- 1Coordenação de Dinâmica Ambiental, Instituto Nacional de Pesquisas da Amazônia, Manaus, Brazil
- 2Geography, College of Life and Environmental Sciences, University of Exeter, Exeter, UK
- 3Laboratório de Ciência do Sistema Terrestre, Departamento de Ecologia, Universidade Estadual Paulista, Rio Claro, Brasil
- 4Land Surface-Atmosphere Interactions, Technical University Munich, Freising, Germany
- 5Earth System Analysis, Potsdam Institute for Climate Impact Research, Potsdam, Germany
The impacts of elevated atmospheric CO2 (eCO2) and alterations in nutrient availability on the carbon (C) storage capacity and resilience of the Amazon forest remain highly uncertain. Carbon dynamics are controlled by multiple eco-physiological processes responding to environmental change, but we lack solid experimental evidence, hampering theory development and thus representation in ecosystem models. Here, we present two ecosystem-scale manipulation experiments, to be carried out in the Amazon, that examine tropical ecosystem responses to eCO2 and alterations in nutrient availability and thus will elucidate the representation of crucial ecological processes by ecosystem models. We highlight current gaps in our understanding of tropical ecosystem responses to projected global changes in light of the eco-physiological assumptions considered by current ecosystem models. We conclude that a more detailed process-based representation of the spatial (e.g., soil type; plant functional type) and temporal (seasonal and inter-annual) variability of tropical forests is needed to enhance model predictions of ecosystem responses to projected global environmental change.
Introduction
Tropical forests account for approximately one fourth of the total global forest carbon (C) stock (Phillips et al., 2009; Pan et al., 2011; Carvalhais et al., 2014). The Amazon basin represents the largest continuous region of tropical forests, storing about 150–200 Pg C (Feldpausch et al., 2012) and accounting for 14% of C fixed by photosynthesis in the terrestrial biosphere (Zhao and Running, 2010). A decrease or cessation of the Amazon forest C sink could create strong positive feedbacks on global climate change. It is therefore of paramount importance to enhance the mechanistic understanding of potential feedbacks between tropical C storage and projected global environmental change (Zhou et al., 2013; Zuidema et al., 2013).
Amazon forests appear vulnerable to increasing moisture stress, with the potential for large C losses to exert feedbacks on climate change (Phillips et al., 2009). Global circulation models project an increase of droughts affecting the Amazon region (Malhi et al., 2008) due to increasing frequency of climate anomalies associated to increasing sea surface temperatures (Lewis et al., 2011), which could lead to loss of Amazon forest further accelerating climate change (Rammig et al., 2010). Efforts to quantify the response of aboveground carbon storage derived from forest inventory plots concluded that such events have the potential to reverse a multi-decadal biomass C sink across Amazonia (Phillips et al., 2009). However, to date predicted responses of the Amazon forest to projected global climate changes (mainly precipitation and temperature) remain largely speculative due to a lack of direct experimental evidence (Malhi et al., 2009; Davidson et al., 2012; Cernusak et al., 2013). As a surrogate, ecosystem models that include the mechanistic representation of key ecosystem processes, such as photosynthesis, respiration, growth, and C allocation, have been used for projections of ecosystem responses to changes in climate, atmospheric CO2 and nutrient availability (e.g., Goll et al., 2012; Huntingford et al., 2013; Joetzjer et al., 2014; Smith et al., 2014; Yang et al., 2014). However, due to differences in the representation of ecophysiological processes and their sensitivity to environmental stresses model simulations predict either dramatic Amazon forest dieback for the twenty first century due to changes in precipitation patterns (Cox et al., 2008) and rising temperatures (Cox et al., 2004), or simulate strong resilience of tropical forests due to a theoretically supposed CO2 fertilization effect on primary productivity (Cox et al., 2013; Huntingford et al., 2013). As a consequence, climate-driven loss of tropical forest biomass could potentially be mitigated by CO2 fertilization of forest productivity (Lapola et al., 2009; Rammig et al., 2010).
Nonetheless, lack of experimental evidence, in particular for the tropics, hinders the validation of current ecosystem models in predicting responses to increasing atmospheric CO2 concentrations and alterations in nutrient availability. In turn, one of the main limitations of state-of-the-art models for reliably projecting potential changes in the Amazon C pool is the uncertainty associated with critical underlying ecosystem processes such as the physiological response of tropical trees to eCO2 and nutrient limitation (Medlyn et al., 2015; Norby et al., 2016; Reed et al., 2015). So far, manipulative ecosystem experiments have predominantly been conducted in temperate and boreal regions. These experiments have greatly enhanced our understanding of forest ecosystem responses to eCO2 and increasing nutrient inputs from anthropogenic sources (e.g., Rastetter and Shaver, 1992; Reich et al., 2014; Norby et al., 2016). For instance, free-air CO2 enrichment (FACE) experiments in temperate areas indicate an initial increase in forest productivity (Norby et al., 2005), which is ultimately limited by soil nutrient availability (Norby et al., 2010). However, temperate and boreal regions are predominantly limited by soil nitrogen (N) availability, whereas lowland tropical forests are relatively rich in N, but characterized by highly weathered soils with low soil phosphorus (P) availability (Walker and Syers, 1976; Vitousek and Sanford, 1986; Quesada et al., 2012). Because of differences between tropical forests and temperate forests in biological complexity, nutrient cycling, and climate regimes, we expect differential responses of tropical forest ecosystems to eCO2 and alterations in nutrient availability.
Ecosystem scale experiments subjecting mature forests to eCO2 and nutrient addition in the tropics, and in particular the Amazon forest, are crucial for filling major gaps in our understanding of expected global change effects (Hickler et al., 2008; Cernusak et al., 2013; Norby et al., 2016). Two major endeavors are currently underway in Amazonia; the first free-air carbon enrichment experiment in a mature tropical old-growth forest (AmazonFACE; Lapola and Norby, 2014), as well as the first large-scale factorial nutrient addition experiment (AFEX; Amazon fertilization experiment). These experiments aim to assess the effects of eCO2 and alterations in nutrient availability on biogeochemistry, ecology and resilience of the Amazon forest. Based on an assumption-centered integrated model-experiment approach (Medlyn et al., 2015), the goal of this paper is to discuss the plausibility and uncertainties of three hypotheses related to potential responses of tropical ecosystem processes to eCO2 and alterations in nutrient availability: (1) Elevated CO2 will increase C source activity (i.e., assimilation) and thus could stimulate C sink activity (i.e., growth) (2) Low nutrient (phosphorus) availability of tropical soils will limit C sink activity but may be alleviated by C allocation belowground; (3) Shifts in plant C allocation will increase C turnover and thus might decrease C storage in tropical forests. We explore each of these hypotheses in relation to current model assumptions and observational evidence (Figure 1).
