- 1Department of Geology, University of Wisconsin Oshkosh, Oshkosh, WI, USA
- 2Department of Geology and Environmental Geosciences, Northern Illinois University, DeKalb, IL, USA
- 3Department of Entomology, University of Wisconsin-Madison, Madison WI, USA
- 4Department of Geoscience, Indiana University of Pennsylvania, Indiana, PA, USA
Microscopic soft tissues have been identified in fossil vertebrate remains collected from various lithologies. However, the diagenetic mechanisms to preserve such tissues have remained elusive. While previous studies have described infiltration of biofilms in Haversian and Volkmann's canals, biostratinomic alteration (e.g., trampling), and iron derived from hemoglobin as playing roles in the preservation processes, the influence of sediment texture has not previously been investigated. This study uses a Kolmogorov Smirnov Goodness-of-Fit test to explore the influence of biostratinomic variability and burial media against the infiltration of biofilms in bone samples. Controlled columns of sediment with bone samples were used to simulate burial and subsequent groundwater flow. Sediments used in this study include clay-, silt-, and sand-sized particles modeled after various fluvial facies commonly associated with fossil vertebrates. Extant limb bone samples obtained from Gallus gallus domesticus (Domestic Chicken) buried in clay-rich sediment exhibit heavy biofilm infiltration, while bones buried in sands and silts exhibit moderate levels. Crushed bones exhibit significantly lower biofilm infiltration than whole bone samples. Strong interactions between biostratinomic alteration and sediment size are also identified with respect to biofilm development. Sediments modeling crevasse splay deposits exhibit considerable variability; whole-bone crevasse splay samples exhibit higher frequencies of high-level biofilm infiltration, and crushed-bone samples in modeled crevasse splay deposits display relatively high frequencies of low-level biofilm infiltration. These results suggest that sediment size, depositional setting, and biostratinomic condition play key roles in biofilm infiltration in vertebrate remains, and may influence soft tissue preservation in fossil vertebrates.
Introduction
The presence of non-biomineralized osteocytes and blood vessels in vertebrate fossils and sub-fossils from various fluvial deposits has been well-established in literature (Pawlicki, 1978; Schweitzer et al., 2005, 2007, 2016; Asara et al., 2007; Bertazzo et al., 2015; Lee et al., 2017). Particles of aggregated hematite have been suggested to play a role in the preservation of collagen in such fossils (Schweitzer et al., 2005, 2007, 2016; Lee et al., 2017). Crucially, growth of microbial biofilms, prokaryotic organisms in an exopolymeric matrix (Schweitzer et al., 2016), on the surface of bone and the subsequent sealing of natural osteological vectors like pores of blood vessels and Haversian and Volkmann's canals have been suggested to facilitate the preservational mechanisms that occur during the early phases of diagenesis; biofilm infiltration leads to mineralization, retarding further metabolization of organic materials and promoting soft tissue preservation (Trinajstic et al., 2007; Peterson et al., 2010; Schweitzer et al., 2016).
Some previous analyses of early diagenetic biofilm growth on archosaur bones used the Extant Phylogenetic Bracket (EPB) method of inference after Witmer (1995) to focus on the influence of higher order phylogenetic variation and biostratinomic processes (e.g., trampling and subsequent fracturing before burial) with extant analogs (Peterson et al., 2010). Crushing of a bioclast (biostratinomy), which can stem from particle transport in high-energy fluvial systems, diagenetic alteration, or macrovertebrate bioturbation, produces new vectors for microbial infiltration of bone pore spaces in the form of cracks and fractures, providing open conditions for decomposition of internal organic materials in bones. Bone alteration, such as crushing and fracturing, has previously been illustrated to have a statistically significant impact on biofilm formation and infiltration (Peterson et al., 2010).
Another critical factor in the preservation of more labile tissues in fossils is the sediment in which they are buried (Allison and Briggs, 1991; Schweitzer et al., 2007; Piñeiro et al., 2012). From site to site, sedimentary deposits will vary in texture, porosity, permeability, groundwater geochemistry, and microbial composition (Briggs, 2003; Maier et al., 2009). As the growth rate of microbial biofilm depends on the nutrients available at nucleation sites, factors that influence the transport of nutrients, such as burial media, must be taken into account (Allison and Briggs, 1991; Briggs, 2003; Trinajstic et al., 2007; Piñeiro et al., 2012).
The hypothesis of this study was that the burial media (sands, silts, and clays) and biostratinomic condition (whole or crushed bone) influence the development and infiltration of biofilms and authigenic mineralization in extant theropod bones. By further understanding the role of biofilms in the early diagenetic phases of fossilization, predictions can be made regarding the conditions necessary for likely soft tissue preservation in fossilized bone.
