- Department of Earth Sciences, University of Southern California, Los Angeles, CA, United States
This study carries out a statistical analysis of high-resolution PSV records for the last ~70 ka from three different regions of the Earth. We consider directional and intensity variability in each region on time scales of 103-105 years in order to evaluate long-term PSV. We then compare those results with more traditional long-term PSV statistical studies averaged over ~106 years. Three replicate PSV records from one region (subtropical North Atlantic Ocean) were averaged at overlapping 3 and 9 ka intervals. Variability in both scalar inclination and declination variability and vector angular dispersion are significant and coherent among the three records. The vector dispersion is relatively low for most of the time but contains two relatively narrow intervals (~30–42 and 60–65 ka) of high dispersion. (Vector dispersion in all records was calculated after removing directions with true excursional VGPs, VGPs < 45° N). We have carried out a comparable statistical analysis on two other PSV records from other parts of the Earth (Chile margin; Philippines/Indonesia). The results for these three regions are comparable in their overall style of variability. The scalar directional variability from the Philippines/Indonesia is quite different in detail from the other two regions, as might be expected, but the scalar directional variability between the Western Hemisphere regions is remarkably consistent considering their distance from one another. This may be associated with them being on the same longitude swath and having some coherent dynamo activity occurring along that path. Three magnetic field excursions occur in the study interval. All three excursions are associated with the two highest vector dispersion intervals. Paleointensity records from the three regions were subjected to the same statistical analysis as the directions. These records are all coherent in their pattern of variability. The similarity in paleointensity variability on a global scale is expected even though the detailed scalar directional variability is not coherent on a global scale. The pattern of intensity variability is strongly correlated with the pattern of vector dispersion and excursions on a global scale—high (low) intensity is associated with low (high plus excursions) vector dispersion. The fact that regional directional variability is always larger than “normal” during low intensity/excursional intervals, even though the effect of true excursional directions was removed, suggests that we need to reevaluate what field variability was like during low intensity/excursional intervals on a global scale and how/why it was different from today's field (last 104 years).
Introduction
Magnetic compass readings have been associated with the Earth as their source since perhaps the thirteenth Century AD; experiments by, first, Petrus Peregrinus (1269) and then Alexander Needham (1600) put that theory on firm ground (see historical summary in Merrill et al., 1998). The fact that geomagnetic field measurements at the Earth's surface have changed over time (secular variation) was first noted by the Chinese by the thirteenth Century AD and later by Henry Gellibrand in Europe in 1634 (Merrill et al., 1998). We now have a good sense of historical secular variation (HSV) of the geomagnetic field for the last 400 years or so (e.g., Bullard et al., 1950; Yukutake, 1967; Yukutake and Tachinaka, 1968; Bloxham and Gubbins, 1985; Jackson et al., 2000). We also understand, to some degree, the source of that magnetic field through dynamo activity in the Earth's outer core (see Merrill et al., 1998 for overview).
Evidence for prehistoric secular variation, based on paleomagnetic measurements, was first noted by Chevaliier (1925) using lava flows from Mount Etna. 1951, Thellier and Thellier (1952) measured directional paleomagnetic secular variation (PSV) in archeological materials (baked hearths and pottery) from Europe. The earliest directional PSV records from sediments were derived from deep-sea sediment cores and exposed varved lake sediments (McNish and Johnson, 1938; Johnson et al., 1948). Thellier (1937a,b) was the first to determine paleointensity variations in archeological materials.
The magnetic field at any one location has a mean direction after averaging secular variation for some period of time—several thousand years or longer. The actual amount of time necessary to average the full range of local secular variation is still not well established (e.g., Merrill et al., 1998). However, paleomagnetic studies suggest that the average field direction at a locality during stable polarity (the period between global polarity reversals) is generally that of a dipole located at the Earth's center and aligned with the rotation axis; this hypothesis is termed the geocentric axial dipole (GAD) hypothesis (see Merrill et al., 1998 for overview).
Merrill et al. (1998) noted that “the interpretation of paleomagnetic results has always depended upon the fundamental hypothesis that the time-averaged geomagnetic field is that of a geocentric axial dipole.” But, a large number of statistical PSV studies have made it clear that there are systematic (but usually subtle) differences in local field directions from the GAD when averaged over millions of years (e.g., Johnson and Constable, 1996; McElhinny et al., 1996; Merrill et al., 1998; Johnson et al., 2008) or shorter intervals (e.g., Lund et al., 1988, 2016a). The unresolved problem is what causes these non-GAD field directions and how do they vary over time-scales less than a million years.
This paper focuses on a new set of replicate, high-resolution PSV records recovered from lake and deep-sea sediment cores for intervals of 50–100,000 years that demonstrate notable long-term variations in the statistical properties of local field. These new statistical results are developed and then compared with traditional results to suggest that a more fundamental understanding of geomagnetic field variability and the underlying dynamo source activity can be discerned from the high-resolution PSV data.
Traditional Analysis of Long-Term PSV
The normal method of long-term PSV analysis is to statistically average a spatially regional group of lava-flow data, which are uncorrelated in time and cover a long time interval (>105-106 years). The expectation is that individual data points are randomly placed in their age distribution and no two data points are strongly serially correlated in both age and direction. These spot averages are then compared on a global scale using spherical harmonic analysis or latitude/longitude transects (e.g., Johnson and Constable, 1996; McElhinny et al., 1996; Merrill et al., 1998; Harrison, 2007; Johnson et al., 2008). In this manner ΔI (the difference between local average inclination and that expected for the GAD), and angular dispersion of field vectors or VGPs (Figures 1A,B, solid dots) can be determined on a global scale (see summary in Merrill et al., 1998).
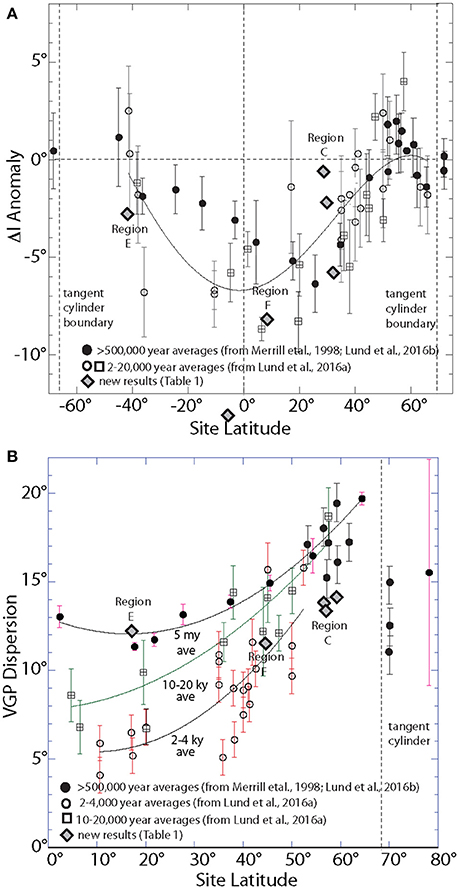
Figure 1. A summary of published Long-term (~106 years) averaged ΔI (A) and VGP (B) angular dispersion and selected more recent (~103 years) results. The data come from a mixture of lava-flow and sediment studies. Long-term averages are indicated by solid dots. ~3 and 10–20 ka averages (all latest Quaternary in age) are indicated by open circles and squares respectively. Smooth curves estimate the general pattern of variability for each average vs. latitude. VGP data from all studies flip Southern Hemisphere data to the Northern Hemisphere for averaging. Gray diamonds indicate statistical averages for our studied PSV data sets (Table 1) (modified from Merrill et al., 1998; Lund et al., 2016a).
