- 1Institute of Geography and Geoecology, Karlsruhe Institute of Technology, Karlsruhe, Germany
- 2Geographic Institute, University of Bern, Bern, Switzerland
- 3Research Directorate, National University of Loja, Loja, Ecuador
In the past two decades, the tropical montane rain forests in south Ecuador experienced increasing deposition of reactive nitrogen mainly originating from Amazonian forest fires, while Saharan dust inputs episodically increased deposition of base metals. Increasing air temperature and unevenly distributed rainfall have allowed for longer dry spells in a perhumid ecosystem. This might have favored mineralization of dissolved organic matter (DOM) by microorganisms and increased nutrient release from the organic layer. Environmental change is expected to impact the functioning of this ecosystem belonging to the biodiversity hotspots of the Earth. In 2007, we established a nutrient manipulation experiment (NUMEX) to understand the response of the ecosystem to moderately increased nutrient inputs. Since 2008, we have continuously applied 50 kg ha−1 a−1 of nitrogen (N), 10 kg ha−1 a−1 of phosphorus (P), 50 kg + 10 kg ha−1 a−1 of N, and P and 10 kg ha−1 a−1 of calcium (Ca) in a randomized block design at 2000 m a.s.l. in a natural forest on the Amazonia-exposed slopes of the south Ecuadorian Andes. Nitrogen concentrations in throughfall increased following N+P additions, while separate N amendments only increased nitrate concentrations. Total organic carbon (TOC) and dissolved organic nitrogen (DON) concentrations showed high seasonal variations in litter leachate and decreased significantly in the P and N+P treatments, but not in the N treatment. Thus, P availability plays a key role in the mineralization of DOM. TOC/DON ratios were narrower in throughfall than in litter leachate but their temporal course did not respond to nutrient amendments. Our results revealed an initially fast, positive response of the C and N cycling to nutrient additions which declined with time. TOC and DON cycling only change if N and P supply are improved concurrently, while NO3-N leaching increases only if N is separately added. This indicates co-limitation of the microorganisms by N and P. The current increasing reactive N deposition will increase N export from the root zone, while it will only accelerate TOC and DON turnover if P availability is simultaneously increased. The Saharan dust-related Ca deposition has no impact on TOC and DON turnover.
Introduction
Tropical forests generate one third of the terrestrial gross primary production (Beer et al., 2010), absorb large amounts of carbon dioxide (CO2) from the atmosphere (Schimel et al., 2014) and store a considerable amount of carbon (C) in the biomass (Jobbagy and Jackson, 2000). Thus, they play a key role in regulating concentrations of greenhouse gases in the atmosphere and global climate. However, they are vulnerable to environmental change and perturbation by human activities. Climate change (Vuille et al., 2003; Urrutia and Vuille, 2009) and nitrogen (N) deposition (Galloway et al., 2004, 2008; Hietz et al., 2011) are current environmental changes which might threaten the functioning of the native tropical ecosystems in the north Andes.
The tropical Andean forest in south Ecuador has experienced improved nutrient supply in the past 15 years because of steadily increasing N and episodic calcium (Ca) and magnesium (Mg) deposition (Boy and Wilcke, 2008; Wilcke et al., 2013a). The observed N deposition is mainly attributable to biomass burning resulting from the conversion of natural forests to agricultural land in the Amazon Basin (Galloway et al., 2004, 2008; Da Rocha et al., 2005; Boy et al., 2008). On the other side, dust particles rich in Ca and Mg originating from the Sahara are transported over the Atlantic and deposited from the atmosphere on the eastern slopes of the Andean cordillera mainly during La Niña events (Kaufman et al., 2005; Boy and Wilcke, 2008; Wilcke et al., 2013b). Phosphorus (P) is predominantly deposited as mineral aerosol dust transported with the trade winds from the Sahara to the Caribbean and the Amazon Basin (Pett-Ridge, 2009). At the same time, increasing air temperature and increasingly unevenly distributed rainfall have allowed for longer dry spells and reduced air humidity (Peters et al., 2013), resulting in a reduction of the water content in the thick organic layer, where the activity of soil organisms is highest.
Dissolved organic matter (DOM) serves as a C and energy source for microorganisms and is an essential component of the global C and N cycles (Van Hees et al., 2005; Kalbitz and Kaiser, 2008; Bolan et al., 2011). Leaching of vegetation canopies, litterfall and soil organic matter are the most important sources of DOM (Kalbitz et al., 2000). There is strong indication that the fate of DOM in soils is controlled by microbial degradation (Kalbitz et al., 2000). Thus, less waterlogging could favor mineralization of DOM by microorganisms and hence increase nutrient release from the organic layer into the mineral soil (Roman et al., 2010).
As a result of further agricultural and industrial development in the Amazon Basin (Walker et al., 2009), biomass burning and thus N supply with easterly winds is expected to continue in the near future. This will result most likely in further changes of the N cycle in the remote natural montane ecosystems of south Ecuador, where dissolved organic nitrogen (DON) dominates the N cycling (Goller et al., 2006; Wilcke et al., 2013a). Increasing N richness can furthermore result in dissolved N losses from terrestrial ecosystems to ground and surface waters, posing a potential threat to water quality (Matson et al., 1999; Corre et al., 2010). Moreover, elevated supply of easily plant-available inorganic N forms—ammonium () and nitrate () – might favor only certain plant species by stimulating biomass production in a way that could lead to changes in species composition and ultimately even to a reduction of species richness in the megadiverse tropical montane forest ecosystem of south Ecuador, which belongs to the global biodiversity hotspots of the Earth (Myers et al., 2000; Phoenix et al., 2006).
In the fragile montane ecosystems, it is frequently assumed that nutrient constraints limit plant growth and primary production, which are known to be regulated by the supply of key elements, like N and P (Vitousek and Howarth, 1991; Tanner et al., 1998). In younger soils, N limitations were alleged to be more frequent, since N must be accumulated from the atmosphere, while P limitations often occur in older soils, because availability of P, which is derived from the parent material, is declining during soil formation (Walker and Syers, 1976). However, this model does not take into account that thick organic layers with high nutrient stocks develop in many tropical montane forests, which may provide sufficient N for tree growth (Wilcke et al., 2002) especially within the context of changing environmental conditions (e.g., reduced waterlogging and increasing air temperature).
Since nutrient limitation was thought to be widespread in tropical soils, P would usually limit productivity in lowland rain forests growing on heavily weathered soils, while N, in contrast, would be the limiting nutrient in montane rain forests growing on less weathered young soils (Tanner et al., 1998). Single nutrient limitations which vary with soil age were tested in several studies, where N additions to tropical montane forests and P additions to lowland forests increased forest growth and primary production (Vitousek, 1984; Tanner et al., 1990, 1992; Vitousek et al., 1993; Adamek et al., 2009). Vitousek et al. (2010) investigated relations between N and P limitations and suggested depletion, soil barriers, and low-P parent material as mechanisms controlling the ecosystem mass balance of P.
Recently, single nutrient limitations have begun to be questioned. The study of Kaspari et al. (2008) in a tropical lowland forest in Panama revealed that nutrient limitations may vary not only between, but also within ecosystems, so that different limitations occur in the canopy, in the organic layer or in the mineral soil and that nutrient limitation can also vary temporally. In the same forest, potassium (K), phosphorus (P), or nitrogen (N) were shown to limit root allocation, tree growth, or litter production (Wright et al., 2011). At the same time, extractable nutrients showed a variable response to fertilizer addition and to the seasonality of the climate (Turner et al., 2013). In the tropical montane forest of south Ecuador, incubation experiments suggested that soil microorganisms might also be limited by calcium, manganese, sulfur, and zinc (Wilcke et al., 2002). In forests of the Oregon coast range, Ca cycling was found to be coupled to the N cycle, particularly if N concentrations in the ecosystem ranged between N limitation and N saturation (Perakis et al., 2006). One of the most comprehensive meta-analyses of studies on nutrient additions emphasized that both N and P limitations were widespread in terrestrial systems and revealed synergistic effects of combined N and P enrichment, which prevailed over individual element limitations (Elser et al., 2007). Multiple nutrient co-limitations were also found in grassland ecosystems for aboveground net primary production (Fay et al., 2015), including N, P, K, and several other micronutrients. In a large-scale study in the Peruvian Andes and Amazonia, Fisher et al. (2013) showed that nutrient–productivity relationships depend on elevation, indicating strong N and P co-limitation at sites up to 1000 m a.s.l. and strong N limitation at sites above 1000 m a.s.l. These results demonstrate that various nutrient co-limitations may occur in different ecosystems and may be widespread across multiple elevation and latitudinal gradients.
However, constraints to primary production may be different from limitations of organic matter cycling. A large amount of nutrients is stored in the organic layer of tropical montane forest soils. Thickness of organic layers was shown to increase with elevation, because of decreasing temperatures and increasing soil humidity, which inhibit mineralization of organic matter and rates of nutrient turnover (Wilcke et al., 2002, 2008; Arnold et al., 2009). Litter decomposition was limited by N and P in a tropical forest in Hawaii, while litterfall and stem growth were only limited by N (Hobbie and Vitousek, 2000). In another nutrient addition experiment in a montane rain forest in Panama, N additions increased not only stem growth and litterfall production (Adamek et al., 2009), but also N-cycling rates in the soil as well as NO3 leaching, resulting in a more open N cycle (Corre et al., 2010).
Nutrient limitations play an important role in the control of C and N cycling in tropical montane forests. Improved nutrient availability favors microbial activity and an elevated N supply is known to increase the turnover of light, easily degradable organic matter, while it further stabilizes heavier, more recalcitrant compounds of the organic matter pool (Neff et al., 2002; Janssens et al., 2010). Fisher et al. (2013) also reported that addition of N was associated with an increase in microbial respiration, which confirms results revealing increased decomposition rates of easily degradable litter as a result of N additions (Janssens et al., 2010). Bragazza et al. (2006) reported a biologically controlled increase in dissolved organic carbon (DOC) release from peat with increasing N deposition in a laboratory incubation of peat bogs from different European sites with varying N deposition rates. For 17 sites across northwest Europe and North America Evans et al. (2008) did not find a consistent effect of experimental N application on DOM concentrations (in nine cases DOC concentrations increased in response to N application and in eight cases DOC concentrations decreased). Acidifying N application (as ) mostly decreased DOC concentrations while non-acidifying N application (as or NH3) increased DOC concentrations. There was no general relationship between N addition rate or N concentrations in soil solution and DOC concentrations. A positive correlation between C/N ratios of the organic layer with DOC concentrations in soil solutions reported by Borken et al. (2011) for a number of south German forests suggests that increasing N availability decreases DOM concentrations, although this does not seem to be generally true (Michalzik et al., 2001). In a tropical montane forest in the south Ecuadorian Andes, Baldos et al. (2015) found that N additions led to a decrease in K2SO4 extractable C, indicating a reduction in the easily degradable C fraction in the organic layer. Finally, at an adjacent study site, results from a long-term monitoring at micro-catchment scale revealed steadily decreasing DON concentrations and fractional contribution of DON to total N in rainfall, throughfall and soil solutions over 15 years (Wilcke et al., 2013a). The observed decreasing organic contributions to total N were not only the result of increasing inorganic N deposition over 15 years, but could also be attributable to enhanced DON decomposition, which might be in turn related with reduced waterlogging occurring simultaneously with higher nutrient availability.
