of certain efflux proteins or upregulation of genes associated with tight junctions compared to the adult (Liddelow et al., 2012; Saunders et al., 2019).
Similar to the BBB, the BCSFB also appears to have function at the time of formation, suggesting that there is not a time period in which the embryo’s brain is completely susceptible to xenobiotics. The choroid plexus forms within the cerebral ventricles (see Dziegielewska et al., 2001 for review), with tight junctions present early in development. Ultrastructure studies have also demonstrated that tight junctions are morphologically similar to adults at the earliest stage studied in rats (GD 14) (Tauc et al., 1984) and humans (7 WPC) (Møllgård and Saunders, 1986). Limited ultrastructure studies have directly looked at tight junctions in mouse embryos; however, it appears that tight junctions are found on differentiating choroid plexus cells across mammalian species (Møllgård and Saunders, 1986; Ek et al., 2003). Efflux transporters are also detected in human (8 WPC) and rat (GD 15) embryos as evidenced by immunostaining (Møllgård et al., 2017) and transcriptomics (Kratzer et al., 2013). Similar results have also been demonstrated in mice (Liddelow et al., 2012).
Structure alone is insufficient to demonstrate a functionally adequate barrier. In support of the aforementioned data, the use of tracers has also signaled that the fetal barrier systems are indeed established during development. However, early tracer studies underwent the same scientifically insidious problem as some of the early dye studies (Behnsen, 1926, 1927; Penta, 1932; Saunders et al., 2015): injection of excessive volumes resulted in extravasation of the dye in the brain tissue, resulting in an inaccurate result that led to the conclusion of a leaky barrier. This experimental artifact was most likely due to toxicity and/or physically damaging the cerebral vessels with high injection volume (see Saunders et al., 2015 for review). However, animal models have found functional effectiveness of the nascent barriers. For example, both the BBB and BCSFB appear functional as early as GD 14 in the rat fetus against tracers like colloidal iron (Shimoda, 1963) and horseradish peroxidase (Tauc et al., 1984), respectively. Prior to the 1970s, the use of radioactively labeled tracers (e.g., sucrose, 32P, amino acids, proteins, etc.) did not accurately demonstrate BBB/BCSFB permeability as it was hard to distinguish between functionally deficient barriers and increased metabolism in the developing brain. It has since been established that metabolically important compounds are consumed at a greater rate than in the adult as opposed to the previously presumed “leakiness.” The more recent use of biotin-labeled small molecules suggest that the barriers are functioning nearly as soon as the brain is vascularized in both mice and rats (Daneman et al., 2010). Although the current discussion has been in mice and rats, it is worth mentioning that studies in the marsupial opossum, a species born at an early stage of brain development, has also demonstrated the obstruction of small molecules across the developmental barriers (Ek et al., 2001; Ek et al., 2003; Ek et al., 2006).
Information regarding human fetuses is incredibly limited. Gröntoft (1954) may be the only functional study (Figure 2C), but other immunocytochemical evidence has shown that endogenous compounds are selectively excluded from the brain. Dziegielewska and Saunders (1988) demonstrated that proteins do not easily pass intercellularly. More recently, Møllgård et al. (2017) found that α-fetoprotein, a plasma protein present in human embryonic circulation, was also excluded from the brain tissue as soon as the neural tube closed (5 WPC, Figures 2D–F). The surmounting evidence is suggestive that there is not a time period in which the barrier function turns “on.” Rather, functionality of the barriers appears almost immediate, with both the BBB and BCSFB exhibiting tight junctions, efflux proteins, and the ability to block molecules from crossing into the brain tissue.
Contaminants of immediate and emerging concern that may cross the brain barriers
In the previous sections, we reviewed the history and importance of the barrier systems and described the numerous experiments that have delineated their form and function. As designing therapeutics that can bypass the brain barriers is a longstanding bottleneck in neuropharmaceutical development (Pardridge, 2007), the BBB and BCSFB have received much attention in pharmacology. In contrast, the field of environmental toxicology has historically exhibited a more tepid regard to brain barrier biology. In the following sections we will evaluate the current data regarding two environmental contaminants of immediate and emerging concern that may enter brain tissue: per- and polyfluorinated substances (PFAS) and bisphenols. The developmental neurotoxicity of these chemicals are reviewed elsewhere (Mariussen, 2012; O'Shaughnessy et al., 2021; Denuziere and Ghersi-Egea, 2022; Welch and Mulligan, 2022), along with other environmental pollutants such as metals and pesticides (Ek et al., 2012).
Per- and polyfluoroalkyl substances (PFAS)
PFAS are a large family of anthropogenic compounds used in a variety of consumer and manufactured products such as electric or electronic parts, firefighting foams, hydraulic fluids, oil- and water-resistant clothing, and stain repellants (Buck et al., 2011). These chemicals are organofluorine with either partial or fully fluorinated alkyl chains; the strength of the carbon-fluorine bond makes these substances extremely stable. Their resistance to degradation has resulted in environmental ubiquity, and PFAS can be found in soil and water where they have never been used or manufactured. Humans can be exposed to PFAS occupationally, via ingestion of contaminated drinking water and food, and through inhalation or dermal exposure from sources like household dust and after application of household products (D’Hollander et al., 2010). PFAS was first discovered in human sera in 1968 (Taves, 1968) and has since been found in over 99% of human blood samples (Calafat et al., 2019), including umbilical cord blood (Kingsley et al., 2018). The most commonly detected PFAS in human samples include perfluorohexane sulfonic acid (PFHxS), perfluorooctane sulfonic acid (PFOS), perfluorooctanoic acid (PFOA), perfluorononanoic acid (PFNA), perfluorodecanoic acid (PFDA), and perfluoroundecanoic acid (PFUnA) (ATSDR, 2021). These chemicals often have long half-lives in humans, ranging from weeks to decades (ATSDR, 2021), which, combined with persistent exposure throughout the lifespan, further underscores the importance of understanding the potential neurotoxicity of these compounds.
Evaluation of PFAS concentrations in brain tissue has not been extensive, although some studies have identified these compounds within the brain parenchyma since at least the early 2000s (Kannan et al., 2001; Austin et al., 2003; Van de Vijver et al., 2005). This includes human brain tissue (Table 1) (Maestri et al., 2006; Perez et al., 2013; Mamsen et al., 2019). Both wildlife and in vivo experiments have claimed that PFAS crosses the adult BBB, as evidenced by quantifiable PFAS concentrations in brain tissue. However, most of these studies did not perform cardiac perfusion or a similar technique to appropriately exsanguinate organs of blood contamination. As discussed in this review, the brain is highly vascularized (Kirst et al., 2020). Therefore, failure to remove internal and/or residual blood should not be considered a “nonissue” (Greaves et al., 2013), especially when examining chemicals like PFAS which bind to blood proteins (e.g., albumin) (Forsthuber et al., 2020). For example, non-perfused brains from human, wildlife, and in vivo studies have shown detectable levels of PFOA. Interestingly, saline perfused brains of adult rats dosed with a large amount of PFOA (50 mg/kg/single dose, oral) did not have detectable concentrations within the brain tissue, while the average blood concentration in the exposed rats was much higher than, or comparable to, other in vivo data (Kawabata et al., 2017). To date, there have only been two studies that perfused animals prior to brain extraction and analysis (Lau et al., 2006; Kawabata et al., 2017). Lau et al. (2006) reported findings via a conference abstract, while Kawabata et al. (2017) showed that, similar to PFOA, PFDA brain concentrations in adult rats were <1/10 of serum concentrations following a single oral dose. However, perfluorodecanoic acid (PFDoA) brain concentrations were higher than the serum, even after saline perfusion to exsanguinate organs. This suggests that some PFAS may enter the brain better than others. Interestingly, PFDoA has a higher molecular weight (614 Da) than either PFOA (414 Da) or PFDA (514 Da), which implies that these differences are likely not due to simple diffusion. This is in contrast to other studies, where authors speculated that small PFAS diffuse across the brain barriers (Greaves et al., 2013; Pizzurro et al., 2019). In addition to the aforementioned experiments, some have attempted to demonstrate the distribution of PFAS in different brain regions (Austin et al., 2003; Eggers Pedersen et al., 2015). But again, these studies do not consider blood contamination and data interpretation is difficult. Blood vessel, diameter and density varies throughout the brain (Zhang et al., 2019), suggesting that some regions may appear to have higher concentrations of PFAS simply because there is more blood contamination in that anatomical area. Another consideration is that the inner regions of the brain (e.g., pons/medulla, hypothalamus, thalamus) are closest to incoming blood flow, placing those areas in contact with the highest xenobiotic concentrations if these compounds can indeed cross the BBB.
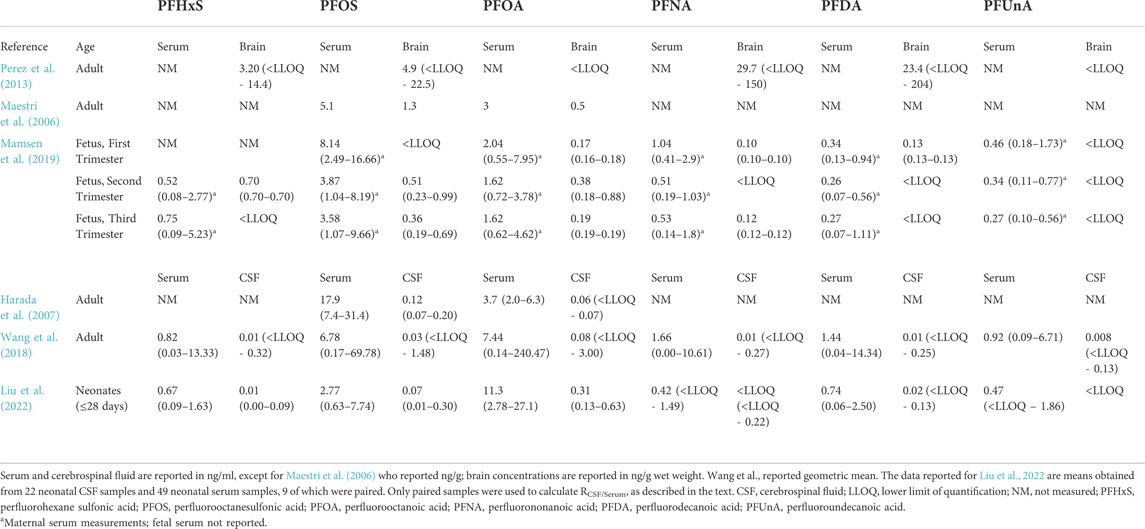
TABLE 1. Mean (range) concentrations of per- and polyfluorinated substances (PFAS) in human brain and sera samples.
There are several publications that have investigated PFAS in the human central nervous system. Two studies have documented PFAS in adult human CSF (Harada et al., 2007; Wang et al., 2018). Both Harada et al. (2007) and Wang et al. (2018) show that PFAS concentrations in the CSF were about 1% of those in serum, suggesting that these chemicals are not able to freely pass the BCSFB (see Table 1). However, both studies received CSF samples from patients that may have been afflicted with illnesses that could alter the integrity of either the BBB or BCSFB, which could result in increased levels of PFAS in the CSF. Thus, it is possible that levels are even lower in the general population. Limited developmental exposure studies exist in humans. Similar to reports by Harada and Wang, a new study investigated nine paired serum and CSF samples from human neonates (Liu et al., 2022). The authors show in human patients no older than 4 weeks old, the mean CSF:serum ratio (RCSF/Serum) of 32 different PFAS was never greater than 0.033 (i.e., ∼3%), and most PFAS in the CSF were below the lower limit of quantification (Liu et al., 2022, Table 1). It is important to note that lumbar punctures are not performed in newborns unless significant health concerns exist (Coughlan et al., 2021). These babies were likely suffering from an illness or disease that warranted this invasive procedure, although the precise disease state for individuals was not disclosed in the study design (Liu et al., 2022). While illness or developmental issues can cause brain barrier dysfunction, and CSF turnover is normally lower in the fetus/infant (see section 3.2 on sink effect), these babies still exhibited a relatively low RCSF/Serum. This indicates that PFAS are not freely entering the newborn CSF, although some (specifically linear isomers of PFHxS, PFOS, and PFOA) were still detectable (Liu et al., 2022). In another study, Mamsen et al. (2019) showed higher average levels of PFAS (PFHxS, PFOS, PFOA, PFNA, PFDA, PFUnA) in placenta as compared to the fetal CNS (first trimester samples consisted of spinal cord, second and third trimester samples consisted of brain), suggesting that the brain barriers are functioning. For example, all second and third trimester placentas had detectable levels of PFOS, while only half of the brain samples from these time periods had concentrations above the limit of detection. Mamsen and others also showed that their fetal CNS samples had the lowest PFAS burden of any fetal tissues sampled across all trimesters (Mamsen et al., 2019). The latter data are a sound indicator that the brain barriers are actively occluding these PFAS to at least some extent in the human fetus. In comparison to the adult brain concentrations reported in Perez et al. (2013) and Maestri et al. (2006), the average fetal brain PFAS concentrations were always less. However, paired PFAS concentrations in the blood and brain were not reported in the fetus (Mamsen et al., 2019), so it is not possible to make definitive conclusions regarding chemical transfer efficacy in the fetus versus adult. It is important to note that the fetal tissues in Mamsen et al. (2019) were obtained from both elective and spontaneous abortions, so it is possible that some of the fetuses suffered from major birth defects and/or abnormalities that could have affected brain barrier function. This could explain why some fetal CNS tissue had higher levels of PFAS while other samples did not.