Another method of analysis is to consider long sequences of successive PSV data points from deep-sea cores (e.g., Opdyke, 1972; Figure 2) or dated lava flows (e.g., McDougall et al., 1977) and look for long-term variations in statistical patterns at one site. One shortcoming is a lack of detailed age control and, thus, a need to average over long time intervals (>105 years) to minimize age uncertainty. Another shortcoming is the lack of certainty about the PSV variability itself. In sediments, that is due to low sedimentation rates (such as the data in Figure 2) and sediment averaging that preclude detailed PSV. In the case of lava flow sequences, unknown data intervals between successive flows preclude detailed PSV. In both cases, statistical studies are carried out over long intervals (>105 years) to be sure that enough recorded PSV is averaged.
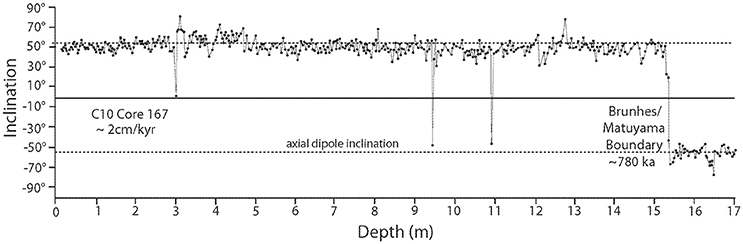
Figure 2. Deep-sea sediment paleomagnetic record from the subtropical Pacific Ocean. The core contains a complete record of PSV for the Brunhes Epoch (last 780 ka) and an average inclination close to axial dipole expectation. This record is a low-resolution (~2 cm/kyr sediment accumulation rate) that does not resolve high-resolution PSV features. But, it does describe the overall near GAD pattern of field during the current Brunhes normal chron and is representative of the available long-term sediment PSV records before the advent of more high-resolution sediment PSV studies such as we present here. Modified from Opdyke (1972).
The key element in all of these studies is that there is not strong evidence for detailed directional field variability as is common in studies of historical secular variation or Holocene PSV (e.g., Creer et al., 1983; Lund, 1996). The resolution of such studies is typically a million years or longer. Also, there is only limited ability to assess paleointensity variability associated with the vector variability.
New Long PSV Time Series
There are now sufficient, published full-vector PSV time-series (inclination, declination, paleointensity) for the last glacial cycle (0–71 ka) from deep-sea and lake sediment sequences to begin to assess long-term PSV on a global scale using high-resolution, serially-correlated data. Appendix 1 summarizes the criteria we use to distinguish what constitutes high-resolution PSV records. Figure 3 shows the locations of long PSV records from 6 different regions (A–F). All of these PSV records come from paleomagnetic studies of deep-sea or lake sediment sequences. There are replicate PSV records within each of these regions with good-quality age control, to permit correlation and analysis of directional-waveform and relative-paleointensity variability.
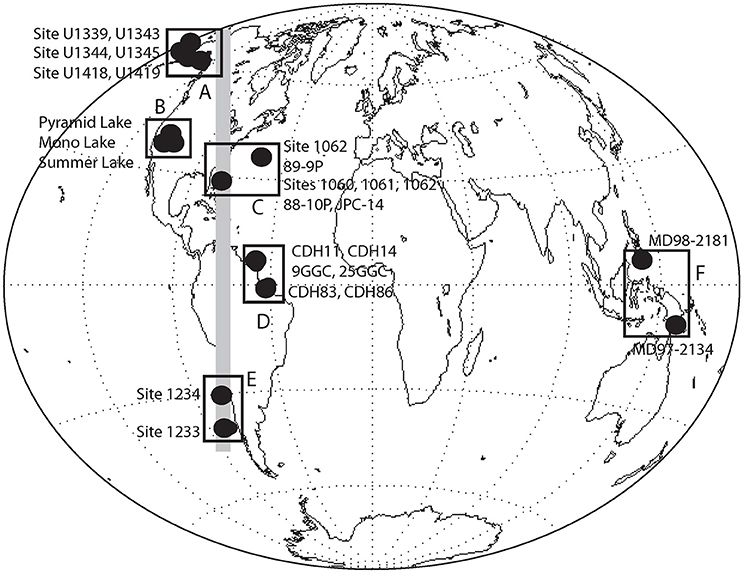
Figure 3. Map of high-resolution long-term PSV time series. There are replicate well-dated PSV records in each region. Five of the regions fall within one general longitude domain (~200–320°) indicated by the gray bar. This paper discusses long-term PSV over the last glacial cycle (0–71 ka) in regions C, E, and F. See text for more detailed information.
Region A (Bering Sea/Gulf of Alaska) contains multiple long PSV records from IODP Sites U1343-1345 from the Bering Sea (Takahashi et al., 2011; Lund et al., 2016b) and U1418-1419 from the Gulf of Alaska (Jaeger et al., 2014). Region B (SW USA) contains records from Mono Lake, California (Lund et al., 1988), Pyramid Lake, Nevada (Lund et al., 2017a), and Summer Lake, Oregon (Negrini et al., 2000; Zic et al., 2002). Region C (subtropical North Atlantic Ocean) contains records from the Bermuda Rise (89-9P, ODP Site 1062) (Keigwin et al., 1998; Schwartz et al., 1998; Lund S. et al., 2001; Lund S.P. et al., 2001) and the Blake-Bahama Outer Ridge (88-10P, JPC-14, ODP Sites 1060, 1061) (Keigwin et al., 1998; Schwartz et al., 1998; Lund S. et al., 2001; Lund S.P. et al., 2001). Region D (equatorial west Atlantic Ocean) contains records from the Demerara Rise (9GGC, 25GGC, CDH11, CDH14) (Huang et al., 2014; Lund et al., 2017b) and the Amazon Fan (CDH83, CDH86) (Nace et al., 2014). Region E (SE Pacific Ocean/Chile Margin) contains records from ODP Sites 1233 and 1234 (Mix et al., 2003; Lund et al., 2007). Region F (Philippines/Indonesia) contains records from MD97-2134 (Blanchet et al., 2006; Lund et al., 2018) and MD98-2181 (Stott et al., 2002; Lund et al., 2018).
This paper will consider, in detail, the statistical pattern of directional field variability from three Region C PSV records and compare it to individual PSV records from Regions E and F. Paleointensity variability will also be compared with all the records from these three regions. Figures 4, 5 show the inclination and declination variability for three PSV records from Region C (subtropical North Atlantic Ocean) over the last 70 ka. These three records span a distance of ~1,500 km and all contain individual inclination and declination records that display good serial correlation. The individual inclination and declination features can be correlated among these three records over the entire time interval.