Studies conducted during the last decade often stressed that N emissions to the atmosphere and the increasing use of fertilizers in the modern world strongly interfere with natural nutrient cycling (Galloway et al., 2004, 2008). In the past century, the N cycle was markedly changed in the northern hemisphere because of human-induced N emissions to the environment and the use of mineral fertilizers to increase crop yields (Van Breemen, 2002; Galloway et al., 2004, 2008; Erisman et al., 2008). A widely used method to determine the response of ecosystems to increased nutrient supply and to determine which elements might have limiting effects on ecosystem processes is to set up fertilizer experiments, were nutrients are applied at a high rates to ecosystems (up to 250 kg ha−1 a−1), to trigger fast responses that can be observed within short time (Tanner et al., 1998; LeBauer and Treseder, 2008; Fisher et al., 2013). However, these rates are much higher than actual or expected nutrient deposition of a few tens of kg ha−1 a−1 (Galloway et al., 2004, 2008). Because responses to low level fertilizer amendments might only be visible after longer periods, studies investigating effects of moderate nutrient additions are rare (Gaige et al., 2007; Fang et al., 2009).
In summary, investigations in tropical montane forests have shown that a considerable uncertainty still persists with regard to the potential response of the N and DOM cycling in these ecosystems to increasing supply of nutrients from atmospheric deposition and from climatically favored, enhanced mineralization of soil organic matter. Therefore, our objectives were to elucidate the response of (i) the N cycle in a tropical montane forest and (ii) the dissolved organic C and N concentrations and TOC/DON ratios to nutrient additions. We hypothesized that (i) N and Ca additions increase N concentrations while P additions (alone and in combination with N) reduce N concentrations in throughfall and soil solutions and (ii) that increasing nutrient availability decreases TOC and DON concentrations and widens the TOC/DON ratios. We tested these hypotheses in a nutrient manipulation experiment (NUMEX), in which N, P, N+P, and Ca were fertilized at moderate levels considered as reflecting realistic future changes in nutrient availability.
Materials and Methods
Study Area
The study area is located in the province of Zamora-Chinchipe, on the Amazon-exposed slopes of the south Ecuadorian Andes (3.58° S, 79.08 W). The experimental plots are situated in the Reserva Biológica San Francisco at an altitude between 2010 and 2128 m a.s.l. (Figure 1). The slope of the plots ranges between 15 and 40°, with an average of 28°. Precipitation is unimodally distributed over the year and reaches its maximum between April and July, yet without a marked dry season. Between 1999 and 2014, the annual rainfall averaged 2291 ± 228 mm (SD). During the same period, at the meteorological station at 1957 m a.s., the mean annual temperature was 15.4 ± 0.3°C and the mean air humidity was 83.5 ± 1.4%. The warmest month was November with 16.4 ± 0.6°C and the coldest month was July with 14.5 ± 0.7°C. Temperature and humidity were aggregated from meteorological data of the ECSF climate station (Rollenbeck et al., 2015).
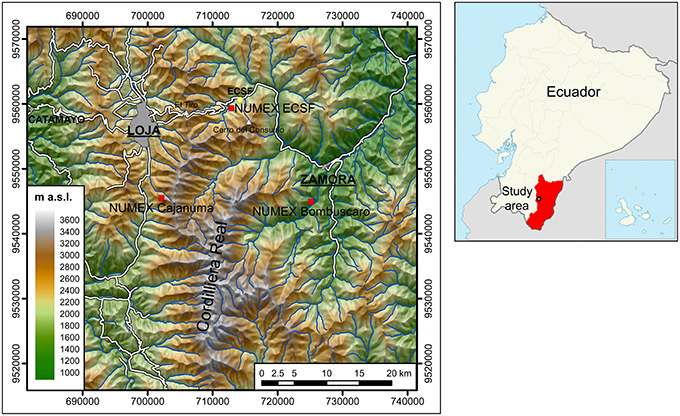
Figure 1. Location of the research area and of the Nutrient Manipulation Experiment (NUMEX). Our study focuses on the experimental site at 2000 m a.s.l. near the Research Station San Francisco (ECSF). Coordinate system: WGS84, UTM Zone 17 M. Cartographic data: Instituto Geográfico Militar (2013).
The vegetation cover consists of a near-natural, little disturbed lower montane forest (Bruijnzeel and Hamilton, 2000; Homeier et al., 2008), which has an extremely high species richness and is considered to be a hotspot of biodiversity (Barthlott et al., 2005). The mean canopy height reaches 12–14 m and the mean crown radius ranges between 2 and 4 m, reaching up to 5–6 m. The most abundant families are Lauraceae, Melastomataceae, and Rubiaceae, with Graffenrieda emarginata Triana (Melastomaceae) being the most abundant tree species (Homeier and Werner, 2007). The canopy cover is very dense (Leaf Area Index of 5.19 to 9.32, Fleischbein et al., 2005) and retains with an evaporative interception loss of about 47% a high proportion of the incident precipitation (Fleischbein et al., 2006; Wullaert et al., 2009).
The soils of the experimental area are young and mostly shallow (< 60 cm), have a loamy texture and were classified according to IUSS Working Group WRB (2006) as Stagnic Cambisols (Hyperdystric, Chromic), which developed from Palaeozoic phyllites, quartzites, and metasandstones belonging to the Chiguinda unit of the Zamora series (Hungerbühler, 1997). The mineral soil is covered by a thick organic layer (30 ± 9 cm) with a very low bulk density (0.08 ± 0.01 g cm−3), which constitutes the main rooting zone for plants and trees (Wilcke et al., 2002).
Experimental Design
NUMEX was established in the year 2007 and is continued until present. It consists of three experimental sites along an altitudinal gradient at 1000, 2000, and 3000 m a.s. (Figure 1). Details on all three NUMEX sites are given by Martinson et al. (2010) and Homeier et al. (2012). We focus on the part of the experiment at 2000 m a.s.l. located in the Reserva Biológica San Francisco. NUMEX is an interdisciplinary experiment and consists of low level, continuous additions of N, P, combined N and P, Ca, and unfertilized control plots. Nutrient additions started in January 2008 and take place twice a year. The fertilizers were applied directly to the forest soil (Wullaert et al., 2010). Special care was taken to insure the homogeneous distribution of the fertilizer on the experimental plots.
The experiment site includes 20 plots (20 × 20 m) in a fourfold replicated, randomized block design (Figure 2). Since 2008, we have continuously applied 50 kg ha−1 a−1 of N, 10 kg ha−1 a−1 of P, 50 kg + 10 kg ha−1 a−1 of N and P, and 10 kg ha−1 a−1 of Ca. The fertilization rates were similar to the values of annual natural deposition (Figure 3), since our aim was to study the response of the forest ecosystem to a moderate increase of nutrient input. Because of Ecuadorian regulations which impeded the use of ammonium nitrate (NH4NO3), N was applied as commercially available urea (46% N). P was applied as sodium di-hydrogen phosphate (NaH2PO4 · H2O, AppliChem GmbH, Darmstadt, Germany) and Ca as calcium chloride (CaCl2 · 2 H2O, Merck, Darmstadt, Germany). Both used chemicals were of analytical reagent grade.
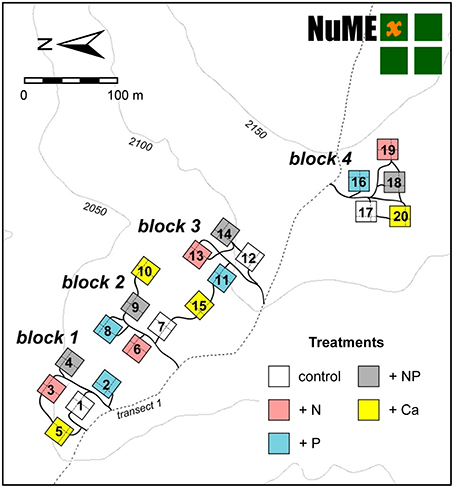
Figure 2. Layout of the Nutrient Manipulation Experiment (NUMEX) in the Reserva Biológica San Francisco at 2000 m a.s.l. The experiment site includes 20 plots in a fourfold replicated, randomized block design (four nutrient addition plots and one control treatment in each block).
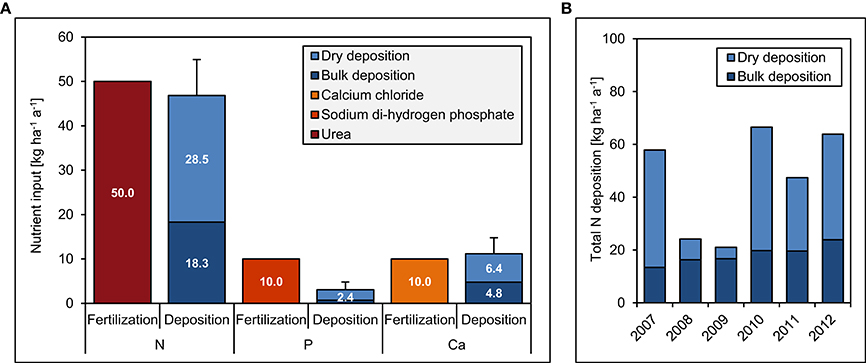
Figure 3. Fertilization and deposition rates between 2007 and 2012 in the Nutrient Manipulation Experiment (NUMEX). The diagrams show (A) annual fertilization and deposition rates of N, P, and Ca and (B) total annual N deposition during the study period. Error bars indicate one standard error of average total deposition rates over the 5 years.
Field Sampling
In 2007, all study plots were equipped with throughfall collectors, zero-tension litter lysimeters, and suction cups at the 0.15 and 0.3 m mineral soil depths.
Throughfall was collected with 20 rain gauges (Hellmann type) which were randomly distributed on each plot along two perpendicular transects, resulting thus in 400 collectors for the whole experiment area. To ensure sampling representativeness, number and spatial distribution of the throughfall collectors were determined taking into account the spatial and temporal variability of precipitation, which was analyzed in a separate experiment between 2004 and 2008 (Wullaert et al., 2009). The collectors consisted of a 2000-mL wide mouth PE bottle, and a PE funnel with a diameter of 118 mm closed at the lower end with a polyethylene net with a 0.5 mm mesh size, to avoid contamination by litter debris and insects. All bottles were wrapped in aluminum foil to reduce irradiation effects and a table-tennis ball was placed inside the funnels to reduce evaporation. Volumes of collected throughfall were measured in the field with a graduated cylinder and samples were bulked plotwise, resulting in 20 volume-weighted samples per collecting date. To guarantee a homogeneous chemical quality of the sampling throughout the duration of the experiment, throughfall collectors were regularly cleaned with distilled water and temporarily covered with plastic bags during fertilization to avoid direct contamination by the applied chemicals.
Litter leachate (solution percolating through the organic layer) was collected using three zero-tension lysimeters per plot, which consisted of plastic boxes covered with a polyethylene net with a 0.5 mm mesh size, connected to sampling bottles, which were wrapped in aluminum foil. The lysimeters were installed underneath the organic layer at 7–51 cm below ground surface from the side of a small soil pit, at upper, middle and lower slope positions, taking care that the organic layer itself was only minimally disturbed during or after installation. Litter leachate was bulked plotwise after individual sampling of the collecting bottles, resulting thus in 20 samples per collecting date.