Methodology
Sample Preparation
To test the hypothesis of this study, controlled column experiments were utilized to simulate groundwater flow during early diagenesis (Leal-Batista and Lenczewski, 2006; Lenczewski et al., 2007; Greenhagen et al., 2014; Waska, 2014). The water flowed through a variety of sediments with textures representative of common fluvial settings containing extant theropod bones, which were then analyzed for degree of biofilm formation, defined as a series of Classes (after Peterson et al., 2010). To appropriately model the taphonomy of fossil theropod specimens previously reported to possess primary soft-tissues that may be influenced by biofilms, such as subadult tyrannosaurs (Peterson et al., 2010), eight femora of the extant theropod Gallus gallus domesticus (Domestic Chicken) were selected and carefully stripped of bulk muscle and connective tissues with a sterilized scalpel. Samples were obtained from commercial sources (i.e., organic grocery stores in Northern Illinois). The handling of all bone samples was performed with nitrile gloves to avoid contamination to the microflora in this experiment. No permits were required for this study.
Column Construction
To explore the role of burial media on the development of microbial biofilms in extant theropod bone, control column experiments were used to simulate groundwater flow after burial in different sediments (Greenhagen et al., 2014; Waska, 2014). Three sedimentary groups were developed and modeled after fluvial facies commonly associated with vertebrate fossils; channel sands, crevasse splays, and distal flood-basin deposits (Table 1). Sediment mass ratios were based on particle size analysis values for common late Cretaceous fluvial depositional environments (Peterson et al., 2011). Sand and clay was obtained from commercial sources (quartz masonry sand and bentonite commonly used in installation of groundwater wells) and silt was obtained from a local Peoria loess deposit in northern Illinois. All sediments were dried prior to use.
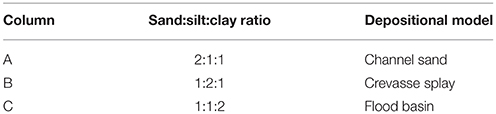
Table 1. Sample columns, particle size ratios, and depositional models based on particle size analysis from Peterson et al. (2011).
Columns were constructed from 10 cm wide PVC pipes and each was approximately 30 cm long with a final volume of approximately 2,700 cm3 (Figure 1). The upper and lowermost 50 mL of each column were filled with high density polyethylene (HDPE) spheres to aid in equal distribution of water inflow and outflow. The lower layer of HDPE spheres was covered with 500 mL of coarse silica sand to further aid in water flow, followed by 1,000 mL of sediment modeling specific depositional environments (Table 1), with each column containing a different fluvial analog and the bone samples. The columns were then filled with 250 mL of coarse silica sand, followed by an additional 500 mL of HDPE spheres. To simulate pre-burial biostratinomic variation, two bone samples were reserved for placement in each sediment column; one sample was placed intact, and the second sample was crushed. Crushing was conducted in a sterile Whirl-Pak bag perpendicular to the long axis of the bone to simulate bones that have been fractured prior to burial, as often observed in the vertebrate fossil record (e.g., Behrensmeyer et al., 1986). The column was subsequently filled with sediment to within 2–3 cm of the top of the column, where a final layer of HDPE spheres were added.
Columns were then placed on a laboratory bench and connected to a low-flow peristaltic pump that bottom fed groundwater directly into the columns. The groundwater was sourced from a well-located near Northern Illinois University campus, however the pump was not connected directly to the well. Instead, an influent carboy, filled with well-water, was connected to the pump. The carboy was connected to the peristaltic pump, and the tubing was flushed with three times its internal volume, before being connected to the bottom influents of the columns.
The time zero for each column started on the first day of observing an effluent from the column. The experiment maintained continuous flushing of the columns for 180 days with short shut-off times (up to 10 min) for water source replacement (i.e., refilling the influent carboy with well-water). Source water was kept the same throughout the experiment to maintain a similar microbial community. Effluent from the columns was monitored for pH and temperature every 3–4 days. The effluent from the columns indicated that the temperature was around room temperature (25°C) and the pH varied from 6.5 to 7.9 throughout the experiment (Figure 2A).
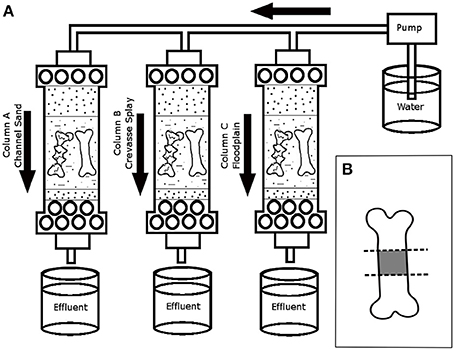
Figure 2. Schematic of experimental columns. (A) Placement of bone samples and water flow to the six columns over the duration of the experiment. Effluent water was collected regularly to test variability in pH. (B) Schematic of section of bone samples following experiment. Diaphyseal portion of the bone samples (in gray) were used in SEM analyses.
The experiment ran for 180 days at a continuous flow rate (500 mL/day), after which the bones and sediment samples in contact with the bones were collected. All handling of specimens was conducted while wearing nitrile gloves. Each bone sample was cut into three equal transverse sections for scanning electron microanalysis (SEM) (Figure 2B), which focused on the diaphyseal portion of each studied limb element. Sectioning was performed using a laminar flow hood, a set of sterilized forceps, and 20 flame-sterilized cutting plates. A new flame-sterilized blade was used for each sample and the forceps were re-sterilized prior to cutting each specimen. All specimens were placed in labeled sterile Whirl-Pak bags and stored in a −70°C freezer to preserve the biofilms until preparation for SEM analyses.