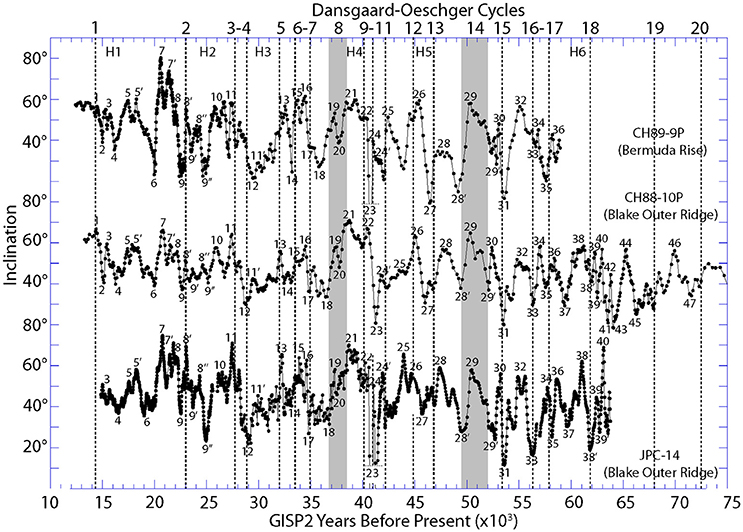
Figure 4. Region C inclinations. The inclination variability of three Region C PSV records are plotted. Forty replicate inclination maxima/minima are identified. Selected Dansgaard/Oeschger (D/O) Cycles are noted for chronologic control. Gray zones document two particular D/O cycles (8, 14) that are longer in duration.
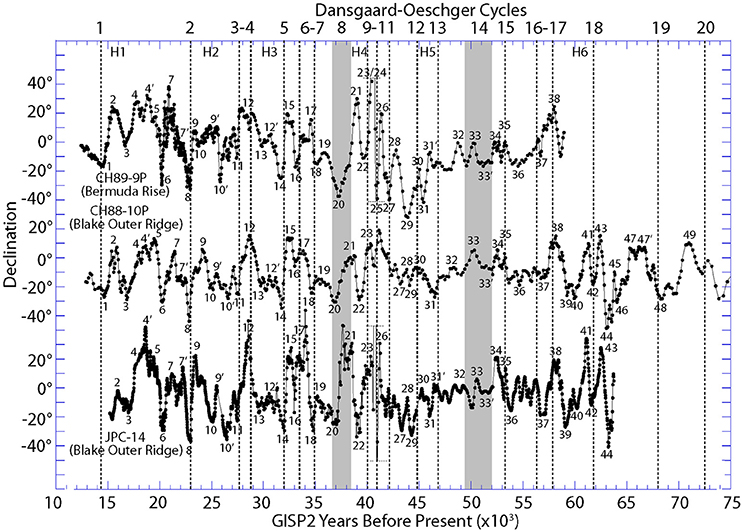
Figure 5. Region C declinations. The declination variability of three Region C PSV records are plotted. Forty replicate inclination maxima/minima are identified. Selected Dansgaard/Oeschger (D/O) Cycles are noted for chronologic control. Gray zones document two particular D/O cycles (8, 14) that are longer in duration.
Statistical Analysis of Long-Term Directional PSV Time Series
Holocene PSV records from around the world suggest that PSV is largely a centennial-millennial scale process with longer-term trends. The traditional long-term PSV studies averaged uncorrelated directional data over ~106 years time scales or longer. Well-dated high-resolution PSV time series permit statistical analysis over significantly shorter time intervals. The argument is that the dynamo process in the Earth's outer core that generates the geomagnetic field is operating on much shorter (~102-104 years) time scales and, if we want to properly understand the space/time pattern of dynamo activity there is merit in considering statistical averages at those shorter time scales.
There is no magical time scale over which to average the field to study dynamo activity, but previous PSV studies suggest that simple PSV directional features or waveforms (e.g., Lund, 2007) that define the pattern of PSV variability between successive extremes in inclination or declination (Figures 4, 5) operate on time scales of ~0.7–1.5 ka (e.g., summaries of regional PSV studies in Creer et al., 1983) and repeating patterns of more complex directional waveforms have been noted by Lund et al. (1988) and Lund (1996) with repeating time intervals of 2.5–3.5 ka. On that basis, we have started by averaging the three Region C PSV records at 3 ka intervals with 1.5-ka overlap between successive averages. We have averaged scalar inclination, declination, vector direction, and angular dispersion of directions. The vector dispersions were calculated after removing directions that have excursional VGPs (VGP latitudes less than 45° N; see Merrill et al., 1998; Johnson et al., 2008 for more detailed discussion). The results of this analysis are plotted in Figure 6 (open circles). The vector inclination variability is not significantly different from the scalar inclination variability plotted in Figure 6. We then carried out a second round of statistical averaging over a longer time average −9 ka with a 4.5 ka overlap between successive averages. Those results are also shown in Figure 6 (closed circles).
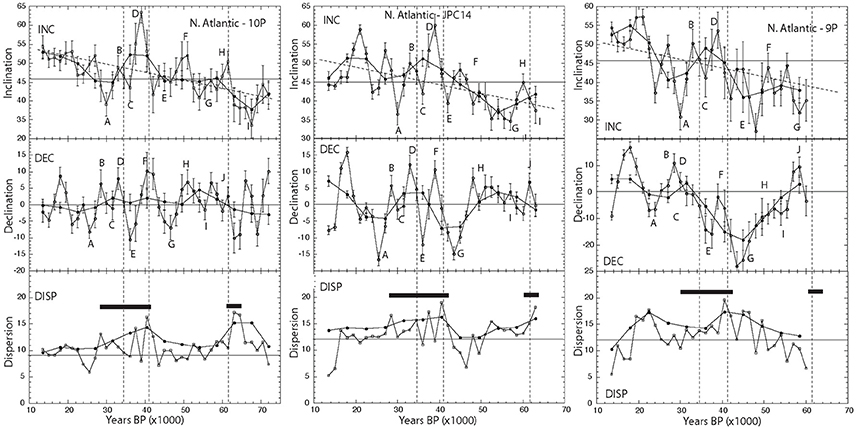
Figure 6. Region C long-term PSV statistics. The three Region C long-term PSV time series in Figures 4, 5 were averaged in 3 ka (open circles) and 9 ka (closed circles) intervals. The statistical parameters averaged in each panel are average scalar inclination (top), average scalar declination (middle), and total vector dispersion (bottom). Correlatable average inclination and declination variability is labeled (A–J) in each panel. Notable high vector-dispersion intervals are indicated by horizontal closed bars. Times of known global magnetic field excursions are indicated by vertical dashed lines.
Both the 3 and 9 ka averages show a strong serial correlation between successive values. This suggests that a shorter averaging interval is not needed to begin to see the temporal range of PSV variability. The 9 ka averages always have maximum or minimum values synchronous with 3 ka averages. This suggests that there is significant dynamical variability in the range of ~3–9 ka. Even so, there is also clear evidence for a long, >60 ka, trend in inclination that is indicated by a dashed line in Figure 6. Older PSV records from these sites (Keigwin et al., 1998) show that the average inclinations of the three records (~45°) are not significantly different from the long-term inclination averages at these sites for the entire Brunhes Epoch, so this trend does disappear in longer time intervals.