Despite the identification of several PFAS compounds in both the adult and developing human brain, current in vivo developmental studies are limited and have utilized chickens (Cassone et al., 2012), mice (Borg et al., 2010), and rats (Lau et al., 2006; Chang et al., 2009; Wang et al., 2010; Zeng et al., 2011; Ishida et al., 2017) as model systems. Cassone et al. (2012) detected PFHxS in the cerebral cortex of chicken embryos, but there were no comments on the mechanism of PFHxS transport. The remaining developmental studies primarily focus on the eight carbon PFAS congeners (PFOA, PFOS) in a murine model (Tables 2, 3). From the published data, only Lau et al. (2006) quantified PFOS in saline perfused rat brain tissue after gestational exposure and found that pups had substantially higher levels as compared to the dams, despite dam blood concentrations being over double that of the fetus. This may suggest that PFOS enters the brain more readily in developing animals, but as discussed below, this may not be the case. Ishida et al. (2017) also measured PFOS concentrations in both dams and neonatal pups after gestational exposure but found similar serum concentrations in the adult and developing animals; however, the authors also detected significantly higher PFOS concentrations in the non-perfused pup brain as compared to adults. Chang et al. (2009), Wang et al. (2010), Macon et al. (2011), and Zeng et al. (2011) evaluated either PFOA or PFOS levels at different developmental time points in rats after gestational exposure and noted that brain concentrations decreased with age; however, authors did not comment on the synchronous decrease in blood levels. One postnatal study conducted by Liu et al. (2009) administered one large subcutaneous dose of PFOS (50 mg/kg) to young mice at different developmental time points and found comparable blood PFOS concentrations across the developmental stages tested. In contrast, the brain PFOS concentrations decreased as the mice aged, which could be explained by increased penetration of PFOS in young animals, or alternatively by a different hypothesis. Irrespective of the study, all authors attributed the PFOS concentrations in the young brains to an “incomplete,” “immature,” “porous,” and/or “undeveloped” BBB (Table 4). There are several possibilities for the observed trending decrease in cerebral PFAS concentrations in rodents. Borg et al. (2010) investigated heavy labeled PFOS distribution in the fetal rat and remarked uneven signal in the brain. The authors commented that PFOS did not appear concentrated in the fetal cortex, and their published autoradiograms instead show PFOS amassed in the ventricles. This is an interesting observation as the developing brain has a much lower turnover of CSF. The slow turnover rate results in slower clearance, and thus a greater accumulation, of compounds in the CSF as compared to older animals (Saunders et al., 2014). Relative to brain size, the developing brain also has much larger ventricles which could contribute to the higher levels of PFAS observed. Finally, the young postnatal brain also undergoes rapid expansion known as the brain growth spurt, and this occurs in both rodents and humans. Peak brain growth in rats occurs a week after birth (Dobbing and Sands, 1979). This corresponds to the observed decreasing level of PFAS in these aforementioned studies (Chang et al., 2009; Wang et al., 2010; Macon et al., 2011; Zeng et al., 2011), which express PFAS concentrations as nanogram of chemical per gram of brain parenchyma. In all, although some publications do report large amounts of PFAS in the brain, these studies are confounded by several technical variables. In humans and animal models that study either environmentally relevant PFAS levels and/or control for variables like blood contamination, it appears that the brain barriers occlude many of these chemicals to varying degrees.
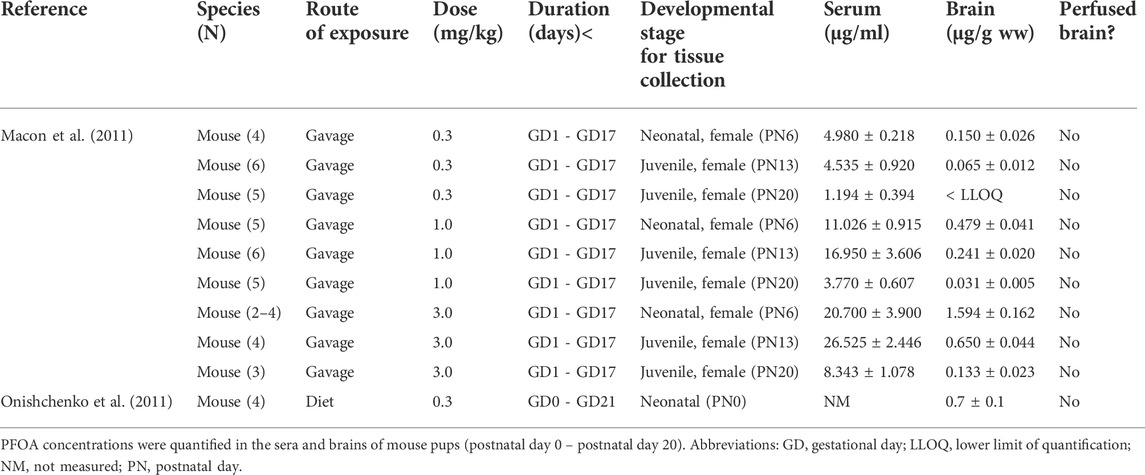
TABLE 2. Pregnant laboratory mice were exposed to perfluorooctanoic acid (PFOA) daily through different exposure routes, doses, and durations as provided below.
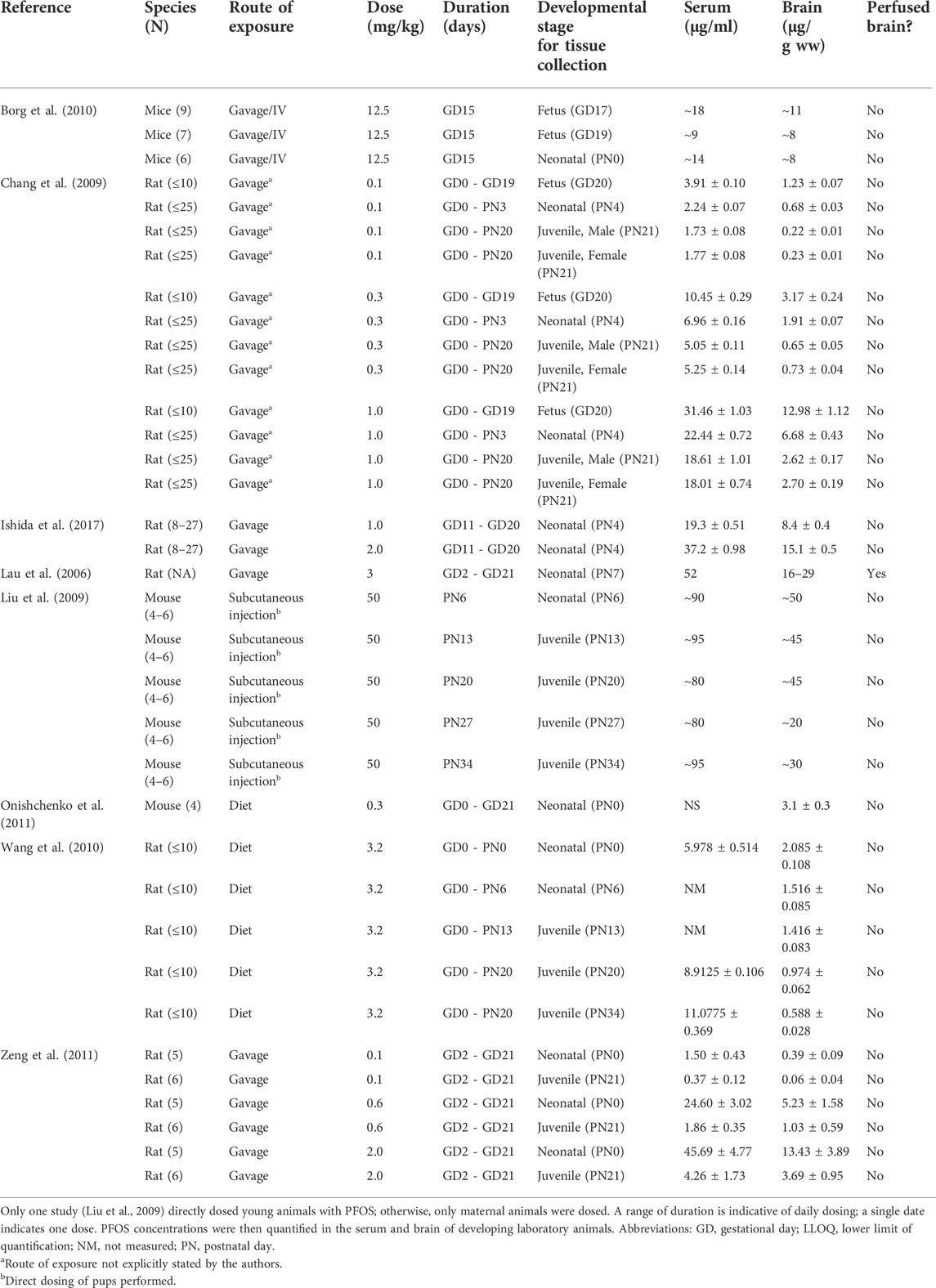
TABLE 3. Pregnant and developing laboratory animals were exposed to perfluorooctanesulfonic acid (PFOS) through different exposure routes, doses, and durations as provided below.
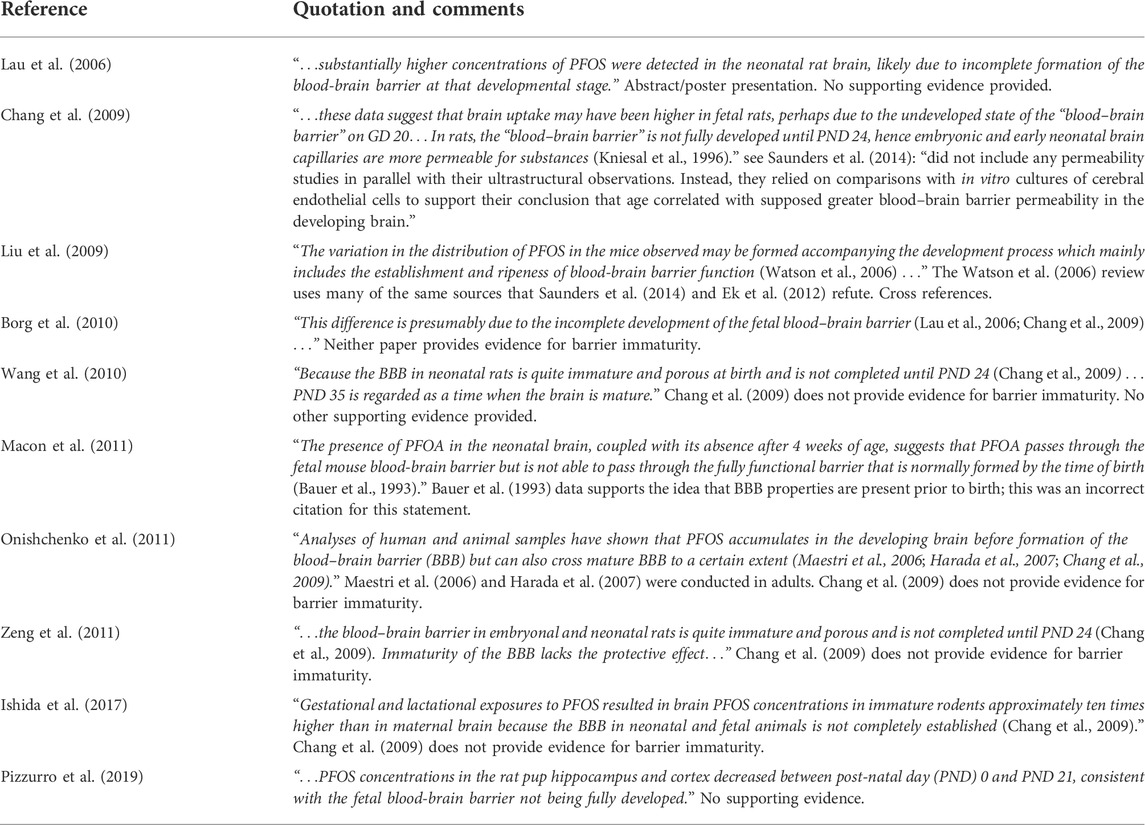
TABLE 4. Statements regarding an immature blood-brain barrier as reasoning for perfluorooctanoic acid (PFOA) or perfluorooctanesulfonic acid (PFOS) crossing into the developing brain with comments regarding the unsupported evidence.
There is very little information available about the mode of transport for PFAS across the brain barriers, including whether or not the compounds act similarly to one another in vivo. There is some speculation that PFAS could diffuse or actively pass through specific membrane transporters (Piekarski et al., 2020), but there are no studies that specifically address either hypothesis. However, the data at hand do not seem to support simple diffusion across the barriers, as the brain and CSF concentrations are much lower than blood levels for those that disseminated paired tissue samples. In addition, studies in animals and humans have shown that brain PFAS concentrations do not seem to correlate to their molecular weight (Kawabata et al., 2017; Wang et al., 2018); if simple diffusion was occuring, smaller PFAS would generally cross into the brain with greater efficacy. Instead, the data at hand suggests the brain barriers are restricting PFAS entry into the brain in developing and adult animals (including humans), but some PFAS are able to bypass the barriers by an unknown mechanism. One potential mechanism for PFAS entry in the adult brain is through alterations of tight junctions, and thus abnormal brain barrier function. Yu et al. (2020) observed decreases in the expression of tight junction-related proteins (ZO-1, claudin-5, claudin-11, occludin) and ultrastructural changes in the BBB in the cerebral cortex of adult mice orally exposed to PFOS. No functional assays were implemented, but their data suggest that PFOS could cross into the tissue through BBB disruption. Wang et al. (2018) showed that in their human samples, high estimates of brain barrier permeability in individuals corresponded to higher PFAS concentrations in CSF. As previously mentioned, these CSF samples were collected via lumbar puncture in adult patients that may have been afflicted with neurological disorders that caused brain barrier dysfunction irrespective of PFAS toxicity. No other studies have investigated BBB disruption in the developing brain. If BBB disruption is occurring, the developing brain could be left more susceptible to interactions with other blood-bound xenobiotics and/or pathogens. As there may be permanent neurological consequences due to early life perturbations of the brain barriers (Najjar et al., 2017; Greene et al., 2018; Pollak et al., 2018; Baruah et al., 2019; Medin et al., 2019; Kealy et al., 2020), further mechanistic and functional studies to address whether PFAS can disrupt the brain barriers are warranted.