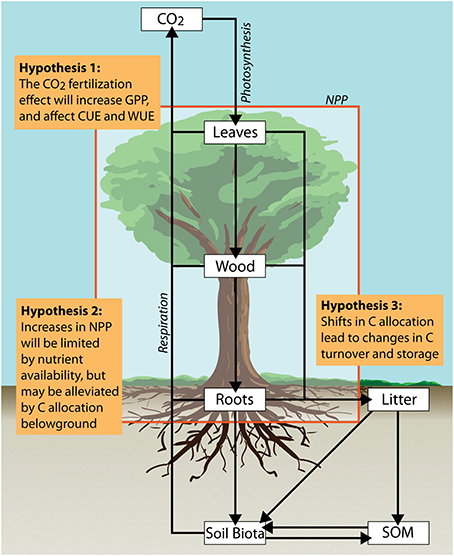
Figure 1. Simplified conceptual model depicting major pools and fluxes of carbon in tropical forests. Assumption-based hypotheses to be targeted by the proposed Amazonian long-term ecosystem experiments are discussed in the text.
Elevated CO2 will Increase C Source Activity (i.e., Assimilation) and Thus Could Stimulate C Sink Activity (i.e., Growth)
Current model formulations assume that tropical forests have the potential to respond more strongly to eCO2 compared to temperate and boreal forests, based on the eco-physiological understanding of photosynthesis that is represented by a scheme introduced by Farquhar et al. (1980). Therefore most ecosystem models incorporating the Farquhar scheme (Long, 1991; Drake et al., 1997; Hickler et al., 2008, 2015; Cernusak et al., 2013) suggest that eCO2 increases rates of leaf-level photosynthesis (Lloyd and Farquhar, 1996) based on the following simplified relationship:
where the rate of gross photosynthetic CO2 assimilation (A) is determined by the rate of carboxylation (Jc), and the potential rate of electron transport (Je) at a particular irradiance (JL). Several factors may limit the rate of carboxylation; i.e., the relative partial pressure of CO2 and O2 (ci), the amount of activated Rubisco enzyme, and the rate of acceptor regeneration (Farquhar et al., 1980). Due to the influence of ci and JL on the temperature (T) dependence of A, and the fact that under eCO2 carboxylation dominates over oxygenation and increases with T until limited by the maximum rate of Je, we might expect a stronger positive response of net photosynthesis to eCO2 in warmer environments, such as tropical forests (Lloyd and Farquhar, 2008).
However, because carbon gain and water loss are regulated by the rate of leaf stomatal closure, a downregulation of stomatal conductance due to high vapor pressure deficit has the potential to substantially reduce the eCO2-induced increase in leaf-level photosynthesis (Beedlow et al., 2008; Körner, 2009; Holtum and Winter, 2010). Based on the observed coupling between photosynthesis and stomatal conductance such effects are included in most ecosystem model formulations and therefore under eCO2, most models simulate an increase in water-use efficiency (WUE; De Kauwe et al., 2013) i.e., the rate of photosynthesis to transpiration. However, studies based on carbon isotope fractionation in tropical tree rings suggest that increasing WUE does not necessarily trigger increased tree growth (Nock et al., 2011) and thus found no stimulation of tree growth in response to CO2 fertilization (van der Sleen et al., 2014). This dilemma might result from the fact that under sub-optimal conditions enhanced “source activity” (i.e., assimilation) of leaves in response to eCO2 might not stimulate increased “sink activity” (i.e., growth) of tropical trees due to other factors limiting plant growth (Körner, 2003). Highlighting the interactive effects of instantaneous biogeochemical processes that have to be considered in global ecosystem models this illustrates why an eCO2-induced stimulation of photosynthetic C assimilation might not necessarily result in enhanced C storage of tropical forests in response to eCO2 (Körner, 2003).
Recent research indicates that during drought trees prioritize growth by reducing autotrophic respiration that is unrelated to growth instead of reducing total productivity (Doughty et al., 2015a). This indicates that projected global changes such as increasing drought frequency and mean annual temperatures influence the ratio of photosynthesis to respiration (Ra) and thus affect plant carbon use-efficiency (CUE) i.e., the rate of net primary production (NPP) to gross primary production (GPP; Gifford, 2003; DeLucia et al., 2007). Similarly, in process-based models the optimum temperature for maximum net primary production (where NPP = GPP−Ra) depends on the balance between temperature effects on photosynthesis and respiration. However, there are still large uncertainties to which extent these physiological processes can acclimate in response to projected global changes (Huntingford et al., 2013). Both photosynthesis and respiration are speculated to be capable of dynamic thermal acclimation as a long-term temperature response. For instance, when acclimation is not considered in ecosystem models this usually leads to widespread forest dieback compared to when photosynthetic temperature acclimation is accounted for (Sitch et al., 2008). However, acclimation is challenging to incorporate in models in a mechanistic way because different species appear to vary in their acclimation strategies (Medlyn et al., 2011). Thus, the overall effect of global changes on tropical forests represents the integration of simultaneous responses of ecophysiological processes, such as those discussed above but also on phenology, allocation, turnover, and decomposition. Ultimately, by integrating this mechanistic understanding based on empirical evidence from experimental studies in next generation ecosystem models we will be able to scale-up plot-level measurements to ecosystem-scale responses under sub-optimal conditions of resource availability.
Low Nutrient (Phosphorus) Availability of Tropical Soils will Limit C-sink Activity but may be Alleviated by C Allocation Belowground
Plants need a broad range of nutrients (N, P) and micronutrients in a certain stoichiometry to maintain NPP, growth and normal functioning (e.g., Liebig, 1840; Sterner and Elser, 2002; Körner, 2003). Hence, we expect that potential initial increases in NPP will be limited by low nutrient availability of tropical soils (Figure 1). In tropical forests N is mainly stored in biomass, and rapidly recycled from organic matter; and in addition to N deposition, symbiotic N2-fixing plants appear at a high abundance (Houlton et al., 2008; Hedin et al., 2009). Phosphorus inputs in contrast are mainly rock-derived; P availability decreases with weathering, and with increasing soil age it becomes gradually transformed and bound in recalcitrant complexes (e.g., Walker and Syers, 1976; Vitousek et al., 2010). Hence, in old and highly weathered Amazonian soils P rather than N appears to limit plant growth. Moreover, P availability seems to exert an important control on N cycling (Quesada et al., 2010; Vitousek et al., 2010), but our understanding of the nature of P limitations and N:P interactions is still expanding (Vitousek et al., 2010; Lambers et al., 2015). Ecosystem C-N models integrating above- and belowground interactions, and including constraints of plant stoichiometry strongly improved the representation of eCO2 responses of two temperate FACE experiments (Zaehle et al., 2014). There is growing recognition that P-dynamics and N:P interactions, particularly for the tropics, are poorly represented in ecosystem models (Wang et al., 2010; Yang et al., 2011; Goll et al., 2012; Powers et al., 2015; Reed et al., 2015) For example, when N and P availability are included in global ecosystem models NPP is reduced by up to 25%, potentially compensating eCO2 effects and turning the terrestrial biosphere into a net C source by 2100 (Wieder et al., 2015).