Throughfall and litter leachate have been collected fortnightly since August 2007. Incident rainfall was collected weekly at 2–4 gauging stations and five rain collectors at each station, with the same Hellmann-type collectors used for sampling throughfall. After collection, throughfall and litter leachate samples were transferred to the field laboratory of the Estación Científica San Francisco (ECSF), where they were immediately filtered through ashless filter paper, pore size 4–7 μm, Type 392 (Sartorius-Stedim GmbH, Göttingen, Germany) and then kept at −20°C until shipping in frozen state to Switzerland for chemical analysis.
Chemical Analyses and Quality Control
For this study, we analyzed a dataset of 5 complete years including a fortnightly record of element concentrations in throughfall and litter leachate between 2007 and 2012, which we mainly measured in the laboratory of the Geographic Institute at the University of Bern, Switzerland. Electrical conductivity (TetraCon 325, WTW GmbH, Weilheim, Germany) and pH values (Sentix 81, WTW GmbH, Weilheim, Germany) were immediately measured in unfiltered aliquots of the collected water samples in the laboratory of the ECSF in Ecuador.
Concentrations of the dissolved N species ammonium-N (NH4-N), nitrate-N (NO3-N) and total nitrogen (TN) were measured by continuous flow analysis (CFA) using high resolution colorimetry and photometric detection (AutoAnalyzer 3 HR, Seal GmbH, Norderstedt, Germany). During the CFA measurements, samples were dialyzed and TN was oxidized to nitrate by UV digestion with potassium peroxydisulfate and sodium hydroxide in presence of a boric acid buffer. NH4-N was determined by the Berthelot reaction and NO3-N by cadmium reduction. The determination of NO3-N concentrations by cadmium reduction usually includes a small amount of nitrite-N (NO2-N), which was below the detection limits and hence considered negligible. We measured TOC (filtered through 4–7 μm pores) as non-purgeable organic carbon (NPOC) by elemental analysis through high temperature combustion and infrared (IR) detection (varioTOC cube, elementar Analysensysteme GmbH, Hanau, Germany), after acidifying 10 mL of the sample with 50 μL of a 10% HCl solution to remove inorganic C.
Average detection limits were 0.024 mg L−1 for NH4-N, 0.016 mg L−1 for NO3-N, 0.042 mg L−1 for TN and 0.16 mg L−1 for TOC. The quality of the CFA measurements was verified by running certified N standards (Merck, Darmstadt, Germany) with a concentration of 0.4 mg L−1 NH4-N and 0.5 mg L−1 NO3-N in each measured batch. Certified standards were combined with in-house control standards, with a concentration of 0.5 mg L−1 NH4-N and 0.25 mg L−1 NO3-N, which were run on average every 20 samples. The quality of TOC measurements was verified by use of in-house control standard only, which had a concentration of 5 mg L−1 and 10 mg L−1 TOC. Measurement batches were only accepted if the results for the control standards deviated by less than 10% from the target values. The relative SD of the measurement precision of the control standards was ± 4% for NH4-N, ± 2% for NO3-N, ± 5% for TN, and ± 3% for TOC.
Calculations and Statistical Evaluation
Throughfall fluxes were measured fortnightly in the field, averaged per plot and summed up to monthly and annual data. Concentrations of dissolved organic nitrogen (DON) were calculated as difference between TN and the sum of NH4-N and NO3-N concentrations. Values below the detection limit were taken as zero for calculations, possibly resulting in an underestimation of the DON concentrations. Monthly mean concentrations were calculated by averaging fortnightly concentrations of each measured element. Annual mean element concentrations were calculated as volume-weighted means of monthly concentrations. The effects of nutrient addition on the investigated element concentrations were expressed as log response ratios (RRx), which were calculated as a natural logarithm of the ratios between the measured values in the nutrient addition treatments and in the control plots. A value of 0.2 indicates thus a relative difference in concentrations of 22% between treatment and control, while a value of 0.5 indicates a relative difference of 65%.
We performed statistical analyses with the software R, version 3.0.2 (R Core Team, 2013). To test for pre-existing differences among the 20 plots of the experiment with respect to element concentrations in throughfall and litter leachate, we performed an analysis of variance (ANOVA) on data collected before the first fertilization (August 2007 to December 2007). To reveal differences in element concentrations in throughfall and litter leachate between treatments and control plots and compare different effects among treatments after the start of nutrient additions in 2008, we used linear mixed effects models implemented in the R-package lme4 (Bates et al., 2015) to perform a repeated measures analysis. We tested for homogeneity of variances and insured normal distribution of the residuals by visual inspection. We specified treatments as fixed effects, with the four blocks as random factor over the 5 years of the experiment, and calculated significance levels of the fixed effects with the functions cftest and glht in the package multcomp (Hothorn et al., 2008).
Time series of element concentrations in monthly data were analyzed using the seasonal Mann-Kendall test, as suggested by Hirsch et al. (1982), which is included in the R-package Kendall (McLeod, 2011). This non-parametric test estimates trends in time series while correcting for seasonality (Helsel and Hirsch, 2002). The rank correlation coefficient tau (τ) measures the strength of the temporal trend. Since it is a sign test, it is robust against outliers and can be used in spite of data gaps, which commonly occur when there is not enough sample volume collected in the field. Linear regression lines in our figures are only meant to illustrate detected significant trends and do not necessarily imply that these trends are linear.
Results
Water Fluxes
We did not detect significant differences between treatments and control plots either before the start of nutrient additions or during the 5 years of the experiment (Table 1). We collected throughfall quantitatively in high spatial resolution, because it is the most relevant water flux which also controls the amount of water percolating through the organic layer. Consequently, in the following we consider element concentrations and their responses to nutrient additions.
Effects of Nutrient Additions on Mean Annual Nutrient Concentrations in Ecosystem Solutions
Cumulative Responses of Element Concentrations in Throughfall after 5 Years
The analysis of element concentrations by linear mixed models (Figure 4) revealed a potentially negative, but not significant effect (p = 0.135) of separate P additions on TOC concentrations in throughfall, which were 17 ± 7% (SE) lower than in the control treatments. Simultaneous additions of N and P led to marginally higher mean concentrations of DON (13 ± 4%, p = 0.092). Separate amendments of Ca did not show significant effects on TOC and DON concentrations. Mean TOC/DON ratios were ca. 10% narrower in all treatments (p < 0.05) compared to the control as a result of overall lower TOC and higher DON concentrations in throughfall.
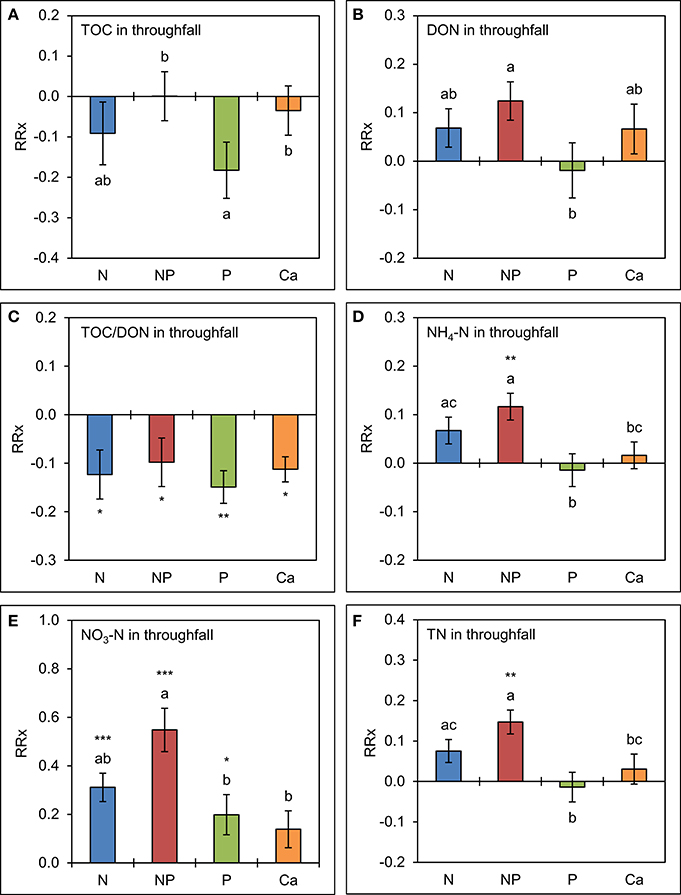
Figure 4. Cumulative effects of nutrient manipulations observed in throughfall after 5 years of nutrient additions. The diagrams show the average responses over 5 years of (A) total organic carbon (TOC) concentrations, (B) dissolved organic nitrogen (DON) concentrations, (C) TOC/DON ratios, (D) ammonium-N (NH4-N) concentrations, (E) nitrate-N (NO3-N) concentrations, and (F) total nitrogen (TN) concentrations to nutrient additions expressed as natural log-response ratios (RRx) between the respective treatments (n = 4) and the unfertilized control plots (n = 4). Error bars indicate plus or minus one standard error. Asterisks indicate significant differences to the control (*p < 0.05, **p < 0.01, ***p < 0.001). Values not sharing a common letter are significantly different from each other (p < 0.05).
Nitrogen additions generally resulted in increased concentrations of inorganic N species. The NH4-N concentrations were 7 ± 3% higher than in the control in the plots with separate N additions (p = 0.080) and 12 ± 3% higher in the N+P treatment (p = 0.002). Nitrate-N concentrations showed a strong response to higher N supply. They were 37 ± 6% higher (p = 0.001) if N was separately added and 73 ± 9% higher (p < 0.001) if N was added in combination with P. While separate P additions had no effect on NH4-N concentrations in throughfall (p = 0.703), they caused an increased NO3-N production in the canopy resulting in concentrations which were 22 ± 9% higher (p = 0.032) than in the control treatments. These results indicate that higher N supply increases the N richness of the canopy and leads to higher concentrations of inorganic N species in throughfall, and that NO3-N production and/or leaching in the canopy is stimulated by the addition of P, both separately or in combination with N. Total N concentrations in throughfall seem to be driven by the direct contributions of NH4-N, while Ca additions did not show any significant effects on inorganic N concentrations in throughfall over 5 years.
Cumulative Responses of Element Concentrations in Litter Leachate after 5 Years
The effects of separate P amendments and of simultaneous N+P additions on TOC and DON concentrations tended to be similar to those already observed in throughfall (Figure 5). Mean TOC concentrations were 15 ± 8% lower (p = 0.121) in the plots with P amendments and mean DON concentrations 16 ± 6% higher (p = 0.103) in the combined N+P treatments compared to the control, but the differences were not significant. Ca additions had no effect on TOC and DON concentrations in litter leachate, while TOC/DON ratios were narrower in the plots to which N was separately added (p = 0.004) relative to the control treatments.