Bisphenols
Bisphenols are a vast family of chemicals, but in vivo toxicity data exist for only a small subset (KEMI 2017; Pelch et al., 2017; Pelch et al., 2019). They are primarily used to make polycarbonate plastics and epoxy resins. Polycarbonate plastics are often used in products such as single-use beverage containers, reusable containers, tableware, and water pipes; epoxy resins can be used as protective lining in food and beverage cans, metal lids on containers, and dental sealants. Other commercial uses include electronics, floor sealants, medical devices, paints, personal care products, thermal receipts, and toys (Chen et al., 2016; Pelch et al., 2017). Human exposure is thought to primarily occur through diet, but other exposure routes include medical and dental products, and household dust (Vandenberg et al., 2007).
Bisphenol A (BPA) is the best studied bisphenol, and one of the most extensively investigated endocrine disrupting chemicals in toxicology. The compound’s estrogenic activity has been recognized since 1936 (Dodds and Lawson, 1936), and there is now evidence to suggest that it also interacts with androgen receptors, sex hormone-binding globulin (SHBG), and thyroid receptors (Mustieles et al., 2015; Vom Saal and Vandenberg, 2021). Growing concerns for BPA’s health effects in humans has resulted in the use of alternative analogues, commonly Bisphenol AF, F, and S (Chen et al., 2016). However, BPA is still often the dominant bisphenol detected in both abiotic and biological matrices (Chen et al., 2016). Production and usage data for bisphenols around the world is generally lacking (Chen et al., 2016), but it appears as though BPA is also one of the most produced chemicals in the world per year (OECD, 2009). Although BPA has been used in plastics since the 1950s (Vom Saal and Vandenberg, 2021) and known to leach from plastics since at least the early 1990s (Krishnan et al., 1993), human biomonitoring studies measuring BPA was not documented until 2005 (Calafat et al., 2005). Now, more than 90% of European and American urine samples have detectable concentrations of BPA (Mustieles and Fernandez, 2020). Studies have also measured this chemical in other human tissues, including blood and amniotic fluid (Ikezuki et al., 2002), but the published data has been under scrutiny as biomonitoring and kinetic studies have reached conflicting conclusions (Vandenberg et al., 2013; Vom Saal and Vandenberg, 2021). Every oral human pharmacokinetic study has shown that the half-life of total BPA (free and conjugated) in adult humans is approximately 6 h (Volkel et al., 2002; Thayer et al., 2015) and nearly 100% is eliminated through the urine within 24 h (Volkel et al., 2002; Volkel et al., 2005; Teeguarden et al., 2015; Thayer et al., 2015). More recently, Sasso et al. (2020) found the half-life of BPA via dermal exposure was slightly longer at approximately 20 h. Because humans can metabolize BPA rapidly, it is speculated that the high human exposure estimates (i.e., within the ng/ml range) may be erroneous, due to BPA contamination from the laboratory plastics used to collect, store, and process biological samples (Doerge et al., 2011; Vandenberg et al., 2013). Although plastic contamination is a potential issue that could artificially inflate exposure estimates, an analysis of CDC data from the National Health and Nutrition Examination Survey proposed that the half-life of BPA may be longer than expected, and humans are ubiquitously exposed via multiple routes (Stahlhut et al., 2009). This suggests that the wide human exposure ranges may not be entirely inaccurate and result from variations in exposure due to differing lifestyle choices.
Extensive literature (500+ articles) investigates the implications of BPA exposure on the brain (Patisaul, 2020), but very few studies evaluate BPA concentrations in the brain, the mechanism by which it may cross the brain barriers, and/or whether the observed endpoints are a result of BPA indirectly interacting with the brain (e.g., affecting the brain through an endocrine or inflammatory mediated mechanism). Corrales et al. (2015) compiled a total of 63 wildlife studies since 1999 that reported BPA concentrations in wildlife (mainly fish, invertebrates, and amphibians), and only one of the studies included chemical estimates in the brain (Renz et al., 2013). Since the 2015 compilation, other wildlife studies have documented the concentrations of bisphenols in the brains of different bird species (Gonzalez-Rubio et al., 2020; Bodziach et al., 2021) and fish (Ros et al., 2016). All three studies failed to provide for bisphenol serum concentrations, making it difficult to draw definitive conclusions regarding transfer across the BBB. Renz et al. (2013) posited that chemicals may be entering the fish brain by either crossing the BBB or via axonal transport. Bodziach et al. (2021) mentioned that the waterbird brains appeared protected from some of the bisphenols measured, but the authors did not speculate on the seemingly large observed BPA concentrations.
In vivo work in rats, mice, and monkeys has also shown BPA in brain tissue, but it has not been particularly consistent (Table 5). In addition to different animal models, studies vary by dosing scheme, the BPA analytes measured (aglycone, conjugated, or total), the brain compartment evaluated, and the time between the last administered BPA dose and tissue analysis. These vastly different experimental designs make it exceedingly difficult to compare results. For example, Shin et al. (2004) reported BPA-brain concentrations at almost double serum concentration levels in adult male rats given an intravenous injection (0.5 mg/kg) every 30 min for 4.5 h, whereas Yoo et al. (2000) found BPA-brain concentrations to be less than serum levels in adult male rats administered a simultaneous intravenous bolus injection (0.73 mg/kg) and infusion to steady state (0.5 mg/h). Neither study speculated on how the chemical reached relatively high concentrations in the brain. Kim et al. (2004) found that brain tissue concentration increased linearly with the oral dose and that there were comparable levels of BPA concentrations in seven different brain regions in the rat 48 h after oral dosing. The authors interpreted these results to mean that BPA can “penetrate [the brain] freely” (Kim et al., 2004). The authors did not mention their possibility of blood contamination in the brain from the lack of perfusion.
Consistent with the wildlife and in vivo studies, human studies show detectable levels of BPA in adult brain tissue. Geens et al. (2012), van der Meer et al. (2017), and Charisiadis et al. (2018) detected BPA in more than 70% of brain samples collected from postmortem adult humans at concentrations ranging from <0.4–26.62 ng/g. All studies reported relatively similar concentrations with median values of 0.57, 0.68 and 1.2 ng/g respectively (see Table 6). Charisiadis et al. (2018) also found almost double median BPF concentrations compared to BPA. van der Meer et al. (2017) and Charisiadis et al. (2018) determined that there was not any preferential accumulation of BPA or BPF between two different brain regions (hypothalamus and white matter tract), which the authors deemed suggestive of the chemicals crossing the BBB. The authors did not propose a potential mechanism for the compounds crossing, nor did they speculate about the potential contamination from residual blood in the brain tissue.
Most, if not all, studies have found some detectable amounts of BPA in the adult brain, but the blood: brain ratio is incredibly inconsistent when available for comparison. No study to date has performed cardiac perfusion to clear the brain of blood and its constituents, or attempted to correct for blood contamination. This suggests that there may be artificially inflated BPA estimates in the brain tissue. Authors often assume that the biologically active circulating BPA (aglycone) can easily and passively cross the adult BBB because of its lipophilic structure (Negri-Cesi, 2015; Santoro et al., 2019; Wang et al., 2019). Interestingly, the authors do not acknowledge that most substrates for the major BBB efflux proteins are lipophilic and contain some polar groups (Ek et al., 2012). One in vivo study showed that BPA can have weak modulatory effects on Breast Cancer Resistant Protein in rats (Nickel and Mahringer, 2014). Some in vitro evidence also suggests that BPA could be a P-glycoprotein substate (Mazur et al., 2012; Dankers et al., 2013); however, in vivo data are lacking. If BPA is interacting with efflux transporters like Breast Cancer Resistant Protein and P-glycoprotein, then several questions remain unanswered: is BPA crossing the BBB because of this interaction? Does this interaction allow for other endogenous or exogenous compounds to cross more easily? If so, could developmental exposure to BPA cause lasting increases in permeability of the BBB?
Unlike PFAS, the bisphenol literature does not often comment on the developing brain barriers. In vivo studies are limited, and brain concentrations often fall below the limit of detection (Domoradzki et al., 2003; Doerge et al., 2011; Patterson et al., 2013). Compared to other tissues, Doerge et al. (2011) found higher concentrations of aglycone BPA in the fetal rat brain following intravenous administration to dams; however, the level of aglycone BPA in fetal tissues dropped below the limit of detection if dams were dosed orally, indicating that the route of exposure can play an important role in tissue accumulation. The authors did not comment on how the chemical could traverse into the young brain. Uchida et al. (2002) estimated that BPA can reach the fetal brain within approximately 1 h for monkeys and 30 min for mice following subcutaneous injection to the mother. They concluded that the fetus is “indefensible” against BPA after it crosses the placenta, implying a lack of any additional barriers such as the BBB. In one developmental study, Mita et al. (2012) exposed mice to BPA from the first day of gestation through 1 week post birth and analyzed different tissues approximately 3 months after their last exposure. Despite having a short serum half-life in rodents (Doerge et al., 2011), authors reported brain tissue concentrations in the µg/g range (Mita et al., 2012). They also found that the male offspring of exposed mothers had the highest concentration of BPA in their brain tissue compared to other tissues, suggesting that BPA could have a longer half-life in the brain, or there was experimental contamination (Mita et al., 2012). Female offspring of exposed mothers did not exhibit the same trend. No serum BPA levels were reported, so conclusions are hard to draw (Mita et al., 2012). Much like the adult literature, the developmental neurotoxicology literature also contains many inconsistencies both within (Patterson et al., 2013) and across developmental studies.
Research regarding the degree to which bisphenols accumulate in the human fetus remains particularly uncertain (Corrales et al., 2015). Bisphenol analogues have been measured in cord blood (Liu et al., 2017; Kolatorova et al., 2018; Pan et al., 2020), with BPA having the highest concentration. BPA has been measured in human fetal cord blood at varying concentrations of 0.14–9.2 ng/ml (Corrales et al., 2015) and has been reported to reach concentrations five-fold higher in the amniotic fluid than in maternal serum in a Japanese cohort (Ikezuki et al., 2002). This is somewhat supported by in vivo pharmacokinetic work in sheep (Gingrich et al., 2019) in which BPA, but not other bisphenol congeners, were higher in fetal compartments. Concerningly, all tested bisphenols (BPA, BPS, and BPF) had a longer half-life in the fetus compared to the dam (Gingrich et al., 2019). However, other in vivo pharmacokinetic studies show amniotic fluid concentrations consistently lower than the corresponding maternal serum levels (Doerge et al., 2011; Patterson et al., 2013).
There is no general agreement on how much the embryo and fetus are truly exposed to bisphenols. Human fetal tissue characterization is especially challenging due to difficulties acquiring samples for analysis. As such, there are no publications to date that examine fetal brain concentrations. Both biomonitoring and in vivo studies have evidence to suggest that bisphenols can reach the fetus via maternal-placental transfer (Nishikawa et al., 2010; Gingrich et al., 2019). Thus, further study for the biodistribution and effects of bisphenol exposure in human neonates is needed. The developing brain is considered to be particularly sensitive to BPA (Pelch et al., 2017; Patisaul, 2020), and epidemiological studies suggest that neonatal exposure to BPA may be associated with altered neurodevelopment (see Ejaredar et al., 2017 and Mustieles and Fernandez 2020 for review). Additionally, current evidence suggests that circulating maternal BPA levels, a proxy for potential prenatal exposure, is more consistently associated with children’s neurobehavior than postnatal exposure (Mustieles and Fernandez, 2020). A large data gap exists investigating whether these changes in neurobehavior are associated with perturbations in the brain barriers, including BPA’s potential interaction with efflux proteins.
Closing remarks
While it is true that the young brain is vulnerable to environmental contaminants (Diamanti-Kandarakis et al., 2009; Landrigan and Goldman, 2011; Axelrad et al., 2013; Gore et al., 2014; Landrigan et al., 2019; O'Shaughnessy et al., 2021), this vulnerability does not imply a lack of protection. The brain barriers are a fundamental defense against foreign compounds and necessary for homeostasis at all life stages, including development. The widespread belief that the barriers are absent or leaky in the embryo and fetus is not founded on cumulative evidence. Instead, this dogma was likely perpetuated following the publication of several studies with contested and now overturned conclusions, stating that the developing brain does not possess functional barrier systems. This has inadvertently led to a large data gap regarding how environmental contaminants may, or may not, penetrate the young brain.