Nutrient limitations might restrain plant productivity, and reduce the proposed C sink strength of Amazonian forests, as has been shown for other tropical forests (Kaspari et al., 2008; Wright et al., 2011; Lambers et al., 2015; Powers et al., 2015). To date, three stand-scale nutrient addition experiments conducted in high diversity lowland tropical forests, indicate co-limitations of several nutrients regulating key plant physiological and ecosystem processes (Mirmanto et al., 1999; Kaspari et al., 2008; Wright et al., 2011; Pasquini and Santiago, 2012; Alvarez-Clare et al., 2013). However, even in relatively P-rich soils in Central America (Cleveland et al., 2011) P additions tend to have the strongest impacts, increasing decomposition rates (Kaspari et al., 2008) and stimulating growth of trees in smaller size classes (Wright et al., 2011; Alvarez-Clare et al., 2013). We therefore expect soil P to be the key nutrient restraining responses to eCO2, buffering the proposed C sink strength (Aragão et al., 2009; Cleveland et al., 2011; Baribault et al., 2012; Quesada et al., 2012).
FACE-experiments conducted in temperate forest ecosystems showed that under eCO2 an initial increase in NPP by enhanced photosynthesis was allocated to fine root production and root exudation to overcome increasing N limitation (Körner et al., 2005; Iversen et al., 2008; Norby et al., 2010). The low P-availability in the Amazon, however, rather hints toward a gradual P-limitation, but similarly as in temperate forests, this may be alleviated by increasing C allocated belowground into root production, exudates, and to mycorrhizal or N-fixing symbionts (Finzi et al., 2007; Houlton et al., 2008; Iversen, 2010). While increased root biomass might promote C sequestration, higher labile C inputs into the rhizosphere will provide energy for microbes and change microbial community composition and turnover (Deng et al., 2016). Increased root exudation could stimulate enzyme (phosphatase) production (Nottingham et al., 2012; Spohn et al., 2013; Stone et al., 2013) and induce changes of soil pH in the close vicinity of fine roots (Lloyd et al., 2001; Jones et al., 2009; Lambers et al., 2015), and enhance decomposition of leaf or root litter or of older soil organic matter (“priming-effect”; Fontaine et al., 2003; Kuzyakov, 2010). This could enhance P availability for microbial and plant uptake, but could lead to CO2 losses as autotrophic respiration (Ra), and enhance heterotrophic soil CO2 efflux (Rhet) due to higher microbial growth and activity (Fontaine et al., 2004; Blagodatskaya et al., 2010; Kelley et al., 2011). If this is the case, we predict that increases in soil respiration will decrease a likely C fertilization effect in tropical forests. Alternatively, eCO2 could increase leaf longevity and alter litter quality (e.g., increase C:P and lignin content), which could reduce decomposition and buffer CO2 losses by Rhet. Hence, conducting a FACE, as well as a factorial nutrient addition experiment will increase our mechanistic understanding of N and P limitation in mature, old growth tropical forests (Cleveland et al., 2011), and allow improving model-assumptions to enhance predictions of tropical ecosystem responses to eCO2 and alterations in nutrient availability.
Shifts in Plant C Allocation will Increase C Turnover and Thus Might Decrease C Storage in Tropical Forests
Due to potentially increased NPP in response to eCO2, and a subsequent relative investment into belowground compartments we expect a general shift in plant C allocation (Figure 1). Nonetheless, the allocation of NPP between plant compartments, as well as to carbohydrate reserves and export to symbionts is usually not accounted for in ecosystem models (Fatichi et al., 2014) or often represented by constant fractions:
where Ci represents a given plant C pool (e.g., leaves, wood, fine roots), α is a parameter in percentage and τ the turnover rate of Ci. Such constant allocation schemes perform poorly when reproducing eCO2 effects (De Kauwe et al., 2014). This is partly due to the fact that key processes such as carbon allocation to leaf, root and wood, plant mortality and soil carbon decomposition fluctuate over time and space (Rowland et al., 2014) and thus this variability should be represented in ecosystem models. Therefore, allocation schemes based on functional relationships among biomass fractions that vary with resource availability perform best in capturing field-based observations (De Kauwe et al., 2014).
Recent observations indicate that photosynthesis and plant carbon usage are temporally decoupled allowing C to be allocated when it is ecological beneficial, rather than when C is environmentally most available (Doughty et al., 2015b). Hence, seasonal reductions in wood NPP were found associated with carbon preferentially allocated to either root or canopy NPP during the dry season (Doughty et al., 2015b). Due to significant differences in turnover times of plant tissues (i.e., leaves, wood, fine roots) this suggests that projected increases in temperature and dry season length could strongly affect tropical C storage by shifting C allocation away from wood NPP (Hofhansl et al., 2015) and toward canopy and root NPP to alleviate drought-induced resource limitation (Doughty et al., 2014). As a result, tropical C storage will differ considerably depending on if C is stored in long-lived wood or allocated to plant tissue with reduced lifespan (Körner et al., 2005). In accordance, global vegetation models diverge considerably between estimates based on the representation of C allocation and pool turnover patterns. Nonetheless, despite the fact that residence time of C was found to dominate the uncertainty in the response of terrestrial vegetation to eCO2 (Friend et al., 2014), most models predict increasing C sequestration in both biomass and soils in response to eCO2 (De Kauwe et al., 2014).
Over the last decades the Amazon C sink has been substantial (Pan et al., 2011), however, recent observations suggest that the sink strength is declining due to increasing tree turnover and mortality rates (Brienen et al., 2015). Therefore, a realistic scenario in line with long-term inventory data (Phillips et al., 1998, 2008) seems that global alterations in atmospheric CO2-concentration and nutrient availability could have generated a more dynamic tropical forests (Körner, 2004). As a result, accelerated life cycles of tropical trees (Bugmann and Bigler, 2011) and associated increases in turnover times and decomposition rates have the potential to adversely affect the Amazonian C sink strength in the long-term (Brienen et al., 2015).
The Way Forward: Model-Experiment Integration
It is becoming increasingly evident that high diversity tropical forests, and in particular the Amazon, pose great challenges for global ecosystem models. Hence, the common use of 1 or 2 plant function types (PFT) with fixed parameterization and thus a common response to environmental change does not apply to highly diverse flora. Recent studies indicate that tropical C storage may be strongly determined by the hyperdominance (Fauset et al., 2015) and functional composition of tropical tree communities in association with different life-history strategies of tropical trees (Fauset et al., 2012). A CO2 induced shift to shorter average tree life span could favor fast-growing tree species that invest in low-cost tissue, which could increase the turnover of C and thus decrease the C storage at the landscape scale (Phillips et al., 2009; Fauset et al., 2012; Cernusak et al., 2013). In accordance, simulations of nonrandom species loss for fast-growing species with low wood density increased C storage by 10%, whereas the loss of high statured slow-growing species decreased C stocks by over 30% (Bunker, 2005). This further highlights that impacts of climate change and eCO2 on the Amazon forest could be more subtle than projected by a catastrophic dieback scenario, and already ongoing.