The range of directional variability in 3 ka averages is dramatic. Individual 3 ka intervals may have inclination and declination averages as much as 30° different from other intervals. The 9 ka variability still sees individual intervals with inclination and declination averages as much as 20° different from other intervals. These values are consistent with the differences noted at mid Northerly latitudes around the world in historical maps of spatial inclination and declination variability (e.g., Merrill et al., 1998). Thus, the 3 and 9 ka statistical variability may simply reflect the overall spatial/temporal dynamics of dynamo activity. The detailed pattern of scalar inclination and declination variability is also consistent among the three records as indicated by inclination features A–I and declination features A–J noted in Figure 6. As noted in other studies (Lund, 1996, 2007), it makes sense that the dynamical variability seen in Figure 6 should be consistent among these three PSV records that are located less than a few thousand km apart in Region C.
The vector statistical variability that results from this study is similar in pattern to the scalar variability and more directly comparable to long-term PSV studies noted above. The pattern of scalar inclination variability is not significantly different from the pattern of vector inclination variability. Thus, one could think of the varying inclination averages as varying ΔI anomalies (site inclination minus site axial dipole expectation, Figure 1). It is clear that the ΔI anomaly can vary by as much as 20° over ~9 ka intervals. The directional angular dispersion can also vary by almost a factor of two, with most time spent in relatively low dispersion, ~10°–12°.
These values can be compared with the overall (~105 years) statistical averages of the entire three time series. The results are summarized in Table 1 and plotted in Figure 1. The ~105 years length of these PSV records produce long-term statistical results that are generally comparable to the traditional long-term (~106) PSV results. Our results suggest, however, that these long-term averages smear out the variability at 103-104 years that probably reflects dynamo activity.
We can also compare the statistical results from Region C with similar results for Regions E and F (Figure 3). We have carried out a comparable statistical analysis on MD98-2181 from Region F and ODP Site 1233 from Region E. The results are shown in Figure 7. The PSV data for these records have been previously published and compared with other records from the same region (Region E: Mix et al., 2003; Lund et al., 2007) (Region F: Stott et al., 2002; Blanchet et al., 2006; Lund et al., 2017a) to verify the PSV pattern and chronology.
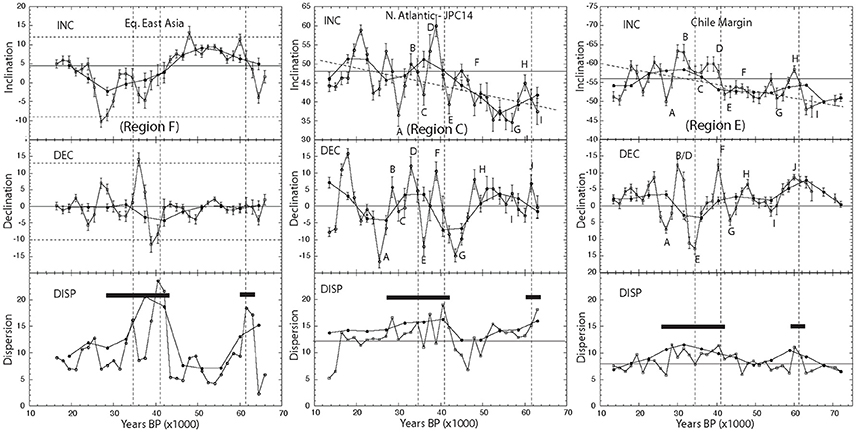
Figure 7. Comparison of PSV statistics among Regions C, E, and F. See Figure 6 caption for more details.
All three regions display an overall sense of directional variability comparable to that of Region C. It is interesting to note, however, that the detailed pattern of scalar inclination and declination variability is very similar between Regions C and Region E, as noted by inclination features A–I and declination features A–J (Figure 7). Both regions also note the same long-term trend in inclination (dashed trend lines in Figure 7). However, the variability in Region F is quite different in its detailed pattern from the other two regions. One possible explanation for this is that Regions C and E are along the same longitude swath (Figure 3) that aligns with the historical location of the North American high-altitude flux lobe (Bloxham and Gubbins, 1985). If that flux lobe is evidence of an outer-core wide pattern of dynamo activity (e.g., convection roll; Gubbins and Bloxham, 1987) along this longitude swath, then it may be that our scalar directional variability in Regions C and E are part of a coherent regional dynamo pattern. The variability in Region F, located along a very different longitude swath, could then be associated with a different longitudinally-controlled dynamo pattern.
We can also evaluate the vector dispersions (or, in principle, the VGP dispersions) associated with each PSV record. Figures 6, 7 show that there are two intervals of enhanced vector dispersion in the last 70 ka—~30–42 and ~60–65 ka (black horizontal bars in Figures 6, 7). This pattern is the same in all three studied regions. The pattern does not go hand-in-hand with the scalar patterns of inclination and declination variability. Also, it appears that the general “level” of vector dispersion, estimated by horizontal lines in Figures 6, 7, is quite a bit lower in each record than the two, relatively narrow, intervals of enhanced vector dispersion. Also, the general “level” of vector dispersion is significantly lower than the long-term average vector dispersion for each record noted in Table 1. It seems that this lower level of vector dispersion may be the more proper parameter for considering the “level” of dynamo activity in a region over time. The higher long-term averaged dispersion (Table 1) is then associated with the average of two different “states” of the local dynamo activity—one that is lower in dispersion and operates for most of the time and a second “state” that is higher in dispersion, but operates only for a more limited portion of the time.
Relationship of Long-Term Directional PSV to Excursions
There are three excursions reported repeatedly during the last glacial cycle: the Mono Lake Excursion (34 ka; Liddicoat and Coe, 1979), the Laschamp Excursion (41 ka; Bonhommet and Babkine, 1967; Bonhommet and Zahringer, 1969) and an unnamed excursion in Stage 4 (61 ka; Nowaczyk and Baumann, 1992; Nowaczyk et al., 1994). The excursion times are shown as vertical dotted lines in Figures 6, 7, 9–11. These excursions are all associated with intervals of notably higher field vector dispersion (Figures 6, 7) and low paleointensity (Figures 9–11).
The higher vector dispersion, in each case, occurs even though the actual excursional field directions in all-time series were deleted from the statistical analyses using a 45° latitude cutoff for VGPs (e.g., Johnson et al., 2008). This suggests that field variability surrounding excursions is routinely more high-amplitude even when individual directions are not excursional. The evidence is not certain, however, that higher amplitude dispersion really precedes excursion intervals, an observation previously noted for the Mono Lake Excursion (Liddicoat and Coe, 1979; Lund et al., 1988). Regions C, E, and F share the relationship of higher amplitude dispersion associated with excursions even though the overall pattern of directional variability in Region F is significantly different from the other two regions. One possibility is that the low paleointensity associated with all the excursional intervals is the driving force in causing higher amplitude dispersions and as well as localized excursions in selected regions.
There is no indication that directional statistical parameters in Regions C, E, or F behave in an anomalous manner before excursional intervals. This might indicate that there are no distinctive changes in the local dynamo convection process before excursions. Rather, it may be that the common factor is the diminished field intensity.