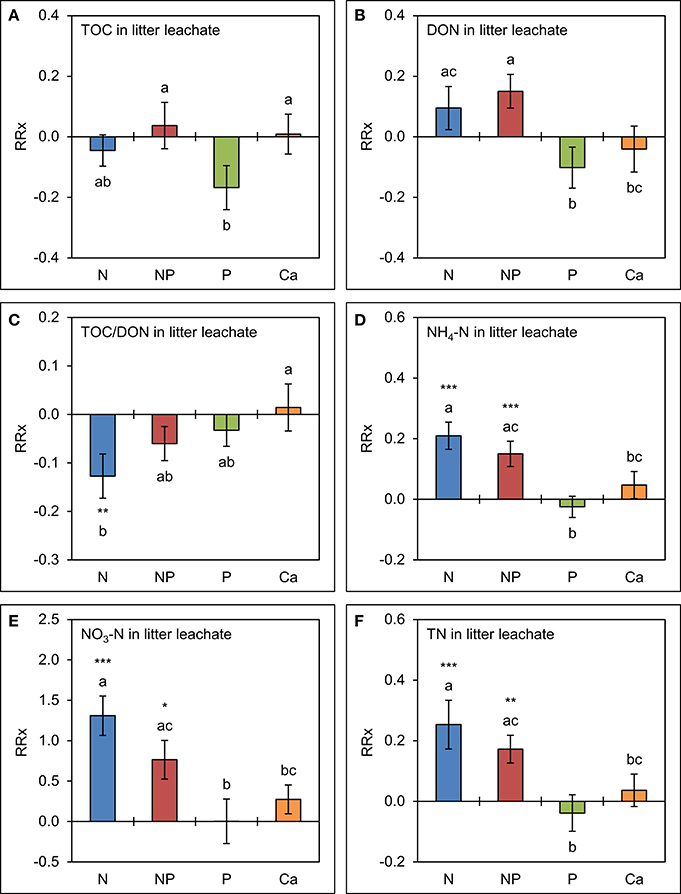
Figure 5. Cumulative effects of nutrient manipulations observed in litter leachate after 5 years of nutrient additions. The diagrams show the average responses over 5 years of (A) total organic carbon (TOC) concentrations, (B) dissolved organic nitrogen (DON) concentrations, (C) TOC/DON ratios, (D) ammonium-N (NH4-N) concentrations, (E) nitrate-N (NO3-N) concentrations, and (F) total nitrogen (TN) concentrations to nutrient additions expressed as natural log-response ratios (RRx) between the respective treatments (n = 4) and the unfertilized control plots (n = 4). Error bars indicate plus or minus one standard error. Asterisks indicate significant differences to the control (*p < 0.05, **p < 0.01, ***p < 0.001). Values not sharing a common letter are significantly different from each other (p < 0.05).
Both N and P amendments to the forest soil showed strong and significant effects on inorganic N concentrations in litter leachate compared with the control treatment. Accordingly, NH4-N concentrations were 16 ± 4% higher (p < 0.001) in response to the simultaneous N+P amendments and 23 ± 5% higher (p < 0.001) after separate N additions, while NO3-N concentrations doubled in the N+P treatments and were 3.7 times higher in the plots where N alone was added. Separate additions of P and of Ca did not increase N concentrations in litter leachate, while TN concentrations were driven by inorganic N contributions.
Concentration Time Series in the Control Plots
Temporal Course of Element Concentrations in Throughfall
The TOC concentrations in throughfall decreased by 16% in the unfertilized control plots between the first and the fifth year of NUMEX (τ = −0.248, p = 0.033). At the same time, the temporal courses of DON concentrations and of TOC/DON ratios did not show significant trends (Figure 6). DON contribution to TN decreased by 20% (Figure 7) because of increasing inorganic N richness in the canopy, which is attributed to the rising N deposition from the atmosphere in the research area (Figure 3).
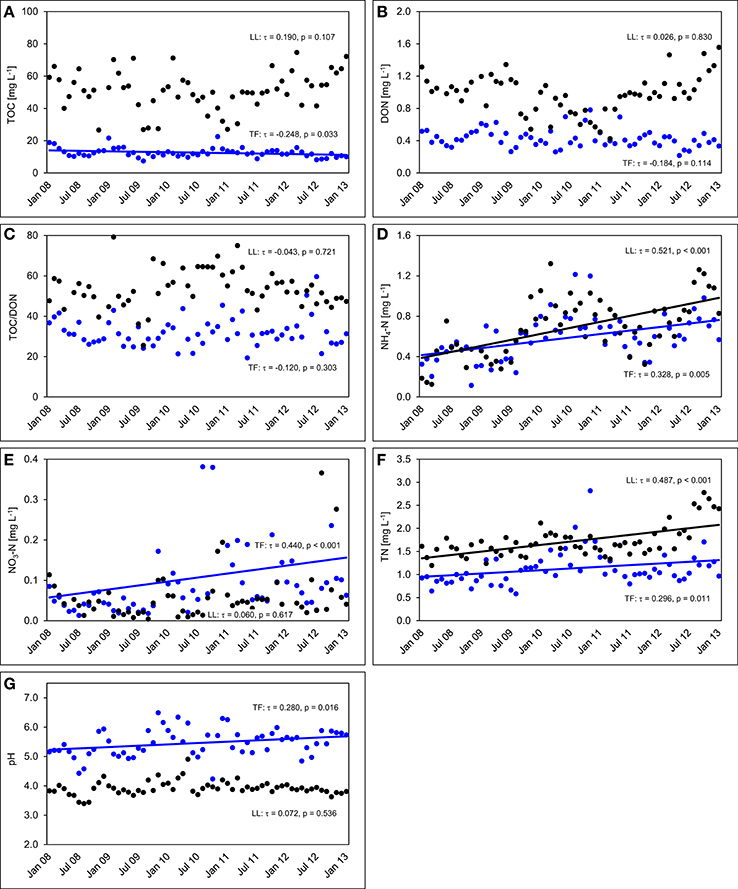
Figure 6. Concentration time series in the control plots between January 2008 and December 2012. The figures show the course of volume-weighted, mean monthly values of (A) total organic carbon (TOC) concentrations, (B) dissolved organic nitrogen (DON) concentrations, (C) TOC/DON ratios, (D) concentrations of ammonium-N (NH4-N), (E) concentrations of nitrate-N (NO3-N), (F) concentrations of total nitrogen (TN), and (G) pH values in throughfall (TF, blue), and litter leachate (LL, black) of the control plots (n = 4) of the nutrient manipulation experiment (NUMEX). Solid lines illustrate significant trends in the dataset (p < 0.05).
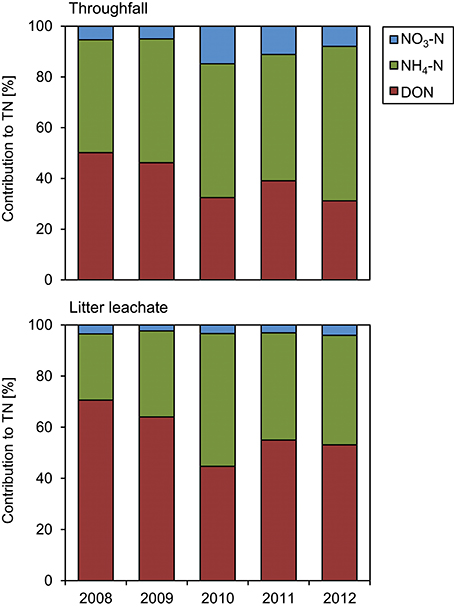
Figure 7. Contributions to total nitrogen (TN) in throughfall and litter leachate between 2008 and 2012. The diagrams show the individual contributions of dissolved organic nitrogen (DON), ammonium-N (NH4-N), and nitrate-N (NO3-N) to total nitrogen (TN) in the control plots of the nutrient manipulation experiment (NUMEX).
Concentrations of inorganic N in throughfall showed a high seasonality and doubled in 5 years (τ = 0.328, p = 0.005 for NH4-N, and τ = 0.440, p < 0.001 for NO3-N). The course of TN concentrations was driven by the strong increase in NH4-N and NO3-N concentrations over time, while the pH values of throughfall increased from 5.0 at the beginning of the experiment in 2008 to 5.4 in 2012, which represents roughly a 50% decrease of the proton concentration after 5 years (τ = −0.280, p = 0.016).
Temporal Course of Element Concentrations in Litter Leachate
In litter leachate (Figure 6), concentrations of TOC and DON showed high seasonality but no overall trend. The pH values of litter leachate did not change (τ = 0.072, p = 0.536), so that there was no acidification of the organic layer in spite of the rising N deposition mainly as , which could have been expected to favor acidification of the organic layer because of the release of protons during nitrification (Malhi et al., 1998). Similar to our observations in throughfall, concentrations of NH4-N varied seasonally and showed a strong positive trend (τ = 0.521, p < 0.001). The NH4-N concentrations of litter leachate in the control plots doubled between beginning of 2008 and end of 2012 while the NO3-N concentrations were often close to the limit of detection and displayed an increasingly higher variance to the end of the analyzed time series, yet without showing a temporal trend (τ = 0.060, p = 0.617).
The concentration ratio of NH4-N to NO3-N is approximatively 10:1, which indicates that the system is dominated by and that there is little nitrification of the NH4-N deposited from the atmosphere in the organic layer. At the same time, TN concentrations increased by 36% (τ = 0.487, p < 0.001) between the beginning and the end of the observations, which was attributable to strongly increasing NH4-N contributions to TN (26 to 44%), while DON contributions to TN dropped from 71 to 53%. NO3-N contributions remained stable at ca. 3% of TN (Figure 7). TOC/DON ratios were lower in throughfall than in litter leachate and showed a high seasonal variation but no temporal trends. In summary, there was a dynamic change of the N cycle in the untreated control plots of our study during the observation period of 5 years, which is mainly attributable to the steadily increasing N deposition from the atmosphere (Figure 3).
Effects of Nutrient Additions on Concentration Time Series
Concentration Time Series and Trends in Throughfall
In throughfall of the plots with separate N additions, TOC (τ = 0.223, p = 0.059) and DON concentrations (τ = 0.248, p = 0.033) increased over the 5 years compared with the control (Figure 8). Separate P additions did not cause a response of TOC concentrations in throughfall (τ = 0.056, p = 0.630), while separate Ca additions led to an ascending, but not significant trend (τ = 0.168, p = 0.149) of TOC concentrations in throughfall over time. Combined N+P additions had apparently no influence on the course of TOC (τ = 0.136, p = 0.243) and DON concentrations (τ = 0.056, p = 0.630) during the observed 5 years. The time series of TOC/DON ratios did not show temporal trends in any of the treatments. After a fast response in the first 2 years, the effects of nutrient additions on TOC and DON concentrations declined and almost disappeared after 5 years.