Scanning Electron Microanalysis
Prior to SEM analysis, each sample was removed from its Whirlpak bag using nitrile gloves and lypholized. Samples were then fixed with Canemco carbon tape to a standard SEM aluminum sample stub. Using an SPI magnetron sputter coater, the specimens were Au/Pd coated to a thickness of ~40 nm to ensure optimal adhesion and conduction in preparation for analysis. Specimens were imaged at 300x magnification utilizing secondary electron imaging (SEI) via a JEOL 5900 Low Vacuum Scanning Electron Microscope with Oxford INCA EDS at Beloit College. All images were taken with a 40 spotsize, 20 keV accelerating voltage at an average working distance of 10 mm. For each specimen, 25–30 images of Haversian and Volkmann's canals were collected. To confirm that the substances encrusting pore spaces in Gallus gallus domesticus study specimens were biofilms, micrographs of colonial bacteria within the material were collected at ultra-high magnifications for each specimen (450x–5,000x) (Figure 3), revealing microbial communities surrounded by an undulatory extracellular matrix [e.g., extracellular polymeric substance (EPS)].
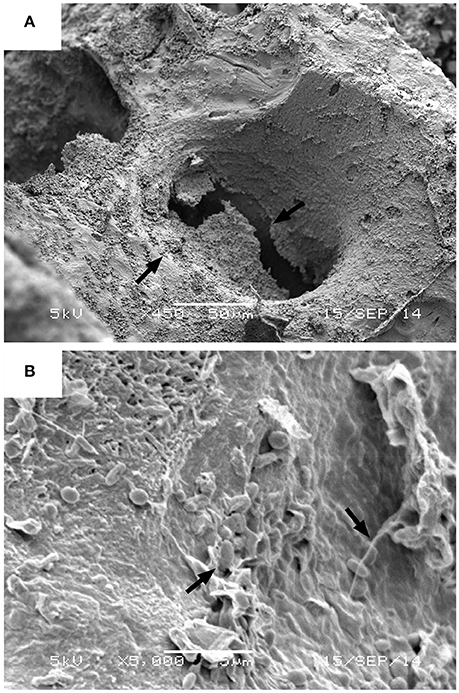
Figure 3. Examples of biofilm morphology. (A) Arrows indicated the presence of encrusting biofilm on Haversian canal wall of a crushed Gallus gallus domesticus femur buried in the modeled crevasse splay deposit, with microbes resting in an undulatory exopolysaccharide (EPS) matrix. Image taken under HV71 at 450x magnification, 56 spotsize, with a 5 keV accelerating voltage (scale = 5 μm). (B) Arrows indicating the presence of bacterial cells in EPS, taken under HV71 at 5,000x magnification, 39 spotsize, with a 5 keV accelerating voltage (scale = 5 μm).
Statistical Analysis
In order to quantify the degree of biofilm infiltration in bone samples, natural vectors in the form of Volkmann's and Haversian canals were identified at the bone surfaces at a magnification of 300x and tabulated for degree of biofilm infiltration (Table 2, Figure 4). In order to standardize comparisons among Haversian and Volkmann's canals, canals and other vectors were chosen based on their diameter, with only vectors between 50 and 100 μm being chosen for analysis.
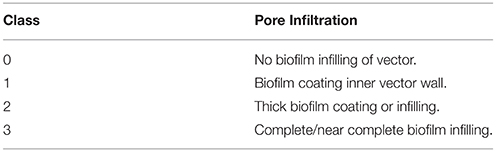
Table 2. Degree of biofilm infiltration into observed natural vectors in bone samples of G. gallus domesticus, defined as 4 distinct nominal Classes (after Peterson et al., 2010).