Relationship of Long-Term Directional PSV to Paleointensity
Figure 8 shows the relative paleointensity variability of the three Region C PSV records. There is a strong sense of centennial- to millennial-scale variability in these records that is coherent among them. They have 47 features in common between 15 and 60 ka. The directional and paleointensity spectra of these three PSV records are summarized in Lund (2007). Furthermore, Figure 9 shows the relative paleointensity variability between Regions C, E, and F. There appears to be a similar coherent pattern of variability among all three regions on at least a millennial scale. This is consistent with other estimates that paleointensity is a coherent process on a global scale (e.g., Channell et al., 2009).
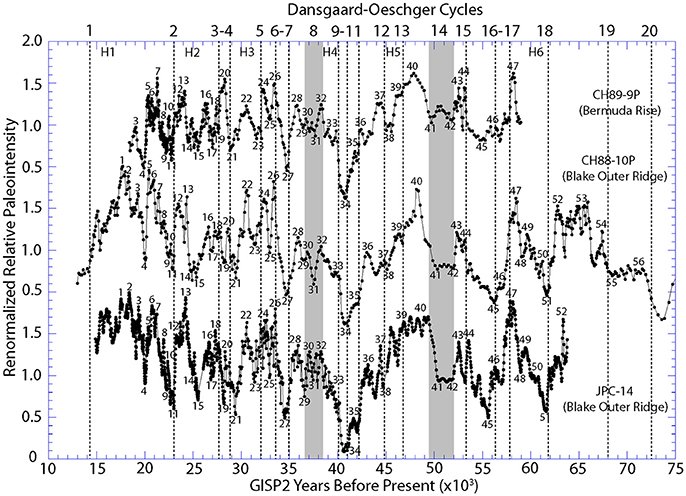
Figure 8. Paleointensity variability in Region C. The paleointensity variability of three Region C PSV records are plotted. Fifty two replicate paleointensity maxima/minima are identified. Selected Dansgaard/Oeschger (D/O) Cycles are noted for chronologic control. Gray zones document two particular D/O cycles (8, 14) that are longer in duration.
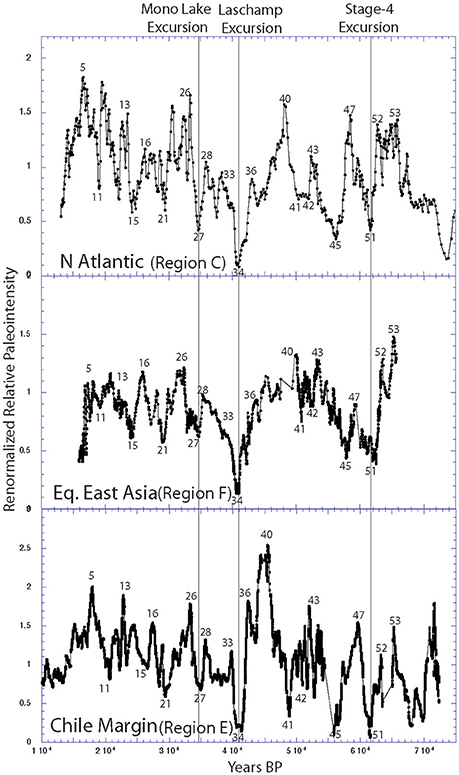
Figure 9. A comparison of paleointensity variability among regions C, E, and F. Selected intensity features noted in Figure 8 are correlated to the other two regions. Vertical lines indicate where the Mono Lake, Laschamp, and Stage-4 excursions occur.
The Regions C, E, and F paleointensity records in Figure 9 have been averaged in 3- and 9-ka intervals just like the directional records in Figures 6, 7. The results are shown in Figure 10. As expected, all three regions share a common pattern of paleointensity variability during the last glacial cycle. These three high-resolution paleointensity records can be compared with the PISO-1500 compilation of Channell et al. (2009) in Figure 11, based on 13 stacked paleointensity records from around the World (average sediment accumulation rate of 11 cm/kyr). The 3–9 ka averaged results from Figure 10 are consistent with the stacked PISO-1500 records (labeled features 2–9 in Figures 10, 11).
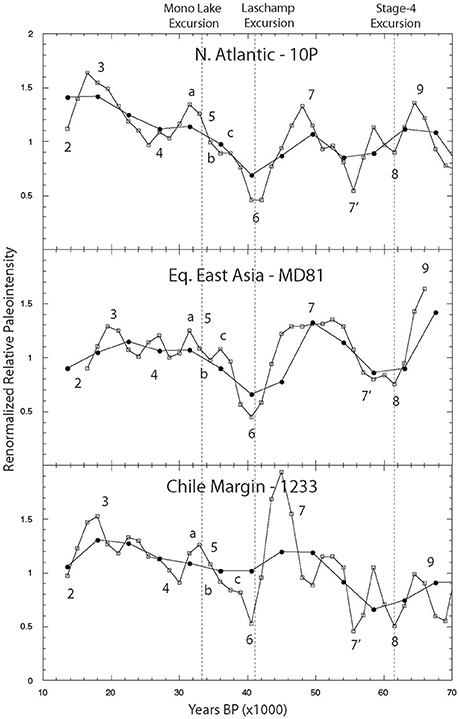
Figure 10. A comparison of average paleointensity in 3 and 9 ka intervals for Regions C, E, and F. Correlatable paleointensity features are numbered 2–9. Vertical lines indicate where the Mono Lake, Laschamp, and Stage-4 excursions occur.
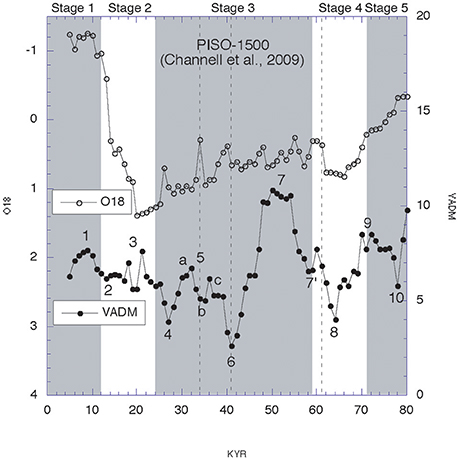
Figure 11. PISO-1500 record of global-averaged paleointensity for the last 80 ka with associated oxygen-isotope stratigraphy for chronostratigraphic control (from Channell et al., 2009).
There is a general sense in the paleomagnetic community (e.g., Merrill et al., 1998) that local directional field variability is inversely proportional to field intensity with low intensity associated with larger field variability and excursions. The three regions in Figure 10 do show low paleointensity during the three excursion intervals (dashed lines) with anomalously high directional dispersion (Figures 6, 7). Similarly, low directional dispersions in all three regions are clearly associated with high intensity intervals around 18 and 48 ka. This does suggest, that regional vector and paleointensity variability are inversely correlated globally even though regional inclination and declination variability (noted above) are not. Correlation of inclination and paleointensity variability at three IODP sites In the Bering Sea (Lund et al., 2017b) for the entire Brunhes Epoch (last 780 ka) corroborate this pattern.