Recent studies evaluating the sensitivity of wood NPP to seasonal and interannual variations in climate showed that the growth response of tropical trees is associated to site-specific differences in drought sensitivity (Hofhansl et al., 2014), such that consistent with a hypothesized tradeoff between maximum potential growth rate and hydraulic safety the strength of the growth seasonality response among trees is significantly correlated to functional traits (Rowland et al., 2013). This suggests that changes in future climate seasonality could differentially affect the C sink strength of tropical forests due to local resource availability and tree species composition (Hofhansl et al., 2014). The recently developed set of ecosystem models based on plant-traits (van Bodegom et al., 2012; Pavlick et al., 2013; Fyllas et al., 2014; Sakschewski et al., 2015) has the potential to capture these changes, since differences in vegetation communities are depicted as a continuum, rather than discretely. Implementation of ecosystem models capable of incorporating more flexible C allocation schemes as well as trait-based parameterization could strongly improve model predictions (Franklin et al., 2012) to simulate the response of tropical forest ecosystems to climate anomalies and other short-term disturbances.
Overall, these observations indicate strong feedbacks of species specific and edaphic factors on ecosystem C storage (see Körner, 2009) and strongly suggest that the interaction between biodiversity and forest dynamics should be considered when making projections about the role of tropical forests in the global C cycle in a CO2-rich future (see Körner, 2004). This highlights the importance of manipulative in-situ experiments that simulate projected future conditions in high diversity tropical forest ecosystems and can be used to improve model-based predictions. Although given the time horizon of the two experiments neither AmazonFACE nor AFEX might capture the change in forest species composition, undoubtedly both experiments provide unique opportunities to investigate which life-history strategies and functional traits will be favored or disfavored under eCO2 and increased nutrient availability. Furthermore, AmazonFACE and AFEX, will provide crucial information on tropical C allocation schemes and the response to global changes by investigating allocation of C to structural and non-structural compounds such as the relative investment belowground to fine roots, associated mycorrhizae, and the rhizosphere as well as monitoring potential feedbacks on autotrophic and heterotrophic CO2 efflux. To that end, these manipulative and long-term ecosystem scale experiments aim to assess how predicted increases in atmospheric CO2 concentrations and alterations in resource availability will affect the growth response and thus the C sink strength of the Amazon forest ecosystem under projected global changes.
Synthesis
Following an integrated model-experiment approach guiding the design of in-situ investigations of tropical forest ecosystem responses to eCO2 and nutrient manipulation in the proposed ecosystem-scale manipulation experiments will enable us to test whether eCO2 has the expected fertilization effect at organ-, plant,- and ecosystem-level. We attempt to enhance the mechanistic understanding of potential responses of highly productive Amazonian forests thriving on highly weathered soils, and investigate whether nutrient (P) limitation affects the proposed CO2 fertilization effect. Specifically, we will investigate in-situ if nutrient limitation triggers belowground C allocation to root biomass, mycorrhizal symbionts, and into the rhizosphere in exchange for nutrients, or stimulates microbial decomposition enhancing P recycling. By conducting the proposed long-term in-situ ecosystem manipulation experiments we aim to resolve whether projected increases in atmospheric CO2 concentration and alterations in nutrient availability will induce shifts in plant C allocation which could in turn increase C turnover, and decrease the long-term C storage capacity of the Amazon forest ecosystem. Generating this novel process-based understanding will further improve model-based predictions that can be used to upscale mechanistic principles to larger spatial scales.
Author Contributions
All authors listed have made substantial contributions to the manuscript. FH, KA, KF, LF and OV wrote the paper; KS designed the conceptual scheme; AR and DL conceived the analysis and contributed valuable comments on earlier manuscript versions.
Funding
The authors gratefully acknowledge funding for the proposed long-term ecosystem experiments. AmazonFACE is supported by the cooperation agreement of MCTI with IDB (BR-T1284) and CNPq (68/2013 MCTI/CNPq/FNDCT) as well as fellowships granted by FAPEAM (2649/2014-FAPEAM). AFEX is funded by NERC (NE/L007223/1).
Conflict of Interest Statement
The authors declare that the research was conducted in the absence of any commercial or financial relationships that could be construed as a potential conflict of interest.
References
Alvarez-Clare, S., Mack, M. C., and Brooks, M. (2013). A direct test of nitrogen and phosphorus limitation to net primary productivity in a lowland tropical wet forest. Ecology 94, 1540–1551. doi: 10.1890/12-2128.1
Aragão, L. E. O. C., Malhi, Y., Metcalfe, D. B., Silva-Espejo, J. E., Jiménez, E., Navarrete, D., et al. (2009). Above- and below-ground net primary productivity across ten Amazonian forests on contrasting soils. Biogeosci. Discuss. 6, 2441–2488. doi: 10.5194/bgd-6-2441-2009
Baribault, T. W., Kobe, R. K., and Finley, A. O. (2012). Tropical tree growth is correlated with soil phosphorus, potassium, and calcium, though not for legumes. Ecol. Monogr. 82, 189–203. doi: 10.1890/11-1013.1
Beedlow, P. A., Tingey, D. T., Phillips, D. L., Hogsett, W. E., and Olszyk, D. M. (2008). Rising atmospheric CO2 and carbon sequestration in forests. Front. Ecol. Environ. 2, 315–322. doi: 10.1890/1540-9295(2004)002[0315:RACACS]2.0.CO;2
Blagodatskaya, E., Blagodatsky, S., Dordnikov, M., and Kuzyakov, Y. (2010). Elevated atmospheric CO2 increases microbial growth rates in soil: results of three CO2 enrichment experiments. Glob. Change Biol. 16, 836–848. doi: 10.1111/j.1365-2486.2009.02006.x