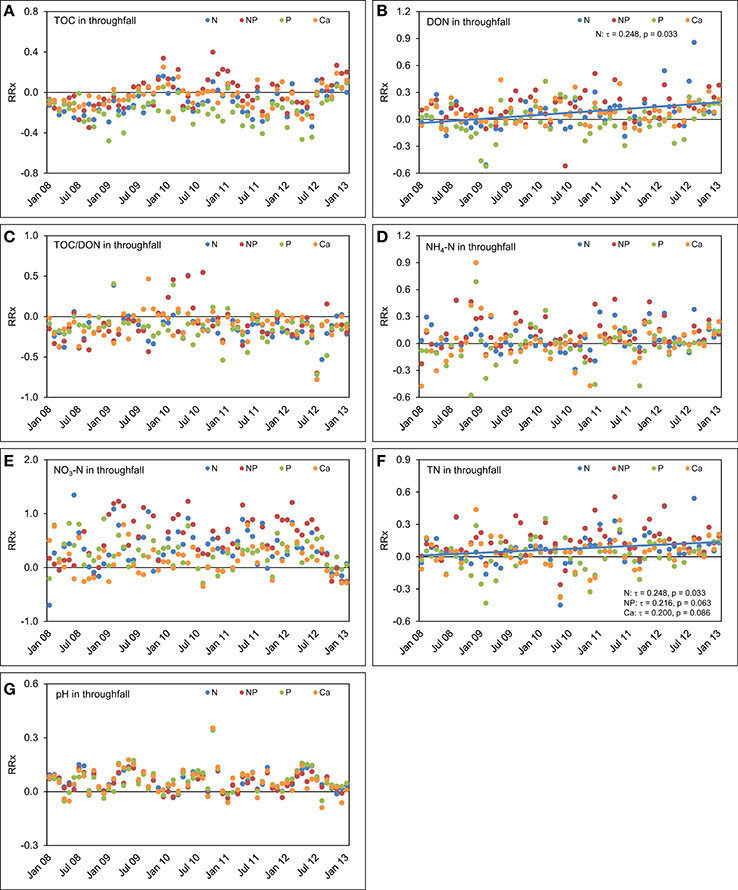
Figure 8. Concentration time series in throughfall between January 2008 and December 2012. The figures show the course of the differences between the four treatments and the control plots expressed as natural log-response ratios (RRx) of (A) total organic carbon (TOC) concentrations, (B) dissolved organic nitrogen (DON) concentrations, (C) TOC/DON ratios, (D) concentrations of ammonium-N (NH4-N), (E) concentrations of nitrate-N (NO3-N), (F) concentrations of total nitrogen (TN), and (G) pH values in throughfall of the nutrient manipulation experiment (NUMEX). Solid lines illustrate significant trends in the dataset (p < 0.05).
The concentrations of NH4-N and NO3-N in throughfall behaved similarly as in the control plots and followed the positive concentration trends described in Figure 6 without major deviations. This indicates that most of the added N is retained in the system. This finding is also supported by the positive response of TN concentration time series in throughfall of both the N (τ = 0.248, p = 0.033) and N+P treatment (τ = 0.216, p = 0.063), although the latter trend was only marginally significant. Steadily increasing TN concentrations in throughfall with time, which represent the sum of organic and inorganic N species, are the effect of a steadily increasing N richness in the canopy because of the fertilizer additions. Ca fertilization did not result in temporal trends of element concentrations in throughfall, beside a marginally significant increase in TN concentrations (τ = 0.200, p = 0.086), while the time series of the pH values in throughfall showed seasonal oscillations, but no trend compared with the control treatment.
Concentration Time Series and Trends in Litter Leachate
In litter leachate (Figure 9), we observed a strong decrease of the TOC concentrations over time as a result of P (τ = −0.322, p = 0.006) and N+P additions (τ = −0.372, p = 0.002). The response of the TOC concentrations was strongest in the time series of the combined N+P treatment (τ = −0.372, p = 0.002). We did not detect significant responses in the temporal course of TOC concentrations in litter leachate after separate addition of N. Contrary to our expectations based on findings of a coupling of Ca cycle and N leaching losses (Perakis et al., 2006), addition of Ca did not generate a response of the concentrations of N species over time. DON concentrations in litter leachate decreased in the plots with P (τ = −0.266, p = 0.030) and simultaneous N+P additions (τ = −0.385, p = 0.001), while the decrease was not significant in the plots where N alone was added (τ = −0.111, p = 0.353). Similarly to the TOC concentrations, the strongest effect on the time series of DON concentrations was observed in the combined N+P treatment.
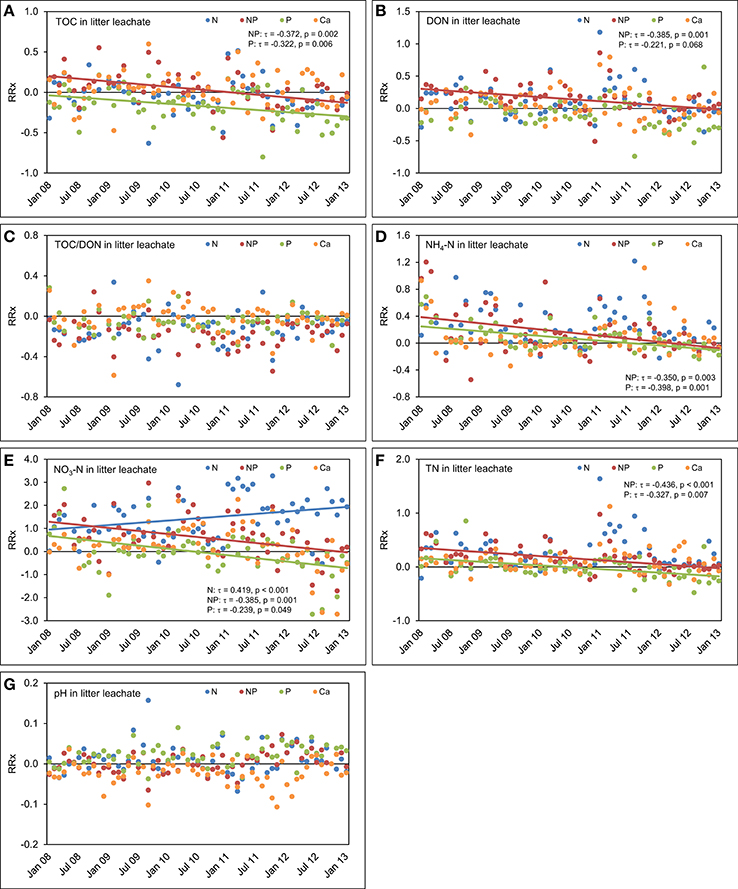
Figure 9. Concentrations time series in litter leachate between January 2008 and December 2012. The figures show the course of the differences between the four treatments and the control plots expressed as natural log-response ratios (RRx) of (A) total organic carbon (TOC) concentrations, (B) dissolved organic nitrogen (DON) concentrations, (C) TOC/DON ratios, (D) concentrations of ammonium-N (NH4-N), (E) concentrations of nitrate-N (NO3-N), (F) concentrations of total nitrogen (TN), and (G) pH values in litter leachate of the nutrient manipulation experiment (NUMEX). Solid lines illustrate significant trends in the dataset (p < 0.05).
There were no significant differences in pH values of litter leachate between the treatments and the control plots over the complete observation period, which confirms that the increased supply of N occurring as NH4-N did not acidify the organic layer during the observation period. The TOC/DON ratios in litter leachate did not significantly change with time after fertilizer additions, which was similarly observed in throughfall.
In the plots with separate N additions, NH4-N concentrations in litter leachate followed the same temporal patterns which we had already observed in the control plots, but decreased with time in the plots with separate P (τ = −0.398, p = 0.001) and combined N+P additions (τ = −0.350, p = 0.003). Furthermore, NO3-N concentrations rose in the N treatment (τ = 0.419, p < 0.001) after 2010, but decreased significantly following separate P additions (τ = −0.239, p = 0.049) and simultaneous N and P amendments (τ = −0.385, p = 0.001). This indicates a possible regulating role of P availability for the N use efficiency in the study ecosystem.
There was a fast initial positive response of N concentrations in litter leachate to P and N+P amendments. However, in the later course of the experiment, the differences to the control plots decreased (Figure 9). This suggests that after a fast increase of N concentrations in response to the N and P additions there was a lag time before the system could make use of the additionally available nitrogen.
We observed no significant effects of inorganic N concentrations in litter leachate in response to Ca additions. Once more, TN concentrations in little leachate were driven by the NH4-N concentrations, while DON contributions to TN dropped by ca. 18%, as similarly observed in the control plots (Figure 7).
Discussion
Effects of Nutrient Addition on N Concentrations in Throughfall and Litter Leachate
Five years after the establishment of NUMEX, we showed that additions of N alone or simultaneously with P generally increased annual mean N concentrations in the above-ground part of the forest water cycle (Figure 4). The absence of trends of the log response ratios of inorganic N concentrations in throughfall (Figure 8) denotes a fast response of the above-ground part of the water-bound forest N cycle to N and P additions or their combination. This confirms previous findings in the research area, where N return with throughfall was increased (Wullaert et al., 2010) and N concentrations in fine litterfall augmented (Homeier et al., 2012) after 1 year of nutrient additions, but also shows that the response to nutrient additions was highly variable during the 5 years of our observations, which is probably attributable to the variable N input from the atmosphere (Figure 3). Increasing inorganic N deposition from the atmosphere, which has already been documented by Boy et al. (2008) and Wilcke et al. (2013a) for our research area, leads to an N enrichment of the canopy, where N could be retained by the abundant epiphytes, which may establish closed nutrient cycles in the associated organic matter accumulations in the canopy (Nadkarni, 1994). Furthermore, N cycling in canopy soils of tropical montane forests was shown to be stimulated by N and P additions to the forest soil, but long-term fertilization may also lead to increased N leaching from the canopy (Matson et al., 2014). The studies of Vance and Nadkarni (1990) and Schwarz et al. (2011) have shown that mineralization of organic matter in the canopy and nitrification on leaf surfaces occur in tropical forests. Overall higher concentrations of NO3-N in throughfall of the N, P, and N+P treatments (Figure 4) denote that nutrient additions to the soil have either favored nitrification in the canopy or increased NO3-N leaching. The lack of trends in the log response ratios of NH4-N and NO3-N concentrations (Figure 8) indicates that the nitrification rates in the canopy did not change over time in response to nutrient additions. Yet, more NO3-N is produced when NH4-N supply through natural deposition or fertilization increases, although the nitrification rate remains constant. We argue that N, P, and N+P additions stimulate N uptake by the vegetation, accelerate inorganic N cycling, and increase NO3-N production in the forest canopy, which consequently leads to increased NO3-N losses with throughfall.
Reactive N species and can be easily taken up by microorganisms and plants and thus stimulate biomass production in N-limited ecosystems (Janssens et al., 2010). It was also shown, however, that NO3-N losses occurred immediately after the first N addition in a lowland tropical forest thought to be N limited (Lohse and Matson, 2005). N and N+P amendments resulted in overall higher inorganic N losses from the forest floor, but showed opposite temporal trends (Figure 9). The negative trends of NH4-N and NO3-N concentrations in litter leachate denote that P amendments alone or in combination with N reduced initially increased inorganic N leaching from the organic layer over time back to the levels of the control or to even slightly lower levels. While separate P additions did not generate a different response of the annual mean N concentrations from the control treatment (Figure 5), the trend analysis revealed that increased P availability leads to a higher N retention in the root zone (Figure 9), which denotes a potential P limitation of plants and micro-organisms. Thus, added N could be efficiently used by plants and the microbial community only if P availability was also improved.
When N demands of microbes and plants are exceeded, N leaching from the ecosystem may be further enhanced by long-term availability of excessive N (Law, 2013). Since, it is known that nitrification occurs even at very low pH values in tropical forest soils (Robertson, 1982), rising NO3-N concentrations with time may also indicate increased nitrification because of drier microclimatic conditions in the organic layer. Accordingly, the increasing NO3-N leaching which we observed in the plots with separate N additions indicates a shift toward a more open N cycle, where N alone is not a limiting nutrient and, once present in excess, it is removed from the system primarily in NO3-N form (Matson et al., 1999).