Discussion
Traditional long-term PSV studies provide a sense of averaged field characteristics on a global scale (SHA or latitude/longitude transects) with averaging over ~106 years. This study estimates averaged field characteristics within limited regions on timescales of 103-104 years. The question is how do these estimates compare and what might we conclude about dynamo activity from their differences?
The PSV data from Region C (subtropical Atlantic Ocean) show strong directional variability on time scales of 103-104 years. The scalar inclinations and declinations display ~30° variations over 3-ka averaging and ~15° variations over 9-ka averaging. The source of that variability must be dynamo activity, with most coming from dynamo activity directly below Region C in the outer core. The fact that all three PSV records in Region C show the same statistical pattern of long-term averaged field suggests that a similar degree of spatial coherence in dynamo activity operates in the outer core. The 9-ka averages are comparable to averaging, at any locality, the entire Holocene variability noted in many recent SHA studies (e.g., Constable et al., 2016). This indicates that the Holocene is not sufficient to characterize the entire space/time pattern of dynamo activity. It also suggests that there is significant regional-scale dynamo activity operating on a 104 years scale (as suggested by Lund et al., 1988). It may be that such PSV analyses may provide a new perspective on the actual space/time pattern of fluid flow and magnetic-flux regeneration that occurs regionally in the outer core.
The PSV data from Regions E and F corroborate the overall style of long-term directional field variability noted in Region C. It is likely that these three regions, together, reflect the overall long-term style of directional variability expected in any region on a global scale over 103-104 years (e.g., Creer et al., 1983; Merrill et al., 1998; Lund, 2007). The detailed pattern of long-term directional variability for Regions C and E is consistent between them, but neither is consistent with Region F. Previous studies have suggested that directional variability in different regions should be different (e.g., Creer et al., 1983; Lund, 2007). So, the fact the Region F is different from Region C or Region E should not be a surprise. The surprise is that Regions C and E appear to be similar. One explanation for that is that the regional-scale dynamo activity is correlated between those two regions in the outer core. Some previous conceptual models of dynamo activity have focused on “convection rolls” as one source for dynamo activity; these convection rolls might operate in longitudinally-constrained swaths of the outer core between northern hemisphere and southern hemisphere high-latitude flux lobes (Bloxham and Gubbins, 1985). Regions C, D (work in progress), and E might all be associated with such a zone of coherent dynamo activity.
The long-term variability in field-vector dispersion is comparable in all three studied regions, even though the details of directional PSV are different in each region (or at least in Region F vs. Regions C and E). The long-term, 105 years average, vector dispersion for each region (Table 1) is comparable to 106 years averages (Figure 2), as well. But, the overall pattern of vector dispersion in each region is characterized by significantly lower vector dispersions for ~75% of the time and significantly high vector dispersion for ~25% of the time. This suggests that the vector dispersion on 103-104 years time scales is much <106 years averages predict. This has been noted before (e.g., Lund, 1985; Lund et al., 2017b). It suggests that dynamo activity operates with a lower range of variability for most time, but is punctuated by shorter times of higher variability.
Long-term intensity variability on 103-104 years timescales is largely synchronous in all studied regions. This has been noted previously in global studies of paleointensity (e.g., Channell et al., 2009). The intervals of lowest intensity (features 6 and 7′/8 in Figure 10) occur at times of largest vector dispersion noted above. This relationship of higher (lower) directional field variability associated with low (high) paleointensity has been noted in other paleomagnetic studies (e.g., Merrill et al., 1998; Lund et al., 2016b). This indicates that vector and intensity variability are global in their pattern even though the details in style of vector variability differ from region to region. This suggests some kind of “connectedness” in the energy content of dynamo activity on a global scale, even though the detailed pattern of dynamo activity may be different from region to region. One speculation is that fluid-flow and flux-regeneration in each region generates toroidal field that connects regions and gives a coherence to the overall energy state of the core dynamo, but region-specific aspects of the fluid-flow and flux regeneration creates poloidal field that is largely region-specific and uncorrelated on a global scale.
The lowest intensity intervals with largest vector dispersion are also times of magnetic field excursions. True excursional directions do not always occur in all of our studied regions. But, enhanced vector dispersion does occur in all excursion intervals. (Remember, the vector dispersions were calculated after all true excursional directions with VGP latitudes <45° N were removed.) This suggests that directional excursions are a characteristic of low intensity intervals. It appears that high vector dispersion is also a characteristic of low intensity intervals. As such, it seems that low intensity is the fundamental feature and driving force (?) for excursions and high vector dispersions. In as much as low vector dispersion dominated for ~75% of our study interval (last ~70 ka), it may be that one can argue the field operates in a normal mode for most time and a separate, distinctive low-intensity (excursional) mode for a smaller portion of time. The low-intensity state may intrinsically yield a more complicated fluid-flow and flux regeneration pattern than high-intensity intervals. This may produce even smaller regional-scale variability in both directions and intensity.
Conclusions
This study carried out a statistical analysis of high-resolution PSV records for the last ~70 ka from three different regions of the Earth. We consider directional and intensity variability in each region on time scales of 103-105 years in order to evaluate long-term PSV. We then compare those results with more traditional long-term PSV statistical studies averaged over ~106 years.
Three replicate PSV records from one region (C, subtropical North Atlantic Ocean) were averaged at overlapping 3 and 9 ka intervals. Variability in both scalar inclination and declination variability and vector angular dispersion are significant and coherent among the three records. The vector dispersion is relatively low for most of the time but contains two relatively narrow intervals (~25–45 and 60–65 ka) of high dispersion. (Vector dispersion in all records was calculated after removing directions with true excursional VGPs, VGPs < 45° N).
We have carried out a comparable statistical analysis on two other PSV records from other parts of the Earth (Region E, Chile margin; Region F, Philippines/Indonesia). The results for these two records are comparable to Region C in their overall style of variability. The scalar directional variability from Region F is quite different in detail from those of Regions C and E, as might be expected, but the scalar directional variability between is Regions C and E is remarkably consistent considering their distance from one another. This may be associated with them being on the same longitude swath and having some coherent dynamo activity occurring along that path.
There is evidence for three magnetic field excursions in the studied interval—Mono Lake Excursion (~34 ka), Laschamp Excursion (~42 ka), and an unnamed excursion in OIS Stage 4 (~61 ka). These three excursions all occur in the lowest intensity intervals and highest vector dispersion intervals in all three regions. It seems likely that low intensity intervals in dynamo activity are distinctly correlated with enhanced vector dispersion and occurrence of excursions around the World.
Three paleointensity records from Region C were subjected to the same statistical analysis as the directions. These records are also coherent in their pattern of variability. We also carried out a statistical analysis on the paleointensity records from Regions E and F. They also displayed the same pattern and coherence in variability that is noted in Region C. That similarity in paleointensity variability on a global scale is expected even though the detailed scalar directional variability is not coherent on a global scale. The pattern of intensity variability is strongly correlated with the pattern of vector dispersion on a global scale—high (low) intensity is associated with low (high) vector dispersion. The excursions all occur in intervals of the lowest paleointensity in all regions.
Author Contributions
The author confirms being the sole contributor of this work and approved it for publication.