Brienen, R., Phillips, O. L., Feldpausch, T. R., and Gloor, E. (2015). Long-term decline of the Amazon carbon sink. Nature 519, 344–348. doi: 10.1038/nature14283
Bugmann, H., and Bigler, C. (2011). Will the CO2 fertilization effect in forests be offset by reduced tree longevity? Oecologia 165, 533–544. doi: 10.1007/s00442-010-1837-4
Bunker, D. E. (2005). Species loss and aboveground carbon storage in a tropical forest. Science 310, 1029–1031. doi: 10.1126/science.1117682
Carvalhais, N., Forkel, M., Khomik, M., Bellarby, J., Jung, M., Migliavacca, M., et al. (2014). Global covariation of carbon turnover times with climate in terrestrial ecosystems. Nature 514, 213–217. doi: 10.1038/nature13731
Cernusak, L. A., Winter, K., Dalling, J. W., Holtum, J. A. M., Jaramillo, C., Körner, C., et al. (2013). Tropical forest responses to increasing atmospheric CO2: current knowledge and opportunities for future research. Funct. Plant Biol. 40, 531–521. doi: 10.1071/FP12309
Cleveland, C. C., Townsend, A. R., Taylor, P., Alvarez-Clare, S., Bustamante, M. M. C., Chuyong, G., et al. (2011). Relationships among net primary productivity, nutrients and climate in tropical rain forest: a pan-tropical analysis. Ecol. Lett. 14, 939–947. doi: 10.1111/j.1461-0248.2011.01658.x
Cox, P. M., Betts, R. A., Collins, M., Harris, P. P., Huntingford, C., and Jones, C. D. (2004). Amazonian forest dieback under climate-carbon cycle projections for the 21st century. Theor. Appl. Climatol. 78, 1–20. doi: 10.1007/s00704-004-0049-4
Cox, P. M., Harris, P. P., Huntingford, C., Betts, R. A., Collins, M., Jones, C. D., et al. (2008). Increasing risk of Amazonian drought due to decreasing aerosol pollution. Nature 453, 212–215. doi: 10.1038/nature06960
Cox, P. M., Pearson, D., Booth, B. B., Friedlingstein, P., Huntingford, C., Jones, C. D., et al. (2013). Sensitivity of tropical carbon to climate change constrained by carbon dioxide variability. Nature 494, 341–344. doi: 10.1038/nature11882
Davidson, E. A., de Araújo, A. C., Artaxo, P., Balch, J. K., Brown, I. F., Bustamante, M. M. C., et al. (2012). The Amazon basin in transition. Nature 481, 321–328. doi: 10.1038/nature10717
De Kauwe, M. G., Medlyn, B. E., Zaehle, S., Walker, A. P., Dietze, M. C., Hickler, T., et al. (2013). Forest water use and water use efficiency at elevated CO2: a model-data intercomparison at two contrasting temperate forest FACE sites. Glob. Chang. Biol. 19, 1759–1779. doi: 10.1111/gcb.12164
De Kauwe, M. G., Medlyn, B. E., Zaehle, S., Walker, A. P., Dietze, M. C., Wang, Y. P., et al. (2014). Where does the carbon go? A model-data intercomparison of vegetation carbon allocation and turnover processes at two temperate forest free-air CO2 enrichment sites. New Phytol. 203, 883–899. doi: 10.1111/nph.12847
DeLucia, E. H., Drake, J. E., Thomas, R. B., and Gonzalez-Meler, M. (2007). Forest carbon use efficiency: is respiration a constant fraction of gross primary production? Glob. Chang. Biol. 13, 1157–1167. doi: 10.1111/j.1365-2486.2007.01365.x
Deng, Y., He, Z., Xiong, J., Yu, H., Xu, M., Hobbie, S. E., et al. (2016). Elevated carbon dioxide accelerates the spatial turnover of soil microbial communities. Glob. Chang. Biol. 22, 957–964. doi: 10.1111/gcb.13098
Doughty, C. E., Malhi, Y., Araujo-Murakami, A., Metcalfe, D. B., Silva Espejo, J. E., Arroyo, L., et al. (2014). Allocation trade-offs dominate the response of tropical forest growth to seasonal and interannual drought. Ecology 95, 2192–2201. doi: 10.1890/13-1507.1
Doughty, C. E., Metcalfe, D. B., Girardin, C. A., Amézquita, F. F., Cabrera, D. G., Huasco, W. H., et al. (2015a). Drought impact on forest carbon dynamics and fluxes in Amazonia. Nature 519, 78–82. doi: 10.1038/nature14213
Doughty, C. E., Metcalfe, D. B., Girardin, C. A., Amézquita, F. F., Durand, L., Huasco, W. H., et al. (2015b). Source and sink carbon dynamics and carbon allocation in the Amazon basin. Global Biogeochem. Cycles 29, 645–655. doi: 10.1002/2014GB005028
Drake, B. G., Gonzàlez-Meler, M. A., and Long, S. P. (1997). More efficient plants: a consequence of rising atmospheric CO2? Annu. Rev. Plant Physiol.Plant Mol. Biol. 48, 609–639.
Farquhar, G. D., von Caemmerer, S., and Berry, J. A. (1980). A biochemical model of the photosynthetic assimilation in C3 species. Planta 149, 78–90.
Fatichi, S., Leuzinger, S., and Körner, C. (2014). Moving beyond photosynthesis: from carbon source to sink-driven vegetation modeling. New Phytol. 201, 1086–1095. doi: 10.1111/nph.12614
Fauset, S., Baker, T. R., Lewis, S. L., Feldpausch, T. R., Affum-Baffoe, K., Foli, E. G., et al. (2012). Drought-induced shifts in the floristic and functional composition of tropical forests in Ghana. Ecol. Lett. 15, 1120–1129. doi: 10.1111/j.1461-0248.2012.01834.x
Fauset, S., Johnson, M. O., Gloor, M., Baker, T. R., Abel Monteagudo, M., Brienen, R. J. W., et al. (2015). Hyperdominance in Amazonian forest carbon cycling. Nat. Commun. 6:6857. doi: 10.1038/ncomms7857
Feldpausch, T. R., Lloyd, J., Lewis, S. L., Brienen, R. J. W., Gloor, M., Monteagudo Mendoza, A., et al. (2012). Tree height integrated into pantropical forest biomass estimates. Biogeosciences 9, 3381–3403. doi: 10.5194/bg-9-3381-2012
Finzi, A. C., Norby, R. J., Calfapietra, C., Gallet-Budynek, A., Gielen, B., Holmes, W. E., et al. (2007). Increases in nitrogen uptake rather than nitrogen-use efficiency support higher rates of temperate forest productivity under elevated CO2. Proc. Natl. Acad. Sci. U.S.A. 104, 14014–14019. doi: 10.1073/pnas.0706518104
Fontaine, S., Bardoux, G., Abbadie, L., and Mariotti, A. (2004). Carbon input to soil may decrease soil carbon content. Ecol. Lett. 7, 314–320. doi: 10.1111/j.1461-0248.2004.00579.x
Fontaine, S., Mariotti, A., and Abbadie, L. (2003). The priming effect of organic matter: a question of microbial competition? Soil Biol. Biochem. 35, 837–843. doi: 10.1016/S0038-0717(03)00123-8
Franklin, O., Johansson, J., Dewar, R. C., Dieckmann, U., McMurtrie, R. E., Brännström, Å., et al. (2012). Modeling carbon allocation in trees - a search for principles. Tree Physiol. 32, 648–666. doi: 10.1093/treephys/tpr138