We expected that Ca additions would generate a similar response of the N cycle as the addition of N, as observed by Perakis et al. (2006) in forest ecosystems developed under maritime climate on the Oregon Coast Range. However, we only found a marginally significant positive trend of TN concentrations in throughfall in response to Ca amendments (Figure 9). We speculate that the highly variable natural deposition of Ca during the 5 years of our study obscured the expected fertilization effects of Ca additions on N concentrations, or annual addition rates of Ca have been too low to trigger significant responses. Consequently, our hypothesis of a possible coupling of Ca addition and N concentrations in the studied forest cannot be confirmed.
Effect of Nutrient Addition on TOC and DON Concentrations in Throughfall and Litter Leachate
Annual mean TOC and DON concentrations in throughfall responded weakly to nutrient amendments. The overall differences from the control treatments were not significant (Figure 4) and only DON concentrations revealed a temporal trend in throughfall during the observation period (Figure 8). The absence of overall effects and the slow change over time indicate that TOC exports from the canopy were not influenced by increased nutrient availability. We attribute increasing DON concentrations with throughfall over time to the overall higher availability of reactive N in the canopy which stimulates biomass production (Janssens et al., 2010) and hence indirectly also production of DOM. Hydrophilic DOM contains more N (Qualls and Haines, 1991), is typically mobile (Kaiser and Zech, 1998) and can thus be directly leached to the forest floor. Gaige et al. (2007) also reported higher DON exports with through fall as a result of direct N additions to the canopy. Additionally, DON can be first immobilized by microorganisms in the phyllosphere through biotic processes (Stadler and Müller, 2000) and subsequently leached from the surface of the leaves. This would explain the increasingly higher TN concentrations in the N treatments (Figure 8).
In litter leachate, the overall response of TOC and DON concentrations was similar to their response in throughfall (Figure 5). However, litter leachate became poorer in TOC and DON with time since the start of the experiment if P was added alone or in combination with N, while N additions did not generate a trend in the response of TOC or DON concentrations (Figure 9). A possible reason for decreasing TOC and DON concentrations in litter leachate could be lower pH values resulting in increasing protonation of the DOM, thus reducing its solubility (Curtin et al., 1998). However, acidity did not increase in litter leachate in response to nutrient amendments, possibly as a consequence of generally low nitrification in the organic layer, which has already been reported by Martinson et al. (2012) for our study area. This is consistent with our trend analysis showing that N originating from atmospheric deposition was increasingly leached as in the litter leachate of the control plots (Figure 6).
Moreover, increasing air temperature and unevenly distributed rainfall have allowed for longer dry spells, leading to reduced air and soil humidity in our study area (Peters et al., 2013; Wilcke et al., 2013a), which likely favored mineralization of DOM by microorganisms and led, consequently, to lower TOC and DON concentrations in litter leachate. Similar experiments in tropical forests showed that microbial mineralization of DOM was stimulated by the increased P content of the DOM (Cleveland et al., 2006). Homeier et al. (2012) have already shown that the P pool of the south Ecuadorian tropical montane forest increased in the organic layer after combined addition of N and P. We demonstrate that concurrent improvement of both N and P availability increases the mineralization of TOC and DON in the organic layer likely by stimulating the activity of microorganisms which, in turn, mineralize more DOM. Since this was true only if P was simultaneously added, we conclude that P demands of microorganisms in the organic layer are not completely met.
Our study confirms the importance of the relationships between nutrient supply, leaching, and demands of organisms (Townsend et al., 2011). Because the strongest negative trends of TOC and DON concentrations in litter leachate always appeared in the N+P treatment (Figure 9), this denotes that plants and microbial community can more efficiently use the available N only as a result of the improved P supply. These observations are in our view a strong indication that microbial activity in the organic layer of the studied tropical montane forest ecosystem is co-limited by N and P. This finding is also supported by the apparent accumulation of N in the organic layer at the beginning of the experiment, which is later increasingly leached as in the plots with separate N amendments (Figure 9). Delayed NO3-N losses have already been observed in the soils of a lowland wet forest in Hawaii as a consequence of a high anion exchange capacity (Lohse and Matson, 2005). We argue therefore that availability of P in the organic layer (e.g., through P deposition with Saharan dust) will play a key role in DOM mineralization in the long run by accelerating TOC and DON turnover in the organic layer.
We expected that nutrient additions would result in wider TOC/DON ratios, because of the preferential mineralization of the more hydrophilic, polar fraction of DOM, but our results contradicted our hypothesis and indicated strong opposite effects for all treatments in throughfall (Figure 4) and weak opposite effects in litter leachate (Figure 5). Narrower TOC/DON ratios may result from (a) an increased DON richness in the canopy and in the organic layer in the case of separate N and combined N+P additions and (b) a reduction of TOC concentrations in response to separate P additions. This may be attributed to a higher phyllosphere activity leading to increased TOC consumption as a consequence of the expected N fertilization effect on canopy and soil microorganisms. Plants and microorganisms can directly assimilate low molecular weight amino acids from DON (Neff et al., 2003), but rising availability of easily assimilable inorganic N species—mainly in litter leachate of the plots with separate N additions—may have diminished the demand of plants and microorganisms for DON, which is instead leached to the mineral soil, leading to narrower TOC/DON ratios in litter leachate.
Conclusions
Our results demonstrate that N additions alone or in combination with P generally increased mean N concentrations in the above-ground part of the forest water cycle and led to an enrichment of the inorganic species and in throughfall. Total N concentrations in throughfall increased with time only in the N treatment, indicating rising N richness in the canopy and suggesting a potential P limitation of canopy organisms. Nitrogen additions alone led to rising NO3-N exports from the organic layer, while separate P and combined N+P amendments reduced NH4-N and NO3-N leaching from the organic layer with time. We conclude that increased P availability stimulates N uptake by plants and immobilization by microorganisms, thus reducing N losses from the ecosystem. However, we did not observe evidence of the coupling of Ca and N concentrations in the studied forest ecosystem.
Separate N additions generated a steady increase of DON concentrations in throughfall. Interestingly, TOC exports from the canopy were not affected by nutrient additions. In the organic layer, separate additions of P and simultaneous additions of N and P stimulated mineralization of TOC and DON, while there was no significant response to separate additions of N. In absence of acidification of the litter leachate following N additions, we conclude that the availability of P plays a key role in the mineralization of TOC and DON by accelerating DOM turnover in the organic layer. Our findings contradicted the hypothesis that nutrient additions would lead to wider TOC/DON ratios over time. Rising availability of inorganic N species favored DON leaching to the mineral soil, leading thus to narrower TOC/DON ratios in litter leachate.
Increased supply of P—alone or together with N—will lead to a more efficient use of available N by microorganisms and plants and hence to reduced N concentrations in solutions originating from the organic layer in the long run. Thus, TOC and DON cycling will only change if the availability of N and P is concurrently improved, while NO3-N leaching from the organic layer will further rise if the N supply alone increases.
Author Contributions
All authors listed, have made substantial, direct and intellectual contribution to the work, and approved it for publication.
Conflict of Interest Statement
The authors declare that the research was conducted in the absence of any commercial or financial relationships that could be construed as a potential conflict of interest.
Acknowledgments
We thank particularly Jürgen Homeier and Hans Wullaert for the initial establishment of NUMEX, Arthur Broadbent and Hannes Thomasch for their valuable contribution during the field work in Ecuador and to the successful accomplishment of the chemical analyses in Bern and José Luis Peña Caivinagua for his support as a field technician. We thank the Deutsche Forschungsgemeinschaft (DFG) for funding the different phases of our projects within the research units FOR 402 and FOR 816. Furthermore, we thank Naturaleza y Cultura Internacional (NCI) in Loja for providing access to the study area and to the research station ECSF and the Ecuadorian Ministry of Environment for the research permits.
References
Adamek, M., Corre, M. D., and Hölscher, D. (2009). Early effect of elevated nitrogen input on above-ground net primary production of a lower montane rain forest, Panama. J. Trop. Ecol. 25, 637–647. doi: 10.1017/s0266467409990253
Arnold, J., Corre, M. D., and Veldkamp, E. (2009). Soil N cycling in oldgrowth forests across an Andosol toposequence in Ecuador. Forest Ecol. Manag. 257, 2079–2087. doi: 10.1016/j.foreco.2009.02.014
Baldos, A. P., Corre, M. D., and Veldkamp, E. (2015). Response of N cycling to nutrient inputs in forest soils across a 1000–3000 m elevation gradient in the Ecuadorian Andes. Ecology 96, 749–761. doi: 10.1890/14-0295.1
Barthlott, W., Mutke, J., Rafiqpoor, M. D., Kier, G., and Kreft, H. (2005). Global centres of vascular plant diversity. Nova Acta Leopoldina 92, 342, 61–83.
Bates, D., Maechler, M., Bolker, B., and Walker, S. (2015). Fitting linear mixed-effects models using lme4. J. Stat. Soft. 67, 1–48 doi: 10.18637/jss.v067.i01
Beer, C., Reichstein, M., Tomelleri, E., Ciais, P., Jung, M., Carvalhais, N., et al. (2010). Terrestrial gross carbon dioxide uptake: Global distribution and covariation with climate. Science 329, 834–838. doi: 10.1126/science.1184984
Bolan, N. S., Adriano, D. C., Kunhikrishnan, A., James, T., McDowell, R., and Senesi, N. (2011). “Chapter one – Dissolved organic matter: Biogeochemistry, dynamics, and environmental significance in soils,” in Advances in Agronomy Vol. 110, ed D. L. Sparks (San Diego: Elsevier Academic Press), 1–75. doi: 10.1016/B978-0-12-385531-2.00001-3
Borken, W., Ahrens, B., Schulz, C., and Zimmermann, L. (2011). Site-to-site variability and temporal trends of DOC concentrations and fluxes in temperate forest soils. Glob. Change Biol. 17, 2428–2443. doi: 10.1111/j.1365-2486.2011.02390.x
Boy, J., Rollenbeck, R., Valarezo, C., and Wilcke, W. (2008). Amazonian biomass burning-derived acid and nutrient deposition in the north Andean montane forest of Ecuador. Glob. Biogeochem. Cycles 22, GB4011. doi: 10.1029/2007GB003158
Boy, J., and Wilcke, W. (2008). Tropical Andean forest derives calcium and magnesium from Saharan dust. Glob. Biogeochem. Cycles 22, GB1027. doi: 10.1029/2007GB002960
Bragazza, L., Freeman, C., Jones, T., Rydin, H., Limpens, J., Fenner, N., et al. (2006). Atmospheric nitrogen deposition promotes carbon loss from peat bogs. Proc. Natl. Acad. Sci. U.S.A. 103, 19386–19389. doi: 10.1073/pnas.0606629104
Bruijnzeel, L. A., and Hamilton, L. S. (2000). Decision Time for Cloud Forests. IHP Humid Tropics Programme Series 13, Paris: IHP - UNESCO.