The two chemical classes reviewed here, PFAS and bisphenols, are some of the most extensively studied pollutants in environmental toxicology. While epidemiological and experimental evidence suggests some of these chemicals may enter the brain, this is inconclusive. The bulk of data for PFAS shows that their concentrations in the central nervous system are lower compared to other tissues at both early and late life stages. This suggests that the brain barriers are active even in the fetus, and these chemicals are not freely diffusing from the blood and to the brain. Data for BPA are more variable, with a wide range of findings regarding brain concentration versus other tissues (including blood). Unfortunately, nearly all the toxicology studies reviewed possess a common flaw: blood contamination in the brain tissue. Without removal of the blood or accurately estimating its contamination, chemical measures in the brain parenchyma will be artificially inflated and data interpretation difficult. One could potentially estimate blood contamination with paired serum/plasma and brain tissue concentrations, but this would not be straightforward. Experimental methods to remove or address blood as a confounder exist (Saunders et al., 2015), but may be difficult for some toxicology laboratories to implement. The most attainable approach is likely transcardiac perfusion using a physiological buffer like saline, which would exsanguinate the brain before performing chemical measurements. Perfusion is a methodology commonly used for in vivo research (see Gage et al., 2012). However, the success of a perfusion is highly dependent on an individual’s skill, as the perfusate flow rate can be either too low or high, leading to inefficient blood removal and/or ruptured microvasculature. It is critical that experienced personnel perform the procedure and care is taken to mimic a physiologically relevant flow rate. Methods like autoradiography permit accurate quantification of compound transfer across the barriers and can show spatial distribution, but these require radioactivity and may pose safety concerns (Bickel, 2005). In all, methodologies more commonplace in neuropharmacology can lead us to experimental approaches that will permit more accurate chemical quantification in brain tissue. This will lead to more soundly supported conclusions regarding a chemical’s ability to enter the brain.
It should be noted that a xenobiotic crossing into the brain does not immediately classify it as neurotoxic. A chemical is only neurotoxic if it exhibits either direct or indirect effects on the central nervous system. As such, a chemical can also possess neurotoxic activity without entering the brain, like through mediation of the endocrine system (e.g., thyroid disruptors) and/or action on the peripheral nervous system (e.g., organophosphates and inhibition of acetylcholinesterase). Nevertheless, chemicals that cross into the brain with high efficacy should be prioritized for thorough in vivo testing, as this is likely an uncommon occurrence (Pardridge, 2016). This knowledge also represents an opportunity for innovation - new approach methodologies that estimate a chemical’s ability to bypass the brain barriers could represent new ways to rapidly prioritize compounds for their neurotoxicity risk. In addition to a chemical crossing into the brain and exerting toxicity, xenobiotics may also influence the barriers’ physical and metabolic properties. Interestingly, it has been hypothesized in the last decade that BBB disruption may act as the initiating trigger of many neurological disorders, as opposed to being a consequence of disease (Stanimirovic and Friedman, 2012). While there are very few environmental toxicology studies that address this possibility, this idea can be captured under the Developmental Origins of Health and Adult Disease (DoHAD) framework (Heindel et al., 2015), and is an important area of future research. In conclusion, the brain barriers are an often overlooked consideration in developmental neurotoxicology. We cannot assume that a compound can freely enter the developing brain in either humans or laboratory animals given the plethora of basic science data that shows otherwise. In the future, planning experiments to account for brain barrier activity will lead to more accurate estimates of chemical transfer into the brain and could impact hazard estimates.
Author contributions
KB conceived the manuscript, and KB and KO wrote the manuscript.
Funding
This work was supported by US EPA’s Office of Research and Development.
Author Disclaimer
This document has been subjected to review by the Center for Public Health and Environmental Assessment and approved for publication. Approval does not signify that the contents reflect the views of the Agency, nor does mention of trade names or commercial products constitute endorsement or recommendation for use.
Acknowledgments
We thank Drs. Katerine Saili and Hisham El-Masri for their insightful comment on previous versions of this manuscript.
Conflict of interest
The authors declare that the research was conducted in the absence of any commercial or financial relationships that could be construed as a potential conflict of interest.
Publisher’s note
All claims expressed in this article are solely those of the authors and do not necessarily represent those of their affiliated organizations, or those of the publisher, the editors and the reviewers. Any product that may be evaluated in this article, or claim that may be made by its manufacturer, is not guaranteed or endorsed by the publisher.
References
Abbott, N. J., Dolman, D. E., and Patabendige, A. K. (2008). Assays to predict drug permeation across the blood-brain barrier, and distribution to brain. Curr. Drug Metab. 9 (9), 901–910. doi:10.2174/138920008786485182
Abbott, N. J., Patabendige, A. A., Dolman, D. E., Yusof, S. R., and Begley, D. J. (2010). Structure and function of the blood-brain barrier. Neurobiol. Dis. 37 (1), 13–25. doi:10.1016/j.nbd.2009.07.030
Abbott, N. J. (2005). Physiology of the blood–brain barrier and its consequences for drug transport to the brain. Int. Congr. Ser. 1277, 3–18. doi:10.1016/j.ics.2005.02.008
Abbott, N. J., Ronnback, L., and Hansson, E. (2006). Astrocyte-endothelial interactions at the blood-brain barrier. Nat. Rev. Neurosci. 7 (1), 41–53. doi:10.1038/nrn1824
Aird, W. C. (2007b). Phenotypic heterogeneity of the endothelium: II. Representative vascular beds. Circ. Res. 100 (2), 174–190. doi:10.1161/01.RES.0000255690.03436.ae
Aird, W. C. (2007a). Phenotypic heterogeneity of the endothelium: I. Structure, function, and mechanisms. Circ. Res. 100 (2), 158–173. doi:10.1161/01.RES.0000255691.76142.4a
Allsopp, G., and Gamble, H. J. (1979). Light and electron microscopic observations on the development of the blood vascular system of the human brain. J. Anat. 128, 461–477.
Armulik, A., Genove, G., Mae, M., Nisancioglu, M. H., Wallgard, E., Niaudet, C., et al. (2010). Pericytes regulate the blood-brain barrier. Nature 468 (7323), 557–561. doi:10.1038/nature09522
Austin, M. E., Kasturi, B. S., Barber, M., Kannan, K., MohanKumar, P. S., and MohanKumar, S. M. (2003). Neuroendocrine effects of perfluorooctane sulfonate in rats. Environ. Health Perspect. 111 (12), 1485–1489. doi:10.1289/ehp.6128
Axelrad, D., Adams, K., Chowdhury, F., D’Amico, L., Douglass, E., Hudson, G., et al. (2013). America’s children and the environment. Third EditionThird ed. Washington, D.C.: United States Environmental Protection Agency.
Baruah, J., Vasudevan, A., and Kohling, R. (2019). Vascular integrity and signaling determining brain development, network excitability, and epileptogenesis. Front. Physiol. 10, 1583. doi:10.3389/fphys.2019.01583
Bauer, H. C., Bauer, H., Lametschwandtner, A., Amberger, A., Ruiz, P., and Steiner, M. (1993). Neovascularization and the appearance of morphological characteristics of the blood-brain barrier in the embryonic mouse central nervous system. Dev. Brain Res. 75 (2), 269–278. doi:10.1016/0165-3806(93)90031-5
Bauer, H. C., Krizbai, I. A., Bauer, H., and Traweger, A. (2014). “You Shall Not Pass”-tight junctions of the blood brain barrier. Front. Neurosci. 8, 392. doi:10.3389/fnins.2014.00392
Behnsen, G. (1926). Farbstoffversuche mit Trypanblau an der Schranke zwischen Blut und Zentralnervensystem der wachsenden Maus. Münch. Med. Wochenschrif 73, 1143–1147.
Behnsen, G. (1927). Über die Farbstoffspeicherung im Zentralnervensystem der eissen Maus in verschiedenen Alterszuständen. Z. fr Zellforsch. Mikrosk. Anat. 4 (4), 515–572. doi:10.1007/bf00637105
Bentivoglio, M., and Kristensson, K. (2014). Tryps and trips: Cell trafficking across the 100-year-old blood-brain barrier. Trends Neurosci. 37 (6), 325–333. doi:10.1016/j.tins.2014.03.007
Berndt, P., Winkler, L., Cording, J., Breitkreuz-Korff, O., Rex, A., Dithmer, S., et al. (2019). Tight junction proteins at the blood-brain barrier: Far more than claudin-5. Cell Mol. Life Sci. 76 (10), 1987–2002. doi:10.1007/s00018-019-03030-7
Bertossi, M., Virgintino, D., Errede, M., and Roncali, L. (1999). Immunohistochemical and ultrastructural characterization of cortical plate microvasculature in the human fetus telencephalon. Microvasc. Res. 58 (1), 49–61. doi:10.1006/mvre.1999.2154
Bickel, U. (2005). How to measure drug transport across the blood-brain barrier. NeuroRx 2 (1), 15–26. doi:10.1602/neurorx.2.1.15
Bodziach, K., Staniszewska, M., Falkowska, L., Nehring, I., Ożarowska, A., Zaniewicz, G., et al. (2021). Distribution paths of endocrine disrupting phenolic compounds in waterbirds (Mergus merganser, Alca torda, Clangula hyemalis) from the Southern Baltic. Sci. Total Environ. doi:10.1016/j.scitotenv.2021.148556
Bohr, V., and Møllgård, K. (1974). Tight junctions in human fetal choroid plexus visualized by freeze-etching. Brain Res. 81 (2), 314–318. doi:10.1016/0006-8993(74)90945-7
Borg, D., Bogdanska, J., Sundstrom, M., Nobel, S., Hakansson, H., Bergman, A., et al. (2010). Tissue distribution of (35)S-labelled perfluorooctane sulfonate (PFOS) in C57Bl/6 mice following late gestational exposure. Reprod. Toxicol. 30 (4), 558–565. doi:10.1016/j.reprotox.2010.07.004
Bradbury, M. (1979). “The blood-brain barrier during the development of the individual and the evolution of the phylum,” in The concept of the blood-brain barrier. Editor M. Bradbury (New York: John Wiley & Sons), 289–322.
Brightman, M. W. (1968). The intracerebral movement of proteins injected into blood and cerebrospinal fluid of mice. Prog. Brain Res. 29, 19–40. doi:10.1016/s0079-6123(08)64147-3
Buck, R. C., Franklin, J., Berger, U., Conder, J. M., Cousins, I. T., de Voogt, P., et al. (2011). Perfluoroalkyl and polyfluoroalkyl substances in the environment: Terminology, classification, and origins. Integr. Environ. Assess. Manag. 7 (4), 513–541. doi:10.1002/ieam.258
Budday, S., Steinmann, P., and Kuhl, E. (2015). Physical biology of human brain development. Front. Cell Neurosci. 9, 257. doi:10.3389/fncel.2015.00257
Calafat, A. M., Kato, K., Hubbard, K., Jia, T., Botelho, J. C., and Wong, L. Y. (2019). Legacy and alternative per- and polyfluoroalkyl substances in the U.S. general population: Paired serum-urine data from the 2013-2014 National Health and Nutrition Examination Survey. Environ. Int. 131, 105048. doi:10.1016/j.envint.2019.105048
Calafat, A. M., Kuklenyik, Z., Reidy, J. A., Caudill, S. P., Ekong, J., and Needham, L. L. (2005). Urinary concentrations of bisphenol A and 4-nonylphenol in a human reference population. Environ. Health Perspect. 113 (4), 391–395. doi:10.1289/ehp.7534
Caley, D. W., and Maxwell, D. S. (1970). Development of the blood vessels and extracellular spaces during postnatal maturation of rat cerebral cortex. J. Comp. Neurol. 138 (1), 31–47. doi:10.1002/cne.901380104
Cassone, C. G., Vongphachan, V., Chiu, S., Williams, K. L., Letcher, R. J., Pelletier, E., et al. (2012). In ovo effects of perfluorohexane sulfonate and perfluorohexanoate on pipping success, development, mRNA expression, and thyroid hormone levels in chicken embryos. Toxicol. Sci. 127 (1), 216–224. doi:10.1093/toxsci/kfs072
Cavanagh, M. E., and Warren, A. (1985). The distribution of native albumin and foreign albumin injected into lateral ventricles of prenatal and neonatal rat forebrains. Anat. Embryol. 172 (3), 345–351. doi:10.1007/bf00318983
Chang, S. C., Ehresman, D. J., Bjork, J. A., Wallace, K. B., Parker, G. A., Stump, D. G., et al. (2009). Gestational and lactational exposure to potassium perfluorooctanesulfonate (K+PFOS) in rats: Toxicokinetics, thyroid hormone status, and related gene expression. Reprod. Toxicol. 27 (3-4), 387–399. doi:10.1016/j.reprotox.2009.01.005
Charisiadis, P., Andrianou, X. D., van der Meer, T. P., den Dunnen, W. F. A., Swaab, D. F., Wolffenbuttel, B. H. R., et al. (2018). Possible obesogenic effects of bisphenols accumulation in the human brain. Sci. Rep. 8 (1), 8186. doi:10.1038/s41598-018-26498-y
Chen, D., Kannan, K., Tan, H., Zheng, Z., Feng, Y. L., Wu, Y., et al. (2016). Bisphenol analogues other than BPA: Environmental occurrence, human exposure, and toxicity-A review. Environ. Sci. Technol. 50 (11), 5438–5453. doi:10.1021/acs.est.5b05387
Cohen, H., and Davies, S. (1938). The morphology and permeability of the roof of the fourth ventricle in some mammalian embryos. J. Anat. 72, 430–455.
Corrales, J., Kristofco, L. A., Steele, W. B., Yates, B. S., Breed, C. S., Williams, E. S., et al. (2015). Global assessment of bisphenol A in the environment: Review and analysis of its occurrence and bioaccumulation. Dose Response 13 (3), 1559325815598308. doi:10.1177/1559325815598308
Coughlan, S., Elbadry, M., Salama, M., Divilley, R., Stokes, H. K., and O'Neill, M. B. (2021). The current use of lumbar puncture in a general paediatric unit. Ir. Med. J. 114 (5), 354.