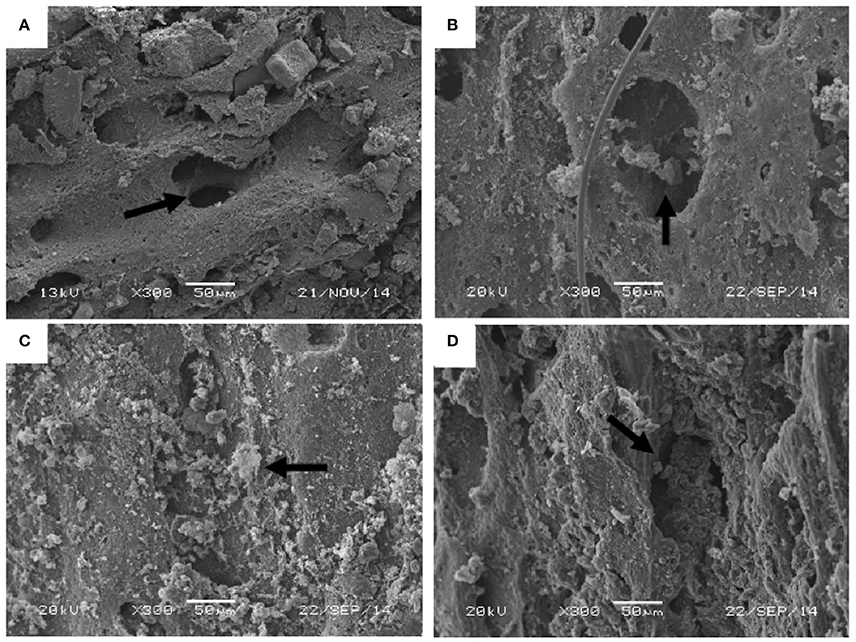
Figure 4. Examples of Vector Infiltration Classes for statistical analysis: (A) Class 0—no biofilm visible inside of canal. Arrow indicating the biofilm-free canal wall; (B) Class 1—some biofilm coating inner pore wall. Arrow indicating the presence of early-stage biofilm development; (C) Class 2—biofilm thickening and beginning to seal pore aperture of a whole femur. Arrow indicating the increasing abundance of biofilm; (D) Class 3—near complete infilling of the canal. Arrow indicating the presence of nearly complete covering of biofilms. All images are SEI, taken under HV71 at 300x magnification, 40 spotsize, with a 20 keV accelerating voltage and a working distance of 8–12 mm.
In order to quantify the degree of modern biofilm infiltration in extant theropod bone, natural osteological vectors were identified on the surface of SEM samples at a magnification of 300×. Following Peterson et al. (2010), a series of four nominal classes were established to categorize the degree of biofilm infiltration (Table 2; Figures 4A–D). A natural vector with no visible biofilm was classified as Class 0 (Figure 4A), a vector with a thin biofilm coating the inner wall of the canal was classified as Class 1 (Figure 4B), a Class 2 vector exhibits thickening biofilm that is beginning to close the aperture of the pore aperture of the vector (Figure 4C), and a Class 3 vector possesses a nearly complete infilling of the canal by biofilm (Figure 4D). Kolmogorov-Smirnov Goodness-of-fit tests were performed on the pore size/infiltration matrix to identify variation in biofilm infiltration among samples in different taphonomic conditions at a 0.05 significance level (α = 0.05).
Results
Variance in the Degree of Biofilm Infiltration
Degree of biofilm infiltration for all analyzed samples is recorded in Tables 3A,B. Both whole and crushed bones from modeled channel sediments displayed the highest frequency of infiltration Class 3 (whole −50%; crushed −40%). Frequencies of infiltration observed for whole and crushed bones in modeled channel sediments decrease with each successive infiltration Class, such that Class 2 is the second most frequently observed, followed by Class 1 and Class 0. For bone samples in modeled crevasse splay sediments, whole bones display a similar pattern to channel sediments, with Class 3 being the most frequent (44%), followed by Classes 2 and 1 (26% each) and finally Class 0. However, crushed bones from modeled crevasse splay sediments displayed the highest proportion of infiltration Class 0 (36%), followed in order of decreasing frequency by Class 1, 2, and 3. Results from modeled flood basin sediments follow those of the channel sediments, with Class 3 being the most frequently recorded for both whole (81%) and crushed (62%) bones. Each subsequent infiltration Class is less frequent for whole bone in modeled flood basin sediments. Crushed bones revealed fewer Class 2 than 3, and equal frequencies of Classes 1 and 0 (8% each).
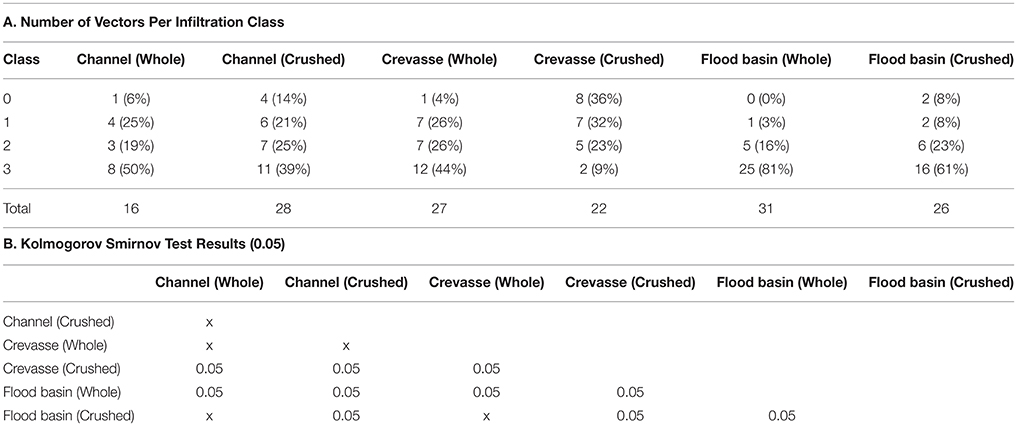
Table 3. Kolmogorov Smirnov Goodness of Fit results: (A) number of natural osteological vectors tallied with Class of biofilm infiltration; (B) results of significant difference comparisons.