Cleveland, C. C., Reed, S. C., and Townsend, A. R. (2006). Nutrient regulation of organic matter decomposition in a tropical rain forest. Ecology 87, 492–503. doi: 10.1890/05-0525
Corre, M. D., Veldkamp, E., Arnold, J., and Wright, S. J. (2010). Impact of elevated N input on soil N cycling and losses in old-growth lowland and montane forests in Panama. Ecology 91, 1715–1929. doi: 10.1890/09-0274.1
Curtin, D., Campbell, C. A., and Jalil, A. (1998). Effects of acidity on mineralization: pH-dependence of organic matter mineralization in weakly acidic soils. Soil Biol. Biochem. 30, 57–64. doi: 10.1016/s0038-0717(97)00094-1
Da Rocha, G. O., Allen, A. G., and Cardoso, A. A. (2005). Influence of agricultural biomass burning on aerosol size distribution and dry deposition in southeastern Brazil. Environ. Sci. Technol. 39, 5293–5301. doi: 10.1021/es048007u
Elser, J. J., Bracken, M. E. S., Cleland, E. E., Gruner, D. S., Harpole, W. S., Hillebrand, H., et al. (2007). Global analysis of nitrogen and phosphorus limitation of primary producers in freshwater, marine and terrestrial ecosystems. Ecol. Lett. 10, 1135–1142. doi: 10.1111/j.1461-0248.2007.01113.x
Erisman, J. W., Sutton, M. A., Galloway, J., Klimont, Z., and Winiwarter, W. (2008). How a century of ammonia synthesis changed the world. Nat. Geosci. 1, 636–639. doi: 10.1038/ngeo325
Evans, C. D., Goodale, C. L., and Caporn, S. J. M. (2008). Does elevated nitrogen deposition or ecosystem recovery from acidification drive increased dissolved organic carbon loss from upland soil? A review of evidence from field nitrogen addition experiments. Biogeochemistry 91, 13–35. doi: 10.1007/s10533-008-9256-x
Fang, Y., Gundersen, P., Mo, J., and Zhu, W. (2009). Nitrogen leaching in response to increased nitrogen inputs in subtropical monsoon forests in southern China. Forest Ecol. Manag. 257, 332–342. doi: 10.1016/j.foreco.2008.09.004
Fay, P. A., Prober, S. M., Harpole, W. S., Knops, J. M. H., Bakker, J. D., Borer, E. T., et al. (2015). Grassland productivity limited by multiple nutrients. Nat. Plants 1, 15080. doi: 10.1038/nplants.2015.80
Fisher, J. B., Malhi, Y., Torres, I. C., Metcalfe, D. B., van de Weg, M. J., Meir, P., et al. (2013). Nutrient limitation in rainforests and cloud forests along a 3,000-m elevation gradient in the Peruvian Andes. Oecologia 172, 889–902. doi: 10.1007/s00442-012-2522-6
Fleischbein, K., Wilcke, W., Goller, R., Boy, J., Valarezo, C., Zech, W., et al. (2005). Rainfall interception in a lower montane forest in Ecuador: effects of canopy properties. Hydrol. Proc. 19, 1355–1371. doi: 10.1002/hyp.5562
Fleischbein, K., Wilcke, W., Valarezo, C., Zech, W., and Knoblich, K. (2006). Water budgets of three small catchments under montane forest in Ecuador: experimental and modelling approach. Hydrol. Proc. 20, 2491–2507. doi: 10.1002/hyp.6212
Gaige, E., Dail, D., Hollinger, D., Davidson, E., Fernandez, I., Sievering, H., et al. (2007). Changes in canopy processes following whole-forest canopy nitrogen fertilization of a mature spruce-hemlock forest. Ecosystems 10, 1133–1147. doi: 10.1007/s10021-007-9081-4
Galloway, J. N., Dentener, F. J., Capone, D. G., Boyer, E. W., Howarth, R. W., Seitzinger, S. P., et al. (2004). Nitrogen cycles: past, present, and future. Biogeochemistry 70, 153–226. doi: 10.1007/s10533-004-0370-0
Galloway, J. N., Townsend, A. R., Erisman, J. W., Bekunda, M., Cai, Z., Freney, J. R., et al. (2008). Transformation of the nitrogen cycle: recent trends, questions, and potential solutions. Science 320, 889–892. doi: 10.1126/science.1136674
Goller, R., Wilcke, W., Fleischbein, K., Valarezo, C., and Zech, W. (2006). Dissolved inorganic nitrogen, phosphorus, and sulfur in the nutrient cycle of a montane forest in Ecuador. Biogeochemistry 77, 57–89. doi: 10.1007/s10533-005-1061-1
Helsel, D. R., and Hirsch, R. M. (2002). “Statistical methods in water resources,” in Techniques of Water-Resources Investigations. Book 4, Hydrologic Analysis and Interpretation, Chapter A3 (Reston, VA: U.S. Geological Survey).
Hietz, P., Turner, B. L., Wanek, W., Richter, A., Nock, C. A., and Wright, S. J. (2011). Long-term change in the nitrogen cycle of tropical forests. Science 334, 664–666. doi: 10.1126/science.1211979
Hirsch, R. M., Slack, J. R., and Smith, R. A. (1982). Techniques for trend analysis for monthly water quality data. Water Resour. Res. 18, 107–121. doi: 10.1029/wr018i001p00107
Hobbie, S. E., and Vitousek, P. M. (2000). Nutrient limitation of decomposition in Hawaiian forests. Ecology 81, 1867–1877. doi: 10.2307/177277
Homeier, J., Hertel, D., Camenzind, T., Cumbicus, N. L., Maraun, M., Martinson, et al. (2012). Tropical Andean forests are highly susceptible to nutrient inputs – rapid effects of experimental N and P addition to an Ecuadorian montane forest. PLoS ONE 7:e47128. doi: 10.1371/journal.pone.0047128
Homeier, J., and Werner, F. (2007). Spermatophyta. Checklist of the Reserva Biológica San Francisco (Prov. Zamora-Chinchipe, S-Ecuador). Ecotropical Monogr. 4, 15–58.
Homeier, J., Werner, F., Gradstein, R., Breckle, S. W., and Richter, M. (2008). “Potential vegetation and floristic composition of Andean forests in South Ecuador, with a focus on the RBSF,” in Gradients in a Tropical Mountain Ecosystem of Ecuador, eds E. Beck, J. Bendix, I. Kottke, F. Makeschin, and R. Mosandl (Berlin; Heidelberg: Springer), 87–100. doi: 10.1007/978-3-540-73526-7_10
Hothorn, T., Bretz, F., and Westfall, P. (2008). Simultaneous Inference in General Parametric Models. Biometrical J. 50, 346–363. doi: 10.1002/bimj.200810425
Hungerbühler, D. (1997). Neogene Basins in the Andes of Southern Ecuador: Evolution, Deformation and Regional Tectonic Implications. Ph.D. Thesis. Eidgenössische Technische Hochschule, Zürich.
IUSS Working Group WRB (2006). World Reference base for Soil Resources 2006. 2nd Edn., World Soil Resources Reports 103. (Rome: FAO).
Janssens, I. A., Dieleman, W., Luyssaert, S., Subke, J. A., Reichstein, M., Ceulemans, R., et al. (2010). Reduction of forest soil respiration in response to nitrogen deposition. Nat. Geosci. 3, 315–322. doi: 10.1038/ngeo844
Jobbagy, E., and Jackson, R. (2000). The vertical distribution of soil organic carbon and its relation to climate and vegetation. Ecol. Appl. 10, 423–436. doi: 10.1890/1051-0761(2000)010[0423:tvdoso]2.0.co;2
Kaiser, K., and Zech, W. (1998). Soil dissolved organic matter sorption as influenced by organic and sesquioxides coatings and sorbed sulfate. Soil Sci. Soc. Am. J. 62, 129–136. doi: 10.2136/sssaj1998.03615995006200010017x
Kalbitz, K., and Kaiser, K. (2008). Contribution of dissolved organic matter to carbon storage in forest mineral soils. J. Plant Nutr. Soil Sci. 171, 52–60. doi: 10.1002/jpln.200700043
Kalbitz, K., Solinger, S., Park, J. H., Michalzik, B., and Matzner, E. (2000). Controls on the dynamics of dissolved organic matter in soils: a review. Soil Sci. 165, 277–304. doi: 10.1097/00010694-200004000-00001
Kaspari, M., Garcia, M. N., Harms, K. E., Santana, M., Wright, S. J., and Yavitt, J. B. (2008). Multiple nutrients limit litterfall and decomposition in a tropical forest. Ecol. Lett. 11, 35–43. doi: 10.1111/j.1461-0248.2007.01124.x
Kaufman, Y. J., Koren, I., Remer, L. A., Tanre, D., Ginoux, P., and Fan, S. (2005). Dust transport and deposition observed from the Terra-MODIS spacecraft over the Atlantic Ocean. J. Geophys. Res. 110, D10S12. doi: 10.1029/2003JD004436
LeBauer, D. S., and Treseder, K. K. (2008). Nitrogen limitation of net primary productivity in terrestrial ecosystems is globally distributed. Ecology 89, 379–379. doi: 10.1890/06-2057.1
Lohse, K. A., and Matson, P. (2005). Consequences of nitrogen additions for soil losses from wet tropical forests. Ecol. Appl. 15, 1629–1648. doi: 10.1890/03-5421
Malhi, S., Nyborg, M., and Harapiak, J. (1998). Effects of long-term N fertilizer-induced acidification and liming on micronutrients in soil and in bromegrass hay. Soil Till. Res. 48, 91–101. doi: 10.1016/s0167-1987(98)00097-x
Martinson, G. O., Corre, M. D., and Veldkamp, E. (2012). Responses of nitrous oxide fluxes and soil nitrogen cycling to nutrient additions in montane forests along an elevation gradient in southern Ecuador. Biogeochemistry 112, 625–636. doi: 10.1007/s10533-012-9753-9
Martinson, G. O., Werner, F. A., Scherber, C., Conrad, R., Veldkamp, E., Flessa, H., et al. (2010). Tank Bromeliads: methane producing ‘canopy wetlands’ in neotropical forests. Nat. Geosci. 3, 766–769. doi: 10.1038/ngeo980
Matson, A. L., Corre, M. D., and Veldkamp, E. (2014). Nitrogen cycling in canopy soils of tropical montane forests responds rapidly to indirect N and P fertilization. Glob. Change Biol. 20, 3802–3813, doi: 10.1111/gcb.12668
Matson, P. A., McDowell, W. H., Townsend, A. R., and Vitousek, P. M. (1999). The globalization of N deposition: Ecosystem consequences in tropical environments, Biogeochemistry 46, 67–83. doi: 10.1023/A:1006152112852
McLeod, A. I. (2011). Kendall: Kendall rank correlation and Mann-Kendall trend test. R package version 2.2. Available online at: https://cran.r-project.org/web/packages/Kendall/Kendall.pdf
Michalzik, B., Kalbitz, B., Park, J.-H., Solinger, S., and Matzner, E. (2001). Fluxes and concentrations of dissolved organic carbon and nitrogen – a synthesis for temperate forests. Biogeochemistry 52, 173–205. doi: 10.1023/A:1006441620810
Myers, N., Mittermeier, C. G., da Fonseca, G. A. B., and Kent, J. (2000). Biodiversity hotspots for conservation priorities. Nature 403, 853–858. doi: 10.1038/35002501
Nadkarni, N. M. (1994). Factors affecting the initiation and growth of aboveground adventitious roots in a tropical cloud forest tree: an experimental approach. Oecologia 100–100, 94–97. doi: 10.1007/bf00317135