Daneman, R., Agalliu, D., Zhou, L., Kuhnert, F., Kuo, C. J., and Barres, B. A. (2009). Wnt/beta-catenin signaling is required for CNS, but not non-CNS, angiogenesis. Proc. Natl. Acad. Sci. U. S. A. 106 (2), 641–646. doi:10.1073/pnas.0805165106
Daneman, R., and Prat, A. (2015). The blood-brain barrier. Cold Spring Harb. Perspect. Biol. 7 (1), a020412. doi:10.1101/cshperspect.a020412
Daneman, R., Zhou, L., Kebede, A. A., and Barres, B. A. (2010). Pericytes are required for blood-brain barrier integrity during embryogenesis. Nature 468 (7323), 562–566. doi:10.1038/nature09513
Dankers, A. C., Roelofs, M. J., Piersma, A. H., Sweep, F. C., Russel, F. G., van den Berg, M., et al. (2013). Endocrine disruptors differentially target ATP-binding cassette transporters in the blood-testis barrier and affect Leydig cell testosterone secretion in vitro. Toxicol. Sci. 136 (2), 382–391. doi:10.1093/toxsci/kft198
de Lange, E. C. (2013). The mastermind approach to CNS drug therapy: Translational prediction of human brain distribution, target site kinetics, and therapeutic effects. Fluids Barriers CNS 10 (1), 12. doi:10.1186/2045-8118-10-12
Denuziere, A., and Ghersi-Egea, J. F. (2022). Cerebral concentration and toxicity of endocrine disrupting chemicals: The implication of blood-brain interfaces. Neurotoxicology 91, 100–118. doi:10.1016/j.neuro.2022.04.004
D’Hollander, W., de Voogt, P., De Coen, W., and Bervoets, L. (2010). “Perfluorinated substances in human food and other sources of human exposure,” in Reviews of environmental contamination and toxicology volume 208: Perfluorinated alkylated substances. Editor P. De Voogt (New York, NYNew York: Springer), 179–215.
Diamanti-Kandarakis, E., Bourguignon, J. P., Giudice, L. C., Hauser, R., Prins, G. S., Soto, A. M., et al. (2009). Endocrine-disrupting chemicals: An endocrine society scientific statement. Endocr. Rev. 30 (4), 293–342. doi:10.1210/er.2009-0002
Dobbing, J., and Sands, J. (1979). Comparative aspects of the brain growth spurt. Early Hum. Dev. 3 (1), 79–83. doi:10.1016/0378-3782(79)90022-7
Dodds, E. C., and Lawson, W. (1936). Synthetic strogenic agents without the phenanthrene nucleus. Nature 137 (3476), 996. doi:10.1038/137996a0
Doerge, D. R., Twaddle, N. C., Vanlandingham, M., Brown, R. P., and Fisher, J. W. (2011). Distribution of bisphenol A into tissues of adult, neonatal, and fetal Sprague-Dawley rats. Toxicol. Appl. Pharmacol. 255 (3), 261–270. doi:10.1016/j.taap.2011.07.009
Dohrmann, G. J., and Bucy, P. C. (1970). Human choroid plexus: A light and electron microscopic study. J. Neurosurg. 33 (5), 506–516. doi:10.3171/jns.1970.33.5.0506
Domoradzki, J. Y., Pottenger, L. H., Thornton, C. M., Hansen, S. C., Card, T. L., Markham, D. A., et al. (2003). Metabolism and pharmacokinetics of bisphenol A (BPA) and the embryo-fetal distribution of BPA and BPA-monoglucuronide in CD Sprague-Dawley rats at three gestational stages. Toxicol. Sci. 76 (1), 21–34. doi:10.1093/toxsci/kfg206
Donahue, S., and Pappas, G. D. (1961). The fine structure of capillaries in the cerebral cortex of the rat at various stages of development. Am. J. Anat. 108, 331–347. doi:10.1002/aja.1001080307
Duckett, S. (1968). The germinal layer of the growing human brain during early fetal life. Anat. Rec. 161 (2), 231–245. doi:10.1002/ar.1091610208
Dziegielewska, K. M., Ek, J., Habgood, M. D., and Saunders, N. R. (2001). Development of the choroid plexus. Microsc. Res. Tech. 52 (1), 5–20. doi:10.1002/1097-0029(20010101)52:1<5:Aid-jemt3>3.0.Co;2-j
Dziegielewska, K. M., and Saunders, N. R. (1988). “The development of the blood-brain barrier: Proteins in fetal and neonatal CSF, their nature and origins,” in Handbook of human growth and biological development. Editors E. Meisami, and P. J. Timiras (Boca Raton, Florida: CRC Press), 169–191.
Eggers Pedersen, K., Basu, N., Letcher, R., Greaves, A. K., Sonne, C., Dietz, R., et al. (2015). Brain region-specific perfluoroalkylated sulfonate (PFSA) and carboxylic acid (PFCA) accumulation and neurochemical biomarker responses in east Greenland polar bears (Ursus maritimus). Environ. Res. 138, 22–31. doi:10.1016/j.envres.2015.01.015
Ehrlich, P. (1885). Das Sauerstoffbedürfnis des Organismus. Eine farbenanalytische Studie. Berlin: Verlag von August Hirschwald.
Ejaredar, M., Lee, Y., Roberts, D. J., Sauve, R., and Dewey, D. (2017). Bisphenol A exposure and children's behavior: A systematic review. J. Expo. Sci. Environ. Epidemiol. 27 (2), 175–183. doi:10.1038/jes.2016.8
Ek, C. J., Dziegielewska, K. M., Habgood, M. D., and Saunders, N. R. (2012). Barriers in the developing brain and Neurotoxicology. Neurotoxicology 33 (3), 586–604. doi:10.1016/j.neuro.2011.12.009
Ek, C. J., Dziegielewska, K. M., Stolp, H., and Saunders, N. R. (2006). Functional effectiveness of the blood-brain barrier to small water-soluble molecules in developing and adult opossum (Monodelphis domestica). J. Comp. Neurol. 496 (1), 13–26. doi:10.1002/cne.20885
Ek, C. J., Habgood, M. D., Dziegielewska, K. M., Potter, A., and Saunders, N. R. (2001). Permeability and route of entry for lipid-insoluble molecules across brain barriers in developing Monodelphis domestica. J. Physiol. 536 (3), 841–853. doi:10.1111/j.1469-7793.2001.00841.x
Ek, C. J., Habgood, M. D., Dziegielewska, K. M., and Saunders, N. R. (2003). Structural characteristics and barrier properties of the choroid plexuses in developing brain of the opossum (Monodelphis Domestica). J. Comp. Neurol. 460 (4), 451–464. doi:10.1002/cne.10661
Ek, C. J., Wong, A., Liddelow, S. A., Johansson, P. A., Dziegielewska, K. M., and Saunders, N. R. (2010). Efflux mechanisms at the developing brain barriers: ABC-transporters in the fetal and postnatal rat. Toxicol. Lett. 197 (1), 51–59. doi:10.1016/j.toxlet.2010.04.025
Enerson, B. E., and Drewes, L. R. (2006). The rat blood-brain barrier transcriptome. J. Cereb. Blood Flow. Metab. 26 (7), 959–973. doi:10.1038/sj.jcbfm.9600249
Fenstermacher, J., Nakata, H., Tajima, A., Lin, S. Z., Otsuka, T., Acuff, V., et al. (1991). Functional variations in parenchymal microvascular systems within the brain. Magn. Reson Med. 19 (2), 217–220. doi:10.1002/mrm.1910190205
Forsthuber, M., Kaiser, A. M., Granitzer, S., Hassl, I., Hengstschlager, M., Stangl, H., et al. (2020). Albumin is the major carrier protein for PFOS, PFOA, PFHxS, PFNA and PFDA in human plasma. Environ. Int. 137, 105324. doi:10.1016/j.envint.2019.105324
Fossan, G., Cavanagh, M. E., Evans, C. A., Malinowska, D. H., Møllgård, K., Reynolds, M. L., et al. (1985). CSF-Brain permeability in the immature sheep fetus: A CSF-brain barrier. Brain Res. 350 (1-2), 113–124. doi:10.1016/0165-3806(85)90255-x
Friden, M., Winiwarter, S., Jerndal, G., Bengtsson, O., Wan, H., Bredberg, U., et al. (2009). Structure-brain exposure relationships in rat and human using a novel data set of unbound drug concentrations in brain interstitial and cerebrospinal fluids. J. Med. Chem. 52 (20), 6233–6243. doi:10.1021/jm901036q
Furuse, M., Fujita, K., Hiiragi, T., Fujimoto, K., and Tsukita, S. (1998). Claudin-1 and -2: Novel integral membrane proteins localizing at tight junctions with no sequence similarity to occludin. J. Cell Biol. 141 (7), 1539–1550. doi:10.1083/jcb.141.7.1539
Gage, G. J., Kipke, D. R., and Shain, W. (2012). Whole animal perfusion fixation for rodents. J. Vis. Exp. 65. doi:10.3791/3564
Geens, T., Neels, H., and Covaci, A. (2012). Distribution of bisphenol-A, triclosan and n-nonylphenol in human adipose tissue, liver and brain. Chemosphere 87 (7), 796–802. doi:10.1016/j.chemosphere.2012.01.002
Gingrich, J., Pu, Y., Ehrhardt, R., Karthikraj, R., Kannan, K., and Veiga-Lopez, A. (2019). Toxicokinetics of bisphenol A, bisphenol S, and bisphenol F in a pregnancy sheep model. Chemosphere 220, 185–194. doi:10.1016/j.chemosphere.2018.12.109
Goldmann, E. E. (1909). Die äussere und innere Sekretion des gesunden und kranken Organismus im Lichte der "vitalen Färbung. Tüb. H. Laupp.
Goldmann, E. E. (1913). Vitalfärbung am Zentralnervensystem : Beitrag zur Physio-Pathologie des Plexus chorioideus und der Hirnhäute. Berlin: Königl. Akademie der Wissenschaften.
Gonzalez-Rubio, S., Vike-Jonas, K., Gonzalez, S. V., Ballesteros-Gomez, A., Sonne, C., Dietz, R., et al. (2020). Bioaccumulation potential of bisphenols and benzophenone UV filters: A multiresidue approach in raptor tissues. Sci. Total Environ. 741, 140330. doi:10.1016/j.scitotenv.2020.140330
Gore, A. C., Crews, D., Doan, L. L., Merrill, M. L., and Zota, A. R. (2014). INTRODUCTION TO ENDOCRINE DISRUPTING CHEMICALS (EDCs) A GUIDE FOR PUBLIC INTEREST ORGANIZATIONS AND POLICY-MAKERS.()
Grandjean, P., and Landrigan, P. J. (2006). Developmental neurotoxicity of industrial chemicals. Lancet 368 (9553), 2167–2178. doi:10.1016/s0140-6736(06)69665-7
Grandjean, P., and Landrigan, P. J. (2014). Neurobehavioural effects of developmental toxicity. Lancet Neurology 13 (3), 330–338. doi:10.1016/s1474-4422(13)70278-3
Grazer, F. M., and Clemente, C. D. (1957). Developing blood brain barrier to trypan blue. Proc. Soc. Exp. Biol. Med. 94 (4), 758–760. doi:10.3181/00379727-94-23077
Greaves, A. K., Letcher, R. J., Sonne, C., and Dietz, R. (2013). Brain region distribution and patterns of bioaccumulative perfluoroalkyl carboxylates and sulfonates in east Greenland polar bears (Ursus maritimus). Environ. Toxicol. Chem. 32 (3), 713–722. doi:10.1002/etc.2107
Greene, C., Kealy, J., Humphries, M. M., Gong, Y., Hou, J., Hudson, N., et al. (2018). Dose-dependent expression of claudin-5 is a modifying factor in schizophrenia. Mol. Psychiatry 23 (11), 2156–2166. doi:10.1038/mp.2017.156
Gröntoft, O. (1954). Intracranial haemorrhage and blood-brain barrier problems in the newborn. Acta Pathol. Microbiol. Scand. Suppl. 100, 1–109.