Funding
This work has been supported by several grants from the USA National Science Foundation (EAR1547605, NSF-OCE0962385, OCE-0082698, EAR-9980410, EAR-9526940).
Conflict of Interest Statement
The author declares that the research was conducted in the absence of any commercial or financial relationships that could be construed as a potential conflict of interest.
References
Blanchet, C., Thouveny, N., and Garidel-Thoron, T. (2006). Evidence for multiple paleomagnetic intensity lows between 30-50 ka BP from a western Equatorial Pacific sedimentary sequence. Quat. Sci. Rev. 25, 1039–1052. doi: 10.1016/j.quascirev.2005.09.001
Bloxham, J., and Gubbins, D. (1985). The secular variation of the Earth's magnetic field. Nature 317, 777–781. doi: 10.1038/317777a0
Bonhommet, N., and Babkine, J. (1967). Sur la presence d'aimentation inverse dans la Chaine des Puys. C.R. Hebs. Seances Acad. Sci. Ser. B. 264, 92–94.
Bonhommet, N., and Zahringer, J. (1969). Paleomagnetism and potassium argon determinations of the Laschamp geomagnetic polarity event. Earth Planet. Sci. Lett. 6, 43–46. doi: 10.1016/0012-821X(69)90159-9
Bullard, E. C., Freedman, C., Gellman, H., and Nixon, J. (1950). The westward drift of the Earth's magnetic field. Philos. Trans. R. Soc. Lond. A 243, 67–92.
Channell, J., Xuan, C., and Hodell, D. (2009). Stacking paleointensity and oxygen isotope data for the last 1.5 Ma (PISO-1500). Earth Planet. Sci. Lett. 283, 14–23. doi: 10.1016/j.epsl.2009.03.012
Chevaliier, R. (1925). L'aimantation des laves de l'Etna et l'orientation du champ terrestre en siscile du XII and XVII siècle. Ann. Phys. 4, 5–162.
Constable, C., Korte, M., and Panovska, S. (2016). Persistern high paleosecular variation activity in the southern hemisphere for the last 10,000 years. Earth Planet. Sci. Lett. 453, 78–86. doi: 10.1016/j.epsl.2016.08.015
Creer, K., Tucholka, P., and Barton, C. (1983). Geomagnetism of Baked Clays and Recent Sediments. Amsterdam: Elsevier Publishing.
Gubbins, D., and Bloxham, J. (1987). Morphology of the geomagnetic field and implications for the geodynamo. Nature 325, 509–511. doi: 10.1038/325509a0
Harrison, C. (2007). “Secular variation model,” in Encyclopedia of Geomagnetism and Paleomagnetism, eds D. Gubbins and E. Herror-Bervera (Springer Publishing), 892–901.
Huang, K., Oppo, D., and Curry, W. (2014). Decreased influence of Antarctic intermediate water in the tropical Atlantic during North Atlantic cold events. Earth Planet. Sci. Lett. 389, 200–208. doi: 10.1016/j.epsl.2013.12.037
Jackson, A., Jonkers, A., and Walker, M. (2000). Four centuries of geomagnetic secular variation from historical records. Philos. Trans. R. Soc. Lond. A 358, 957–990. doi: 10.1098/rsta.2000.0569
Johnson, C., and Constable, C. (1996). Paleosecular variation recorded by lava flows over the last five million years. Philos. Trans. R. Astron. Soc. A 354. 89–141. doi: 10.1098/rsta.1996.0004
Johnson, C. L., Constable, C. G., Tauxe, L., Barendregt, R., Brown, L. L., Coe, R. S., et al. (2008). Recent investigations of the 0-5 MA geomagnetic field recorded by lava flows. Geochem. Geophys. Geosys. 9:Q04032. doi: 10.1029/2007GC001696
Johnson, E. A., Murphy, T., and Torreson, O. W. (1948). Pre-history of the Earth's magnetic field. J. Geophys. Res. 53, 349–372.
Keigwin, L., Rio, D., and Acton, G. (1998). Initial Results from ODP Leg 172, Ocean Drilling Program. College Station, TX.
Liddicoat, J. C., and Coe, R. S. (1979). Mono Lake geomagnetic excursion. J. Geophys. Res. 84, 261–271. doi: 10.1029/JB084iB01p00261
Lund, S. (1985). A comparison of the late Quaternary statistical secula variation that is recorded in lava flows and both wet and dry sediments. Geophys. Res. Lett. 12, 251–254. doi: 10.1029/GL012i005p00251
Lund, S. (2007). “Paleomagnetic secular variation,” in Encyclopedia of Geomagnetism and Paleomagnetism, eds D. Gubbins and E. Herror-Bervera (Springer Publishing), 766–775.
Lund, S., Benson, L., Negrini, R., Liddicoat, J., and Mensing, S. (2017a). A full-vector paleomagnetic secular variation record (PSV) from Pyramid Lake (Nevada) from 47-17 ka: evidence for the successive Mono Lake and Laschamp Excursions. Earth Planet. Sci. Lett. 458, 120–129. doi: 10.1016/j.epsl.2016.09.036
Lund, S., Keigwin, L., and Darby, D. (2016a). Character of paleomagnetic secular variation in the tangent cylinder: evidence from the Chukchi Sea. Phys. Earth Planet. Int. 256, 49–58. doi: 10.1016/j.pepi.2016.03.005
Lund, S., Liddicoat, J., Lajoie, K., Henyey, T., and Robinson, S. (1988). Paleomagnetic evidence for the long-term (104 year) memory and periodic behavior in the Earth's core dynamo process. Geophys. Res. Lett. 15, 1101–1104.
Lund, S., Oppo, D., and Curry, W. (2017b). Late quaternary paleomagnetic secular variation recorded in deep-sea sediments from the Demerara Rise, Equatorial west Atlantic Ocean. Phys. Earth Planet. Int. 272, 12–24. doi: 10.1016/j.pepi.2017.04.010
Lund, S., Schwartz, M., and Stott, L. (2018). Long-term paleomagnetic secular variation and excursions from Equatorial Pacific Ocean (MIS2-4). Geophys. J. Int. 209:ggx029. doi: 10.1093/gji/ggx029
Lund, S., Stoner, J., and Lamy, F. (2007). “Late quaternary paleomagnetic secular variation records and chronostratigraphy from ODP sites 1233 and 1234,” in Proceedings of the Ocean Drilling Project, Scientific Results, Vol. 202, eds A. Mix, R. Tiedeman, and P. Blum.
Lund, S., Stoner, J., Okada, M., and Mortazavi, E. (2016b). Paleomagnetic field variability and Chronostratigraphy of Brunhes-Epoch deep-sea sediments from the Bering Sea: IODP Expedition 323. Deep Sea Res. II 125–126, 107–116. doi: 10.1016/j.dsr2.2016.02.004
Lund, S., Williams, T., Acton, G., Clement, B., and Okada, M. (2001). “Brunhes Epoch magnetic field excursions recorded in ODP Leg 172 sediments,” in Proceedings of the Ocean Drilling Project, Scientific Results, Vol. 172, eds L. Keigwin, D. Rio, and G. Acton.