Burial Media
Bone samples buried in the modeled flood-basin deposit (clay) exhibited a trend toward high frequencies of Class 3 biofilm infiltration and very low frequencies of lower-class biofilm infiltration (Figure 5). Samples buried in modeled channel deposits (sand) and crevasse splays (silt) exhibited similar trends of moderate frequencies of mid-level biofilm infiltration (Classes 1 and 2).
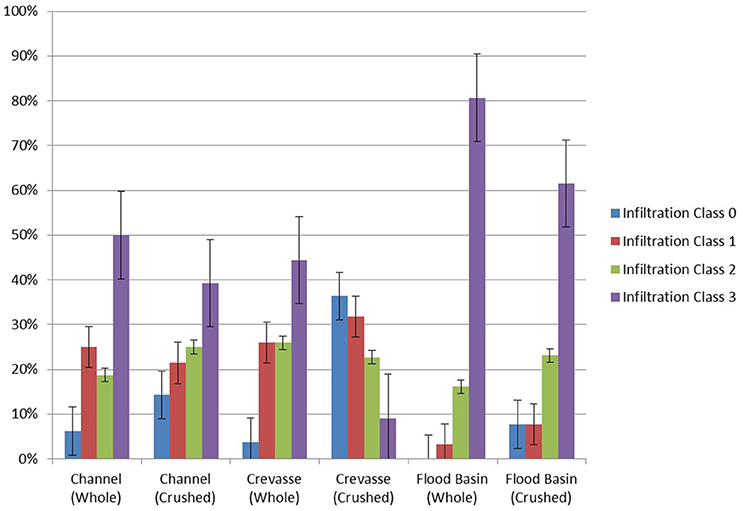
Figure 5. Degree of biofilm infiltration of Haversian and Volkmann's canals of bone samples in various burial media and biostratinomic conditions. Error bars represent standard error.
Biostratinomic Condition
Crushed-bone samples consistently exhibited lower frequencies of maximum biofilm infiltration (Class 3) than their whole-bone counterparts (Figure 5) in channel and flood-basin deposits. Crushed- and whole-bone samples buried in modeled crevasse splay deposits exhibit considerable variability. Alternatively, whole- and crushed-bone samples in channel sands follow comparable trends, exhibiting moderate-levels (Classes 1 and 2) of biofilm infiltration. Whole- and crushed-bone samples buried in modeled flood basin deposits exhibited very high frequencies of Class 3 biofilm infiltration compared to all other samples, and very low frequencies of other infiltration Classes.
Statistical Analysis
Kolmogorov Smirnov Goodness-of-Fit test results show significant differences (p < 0.05) (Tables 3A,B) between biofilm infiltration in whole-bone vs. crushed-bone samples in modeled crevasse splay and flood basin sediments. Biofilm development in whole-bone and crushed-bone samples buried in a modeled channel sand show similar frequencies of biofilm Classes and were not found to be significantly different.
Differences between bone samples (whole and crushed) buried in modeled flood basins and channel sands also show significant differences, as do bone samples buried in modeled flood basins and crevasse splay sediments. However, differences between channel sands and crevasse splay sediments are only significant in crushed samples; whole-bone samples between channel and crevasse splay sediments were not found to be significantly different (Table 3B).
Discussion
These results confirm previous analyses concerning the relationship between biostratinomy and biofilm growth in modern bones (Peterson et al., 2010), and further demonstrate that the grain size of burial media plays a significant role in the degree of biofilm development. However, while both biostratinomic and depositional variables significantly influence the degree of biofilm growth in bone samples, it is clear biostratinomy plays a greater role than depositional setting. Biofilm infiltration is the highest in whole-bone samples buried in flood-basin analogs (clay-rich), and lowest in crushed samples in crevasse splay analogs (silt-rich). All samples, with the exception of crushed samples buried in crevasse splay analogs, show a similar trend of dominance Class 3 (Table 2), likely due to the prolonged duration of the experiment (6 months).
Peterson et al. (2010) experimentally demonstrated the potential relationship between relative degree of biofilm infilling of natural osteological vectors (e.g., Haversian and Volkmann's canals) and osteological soft tissue preservation in vertebrate fossils. The mineralization of biofilms established in osteological vectors during decomposition may promote the preservation of primary soft-tissues in extant and fossil archosaur bones by retarding further microbial metabolization of tissues in bones (Trinajstic et al., 2007; Peterson et al., 2010; Schweitzer et al., 2016). As such, bones which have experienced less biostratinomic alteration, i.e., bones which have not been crushed, are more likely to preserve soft tissues than those which have been altered (Peterson et al., 2010).
The results of this study demonstrate that well-developed biofilms infiltrate the vectors of whole bones better and more thoroughly than in crushed bones, mirroring the results of Peterson et al. (2010). Whole-bones samples buried in modeled channel sands and crevasse splay sediments exhibited relatively high frequencies of nearly complete or completely biofilm-filled Haversian and Volkmann's canals (Classes 2 and 3), and thus would be expected to yield primary soft-tissues in vertebrate fossils. Crushed bones in modeled channel and crevasse sediments display decreased frequencies of Class 3 infiltration, and are less likely to preserve soft tissues. However, whole and crushed bones in modeled flood-basin sediments both exhibited extremely high frequencies of biofilm infiltration (Class 3), demonstrating an interaction between biostratinomic condition and sediment grain size.