Gunzel, D., and Yu, A. S. (2013). Claudins and the modulation of tight junction permeability. Physiol. Rev. 93 (2), 525–569. doi:10.1152/physrev.00019.2012
Harada, K. H., Hashida, S., Kaneko, T., Takenaka, K., Minata, M., Inoue, K., et al. (2007). Biliary excretion and cerebrospinal fluid partition of perfluorooctanoate and perfluorooctane sulfonate in humans. Environ. Toxicol. Pharmacol. 24 (2), 134–139. doi:10.1016/j.etap.2007.04.003
Heindel, J. J., Balbus, J., Birnbaum, L., Brune-Drisse, M. N., Grandjean, P., Gray, K., et al. (2015). Developmental origins of health and disease: Integrating environmental influences. Endocrinology 156 (10), 3416–3421. doi:10.1210/EN.2015-1394
Heyer, D. B., and Meredith, R. M. (2017). Environmental toxicology: Sensitive periods of development and neurodevelopmental disorders. Neurotoxicology 58, 23–41. doi:10.1016/j.neuro.2016.10.017
Huttunen, K. M., Terasaki, T., Urtti, A., Montaser, A. B., and Uchida, Y. (2022). Pharmacoproteomics of brain barrier transporters and substrate design for the brain targeted drug delivery. Pharm. Res. 39 (7), 1363–1392. doi:10.1007/s11095-022-03193-2
Iadecola, C., and Nedergaard, M. (2007). Glial regulation of the cerebral microvasculature. Nat. Neurosci. 10 (11), 1369–1376. doi:10.1038/nn2003
Ikezuki, Y., Tsutsumi, O., Takai, Y., Kamei, Y., and Taketani, Y. (2002). Determination of bisphenol A concentrations in human biological fluids reveals significant early prenatal exposure. Hum. Reprod. 17 (11), 2839–2841. doi:10.1093/humrep/17.11.2839
International Transporter, C., Giacomini, K. M., Huang, S. M., Tweedie, D. J., Benet, L. Z., Brouwer, K. L., et al. (2010). Membrane transporters in drug development. Nat. Rev. Drug Discov. 9 (3), 215–236. doi:10.1038/nrd3028
Iqubal, A., Ahmed, M., Ahmad, S., Sahoo, C. R., Iqubal, M. K., and Haque, S. E. (2020). Environmental neurotoxic pollutants: Review. Environ. Sci. Pollut. Res. Int. 27 (33), 41175–41198. doi:10.1007/s11356-020-10539-z
Ishida, K., Tsuyama, Y., Sanoh, S., Ohta, S., and Kotake, Y. (2017). Perfluorooctane sulfonate induces neuronal vulnerability by decreasing GluR2 expression. Arch. Toxicol. 91 (2), 885–895. doi:10.1007/s00204-016-1731-x
Johanson, C. E., Duncan, J. A., Klinge, P. M., Brinker, T., Stopa, E. G., and Silverberg, G. D. (2008). Multiplicity of cerebrospinal fluid functions: New challenges in health and disease. Cerebrospinal Fluid Res. 5, 10. doi:10.1186/1743-8454-5-10
Johanson, C. E. (1980). Permeability and vascularity of the developing brain: Cerebellum vs cerebral cortex. Brain Res. 190 (1), 3–16. doi:10.1016/0006-8993(80)91155-5
Judson, R., Richard, A., Dix, D. J., Houck, K., Martin, M., Kavlock, R., et al. (2009). The toxicity data landscape for environmental chemicals. Environ. Health Perspect. 117 (5), 685–695. doi:10.1289/ehp.0800168
Kannan, K., Koistinen, J., Beckmen, K., Evans, T., Gorzelany, J. F., Hansen, K. J., et al. (2001). Accumulation of perfluorooctane sulfonate in marine mammals. Environ. Sci. Technol. 35 (8), 1593–1598. doi:10.1021/es001873w
Kawabata, K., Matsuzaki, H., Nukui, S., Okazaki, M., Sakai, A., Kawashima, Y., et al. (2017). Perfluorododecanoic acid induces cognitive deficit in adult rats. Toxicol. Sci. 157 (2), 421–428. doi:10.1093/toxsci/kfx058
Kealy, J., Greene, C., and Campbell, M. (2020). Blood-brain barrier regulation in psychiatric disorders. Neurosci. Lett. 726, 133664. doi:10.1016/j.neulet.2018.06.033
KEMI (2017). Bisfenoler – en kartläggning och analys Rapport från ett deluppdrag inom Handlingsplanen för en giftfri vardag. Availableat: https://www.kemi.se/download/18.60cca3b41708a8aecdbba25d/1586867003323/rapport-5-17-bisfenoler-en-kartlaggning-och-analys.pdf.
Kim, C. S., Sapienza, P. P., Ross, I. A., Johnson, W., Luu, H. M., and Hutter, J. C. (2004). Distribution of bisphenol A in the neuroendocrine organs of female rats. Toxicol. Ind. Health 20 (1-5), 41–50. doi:10.1191/0748233704th186oa
Kingsley, S. L., Eliot, M. N., Kelsey, K. T., Calafat, A. M., Ehrlich, S., Lanphear, B. P., et al. (2018). Variability and predictors of serum perfluoroalkyl substance concentrations during pregnancy and early childhood. Environ. Res. 165, 247–257. doi:10.1016/j.envres.2018.04.033
Kirst, C., Skriabine, S., Vieites-Prado, A., Topilko, T., Bertin, P., Gerschenfeld, G., et al. (2020). Mapping the fine-scale organization and plasticity of the brain vasculature. Cell 180 (4), 780–795 e725. doi:10.1016/j.cell.2020.01.028
Kolatorova, L., Vitku, J., Hampl, R., Adamcova, K., Skodova, T., Simkova, M., et al. (2018). Exposure to bisphenols and parabens during pregnancy and relations to steroid changes. Environ. Res. 163, 115–122. doi:10.1016/j.envres.2018.01.031
Kratzer, I., Liddelow, S. A., Saunders, N. R., Dziegielewska, K. M., Strazielle, N., and Ghersi-Egea, J. F. (2013). Developmental changes in the transcriptome of the rat choroid plexus in relation to neuroprotection. Fluids Barriers CNS 10 (1), 25. doi:10.1186/2045-8118-10-25
Krishnan, A. V., Stathis, P., Permuth, S. F., Tokes, L., and Feldman, D. (1993). Bisphenol-A: An estrogenic substance is released from polycarbonate flasks during autoclaving. Endocrinology 132 (6), 2279–2286. doi:10.1210/endo.132.6.8504731
Landrigan, P. J., Fuller, R., Fisher, S., Suk, W. A., Sly, P., Chiles, T. C., et al. (2019). Pollution and children's health. Sci. Total Environ. 650 (2), 2389–2394. doi:10.1016/j.scitotenv.2018.09.375
Landrigan, P. J., and Goldman, L. R. (2011). Children's vulnerability to toxic chemicals: A challenge and opportunity to strengthen health and environmental policy. Health Aff. (Millwood) 30 (5), 842–850. doi:10.1377/hlthaff.2011.0151
Lau, C., Thibodeaux, J. R., Das, K., Ehresman, D. J., Tanaka, S., Froehlich, J., et al. (2006). “Evaluation of perfluorooctane sulfonate in the rat brain,” in Society of toxicology (San Diego, California.
Lauwers, F., Cassot, F., Lauwers-Cances, V., Puwanarajah, P., and Duvernoy, H. (2008). Morphometry of the human cerebral cortex microcirculation: General characteristics and space-related profiles. Neuroimage 39 (3), 936–948. doi:10.1016/j.neuroimage.2007.09.024
Liddelow, S. A., Temple, S., Møllgård, K., Gehwolf, R., Wagner, A., Bauer, H., et al. (2012). Molecular characterisation of transport mechanisms at the developing mouse blood-CSF interface: A transcriptome approach. PLoS One 7 (3), e33554. doi:10.1371/journal.pone.0033554
Lin, J. H. (2008). CSF as a surrogate for assessing CNS exposure: An industrial perspective. Curr. Drug Metab. 9 (1), 46–59. doi:10.2174/138920008783331077
Liu, J., Li, J., Wu, Y., Zhao, Y., Luo, F., Li, S., et al. (2017). Bisphenol A metabolites and bisphenol S in paired maternal and cord serum. Environ. Sci. Technol. 51 (4), 2456–2463. doi:10.1021/acs.est.6b05718
Liu, L., Liu, W., Song, J., Yu, H., Jin, Y., Oami, K., et al. (2009). A comparative study on oxidative damage and distributions of perfluorooctane sulfonate (PFOS) in mice at different postnatal developmental stages. J. Toxicol. Sci. 34 (3), 245–254. doi:10.2131/jts.34.245
Liu, Y., Zhou, X., Wu, Y., Yang, X., Wang, Y., Li, S., et al. (2022). Exposure and blood–cerebrospinal fluid barrier permeability of PFASs in neonates. Environ. Sci. Technol. Lett. 9 (1), 64–70. doi:10.1021/acs.estlett.1c00862
Loscher, W., and Potschka, H. (2005). Drug resistance in brain diseases and the role of drug efflux transporters. Nat. Rev. Neurosci. 6 (8), 591–602. doi:10.1038/nrn1728
Lun, M. P., Monuki, E. S., and Lehtinen, M. K. (2015). Development and functions of the choroid plexus-cerebrospinal fluid system. Nat. Rev. Neurosci. 16 (8), 445–457. doi:10.1038/nrn3921
Macon, M. B., Villanueva, L. R., Tatum-Gibbs, K., Zehr, R. D., Strynar, M. J., Stanko, J. P., et al. (2011). Prenatal perfluorooctanoic acid exposure in CD-1 mice: Low-dose developmental effects and internal dosimetry. Toxicol. Sci. 122 (1), 134–145. doi:10.1093/toxsci/kfr076
Maestri, L., Negri, S., Ferrari, M., Ghittori, S., Fabris, F., Danesino, P., et al. (2006). Determination of perfluorooctanoic acid and perfluorooctanesulfonate in human tissues by liquid chromatography/single quadrupole mass spectrometry. Rapid Commun. Mass Spectrom. 20 (18), 2728–2734. doi:10.1002/rcm.2661
Mamsen, L. S., Bjorvang, R. D., Mucs, D., Vinnars, M. T., Papadogiannakis, N., Lindh, C. H., et al. (2019). Concentrations of perfluoroalkyl substances (PFASs) in human embryonic and fetal organs from first, second, and third trimester pregnancies. Environ. Int. 124, 482–492. doi:10.1016/j.envint.2019.01.010
Mariussen, E. (2012). Neurotoxic effects of perfluoroalkylated compounds: Mechanisms of action and environmental relevance. Arch. Toxicol. 86 (9), 1349–1367. doi:10.1007/s00204-012-0822-6
Mazur, C. S., Marchitti, S. A., Dimova, M., Kenneke, J. F., Lumen, A., and Fisher, J. (2012). Human and rat ABC transporter efflux of bisphenol a and bisphenol a glucuronide: Interspecies comparison and implications for pharmacokinetic assessment. Toxicol. Sci. 128 (2), 317–325. doi:10.1093/toxsci/kfs167
McCaffrey, G., and Davis, T. P. (2012). Physiology and pathophysiology of the blood-brain barrier: P-Glycoprotein and occludin trafficking as therapeutic targets to optimize central nervous system drug delivery. J. Investig. Med. 60 (8), 1131–1140. doi:10.2310/JIM.0b013e318276de79
Medin, T., Medin, H., Hefte, M. B., Storm-Mathisen, J., and Bergersen, L. H. (2019). Upregulation of the lactate transporter monocarboxylate transporter 1 at the blood-brain barrier in a rat model of attention-deficit/hyperactivity disorder suggests hyperactivity could be a form of self-treatment. Behav. Brain Res. 360, 279–285. doi:10.1016/j.bbr.2018.12.023
Millen, J. W., and Hess, A. (1958). The blood-brain barrier: An experimental study with vital dyes. Brain 81 (2), 248–257. doi:10.1093/brain/81.2.248
Mita, L., Baldi, A., Diano, N., Viggiano, E., Portaccio, M., Nicolucci, C., et al. (2012). Differential accumulation of BPA in some tissues of offspring of Balb-C mice exposed to different BPA doses. Environ. Toxicol. Pharmacol. 33 (1), 9–15. doi:10.1016/j.etap.2011.09.008
Møllgård, K., Balslev, Y., Lauritzen, B., and Saunders, N. R. (1987). Cell junctions and membrane specializations in the ventricular zone (germinal matrix) of the developing sheep brain: A CSF-brain barrier. J. Neurocytol. 16 (4), 433–444. doi:10.1007/bf01668498
Møllgård, K., Dziegielewska, K. M., Holst, C. B., Habgood, M. D., and Saunders, N. R. (2017). Brain barriers and functional interfaces with sequential appearance of ABC efflux transporters during human development. Sci. Rep. 7 (1), 11603. doi:10.1038/s41598-017-11596-0
Møllgård, K., and Saunders, N. R. (1975). Complex tight junctions of epithelial and of endothelial cells in early foetal brain. J. Neurocytol. 4 (4), 453–468. doi:10.1007/bf01261375
Møllgård, K., and Saunders, N. R. (1986). The development of the human blood-brain and blood-CSF barriers. Neuropathol. Appl. Neurobiol. 12 (4), 337–358. doi:10.1111/j.1365-2990.1986.tb00146.x
Moos, T., and Møllgård, K. (1993). Cerebrovascular permeability to azo dyes and plasma proteins in rodents of different ages. Neuropathol. Appl. Neurobiol. 19 (2), 120–127. doi:10.1111/j.1365-2990.1993.tb00416.x
Morita, K., Sasaki, H., Furuse, M., and Tsukita, S. (1999). Endothelial claudin: claudin-5/TMVCF constitutes tight junction strands in endothelial cells. J. Cell Biol. 147 (1), 185–194. doi:10.1083/jcb.147.1.185
Morris, M. E., Rodriguez-Cruz, V., and Felmlee, M. A. (2017). SLC and ABC transporters: Expression, localization, and species differences at the blood-brain and the blood-cerebrospinal fluid barriers. AAPS J. 19 (5), 1317–1331. doi:10.1208/s12248-017-0110-8
Mustieles, V., and Fernandez, M. F. (2020). Bisphenol A shapes children's brain and behavior: Towards an integrated neurotoxicity assessment including human data. Environ. Health 19 (1), 66. doi:10.1186/s12940-020-00620-y
Mustieles, V., Perez-Lobato, R., Olea, N., and Fernandez, M. F. (2015). Bisphenol A: Human exposure and neurobehavior. Neurotoxicology 49, 174–184. doi:10.1016/j.neuro.2015.06.002
Myburgh, E., Coles, J. A., Ritchie, R., Kennedy, P. G., McLatchie, A. P., Rodgers, J., et al. (2013). In vivo imaging of trypanosome-brain interactions and development of a rapid screening test for drugs against CNS stage trypanosomiasis. PLoS Negl. Trop. Dis. 7 (8), e2384. doi:10.1371/journal.pntd.0002384
Najjar, S., Pahlajani, S., De Sanctis, V., Stern, J. N. H., Najjar, A., and Chong, D. (2017). Neurovascular unit dysfunction and blood-brain barrier hyperpermeability contribute to schizophrenia neurobiology: A theoretical integration of clinical and experimental evidence. Front. Psychiatry 8, 83. doi:10.3389/fpsyt.2017.00083
Negri-Cesi, P. (2015). Bisphenol A interaction with brain development and functions. Dose Response 13 (2), 1559325815590394. doi:10.1177/1559325815590394
Neuwelt, E. A., Bauer, B., Fahlke, C., Fricker, G., Iadecola, C., Janigro, D., et al. (2011). Engaging neuroscience to advance translational research in brain barrier biology. Nat. Rev. Neurosci. 12 (3), 169–182. doi:10.1038/nrn2995
Nickel, S., and Mahringer, A. (2014). The xenoestrogens ethinylestradiol and bisphenol A regulate BCRP at the blood-brain barrier of rats. Xenobiotica 44 (11), 1046–1054. doi:10.3109/00498254.2014.922226
Nishikawa, M., Iwano, H., Yanagisawa, R., Koike, N., Inoue, H., and Yokota, H. (2010). Placental transfer of conjugated bisphenol A and subsequent reactivation in the rat fetus. Environ. Health Perspect. 118 (9), 1196–1203. doi:10.1289/ehp.0901575
Nitta, T., Hata, M., Gotoh, S., Seo, Y., Sasaki, H., Hashimoto, N., et al. (2003). Size-selective loosening of the blood-brain barrier in claudin-5-deficient mice. J. Cell Biol. 161 (3), 653–660. doi:10.1083/jcb.200302070
Nunez, A. A., Kannan, K., Giesy, J. P., Fang, J., and Clemens, L. G. (2001). Effects of Bisphenol A on energy balance and accumulation in Brown adipose tissue in rats. Chemosphere 42 (8), 917–922. doi:10.1016/s0045-6535(00)00196-x
O'Shaughnessy, K. L., Fischer, F., and Zenclussen, A. C. (2021). Perinatal exposure to endocrine disrupting chemicals and neurodevelopment: How articles of daily use influence the development of our children. Best. Pract. Res. Clin. Endocrinol. Metab. 35 (5), 101568. doi:10.1016/j.beem.2021.101568
Ohtsuki, S., Sato, S., Yamaguchi, H., Kamoi, M., Asashima, T., and Terasaki, T. (2007). Exogenous expression of claudin-5 induces barrier properties in cultured rat brain capillary endothelial cells. J. Cell Physiol. 210 (1), 81–86. doi:10.1002/jcp.20823
Onishchenko, N., Fischer, C., Wan Ibrahim, W. N., Negri, S., Spulber, S., Cottica, D., et al. (2011). Prenatal exposure to PFOS or PFOA alters motor function in mice in a sex-related manner. Neurotox. Res. 19 (3), 452–461. doi:10.1007/s12640-010-9200-4
Oshio, K., Watanabe, H., Song, Y., Verkman, A. S., and Manley, G. T. (2005). Reduced cerebrospinal fluid production and intracranial pressure in mice lacking choroid plexus water channel Aquaporin-1. FASEB J. 19 (1), 76–78. doi:10.1096/fj.04-1711fje
Pan, Y., Deng, M., Li, J., Du, B., Lan, S., Liang, X., et al. (2020). Occurrence and maternal transfer of multiple bisphenols, including an emerging derivative with unexpectedly high concentrations, in the human maternal-fetal-placental unit. Environ. Sci. Technol. 54 (6), 3476–3486. doi:10.1021/acs.est.0c00206
Pardridge, W. M. (2007). Blood-brain barrier delivery. Drug Discov. Today 12 (1-2), 54–61. doi:10.1016/j.drudis.2006.10.013
Pardridge, W. M. (2016). CSF, blood-brain barrier, and brain drug delivery. Expert Opin. Drug Deliv. 13 (7), 963–975. doi:10.1517/17425247.2016.1171315
Pardridge, W. M. (2006). Molecular Trojan horses for blood-brain barrier drug delivery. Discov. Med. 6 (34), 139–143.