Friend, A. D., Lucht, W., Rademacher, T. T., Keribin, R., Betts, R., Cadule, P., et al. (2014). Carbon residence time dominates uncertainty in terrestrial vegetation responses to future climate and atmospheric CO 2. Proc. Natl. Acad. Sci. U.S.A. 111, 3280–3285. doi: 10.1073/pnas.1222477110
Fyllas, N. M., Gloor, E., Mercado, L. M., Sitch, S., Quesada, C. A., Domingues, T., et al. (2014). Analysing Amazonian forest productivity using a new individual trait-based model (TFS v.1). Geosci. Model Dev. 7, 1251–1269. doi: 10.5194/gmd-7-1251-2014
Gifford, R. M. (2003). Plant respiration in productivity models: conceptualization, representation and issues for global terrestrial carbon-cycle research. Funct. Plant Biol. 30, 171–186. doi: 10.1071/FP02083
Goll, D. S., Brovkin, V., Parida, B. R., Reick, C. H., Kattge, J., Reich, P. B., et al. (2012). Nutrient limitation reduces land carbon uptake in simulations with a model of combined carbon, nitrogen and phosphorus cycling. Biogeosciences 9, 3547–3569. doi: 10.5194/bg-9-3547-2012
Hedin, L. O., Brookshire, E. N. J., Menge, D. N. L., and Barron, A. R. (2009). The Nitrogen paradox in tropical forest ecosystems. Annu. Rev. Ecol. Evol. Syst. 40, 613–635. doi: 10.1146/annurev.ecolsys.37.091305.110246
Hickler, T., Rammig, A., and Werner, C. (2015). Modelling CO2 impacts on forest productivity. Curr. Forest. Rep. 1, 69–80. doi: 10.1007/s40725-015-0014-8
Hickler, T., Smith, B., Prentice, I. C., Mjöfors, K., Miller, P., Arneth, A., et al. (2008). CO2 fertilization in temperate FACE experiments not representative of boreal and tropical forests. Glob. Chang. Biol. 14, 1531–1542. doi: 10.1111/j.1365-2486.2008.01598.x
Hofhansl, F., Kobler, J., Ofner, J., Drage, S., Pölz, E.-M., and Wanek, W. (2014). Sensitivity of tropical forest aboveground productivity to climate anomalies in SW Costa Rica. Global Biogeochem. Cycles 28, 1437–1454. doi: 10.1002/2014GB004934
Hofhansl, F., Schnecker, J., Singer, G., and Wanek, W. (2015). New insights into mechanisms driving carbon allocation in tropical forests. New Phytol. 205, 137–146. doi: 10.1111/nph.13007
Holtum, J. A. M., and Winter, K. (2010). Elevated [CO2] and forest vegetation: more a water issue than a carbon issue. Funct. Plant Biol. 37, 694–702 doi: 10.1071/FP10001
Houlton, B. Z., Wang, Y.-P., Vitousek, P. M., and Field, C. B. (2008). A unifying framework for dinitrogen fixation in the terrestrial biosphere. Nature 454, 327–330. doi: 10.1038/nature07028
Huntingford, C., Zelazowski, P., Galbraith, D., Mercado, L. M., Sitch, S., Fisher, R., et al. (2013). Simulated resilience of tropical rainforests to CO2-induced climate change. Nat. Geosci. 6, 268–273. doi: 10.1038/ngeo1741
Iversen, C. M. (2010). Digging deeper: fine-root responses to rising atmospheric CO2 concentration in forested ecosystems. New Phytol. 186, 346–357. doi: 10.1111/j.1469-8137.2009.03122.x
Iversen, C. M., Ledford, J., and Norby, R. J. (2008). CO2 enrichment increases carbon and nitrogen input from fine roots in a deciduous forest. New Phytol. 179, 837–847. doi: 10.1111/j.1469-8137.2008.02516.x
Joetzjer, E., Delire, C., Douville, H., Ciais, P., Decharme, B., Fisher, R., et al. (2014). Predicting the response of the Amazon models. Geosci. Model Dev. 7, 2933–2950. doi: 10.5194/gmd-7-2933-2014
Jones, D. L., Nguyen, C., and Finlay, R. (2009). Carbon flow in the rhizosphere: carbon trading at the soil–root interface. Plant Soil 321, 5–33. doi: 10.1007/s11104-009-9925-0
Kaspari, M., Garcia, M. N., Harms, K. E., Santana, M., Wright, S. J., and Yavitt, J. B. (2008). Multiple nutrients limit litterfall and decomposition in a tropical forest. Ecol. Lett. 11, 35–43. doi: 10.1111/j.1461-0248.2007.01124.x
Kelley, A. M., Fay, P. A., Polley, H. W., Gill, R. A., and Jackson, R. B. (2011). Atmospheric CO2 and soil extracellular enzyme activity: a meta-analysis and CO2 gradient experiment. Ecosphere 2:art96. doi: 10.1890/ES11-00117.1
Körner, C. (2003). Carbon limitation in trees. J. Ecol. 91, 4–17. doi: 10.1046/j.1365-2745.2003.00742.x
Körner, C. (2004). Through enhanced tree dynamics carbon dioxide enrichment may cause tropical forests to lose carbon. Philos. Trans. R. Soc. Lond. B Biol. Sci. 359, 493–498. doi: 10.1098/rstb.2003.1429
Körner, C. (2009). Responses of humid tropical trees to rising CO2. Annu. Rev. Ecol. Evol. Syst. 40, 61–79. doi: 10.1146/annurev.ecolsys.110308.120217
Körner, C., Asshoff, R., Bignucolo, O., Hattenschwiler, S., Keel, S. G., Pelaez-Riedl, S., et al. (2005). Carbon flux and growth in mature deciduous forest trees exposed to elevated CO2. Science 309, 1360–1362. doi: 10.1126/science.1113977
Kuzyakov, Y. (2010). Priming effects: interactions between living and dead organic matter. Soil Biol. Biochem. 42, 1363–1371. doi: 10.1016/j.soilbio.2010.04.003
Lambers, H., Hayes, P. E., Laliberte, E., Oliveira, R. S., and Turner, B. L. (2015). Leaf manganese accumulation and phosphorus-acquisition efficiency. Trends Plant Sci. 20, 83–90. doi: 10.1016/j.tplants.2014.10.007
Lapola, D. M., and Norby, R. J. (2014). Amazon-FACE: Assessing the Effects of Increased Atmospheric CO2 on the Ecology and Resilience of the Amazon Forest - Science Plan and Implementation Strategy. Brasília: Ministério de Ciência, Tecnologia e Inovação.
Lapola, D. M., Oyama, M. D., and Nobre, C. A. (2009). Exploring the range of climate biome projections for tropical South America: the role of CO2 fertilization and seasonality. Global Biogeochem. Cycles 23, 1–16. doi: 10.1029/2008GB003357
Lewis, S. L., Brando, P. M., Phillips, O. L., van der Heijden, G. M. F., and Nepstad, D. (2011). The 2010 Amazon Drought. Science 331, 554–554. doi: 10.1126/science.1200807
Liebig, J. (1840). Die organische Chemie in ihrer Anwendung auf Agrikultur und Physiologie. Braunschweig: Friedrich Vieweg und Sohn Publ. Co.
Lloyd, J., Bird, M. I., Veenendaal, E. M., and Kruijt, B. (2001). “Should phosphorus availability be constraining moist tropical forest responses to increasing CO2 concentrations?” in Global Biogeochemical Cycles in the Climate System, eds E.-D. Schulze, M. Heimann, S. P. Harrison, E. Holland, J. Lloyd, I. C. Prentice, and D. Schimel (San Diego, CA: Academic Press), 96–109.