Samples buried in flood basin sediments possess an increased frequency of advanced biofilm development, likely due to the increased concentrations of microbial communities in mud and clay-rich sediments (Camesano and Logan, 1998). However, lithification of mudstones involves a more pronounced degree of compaction, and slickenside surfaces that develop during the early diagenetic stage can fracture and displace bioclasts, opening additional vectors for microorganisms to enter bones and metabolize soft tissues (Peterson et al., 2010). Furthermore, mud-rich flood-basin deposits are generally more likely to yield remains that have been trampled, crushed, and subaerially exposed for a considerable period of time prior to burial (Behrensmeyer, 1982; Gates, 2005), thus reducing the likelihood of preserved primary soft-tissues. The results of this experiment verify these findings. While Class 3 infiltration is most commonly exhibited by both whole and crushed bones in modeled flood basin sediments, the whole bones have a higher frequency of Class 3 (81%) compared to crushed bones (61%). Hence, while mud and clay-rich flood basin sediments are more likely to preserve soft tissues compared to deposits with larger grain sizes, biostratinomic effects still play a role in soft tissue preservation in fine-grained deposits.
Sands, while lower in concentrations of microbial communities (Camesano and Logan, 1998), provide a grain-supported framework which would reduce crushing and keep the natural vectors as the primary means for microbial integration. From these results, a prediction can be proposed that un-fractured bones in sand to silt-sized sediments are more likely to yield primary soft tissues in vertebrate fossils than crushed bones in either setting.
While previous studies on biofilm development in vertebrate fossils show a potential influence on the preservation of osteological soft-tissue in the fossil record (Trinajstic et al., 2007; Peterson et al., 2010; Schweitzer et al., 2016), it should be noted that the observations of this study do not necessarily imply that primary osteological soft tissue preservation will always occur in accordance with biofilm development. Though primary soft-tissues in vertebrate fossils are commonly associated with relatively coarse-grained facies (e.g., Schweitzer et al., 2005; Asara et al., 2007), the results of this study suggest soft tissue preservation should be more common in fine-grained sediments. The limited exposure and rapid burial of samples in this experiment may produce unique conditions for soft-tissue preservation in remains buried in finer-grained facies. However, Peterson et al. (2010) reported on the extraction of primary soft-tissues from tyrannosaurid, ceratopsian, and ornithopod dinosaurs encased in a variety of depositional settings, and only those ceratopsian bone fragments which were buried in a flood-basin deposit yielded pliable tissues, following the results of this study. The nature of the deposit studied by Peterson et al. (2010) may explain the departure from the commonly observed pattern. The taphonomy for this deposit has been thoroughly described (Mathews et al., 2009) and the remains exhibit virtually no abrasion, crushing, or fracturing, and are attributed to three individual juvenile Triceratops; a unique age profile that suggests a single depositional event and limited exposure prior to burial (Mathews et al., 2009), similar to the conditions created in this study. As such, the preservation of pliable primary soft-tissues may be due to biostratinomic processes such as the rate of burial. Rapid burial of the remains could promote soft-tissue preservation rather than the usual taphonomic processes associated with floodplain deposits, such as prolonged exposure and biostratinomic reworking (Behrensmeyer, 1982; Gates, 2005).
The mechanisms required to promote preservation of soft-tissue internal to bone in vertebrate fossils are complex. Despite previously proposed alternative hypotheses for the presence of primary soft-tissues in Mesozoic vertebrate fossils, such as contaminants or recent biofilms (Kaye et al., 2008), an abundance of biomolecular evidence has confirmed their initial interpretations as primary soft-tissues (Schweitzer et al., 2005, 2009, 2016; Asara et al., 2007). Biochemical processes have been proposed to play a significant role in the preservation of soft osteological tissues; recently iron has been suggested as an alternative mechanism to explain the process by which primary soft-tissues can remain pliable over geologic time, by which iron from hemoglobin serves as a natural chelator to increase tissue immunoreactivity in vertebrate remains (Schweitzer et al., 2014; Lee et al., 2017). However, the presence of mineralized biofilms in vertebrate samples were osteological soft-tissues have also been recovered suggests a role in tissue preservation (Briggs, 2003; Peterson et al., 2010; Kremer et al., 2012; Raff et al., 2013, 2014; Schweitzer et al., 2016), where biofilm crystallization in Haversian and Volkmann's canals seals natural vectors from further microbial penetration and retards subsequent metabolization of soft-tissues. Therefore, by sealing the internal pore spaces of bones, biofilms promote the preservation of those soft tissues found within bone. Furthermore, iron has also been previously associated with soft-tissue preservation and biofilms and interpreted as pyrite framboids (Kaye et al., 2008; Peterson et al., 2010). The presence of iron associated with both preserved soft-tissues and mineralized biofilms suggests that multiple mechanisms may be involved in the preservation of primary biomolecules in fossil vertebrates (e.g., Kaye et al., 2008; Peterson et al., 2010; Schweitzer et al., 2014), including depositional chemistry, biostratinomy and sediment grain size. In order to fully explain the complex processes required to preserve primary soft-tissues in vertebrate remains over tens of millions of years, further analysis and actualistic taphonomic experimentation of biochemistry, geochemistry, and paleoenvironmental factors are needed. Furthermore, future experimentation is needed in order to understand the complex interactions between environmental factors which may lead to the preservation of those soft tissues not found within bone.