Patisaul, H. B. (2020). Achieving CLARITY on bisphenol A, brain and behaviour. J. Neuroendocrinol. 32 (1), e12730. doi:10.1111/jne.12730
Patterson, T. A., Twaddle, N. C., Roegge, C. S., Callicott, R. J., Fisher, J. W., and Doerge, D. R. (2013). Concurrent determination of bisphenol A pharmacokinetics in maternal and fetal rhesus monkeys. Toxicol. Appl. Pharmacol. 267 (1), 41–48. doi:10.1016/j.taap.2012.12.006
Paulson, O. B., and Newman, E. A. (1987). Does the release of potassium from astrocyte endfeet regulate cerebral blood flow? Science 237 (4817), 896–898. doi:10.1126/science.3616619
Pelch, K. E., Wignall, J. A., Goldstone, A. E., Ross, P. K., Blain, R. B., Shapiro, A. J., et al. (2017). NTP research report on biological activity of bisphenol A (BPA) structural analogues and functional alternatives: Research report 4. Research Triangle Park, NC: National Toxicology Program.
Pelch, K., Wignall, J. A., Goldstone, A. E., Ross, P. K., Blain, R. B., Shapiro, A. J., et al. (2019). A scoping review of the health and toxicological activity of bisphenol A (BPA) structural analogues and functional alternatives. Toxicology 424, 152235. doi:10.1016/j.tox.2019.06.006
Penta, P. (1932). Sulla colorazione vitale del sistema nervosa negli centrale animali neonati. Riv. Neurol. 5, 62–80.
Perez, F., Nadal, M., Navarro-Ortega, A., Fabrega, F., Domingo, J. L., Barcelo, D., et al. (2013). Accumulation of perfluoroalkyl substances in human tissues. Environ. Int. 59, 354–362. doi:10.1016/j.envint.2013.06.004
Piekarski, D. J., Diaz, K. R., and McNerney, M. W. (2020). Perfluoroalkyl chemicals in neurological health and disease: Human concerns and animal models. Neurotoxicology 77, 155–168. doi:10.1016/j.neuro.2020.01.001
Pizzurro, D. M., Seeley, M., Kerper, L. E., and Beck, B. D. (2019). Interspecies differences in perfluoroalkyl substances (PFAS) toxicokinetics and application to health-based criteria. Regul. Toxicol. Pharmacol. 106, 239–250. doi:10.1016/j.yrtph.2019.05.008
Pollak, T. A., Drndarski, S., Stone, J. M., David, A. S., McGuire, P., and Abbott, N. J. (2018). The blood–brain barrier in psychosis. Lancet Psychiatry 5 (1), 79–92. doi:10.1016/s2215-0366(17)30293-6
Qin, Y., and Sato, T. N. (1995). Mouse multidrug resistance 1a/3 gene is the earliest known endothelial cell differentiation marker during blood-brain barrier development. Dev. Dyn. 202 (2), 172–180. doi:10.1002/aja.1002020209
Rayasam, S. D. G., Koman, P. D., Axelrad, D. A., Woodruff, T. J., and Chartres, N. (2022). Toxic substances control act (TSCA) implementation: How the amended law has failed to protect vulnerable populations from toxic chemicals in the United States. Environ. Sci. Technol. doi:10.1021/acs.est.2c02079
Raybaud, C. (2010). Normal and abnormal embryology and development of the intracranial vascular system. Neurosurg. Clin. N. Am. 21 (3), 399–426. doi:10.1016/j.nec.2010.03.011
Redzic, Z. (2011). Molecular biology of the blood-brain and the blood-cerebrospinal fluid barriers: Similarities and differences. Fluids Barriers CNS 8 (1), 3. doi:10.1186/2045-8118-8-3
Reichel, A. (2015). “Pharmacokinetics of CNS penetration,” in Blood-brain barrier in drug discovery: Optimizing brain exposure of CNS drugs and minimizing brain side effects for peripheral drugs. Editors L. D. Kerns, and H. E. (Hoboken, New Jersey: John Wiley & Sons), 7–41.
Renz, L., Volz, C., Michanowicz, D., Ferrar, K., Christian, C., Lenzner, D., et al. (2013). A study of parabens and bisphenol A in surface water and fish brain tissue from the Greater Pittsburgh Area. Ecotoxicology 22 (4), 632–641. doi:10.1007/s10646-013-1054-0
Roberts, D. J., and Goralski, K. B. (2008). A critical overview of the influence of inflammation and infection on P-glycoprotein expression and activity in the brain. Expert Opin. Drug Metab. Toxicol. 4 (10), 1245–1264. doi:10.1517/17425255.4.10.1245
Rock, K. D., and Patisaul, H. B. (2018). Environmental mechanisms of neurodevelopmental toxicity. Curr. Environ. Health Rep. 5 (1), 145–157. doi:10.1007/s40572-018-0185-0
Ros, O., Vallejo, A., Olivares, M., Etxebarria, N., and Prieto, A. (2016). Determination of endocrine disrupting compounds in fish liver, brain, and muscle using focused ultrasound solid-liquid extraction and dispersive solid phase extraction as clean-up strategy. Anal. Bioanal. Chem. 408 (21), 5689–5700. doi:10.1007/s00216-016-9697-3
Saili, K. S., Zurlinden, T. J., Schwab, A. J., Silvin, A., Baker, N. C., Hunter, E. S., et al. (2017). Blood-brain barrier development: Systems modeling and predictive toxicology. Birth Defects Res. 109 (20), 1680–1710. doi:10.1002/bdr2.1180
Saitou, M., Furuse, M., Sasaki, H., Schulzke, J. D., Fromm, M., Takano, H., et al. (2000). Complex phenotype of mice lacking occludin, a component of tight junction strands. Mol. Biol. Cell 11 (12), 4131–4142. doi:10.1091/mbc.11.12.4131
Sakka, L., Coll, G., and Chazal, J. (2011). Anatomy and physiology of cerebrospinal fluid. Eur. Ann. Otorhinolaryngology, Head Neck Dis. 128 (6), 309–316. doi:10.1016/j.anorl.2011.03.002
Santoro, A., Chianese, R., Troisi, J., Richards, S., Nori, S. L., Fasano, S., et al. (2019). Neuro-toxic and reproductive effects of BPA. Curr. Neuropharmacol. 17 (12), 1109–1132. doi:10.2174/1570159x17666190726112101
Sarin, H. (2010). Physiologic upper limits of pore size of different blood capillary types and another perspective on the dual pore theory of microvascular permeability. J. Angiogenes Res. 2, 14. doi:10.1186/2040-2384-2-14
Sasso, A. F., Pirow, R., Andra, S. S., Church, R., Nachman, R. M., Linke, S., et al. (2020). Pharmacokinetics of bisphenol A in humans following dermal administration. Environ. Int. 144, 106031. doi:10.1016/j.envint.2020.106031
Saunders, N. R., Dreifuss, J. J., Dziegielewska, K. M., Johansson, P. A., Habgood, M. D., Møllgård, K., et al. (2014). The rights and wrongs of blood-brain barrier permeability studies: A walk through 100 years of history. Front. Neurosci. 8, 404. doi:10.3389/fnins.2014.00404
Saunders, N. R., Dziegielewska, K. M., Møllgård, K., and Habgood, M. D. (2015). Markers for blood-brain barrier integrity: How appropriate is evans blue in the twenty-first century and what are the alternatives? Front. Neurosci. 9, 385. doi:10.3389/fnins.2015.00385
Saunders, N. R., Dziegielewska, K. M., Møllgård, K., and Habgood, M. D. (2018). Physiology and molecular biology of barrier mechanisms in the fetal and neonatal brain. J. Physiol. 596 (23), 5723–5756. doi:10.1113/jp275376
Saunders, N. R., Dziegielewska, K. M., Møllgård, K., and Habgood, M. D. (2019). Recent developments in understanding barrier mechanisms in the developing brain: Drugs and drug transporters in pregnancy, susceptibility or protection in the fetal brain? Annu. Rev. Pharmacol. Toxicol. 59, 487–505. doi:10.1146/annurev-pharmtox-010818-021430
Shimoda, A. (1963). An electron microscope study of the developing rat brain, concerned with the morphological basis of the blood-brain barrier. Acta Pathol. Jpn. 13, 95–105. doi:10.1111/j.1440-1827.1963.tb01913.x
Shin, B. S., Kim, C. H., Jun, Y. S., Kim, D. H., Lee, B. M., Yoon, C. H., et al. (2004). Physiologically based pharmacokinetics of bisphenol A. J. Toxicol. Environ. Health A 67 (23-24), 1971–1985. doi:10.1080/15287390490514615
Solár, P., Zamani, A., Kubíčková, L., Dubovy, P., and Joukal, M. (2020). Choroid plexus and the blood-cerebrospinal fluid barrier in disease. Fluids Barriers CNS 17 (1), 35. doi:10.1186/s12987-020-00196-2
Stahlhut, R. W., Welshons, W. V., and Swan, S. H. (2009). Bisphenol A data in NHANES suggest longer than expected half-life, substantial nonfood exposure, or both. Environ. Health Perspect. 117 (5), 784–789. doi:10.1289/ehp.0800376
Stanimirovic, D. B., and Friedman, A. (2012). Pathophysiology of the neurovascular unit: Disease cause or consequence? J. Cereb. Blood Flow. Metab. 32 (7), 1207–1221. doi:10.1038/jcbfm.2012.25
Stern, L., Gautier, R., and Raymo, C. R. (1918). Passage simultané des substances dans le liquide céphalo-rachidien et dans les centres nerveux. . d. Ia Soc. de Phys. d'hist. natur. de Genève 35, 58–60.
Stern, L., and Rapoport, J. L. (1928). Les rapports entre l'augmentation de la perméabilité de la barrière hémato-encéphalique et les altérations de son substratum morphologique. C. R. Soc. Biol. 98, 1515–1517.
Stern, L., Rapoport, J. L., and Lokschina, E. S. (1929). Le fonctionnement de la barrière hémato-encéphalique chez les nouveau nés. C. R. Soc. Biol. 100, 231–233.