Lloyd, J., and Farquhar, G. D. (1996). The CO2 dependence of photosynthesis, plant growth responses to elevated atmospheric CO2 concentrations and their interaction with plant nutrient status. Funct. Ecol. 10, 4–32. doi: 10.2307/2390258
Lloyd, J., and Farquhar, G. D. (2008). Effects of rising temperatures and [CO2] on the physiology of tropical forest trees. Philos. Trans. R. Soc. Lond. B Biol. Sci. 363, 1811–1817. doi: 10.1098/rstb.2007.0032
Long, S. P. (1991). Modification of the response of photosynthetic productivity to rising temperature by atmospheric CO2 concentrations: has its importance been underestimated? Plant Cell Environ. 14, 729–739. doi: 10.1111/j.1365-3040.1991.tb01439.x
Malhi, Y., Aragão, L. E. O. C., Galbraith, D., Huntingford, C., Fisher, R., Zelazowski, P., et al. (2009). Exploring the likelihood and mechanism of a climate-change-induced dieback of the Amazon rainforest. Proc. Natl. Acad. Sci. U.S.A. 106, 20610–20615. doi: 10.1073/pnas.0804619106
Malhi, Y., Roberts, J. T., Betts, R. A., Killeen, T. J., Li, W., and Nobre, C. A. (2008). Climate change, deforestation, and the fate of the Amazon. Science 319, 169–172. doi: 10.1126/science.1146961
Medlyn, B. E., Duursma, R. A., Eamus, D., Ellsworth, D. S., Prentice, I. C., Barton, C. V. M., et al. (2011). Reconciling the optimal and empirical approaches to modelling stomatal conductance. Glob. Chang. Biol. 17, 2134–2144. doi: 10.1111/j.1365-2486.2010.02375.x
Medlyn, B. E., Zaehle, S., De Kauwe, M. G., Walker, A. P., Dietze, M. C., Hanson, P. J., et al. (2015). Using ecosystem experiments to improve vegetation models. Nat. Clim. Chang. 5, 528–534. doi: 10.1038/nclimate2621
Mirmanto, E., Proctor, J., Green, J., Nagy, L., and Suriantata. (1999). Effects of nitrogen and phosphorus fertilization in a lowland evergreen rainforest. Philos. Trans. R. Soc. Lond. B Biol. Sci. 354, 1825–1829. doi: 10.1098/rstb.1999.0524
Nock, C. A., Baker, P. J., Wanek, W., Leis, A., Grabner, M., Bunyavejchewin, S., et al. (2011). Long-term increases in intrinsic water-use efficiency do not lead to increased stem growth in a tropical monsoon forest in western Thailand. Glob. Chang. Biol. 17, 1049–1063. doi: 10.1111/j.1365-2486.2010.02222.x
Norby, R. J., De Kauwe, M. G., Domingues, T. F., Duursma, R. A., Ellsworth, D. S., Goll, D. S., et al. (2016). Model–data synthesis for the next generation of forest free air CO2 enrichment (FACE) experiments. New Phytol. 209, 17–28. doi: 10.1111/nph.13593
Norby, R. J., DeLucia, E. H., Gielen, B., Calfapietra, C., Giardina, C. P., King, J. S., et al. (2005). Forest response to elevated CO2 is conserved across a broad range of productivity. Proc. Natl. Acad. Sci. U.S.A. 102, 18052–18056. doi: 10.1073/pnas.0509478102
Norby, R. J., Warren, J. M., Iversen, C. M., Medlyn, B. E., and McMurtrie, R. E. (2010). CO2 enhancement of forest productivity constrained by limited nitrogen availability. Proc. Natl. Acad. Sci. U.S.A. 107, 19368–19373. doi: 10.1073/pnas.1006463107
Nottingham, A. T., Turner, B. L., Chamberlain, P. M., Stott, A. W., and Tanner, E. V. J. (2012). Priming and microbial nutrient limitation in lowland tropical forest soils of contrasting fertility. Biogeochemistry 111, 219–237. doi: 10.1007/s10533-011-9637-4
Pan, Y., Birdsey, R. A., Fang, J., Houghton, R., Kauppi, P. E., Kurz, W. A., et al. (2011). A large and persistent carbon sink in the world's forests. Science 333, 988–993. doi: 10.1126/science.1201609
Pasquini, S. C., and Santiago, L. S. (2012). Nutrients limit photosynthesis in seedlings of a lowland tropical forest tree species. Oecologia 168, 311–319. doi: 10.1007/s00442-011-2099-5
Pavlick, R., Drewry, D. T., Bohn, K., Reu, B., and Kleidon, A. (2013). The Jena Diversity-Dynamic Global Vegetation Model (JeDi-DGVM): a diverse approach to representing terrestrial biogeography and biogeochemistry based on plant functional trade-offs. Biogeosciences 10, 4137–4177. doi: 10.5194/bg-10-4137-2013
Phillips, O. L., Aragão, L. E. O. C., Lewis, S. L., Fisher, J. B., Lloyd, J., Lopez-Gonzalez, G., et al. (2009). Drought sensitivity of the Amazon Rainforest. Science 323, 1344–1347. doi: 10.1126/science.1164033
Phillips, O. L., Lewis, S. L., Baker, T. R., Chao, K.-J., and Higuchi, N. (2008). The changing Amazon forest. Philos. Trans. R. Soc. B 363, 1819–1827. doi: 10.1098/rstb.2007.0033
Phillips, O. L., Malhi, Y., Higuchi, N., Laurance, W. F., Núñez, P. V., Vásquez, R. M., et al. (1998). Changes in the carbon balance of tropical forests: evidence from long-term plots. Science 282, 439–442.
Powers, J. S., Becklund, K. K., Gei, M. G., Iyengar, S. B., Meyer, R., O'Connell, C. S., et al. (2015). Nutrient addition effects on tropical dry forests: a mini-review from microbial to ecosystem scales. Front. Earth Sci. 3:34. doi: 10.3389/feart.2015.00034
Quesada, C. A., Lloyd, J., Schwarz, M., Patino, S., Baker, T. R., Czimczik, C., et al. (2010). Variations in chemical and physical properties of Amazon forest soils in relation to their genesis. Biogeosciences 7, 1515–1541. doi: 10.5194/bg-7-1515-2010
Quesada, C. A., Phillips, O. L., Schwarz, M., Czimczik, C. I., Baker, T. R., Patino, S., et al. (2012). Basin-wide variations in Amazon forest structure and function are mediated by both soils and climate. Biogeosciences 9, 2203–2246. doi: 10.5194/bg-9-2203-2012
Rammig, A., Jupp, T., Thonicke, K., Tietjen, B., Heinke, J., Ostberg, S., et al. (2010). Estimating the risk of Amazonian forest dieback. New Phytol. 187, 694–706. doi: 10.1111/j.1469-8137.2010.03318.x
Rastetter, E. B., and Shaver, G. R. (1992). A model of multiple-element limitation for acclimating vegetation. Ecology 73, 1157–1174. doi: 10.2307/1940666
Reed, S. C., Yang, X., and Thornton, P. E. (2015). Incorporating phosphorus cycling into global modeling efforts: a worthwhile, tractable endeavor. New Phytol. 208, 324–329. doi: 10.1111/nph.13521
Reich, P. B., Luo, Y., Bradford, J. B., Poorter, H., Perry, C. H., and Oleksyn, J. (2014). Temperature drives global patterns in forest biomass distribution in leaves, stems, and roots. Proc. Natl. Acad. Sci. U.S.A. 111, 13721–13726. doi: 10.1073/pnas.1216053111
Rowland, L., Hill, T. C., Stahl, C., Siebicke, L., Burban, B., Zaragoza-Castells, J., et al. (2014). Evidence for strong seasonality in the carbon storage and carbon use efficiency of an Amazonian forest. Glob. Chang. Biol. 20, 979–991. doi: 10.1111/gcb.12375
Rowland, L., Malhi, Y., Silva-Espejo, J. E., Farfán-Amézquita, F., Halladay, K., Doughty, C. E., et al. (2013). The sensitivity of wood production to seasonal and interannual variations in climate in a lowland Amazonian rainforest. Oecologia 174, 295–306. doi: 10.1007/s00442-013-2766-9
Sakschewski, B., Bloh, W., Boit, A., and Rammig, A. (2015). Leaf and stem economics spectra drive diversity of functional plant traits in a dynamic global vegetation model. Glob. Chang. Biol. 21, 2711–2725. doi: 10.1111/gcb.12870
Sitch, S., Huntingford, C., Gedney, N., and Levy, P. E. (2008). Evaluation of the terrestrial carbon cycle, future plant geography and climate-carbon cycle feedbacks using five Dynamic Global Vegetation Models (DGVMs). Glob. Chang. Biol. 14, 2015–2039. doi: 10.1111/j.1365-2486.2008.01626.x
Smith, B., Wårlind, D., Arneth, A., Hickler, T., Leadley, P., Siltberg, J., et al. (2014). Implications of incorporating N cycling and N limitations on primary production in an individual-based dynamic vegetation model. Biogeosciences 11, 2027–2054. doi: 10.5194/bg-11-2027-2014
Spohn, M., Ermak, A., and Kuzyakov, Y. (2013). Microbial gross organic phosphorus mineralization can be stimulated by root exudates - A 33P isotopic dilution study. Soil Biol. Biochem. 65, 254–263. doi: 10.1016/j.soilbio.2013.05.028
Sterner, R. W., and Elser, J. J. (2002). Ecological Stoichiometry: the Biology of Elements from Molecules to the Biosphere. Princton, NJ: Princton University Press.