Neff, J. C., Chapin, F. S., and Vitousek, P. M. (2003). Breaks in the cycle: dissolved organic nitrogen in terrestrial ecosystems. Front. Ecol. Environ. 1:205. doi: 10.1890/1540-9295(2003)001[0205:BITCDO]2.0.CO;2
Neff, J. C., Townsend, A. R., Gleixner, G., Lehman, S. J., Turnbull, J., and Bowman, W. D. (2002). Variable effects of nitrogen additions on the stability and turnover of soil carbon. Nature 419, 915–917. doi: 10.1038/nature01136
Perakis, S. S., Maguire, D. A., Bullen, T. D., Cromack, K., Waring, R. H., and Boyle, J. R. (2006). Coupled nitrogen and calcium cycles in forests of the oregon coast range. Ecosystems 9, 63–74. doi: 10.1007/s10021-004-0039-5
Peters, T., Drobnik, T., Meyer, H., Rankl, M., Richter, M., Rollenbeck, R., et al. (2013). “Environmental changes affecting the Andes of Ecuador,” in Ecosystem Services, Biodiversity and Environmental Change in a Tropical Mountain Ecosystem of South Ecuador, eds J. Bendix, E. Beck, A. Bräuning, F. Makeschin, R. Mosandl, S. Scheu, and W. Wilcke (Berlin; Heidelberg: Springer), 19–29. doi: 10.1007/978-3-642-38137-9_2
Pett-Ridge, J. C. (2009). Contributions of dust to phosphorus cycling in tropical forests of the Luquillo Mountains, Puerto Rico. Biogeochemistry 94, 63–80. doi: 10.1007/s10533-009-9308-x
Phoenix, G. K., Hicks, W. K., Cinderby, S., Kuylenstierna, J. C. I., Stock, W. D., Dentener, F. J., et al. (2006). Atmospheric nitrogen deposition in world biodiversity hotspots: the need for a greater global perspective in assessing N deposition impacts, Global Change Biol. 12, 470–476. doi: 10.1111/j.1365-2486.2006.01104.x
Qualls, R., and Haines, B. L. (1991). Geochemistry of dissolved organic nutrients in water percolating through a forest ecosystem. Soil Sci. Soc. Am. J. 55. 1112–1123. doi: 10.2136/sssaj1991.03615995005500040036x
R Core Team (2013). R: A Language and Environment for Statistical Computing. R Foundation for Statistical Computing. Vienna, Austria. Available online at: http://www.R-project.org
Robertson, G. P. (1982). Nitrification in Forested Ecosystems. Philos. Trans. R. Soc. Lond. B 296, 445–457. doi: 10.1098/rstb.1982.0019
Rollenbeck, R., Peters, T., Emck, P., and Richter, M. (2015). ECSF_Climate Station Data Best Estimate ver. 1. Available online from DFG-FOR816dw. http://www.tropicalmountainforest.org/data_pre.do?citid=1382
Roman, L., Scatena, F. N., and Bruijnzeel, L. A. (2010). “Chapter 6: Global and local variations in tropical montane cloud forest soils,” in Tropical Montane Cloud Forest, International Hydrology Series, eds L. A. Bruijnzeel, F. N. Scatena, and L. S. Hamilton (Cambridge: Cambridge University Press), 77–89. doi: 10.1017/cbo9780511778384.008
Schimel, D., Stephens, B. B., and Fisher, J. B. (2014). Effect of increasing CO2 on the terrestrial carbon cycle. Proc. Natl. Acad. Sci. U.S.A. 112, 436–441. doi: 10.1073/pnas.1407302112
Schwarz, M. T., Oelmann, Y., and Wilcke, W. (2011). Stable N isotope composition of nitrate reflects N transformations during the passage of water through a montane rain forest in Ecuador. Biogeochemistry 102, 195–208, doi: 10.1007/s10533-010-9434-5
Stadler, B., and Müller, T. (2000). Effects of aphids and moth caterpillars on epiphytic microorganisms in canopies of forest trees. Can. J. For. Res. 30, 631–638. doi: 10.1139/x99-253
Tanner, E. V. J., Kapos, V., and Franco, W. (1992). Nitrogen and phosphorus fertilization effects on Venezuelan montane forest trunk growth and litterfall. Ecology 73, 78–86. doi: 10.2307/1938722
Tanner, E. V. J., Kapos, V., Freskos, S., Healey, J. R., and Theobald, A. M. (1990). Nitrogen and phosphorus fertilization of Jamaican montane forest trees. J. Trop. Ecol. 6, 231–238. doi: 10.1017/s0266467400004375
Tanner, E. V. J., Vitousek, P. M., and Cuevas, E. (1998). Experimental investigation of nutrient limitation of forest growth on wet tropical mountains. Ecology 79, 10–22. doi: 10.2307/176860
Townsend, A. R., Cleveland, C. C., Houlton, B. Z., Alden, C. B., and White, J. W. (2011). Multi-element regulation of the tropical forest carbon cycle. Front. Ecol. Environ. 9:47. doi: 10.1890/100047
Turner, B. L., Yavitt, J. B., Harms, K. E., Garcia, M. N., Romero, T. E., and Wright, S. J. (2013). Seasonal changes and treatment effects on soil inorganic nutrients following a decade of fertilizer addition in a lowland tropical forest. Soil Sci. Soc. Am. J. 77, 1357. doi: 10.2136/sssaj2012.0128
Urrutia, R., and Vuille, M. (2009). Climate change projections for the tropical Andes using a regional climate model: temperature and precipitation simulations for the end of the 21st century, J. Geophys. Res. 114, D02108. doi: 10.1029/2008JD011021
Van Hees, P. A. W., Jones, D. L., Finlay, R., Godbold, D. L., and Lundström, U. S. (2005). The carbon we do not see – the impact of low molecular weight compounds on carbon dynamics and respiration in forest soils: a review. Soil Biol.Biochem. 37, 1–13. doi: 10.1016/j.soilbio.2004.06.010
Vance, E. D., and Nadkarni, N. M. (1990). Microbial biomass and activity in canopy organic-matter and the forest floor of a tropical cloud forest. Soil Biol. Biochem. 22, 677–684. doi: 10.1016/0038-0717(90)90015-r
Vitousek, P. M., and Howarth, R. W. (1991). Nitrogen limitation on land and in the sea – how can it occur? Biogeochemistry 13, 87–115. doi: 10.1007/bf00002772
Vitousek, P. M., Porder, S., Houlton, B. Z., and Chadwick, O. A. (2010). Terrestrial phosphorus limitation: mechanisms, implications, and nitrogen–phosphorus interactions. Ecol. Appl. 20, 5–15. doi: 10.1890/08-0127.1
Vitousek, P. M., Walker, L. R., Whiteaker, L. D., and Matson, P. A. (1993). Nutrient limitations to plant-growth during primary succession in Hawaii-volcanos-national-park. Biogeochemistry 23, 197–215. doi: 10.1007/bf00023752
Vitousek, P. M. (1984). Litterfall, nutrient cycling, and nutrient limitation in tropical forests. Ecology 65, 285. doi: 10.2307/1939481
Vuille, M., Bradley, R. S., Werner, M., and Keimig, F. (2003). 20th century climate change in the tropical Andes: observations and model results. Clim. Change 59, 75–99. doi: 10.1023/A:1024406427519
Walker, R., DeFries, R., Del Carmen, M., Diaz, V., Shimabukuro, Y. E., and Venturieri, A. (2009). “The expansion of intensive agriculture and ranching in Brazilian Amazonia,” in Amazonia and Global Change, Vol. 186, eds J. C. H. Gash, M. Keller, M. Bustamante, and P. S. Dias (Washington, DC: American Geophysical Union), 61–81.
Walker, T., and Syers, J. (1976). The fate of phosphorus during pedogenesis. Geoderma 15, 1–19. doi: 10.1016/0016-7061(76)90066-5
Wilcke, W., Leimer, S., Peters, T., Emck, P., Rollenbeck, R., Trachte, K., et al. (2013a). The nitrogen cycle of tropical montane forest in Ecuador turns inorganic under environmental change. Global Biogeochem. Cycles 27, 1194–1204. doi: 10.1002/2012GB004471
Wilcke, W., Boy, J., Hamer, U., Potthast, K., Rollenbeck, R., and Valarezo, C. (2013b). “Current regulating and supporting services: nutrient cycles,” in Ecosystem Services, Biodiversity and Environmental Change in a Tropical Mountain Ecosystem of South Ecuador, eds J. Bendix, E. Beck, A. Bräuning, F. Makeschin, R. Mosandl, S. Scheu, and W. Wilcke (Berlin; Heidelberg: Springer), 141–151. doi: 10.1007/978-3-642-38137-9_11
Wilcke, W., Oelmann, Y., Schmitt, A., Valarezo, C., Zech, W., and Homeier, J. (2008). Soil properties and tree growth along an altitudinal transect in Ecuadorian tropical montane forest. J. Plant Nutr. Soil Sci. 171, 220–230, doi: 10.1002/jpln.200625210
Wilcke, W., Yasin, S., Abramowski, U., Valarezo, C., and Zech, W. (2002). Nutrient storage and turnover in organic layers under tropical montane rain forest in Ecuador. Eur. J. Soil Sci. 53, 15–27. doi: 10.1046/j.1365-2389.2002.00411.x
Wright, S. J., Yavitt, J. B., Wurzburger, N., Turner, B. L., Tanner, E. V. J., Sayer, E. J., et al. (2011). Potassium, phosphorus, or nitrogen limit root allocation, tree growth, or litter production in a lowland tropical forest. Ecology 92, 1616–1625. doi: 10.1890/10-1558.1
Wullaert, H., Homeier, J., Valarezo, C., and Wilcke, W. (2010). Response of the N and P cycles of an old-growth montane forest in Ecuador to experimental low-level N and P amendments. Forest Ecol. Manag. 260, 1434–1445. doi: 10.1016/j.foreco.2010.07.021
Keywords: nutrient manipulation experiment, nitrogen (N) phosphorus (P) and calcium (Ca) additions, total organic carbon (TOC), dissolved organic nitrogen (DON), nitrate leaching, tropical montane forest, Ecuador
Citation: Velescu A, Valarezo C and Wilcke W (2016) Response of Dissolved Carbon and Nitrogen Concentrations to Moderate Nutrient Additions in a Tropical Montane Forest of South Ecuador. Front. Earth Sci. 4:58. doi: 10.3389/feart.2016.00058
Received: 09 December 2016; Accepted: 26 April 2016;
Published: 23 May 2016.
Edited by:
Dietrich Hertel, Georg-August-Universität Göttingen, GermanyReviewed by:
Ute Hamer, Westfälische Wilhelms-Universität Münster, GermanyFelix Heitkamp, Georg-August-Universität Göttingen, Germany
Copyright © 2016 Velescu, Valarezo and Wilcke. This is an open-access article distributed under the terms of the Creative Commons Attribution License (CC BY). The use, distribution or reproduction in other forums is permitted, provided the original author(s) or licensor are credited and that the original publication in this journal is cited, in accordance with accepted academic practice. No use, distribution or reproduction is permitted which does not comply with these terms.
*Correspondence: Andre Velescu, YW5kcmUudmVsZXNjdUBraXQuZWR1