Lund, S. P. (1996). A comparison of Holocene paleomagnetic secular variation records from North America. J. Geophys. Res. 101, 8007–8024. doi: 10.1029/95JB00039
Lund, S. P., Acton, G. D., Clement, B., Okada, M., and Williams, T. (2001). “Paleomagnetic records of Stage 3 excursions from ODP Leg 172 sediments,” in Proceedings of the Ocean Drilling Project, Scientific Results, Vol. 172, eds L. Keigwin, D. Rio, and G. Acton.
McDougall, I., Saemundsson, K., Watkins, N., and Kristjansson, L. (1977). Extension of the geomagnetic timescale to 6.5 ma: k-ar dating, geological, and paleomagnetic study of a 3,500 m lava succession in western Iceland. Geol. Soc. Am. Bull. 88, 1–15.
McElhinny, M., McFadden, P., and Merrill, R. (1996). The time-averaged paleomagnetic field 0-65 Ma. J. Geophys. Res. 101, 25007–25027. doi: 10.1029/96JB01911
McNish, A. G., and Johnson, E. A. (1938). Magnetization of unmetamorphosed varves and marine sediments. J. Geopjys. Res. 53, 401–407. doi: 10.1029/TE043i004p00401
Merrill, R., McElhinny, M., and McFadden, P. (1998). The Magetic Field of the Earth: Paleomagnetism, the Cores, and the Deep Mantle. International Geophysics Series, Vol. 63. San Diego, CA: Academic Press.
Mix, T. R., and Blum, P. (2003). Initial Results from ODP Leg 202, Ocean Drilling Program. College Station, TX.
Nace, T., Baker, P., Dwyer, G., Silva, C., Rigsby, C., Burns, S., et al. (2014). The role of North Brazil Current transport in the paleoclimate of the Brazilian North margin and paleoceanography of the western tropical Atlantic during the Late Quaternary. Palaeog. Palaeoclim. Palaeoecel. 415, 3–13. doi: 10.1016/j.palaeo.2014.05.030
Negrini, R., Erbes, D., Faber, K., Herrera, A., Roberts, A., Cohen, A., et al. (2000). A paleoclimate record for the last 250,000 years from Summer Lake, Oregon, USA, I Chronology and magnetic proxies of lake level. J. Paleolim. 24, 125–149. doi: 10.1023/A:1008144025492
Nowaczyk, N. R., Frederichs, T. W., Eisenhauer, A., and Gard, G. (1994). Magnetostratigraphic data from late Quaternary sediments from the Yermak Plateau, Arctic Ocean: evidence for four geomagnetic polarity events within the last 170 ka of the Brunhes Chron. Geophys. J. Int. 117, 453–471. doi: 10.1111/j.1365-246X.1994.tb03944.x
Nowaczyk, N., and Baumann, M. (1992). Combined high-resolution magnetostratigraphy and nannofossil biostratigraphy for late Quaternary Arctic Ocean sediments. Deep-Sea Res. 39, 567–701. doi: 10.1016/S0198-0149(06)80021-X
Opdyke, N. (1972). Paleomagnetism of deep-sea cores. Rev. Geophys. Space Phys. 10, 213–249. doi: 10.1029/RG010i001p00213
Schwartz, M., Lund, S. P., and Johnson, T. C. (1998). Geomagnetic field intensity from 12,000-71,000 years BP as recorded in deep sea sediments of the Blake Outer Ridge, North Atlantic Ocean. J. Geophys. Res. 103, 30407–30416. doi: 10.1029/1998JB900003
Stott, L., Poulsen, C., and Lund, S. P. (2002). Super ENSO and global climate change oscillations at millennial time scales. Science 297, 222–226. doi: 10.1126/science.1071627
Takahashi, K., Ravelo, C., Alvarez Zarikian, C., Guèrin, G., Liu, T., Aiello, T., et al. (2011). “Bering Sea paleoceanography: Plio-pleistocene paleoceanography and climate history of the Bering Sea,” in Integrated Ocean Drilling Program Expedition 323 Scientists, Initial Report 323.
Thellier, E. (1937a). Ainantation des terres ciutes: application a la recherché de l'intensite du champ magnetique terrestre dans le passé. C. R. Acad. Sci. Paris 204, 184–186.
Thellier, E. (1937b). Recherché de l'intenste du champ magnetique terrestre dans le passé: premier resultats. Ann. Inst. Phys. Globe Univ. Paris 15, 179–184.
Thellier, E., and Thellier, O. (1951). Sur la direction du champ magnetique terrestre, retrouvee sur des parois de fours des epoques punique et romaine, a Carthage. C. R. Acad. Sci. Paris 233, 1476–1478.
Thellier, E., and Thellier, O. (1952). Sur la direction du champ magnetiques terrestre, dans la regions de Treves, vers 380 apres J-C. C. R. Acad. Sci. Paris 234, 1464–1466.
Yukutake, T. (1967). The westward drift of the Earth's magnetic field in historic times. J. Gemag. Geoelect. 19, 103–116.
Yukutake, T., and Tachinaka, H. (1968). The non-dipole part of the Earth's magnetic field, Bull. Earthquake Res. Inst. Univ. Tokyo 46, 1027–1074.
Zic, M., Negrini, R., and Wigand, P. (2002). Evidence of synchronous climate change across the Northern Hemisphere between the North atlantic and the northwestern Great Basin, USA. Geology 30, 635–638. doi: 10.1130/0091-7613(2002)030<0635:EOSCCA>2.0.CO;2
Appendix 1
Data quality required for high-resolution, long-term PSV studies of sediment sequences.
Minimum requirements:
1) Average sediment accumulation rates of >~20 cm/key.
2) inclination and declination waveform variability in individual records (good serial correlation between successive directional measurements).
3) replicate waveform variability (both inclination and declination) at local site (or at least <4,000 km region).
4) good quality age control at site (C14, oxygen isotopes) or through PSV correlations to other well-dated sites.
5) Overall correlation among replicate records of inclination and declination with consistent phase relationship between both parameters.
Preferred additional requirements:
6) paleointensity variability on same scale as directional variability in individual records.
7) replicate paleointensity variability at local site (or at least <4,000 km region).
8) Overall correlation among replicate records of inclination, declination, and paleointensity with consistent phase relationship among the three scalar parameters.
Keywords: secular variation, excursions, paleointensity, dynamo, paleomagnetism
Citation: Lund SP (2018) A New View of Long-Term Geomagnetic Field Secular Variation. Front. Earth Sci. 6:40. doi: 10.3389/feart.2018.00040
Received: 14 December 2017; Accepted: 04 April 2018;
Published: 04 May 2018.
Edited by:
Christopher Davies, University of Leeds, United KingdomReviewed by:
Geoffrey Cromwell, United States Geological Survey, United StatesPeter Aaron Selkin, University of Washington Tacoma, United States
Copyright © 2018 Lund. This is an open-access article distributed under the terms of the Creative Commons Attribution License (CC BY). The use, distribution or reproduction in other forums is permitted, provided the original author(s) and the copyright owner are credited and that the original publication in this journal is cited, in accordance with accepted academic practice. No use, distribution or reproduction is permitted which does not comply with these terms.
*Correspondence: Steve P. Lund, c2x1bmRAdXNjLmVkdQ==