Stone, M., Plante, A., and Casper, B. (2013). Plant and nutrient controls on microbial functional characteristics in a tropical Oxisol. Plant Soil 373, 893–905. doi: 10.1007/s11104-013-1840-8
van Bodegom, P. M., Douma, J. C., Witte, J. P. M., Ordoñez, J. C., Bartholomeus, R. P., and Aerts, R. (2012). Going beyond limitations of plant functional types when predicting global ecosystem-atmosphere fluxes: exploring the merits of traits-based approaches. Glob. Ecol. Biogeogr. 21, 625–636. doi: 10.1111/j.1466-8238.2011.00717.x
van der Sleen, P., Groenendijk, P., Vlam, M., Anten, N. P. R., Boom, A., Bongers, F., et al. (2014). No growth stimulation of tropical trees by 150 years of CO2 fertilization but water-use efficiency increased. Nat. Geosci. 8, 24–28. doi: 10.1038/ngeo2313
Vitousek, P. M., Porder, S., Houlton, B. Z., and Chadwick, O. A. (2010). Terrestrial phosphorus limitation: mechanisms, implications, and nitrogen – phosphorus interactions. Ecol. Appl. 20, 5–15. doi: 10.1890/08-0127.1
Vitousek, P. M., and Sanford, R. L. Jr. (1986). Nutrient cycling in moist tropical forest. Annu. Rev. Ecol. Syst. 17, 137–167. doi: 10.1146/annurev.es.17.110186.001033
Walker, T. W., and Syers, J. K. (1976). The fate of phosphorus during pedogenesis. Geoderma 15, 1–19. doi: 10.1016/0016-7061(76)90066-5
Wang, Y. P., Law, R. M., and Pak, B. (2010). A global model of carbon, nitrogen and phosphorus cycles for the terrestrial biosphere. Biogeosciences 7, 2261–2282. doi: 10.5194/bg-7-2261-2010
Wieder, W. R., Cleveland, C. C., Smith, W. K., and Todd-Brown, K. (2015). Future productivity and carbon storage limited by terrestrial nutrient availability. Nat. Geosci. 8, 441–444. doi: 10.1038/ngeo2413
Wright, S. J., Yavitt, J. B., Wurzburger, N., Turner, B. L., Tanner, E. V. J., Sayer, E. J., et al. (2011). Potassium, phosphorus, or nitrogen limit root allocation, tree growth, or litter production in a lowland tropical forest. Ecology 92, 1616–1625. doi: 10.1890/10-1558.1
Yang, X., Thornton, P. E., Ricciuto, D. M., and Post, W. M. (2014). The role of phosphorus dynamics in tropical forests – a modelling study using CLM-CNP. Biogeosciences 11, 1667–1681. doi: 10.5194/bg-11-1667-2014
Yang, Y., Luo, Y., Lu, M., Schädel, C., and Han, W. (2011). Terrestrial C:N stoichiometry in response to elevated CO2 and N addition: a synthesis of two meta-analyses. Plant Soil 343, 393–400. doi: 10.1007/s11104-011-0736-8
Zaehle, S., Medlyn, B. E., De Kauwe, M. G., Walker, A. P., Dietze, M. C., Hickler, T., et al. (2014). Evaluation of 11 terrestrial carbon-nitrogen cycle models against observations from two temperate Free-Air CO2 Enrichment studies. New Phytol. 202, 803–822. doi: 10.1111/nph.12697
Zhao, M., and Running, S. W. (2010). Drought-induced reduction in global terrestrial net primary production from 2000 through 2009. Science 329, 940–943. doi: 10.1126/science.1192666
Zhou, X. H., Fu, Y. L., Zhou, L. Y., Li, B., and Luo, Y. Q. (2013). An imperative need for global change research in tropical forests. Tree Physiol. 33, 903–912. doi: 10.1093/treephys/tpt064
Keywords: Amazon, carbon allocation, elevated CO2, free-air CO2 enrichment (FACE), nutrient addition, tropical forest
Citation: Hofhansl F, Andersen KM, Fleischer K, Fuchslueger L, Rammig A, Schaap KJ, Valverde-Barrantes OJ and Lapola DM (2016) Amazon Forest Ecosystem Responses to Elevated Atmospheric CO2 and Alterations in Nutrient Availability: Filling the Gaps with Model-Experiment Integration. Front. Earth Sci. 4:19. doi: 10.3389/feart.2016.00019
Received: 31 October 2015; Accepted: 11 February 2016;
Published: 26 February 2016.
Edited by:
Juergen Homeier, University of Goettingen, GermanyReviewed by:
Derrick Y. F. Lai, The Chinese University of Hong Kong, ChinaLucas Cernusak, James Cook University, Australia
Copyright © 2016 Hofhansl, Andersen, Fleischer, Fuchslueger, Rammig, Schaap, Valverde-Barrantes and Lapola. This is an open-access article distributed under the terms of the Creative Commons Attribution License (CC BY). The use, distribution or reproduction in other forums is permitted, provided the original author(s) or licensor are credited and that the original publication in this journal is cited, in accordance with accepted academic practice. No use, distribution or reproduction is permitted which does not comply with these terms.
*Correspondence: Florian Hofhansl, Zmxvcmlhbi5ob2ZoYW5zbEBpbnBhLmdvdi5icg==