Stiles, J., and Jernigan, T. L. (2010). The basics of brain development. Neuropsychol. Rev. 20 (4), 327–348. doi:10.1007/s11065-010-9148-4
Stolp, H. B., Liddelow, S. A., Sa-Pereira, I., Dziegielewska, K. M., and Saunders, N. R. (2013). Immune responses at brain barriers and implications for brain development and neurological function in later life. Front. Integr. Neurosci. 7, 61. doi:10.3389/fnint.2013.00061
Stolp, H. B., Turnquist, C., Dziegielewska, K. M., Saunders, N. R., Anthony, D. C., and Molnar, Z. (2011). Reduced ventricular proliferation in the foetal cortex following maternal inflammation in the mouse. Brain 134 (11), 3236–3248. doi:10.1093/brain/awr237
Stonestreet, B. S., Sadowska, G. B., Leeman, J., Hanumara, R. C., Petersson, K. H., and Patlak, C. S. (2006). Effects of acute hyperosmolality on blood-brain barrier function in ovine fetuses and lambs. Am. J. Physiol. Regul. Integr. Comp. Physiol. 291 (4), R1031–R1039. doi:10.1152/ajpregu.00883.2005
Strazielle, N., and Ghersi-Egea, J. F. (2016). Potential pathways for CNS drug delivery across the blood-cerebrospinal fluid barrier. Curr. Pharm. Des. 22 (35), 5463–5476. doi:10.2174/1381612822666160726112115
Sturrock, R. R. (1979). A morphological study of the development of the mouse choroid plexus. J. Anat. 129 (4), 777–793.
Tachikawa, M., Uchida, Y., Ohtsuki, S., and Terasaki, T. (2014). “Recent progress in blood–brain barrier and blood–CSF barrier transport research: Pharmaceutical relevance for drug delivery to the brain,” in Drug delivery to the brain. Editor D. L. E. T. R. M. Hammarlund-Udenaes (New York, NY: Springer), 23–62.
Tachikawa, M., Watanabe, M., Hori, S., Fukaya, M., Ohtsuki, S., Asashima, T., et al. (2005). Distinct spatio-temporal expression of ABCA and ABCG transporters in the developing and adult mouse brain. J. Neurochem. 95 (1), 294–304. doi:10.1111/j.1471-4159.2005.03369.x
Takano, T., Tian, G. F., Peng, W., Lou, N., Libionka, W., Han, X., et al. (2006). Astrocyte-mediated control of cerebral blood flow. Nat. Neurosci. 9 (2), 260–267. doi:10.1038/nn1623
Tauc, M., Vignon, X., and Bouchaud, C. (1984). Evidence for the effectiveness of the blood—CSF barrier in the fetal rat choroid plexus. A freeze-fracture and peroxidase diffusion study. Tissue Cell 16 (1), 65–74. doi:10.1016/0040-8166(84)90019-3
Taves, D. R. (1968). Evidence that there are two forms of fluoride in human serum. Nature 217 (5133), 1050–1051. doi:10.1038/2171050b0
Teeguarden, J. G., Twaddle, N. C., Churchwell, M. I., Yang, X., Fisher, J. W., Seryak, L. M., et al. (2015). 24-hour human urine and serum profiles of bisphenol A: Evidence against sublingual absorption following ingestion in soup. Toxicol. Appl. Pharmacol. 288 (2), 131–142. doi:10.1016/j.taap.2015.01.009
Tennyson, V. M., and Pappas, G. D. (1962). An electron microscope study of ependymal cells of the fetal, early postnatal and adult rabbit. Z. Zellforsch. 56, 595–618. doi:10.1007/bf00540584
Thayer, K. A., Doerge, D. R., Hunt, D., Schurman, S. H., Twaddle, N. C., Churchwell, M. I., et al. (2015). Pharmacokinetics of bisphenol A in humans following a single oral administration. Environ. Int. 83, 107–115. doi:10.1016/j.envint.2015.06.008
Thomsen, M. S., Routhe, L. J., and Moos, T. (2017). The vascular basement membrane in the healthy and pathological brain. J. Cereb. Blood Flow. Metab. 37 (10), 3300–3317. doi:10.1177/0271678x17722436
Timpl, R. (1989). Structure and biological activity of basement membrane proteins. Eur. J. Biochem. 180 (3), 487–502. doi:10.1111/j.1432-1033.1989.tb14673.x
Tong, L., Hill, R. A., Damisah, E. C., Murray, K. N., Yuan, P., Bordey, A., et al. (2020). Imaging and optogenetic modulation of vascular mural cells in the live brain. Nat. Protoc. doi:10.1038/s41596-020-00425-w
Tsukita, S., Furuse, M., and Itoh, M. (2001). Multifunctional strands in tight junctions. Nat. Rev. Mol. Cell Biol. 2 (4), 285–293. doi:10.1038/35067088
Tsukita, S., Tanaka, H., and Tamura, A. (2019). The claudins: From tight junctions to biological systems. Trends Biochem. Sci. 44 (2), 141–152. doi:10.1016/j.tibs.2018.09.008
Uchida, K., Suzuki, A., Kobayashi, Y., Buchanan, D. L., Sato, T., Watanabe, H., et al. (2002). Bisphenol-A administration during pregnancy results in fetal exposure in mice and monkeys. J. Health Sci. 48 (6), 579–582. doi:10.1248/jhs.48.579
Van de Vijver, K. I., Hoff, P., Das, K., Brasseur, S., Van Dongen, W., Esmans, E., et al. (2005). Tissue distribution of perfluorinated chemicals in harbor seals (Phoca vitulina) from the Dutch Wadden Sea. Environ. Sci. Technol. 39 (18), 6978–6984. doi:10.1021/es050942+
van der Meer, T. P., Artacho-Cordon, F., Swaab, D. F., Struik, D., Makris, K. C., Wolffenbuttel, B. H. R., et al. (2017). Distribution of non-persistent endocrine disruptors in two different regions of the human brain. Int. J. Environ. Res. Public Health 14 (9). doi:10.3390/ijerph14091059
Vandenberg, L. N., Hauser, R., Marcus, M., Olea, N., and Welshons, W. V. (2007). Human exposure to bisphenol A (BPA). Reprod. Toxicol. 24 (2), 139–177. doi:10.1016/j.reprotox.2007.07.010
Vandenberg, L. N., Hunt, P. A., Myers, J. P., and Vom Saal, F. S. (2013). Human exposures to bisphenol A: Mismatches between data and assumptions. Rev. Environ. Health 28 (1), 37–58. doi:10.1515/reveh-2012-0034
Virgintino, D., Errede, M., Robertson, D., Capobianco, C., Girolamo, F., Vimercati, A., et al. (2004). Immunolocalization of tight junction proteins in the adult and developing human brain. Histochem Cell Biol. 122 (1), 51–59. doi:10.1007/s00418-004-0665-1
Volkel, W., Bittner, N., and Dekant, W. (2005). Quantitation of bisphenol A and bisphenol A glucuronide in biological samples by high performance liquid chromatography-tandem mass spectrometry. Drug Metab. Dispos. 33 (11), 1748–1757. doi:10.1124/dmd.105.005454
Volkel, W., Colnot, T., Csanady, G. A., Filser, J. G., and Dekant, W. (2002). Metabolism and kinetics of bisphenol a in humans at low doses following oral administration. Chem. Res. Toxicol. 15 (10), 1281–1287. doi:10.1021/tx025548t
Vom Saal, F. S., and Vandenberg, L. N. (2021). Update on the health effects of bisphenol A: Overwhelming evidence of harm. Endocrinology 162 (3). doi:10.1210/endocr/bqaa171
Wang, F., Liu, W., Jin, Y., Dai, J., Yu, W., Liu, X., et al. (2010). Transcriptional effects of prenatal and neonatal exposure to PFOS in developing rat brain. Environ. Sci. Technol. 44 (5), 1847–1853. doi:10.1021/es902799f
Wang, H., Chang, L., Aguilar, J. S., Dong, S., and Hong, Y. (2019). Bisphenol-A exposure induced neurotoxicity in glutamatergic neurons derived from human embryonic stem cells. Environ. Int. 127, 324–332. doi:10.1016/j.envint.2019.01.059
Wang, J., Pan, Y., Cui, Q., Yao, B., Wang, J., and Dai, J. (2018). Penetration of PFASs across the blood cerebrospinal fluid barrier and its determinants in humans. Environ. Sci. Technol. 52 (22), 13553–13561. doi:10.1021/acs.est.8b04550
Weed, L. H. (1917). “The development of the cerebrospinal fluid spaces in pig and in man,” in Contributions to embryology (Washington: Carnegie Institution of Washington), 3–116.
Welch, C., and Mulligan, K. (2022). Does bisphenol A confer risk of neurodevelopmental disorders? What we have learned from developmental neurotoxicity studies in animal models. Int. J. Mol. Sci. 23 (5). doi:10.3390/ijms23052894
Whish, S., Dziegielewska, K. M., Møllgård, K., Noor, N. M., Liddelow, S. A., Habgood, M. D., et al. (2015). The inner CSF-brain barrier: Developmentally controlled access to the brain via intercellular junctions. Front. Neurosci. 9, 16. doi:10.3389/fnins.2015.00016
Winkler, E. A., Bell, R. D., and Zlokovic, B. V. (2011). Central nervous system pericytes in health and disease. Nat. Neurosci. 14 (11), 1398–1405. doi:10.1038/nn.2946
Wislocki, G. B. (1920). “Experimental studies on fetal absorption. I. The vitality stained fetus,” in Contributions to embryology (Washington: Carnegie Institution of Washington), 45–60.
Wolburg, H., Noell, S., Wolburg-Buchholz, K., Mack, A., and Fallier-Becker, P. (2009). Agrin, aquaporin-4, and astrocyte polarity as an important feature of the blood-brain barrier. Neuroscientist 15 (2), 180–193. doi:10.1177/1073858408329509
Wolburg, H., Wolburg-Buchholz, K., Liebner, S., and Engelhardt, B. (2001). Claudin-1, claudin-2 and claudin-11 are present in tight junctions of choroid plexus epithelium of the mouse. Neurosci. Lett. 307 (2), 77–80. doi:10.1016/s0304-3940(01)01927-9
Wong, A. D., Ye, M., Levy, A. F., Rothstein, J. D., Bergles, D. E., and Searson, P. C. (2013). The blood-brain barrier: An engineering perspective. Front. Neuroeng 6, 7. doi:10.3389/fneng.2013.00007
Yoo, S. D., Shin, B. S., Kwack, S. J., Lee, B. M., Park, K. L., Han, S. Y., et al. (2000). Pharmacokinetic disposition and tissue distribution of bisphenol A in rats after intravenous administration. J. Toxicol. Environ. Health A 61 (2), 131–139. doi:10.1080/00984100050120415
Yu, Y., Wang, C., Zhang, X., Zhu, J., Wang, L., Ji, M., et al. (2020). Perfluorooctane sulfonate disrupts the blood brain barrier through the crosstalk between endothelial cells and astrocytes in mice. Environ. Pollut. 256, 113429. doi:10.1016/j.envpol.2019.113429
Zablotsky, B., Black, L. I., Maenner, M. J., Schieve, L. A., Danielson, M. L., Bitsko, R. H., et al. (2019). Prevalence and trends of developmental disabilities among children in the United States: 2009-2017. Pediatrics 144 (4). doi:10.1542/peds.2019-0811
Zeng, H. C., Zhang, L., Li, Y. Y., Wang, Y. J., Xia, W., Lin, Y., et al. (2011). Inflammation-like glial response in rat brain induced by prenatal PFOS exposure. Neurotoxicology 32 (1), 130–139. doi:10.1016/j.neuro.2010.10.001
Zhang, X., Yin, X., Zhang, J., Li, A., Gong, H., Luo, Q., et al. (2019). High-resolution mapping of brain vasculature and its impairment in the hippocampus of Alzheimer's disease mice. Natl. Sci. Rev. 6 (6), 1223–1238. doi:10.1093/nsr/nwz124
Zhao, Y., Xin, Y., He, Z., and Hu, W. (2018). Function of connexins in the interaction between glial and vascular cells in the central nervous system and related neurological diseases. Neural Plast. 2018, 6323901. doi:10.1155/2018/6323901
Zheng, W., Aschner, M., and Ghersi-Egea, J. F. (2003). Brain barrier systems: A new frontier in metal neurotoxicological research. Toxicol. Appl. Pharmacol. 192 (1), 1–11. doi:10.1016/s0041-008x(03)00251-5
Zheng, W., and Ghersi-Egea, J. F. (2020). Brain barrier systems play No small roles in toxicant-induced brain disorders. Toxicol. Sci. 175 (2), 147–148. doi:10.1093/toxsci/kfaa053
Keywords: brain development, blood-brain barrier, blood-cerebrospinal fluid barrier, environmental contaminants, mechanisms of neurotoxicity, perfluoroalkyl substances (PFAS), neurodevelopmental disorders, bisphenol
Citation: Bell KS and O’Shaughnessy KL (2022) The development and function of the brain barriers – an overlooked consideration for chemical toxicity. Front.Toxicol. 4:1000212. doi: 10.3389/ftox.2022.1000212
Received: 21 July 2022; Accepted: 08 September 2022;
Published: 18 October 2022.
Edited by:
Timothy J Shafer, United States Environmental Protection Agency (EPA), United StatesReviewed by:
Juzaili Azizi, Universiti Sains Malaysia (USM), MalaysiaGeertje Lewin, Consultant, Germany
Gianfranco Santovito, University of Padua, Italy
Copyright © 2022 Bell and O’Shaughnessy. This is an open-access article distributed under the terms of the Creative Commons Attribution License (CC BY). The use, distribution or reproduction in other forums is permitted, provided the original author(s) and the copyright owner(s) are credited and that the original publication in this journal is cited, in accordance with accepted academic practice. No use, distribution or reproduction is permitted which does not comply with these terms.
*Correspondence: Katherine L. O’Shaughnessy, b3NoYXVnaG5lc3N5LmthdGllQGVwYS5nb3Y=