Author Contributions
JP and ML conceptualized the study, designed the experiments, obtained samples, and collected data. JP, ML, SC, and JW analyzed the data, and wrote the manuscript.
Conflict of Interest Statement
The authors declare that the research was conducted in the absence of any commercial or financial relationships that could be construed as a potential conflict of interest.
The reviewer LZ and handling Editor declared their shared affiliation, and the handling Editor states that the process nevertheless met the standards of a fair and objective review.
Acknowledgments
We thank the Department of Geology at Beloit College for access to their SEM facilities. We also thank Dr. Rongping Deng and Dr. Jim Rougvie of Beloit College for their expert guidance and instruction in the operation of instrumentation used in this study. This research was funded by the University of Wisconsin Oshkosh External Grants Expansion Program grant number 102-222051-1 and the Northern Illinois University Department of Environmental Studies. We also thank Michel Laurin for editorial assistance and three reviewers for offering constructive criticism and helpful feedback.
References
Allison, P. A., and Briggs, D. E. G. (1991). “The taphonomy of soft-bodied animals,” in Fossilization: the Process of Taphonomy, ed. S. K. Donovan (London: Belhaven Press), 120–140.
Asara, J. M., Schweitzer, M. H., Freimark, L. M., Phillips, M., and Cantley, L. C. (2007). Protein sequences from Mastodon and Tyrannosaurus rex revealed by mass spectrometry. Science 316, 280–285. doi: 10.1126/science.1137614
Behrensmeyer, A. K. (1982). Time resolution in fluvial vertebrate assemblages. Paleobiology 8, 211–227. doi: 10.1017/S0094837300006941
Behrensmeyer, A. K., Gordon, K. D., and Yanahi, G. T. (1986). Trampling as a cause of bone surface damage and pseudo-cutmarks. Nature 319, 768–771. doi: 10.1038/319768a0
Bertazzo, S., Maidment, S. C., Kallepitis, C., Fearn, S., Stevens, M. M., and Xie, H. (2015). Fibres and cellular structures preserved in 75-million-year-old dinosaur specimens. Nat. Commun. 6:7352. doi: 10.1038/ncomms8352
Briggs, D. E. G. (2003). The role of decay and mineralization in the preservation of soft-bodied fossils. Annu. Reb. Earth Planet Sci. 31, 275–301. doi: 10.1146/annurev.earth.31.100901.144746
Camesano, T. A., and Logan, B. E. (1998). Influence of fluid velocity and cell concentration on the transport of motile and nonmotile bacteria in porous media. Environ. Sci. Technol. 32, 1699–1708. doi: 10.1021/es970996m
Gates, T. A. (2005). The late Jurassic Cleveland-Lloyd Quarry as a drought-induces assemblage. Palaios 20, 363–375. doi: 10.2110/palo.2003.p03-22
Greenhagen, A., Lenczewski, M., and Carroll, M. (2014). Natural attenuation of pharmaceuticals and an Illicit drug in laboratory column. Chemosphere 115, 13–19. doi: 10.1016/j.chemosphere.2014.01.015
Kaye, T. G., Gaugler, C., and Sawlowicz, Z. (2008). Dinosaurian soft tissues interpreted as bacterial biofilms. PLoS ONE 3:e2802. doi: 10.1371/journal.pone.0002808
Kremer, B., Owocki, K., Królikowska, A., Wrzosek, B., and Kazmierczal, J. (2012). Mineral microbial structures in a bone of the Late Cretaceous dinosaur Saurolophus angustirostris from the Gobi desert, Mongolia – a Raman spectroscopy study. Palaeogeogr. Palaeoclimatol. Palaeoecol. 358–360, 51–61. doi: 10.1016/j.palaeo.2012.07.020
Leal-Batista, R., and Lenczewski, M. (2006). Sorption of MTBE and benzene in fine-grained materials from northern Illinois and the Chalco Basin, Mexico. Environ. Geosci. 13, 31–41. doi: 10.1306/eg.09280404027
Lee, Y. C., Chiang, C. C., Huang, P. Y., Chung, C. Y., Huang, T. D., Wang, C. C., et al. (2017). Evidence of preserved collagen in an Early Jurassic sauropodomorph dinosaur revealed by synchrotron FTIR microspectroscopy. Nat. Commun. 8:14220. doi: 10.1038/ncomms14220
Lenczewski, M., Leal-Baustista, R., and Kroll, S. (2007). Influence of Ethanol and MTBE on the biodegradation and transport of benzene in loess. Environ. Geosci. 14, 137–148. doi: 10.1306/eg.09150606006
Maier, R., Pepper, I., and Gerba, C. C. (2009). Environmental Microbiology, 2nd Edn. Amsterdam: Academic Press.
Mathews, J. C., Brusatte, S. L., Williams, S. A., and Henderson, M. D. (2009). The first Triceratops bonebed and its implications for gregarious behavior. J. Vertebrate Paleontol. 29, 286–290. doi: 10.1080/02724634.2009.10010382
Pawlicki, R. (1978). Morphological differentiation of the fossil dinosaur bone cells Light, transmission electron-, and scanning electron-microscopic studies. Acta Anatomica 100, 411–418.
Peterson, J. E., Lenczewski, M. E., and Scherer, R. P. (2010). Influence of microbial biofilms on the preservation of primary soft tissue in fossil and extant archosaurs. PLoS ONE 5:e13334. doi: 10.1371/journal.pone.0013334
Peterson, J. E., Scherer, R. P., and Huffman, K. M. (2011). Methods of microvertebrate sampling and their influences on taphonomic interpretations. Palaios 26, 81–88. doi: 10.2110/palo.2010.p10-080r
Piñeiro, G., Ramos, A., Godo, C., Scarabino, F., and Laurin, M. (2012). Unusual environmental conditions preserve a Permian mesosaur-bearing Konservat-Lagerstätte from Uruguay. Acta Palaeontol. Pol. 57, 299–318. doi: 10.4202/app.2010.0113
Raff, E. C., Andrews, M. E., Turner, F. R., Toh, E., Nelson, D. E., and Raff, R. A. (2013). Contingent interactions among biofilm-forming bacteria determine preservation or decay in the first steps toward fossilization in marine embryos. Evol. Dev. 15, 243–256. doi: 10.1111/ede.12028
Raff, R. A., Andrews, M. E., Pearson, R. L., Turner, R., Saur, S. T., Thomas, D. C., et al. (2014). Microbial ecology and biofilms in the taphonomy of soft tissues. Palaios 29, 560–569. doi: 10.2110/palo.2014.043
Schweitzer, M. H., Moyer, A. E., and Zheng, W. (2016). Testing the hypothesis of biofilm as a source for soft tissue and cell-like structures preserved in dinosaur bone. PLoS ONE. 11:e0150238. doi: 10.1371/journal.pone.0150238
Schweitzer, M. H., Wittmeyer, J. L., and Horner, J. R. (2007). Soft tissue and cellular preservation in vertebrate skeletal elements from the Cretaceous to the present. Proc R. Soc. B Biol. Sci. 274, 183–197. doi: 10.1098/rspb.2006.3705
Schweitzer, M. H., Wittmeyer, J. L., Horner, J. R., and Toporski, J. K. (2005). Soft-tissue vessels and cellular preservation in Tyrannosaurus rex. Science 307, 1952–1955. doi: 10.1126/science.1108397
Schweitzer, M. H., Zheng, W., Cleland, T. P., Goodwin, M. B., Boatman, E., Theil, E., et al. (2014). A role for iron and oxygen chemistry in preserving soft tissues, cells and molecules from deep time. Proc. R. Soc. B 281:20132741. doi: 10.1098/rspb.2013.2741
Schweitzer, M. H., Zheng, W., Organ, C. L., Avci, R., Suo, Z., Freimark, L. M., et al. (2009). Biomolecular characterization and protein sequences of the Campanian hadrosaur B. canadensis. Science 324, 626–631. doi: 10.1126/science.1165069
Trinajstic, K., Marshall, C., Long, J., and Bifield, K. (2007). Exceptional preservation of nerve and muscle tissues in Late Devonian placoderm fish and their evolutionary implications. Biol. Lett. 3, 197–200. doi: 10.1098/rsbl.2006.0604
Keywords: taphonomy, biofilm, biostratinomy, vertebrate paleontology, sedimentology
Citation: Peterson JE, Lenczewski ME, Clawson SR and Warnock JP (2017) Role of Sediment Size and Biostratinomy on the Development of Biofilms in Recent Avian Vertebrate Remains. Front. Earth Sci. 5:30. doi: 10.3389/feart.2017.00030
Received: 05 December 2016; Accepted: 31 March 2017;
Published: 26 April 2017.
Edited by:
Michel Laurin, UMR7207 Centre de Recherche sur la Paléobiodiversité et les Paléoenvironnements (CR2P), FranceReviewed by:
Subir Bera, University of Calcutta, IndiaJürgen Kriwet, University of Vienna, Austria
Louise Zylberberg, Centre National de la Recherche Scientifique (CNRS), France
Copyright © 2017 Peterson, Lenczewski, Clawson and Warnock. This is an open-access article distributed under the terms of the Creative Commons Attribution License (CC BY). The use, distribution or reproduction in other forums is permitted, provided the original author(s) or licensor are credited and that the original publication in this journal is cited, in accordance with accepted academic practice. No use, distribution or reproduction is permitted which does not comply with these terms.
*Correspondence: Joseph E. Peterson, cGV0ZXJzb2pAdXdvc2guZWR1