- 1School of Energy Resources, China University of Geosciences (Beijing), Beijing, China
- 2Key Laboratory of Marine Reservoir Evolution and Hydrocarbon Enrichment Mechanism, Ministry of Education, China University of Geosciences (Beijing), Beijing, China
In sedimentary basins, the lithologic characteristics of mudstone in different lithofacies differ, which is caused by the different types of diagenesis after sedimentation. An accurate understanding of the changes in and interactions among minerals in mudstone during diagenesis plays a key role in shale oil and gas exploration and development. In this work, the petrologic characteristics, XRD data, carbon and oxygen isotope data, main trace element data, and rock pyrolysis data of a total of 137 samples from 3 wells were used to divide the studied mudstone into 5 different types of lithofacies. For each rock phase and all kinds of primary minerals, the changes in secondary minerals and the relationships among them were studied. The five types of lithofacies belong to a relatively closed diagenetic system. The main development space of fibrous calcite/ankerite is laminar fractures formed during the sedimentary period. Ca2+, Mg2+ and Fe2+ ions are provided by early carbonate and smectite–illite transformation. According to the temperature calculated from the δ18O values, the formation time of fibrous calcite/ankerite is the same as that of organic acid production. A large amount of fine quartz is formed by the transformation of clay minerals, and SiO2 precipitation is formed by the dissolution of terrigenous feldspar. The authigenic albite precipitates in carbonate dissolution pores when the production of organic acids is reduced. Authigenic carbonate affects the smectite‒illite transformation process by influencing the ion concentration in the pore fluid. Pyrite transforms from colloidal pyrite formed by the action of BSR in the early stage of diagenesis and affects the concentration of Fe2+ ions in the early pore fluid. The contents and types of organic matter in different lithofacies differ, which directly leads to differences in pore fluid pressure and organic acid concentration during the thermal evolution stage of organic matter, thus affecting the diagenesis of different lithofacies.
1 Introduction
In recent years, shale oil and gas exploration has increased considerably in many countries, especially North America and China (U.S. Energy Information Administration, 2020). The shale oil and gas resources in North America are derived mainly from marine organic-rich shales such as the Barnett shale (Montgomery et al., 2005; Loucks et al., 2009), Marcellus shale (Milliken et al., 2013) and Haynesville shale. Continental organic-rich shale systems are widely distributed in China, such as the Cretaceous Qingshankou Formation in the Songliao Basin (Huang et al., 2013), the Triassic Yanchang Formation in the Ordos Basin (Cao et al., 2015; Wu et al., 2015), the Jurassic Ziliujing Formation in the Sichuan Basin (Nie et al., 2017), the Palaeogene Shahejie Formation (Li, 2015; Sun, 2017; Zhang et al., 2018) and the Kongdian Formation (Zhao et al., 2018) in the Bohai Bay Basin.
With the development of shale exploration and theoretical research, an increasing number of scholars are concerned with the change in shale porosity during diagenesis (Wu et al., 2017; Hu et al., 2019). The change in porosity is related mainly to the diagenetic evolution of minerals (Bilal et al., 2022a), the thermal evolution of organic matter (Chen and Xiao, 2014) and the development of fractures (Ma et al., 2020). Fibrous calcite (including beef veins and cone-in-cone structures) is a common mineral occurrence in mudstone; it is commonly found in black shale rich in organic matter and carbonate rock and has attracted extensive attention (Durney and Ramsay, 1973; Marshall, 1982; Cobbold et al., 2013; Weger et al., 2019; Song et al., 2020). Studies have shown that fibrous calcite formation is related to hydrocarbon generation overpressure (Zhang et al., 2016; Bilal et al., 2022b) and dissolution of carbonate formed during the sedimentary period (Luan et al., 2019). In the process of clay mineral transformation, large amounts of Si, Na, Ca, Mg, and Fe are discharged into the pore mixture and precipitate into quartz, feldspar, chlorite, and carbonate (Hower et al., 1976; Boles and Franks, 1979; Abercrombie et al., 1994; Bjørlykke, 1998), affecting the density and hardness of the reservoir (Yang et al., 2018; Xi et al., 2019). Strawberry pyrite may be converted from mackinaite formed by bacterial sulfate reduction (BSR) (Marin-Carbonne et al., 2014). Organic matter can form many organic acids and CO2 during thermal evolution (Lundegard et al., 1984; Barth and Bjørlykke, 1993; Seewald, 2003), and the amount of organic acids and CO2 production are closely related to the type of organic matter (Hu et al., 2020). When these fluids enter the pore fluid of mudstone, the diagenetic environment inside the mudstone changes.
This study investigates mudstone of the Es3x and Es4s submembers of the Dongying Depression, Bohai Bay Basin. The division of lithofacies and diagenesis of each lithofacies are investigated based on the petrology, mineral composition, stable isotope characteristics, major and trace element characteristics, and rock pyrolysis parameters of mudstone. The interactions between these minerals and minerals, between minerals and organic matter, and their secondary changes during burial constitute the so-called “coevolution of minerals.” This evolution is crucial for the development of sweet spots. The main objectives of this study are (1) to explain the diagenesis types and diagenetic evolution of mudstone under lithofacies constraints and (2) to explore the evolution and relationships of primary and secondary minerals in each stage of petrogenesis. These works help to understand mineral development in mudstones and identify potential “sweet spots.” This information is helpful for understanding how diagenesis changes the physical properties of mudstone, which is highly important for shale oil and gas exploration and development.
2 Geologic setting
The Dongying Depression is a typical lacustrine half-graben located in the Bohai Bay Basin in eastern China (Figure 1A). The Dongying Depression covers an area of approximately 5,000 km2 and is adjacent to the Chenjiazhuang uplift to the north; the Luxi uplift and Guangrao uplift to the south; the Qingcheng uplift to the west; and the Qingtuozi uplift to the east (Figure 1B). It contains Palaeogene, Neogene and Quaternary strata, including the Shahejie (Es), Dongying (Ed), Guantao (Ng), Minghuazhen (Nm) and Pingyuan (Qp) formations, in ascending order. The Es Formation can be further divided into members Es4 (lower Es4x Submember and upper Es4s Submember) and Es3 (lower Es3x Submember, middle Es3z Submember, upper Es3s Submember) (Figures 1C, D). This depression hosts five secondary tectonic zones from south to north: the southern gentle slope zone, the southern zone (Niuzhuang Sag), the central anticline zone, the northern zone (Lijin Sag), and the northern steep slope zone (Figure 1D), with a central local thickness of approximately 5,000 m (Chen et al., 2007; Guo et al., 2010).
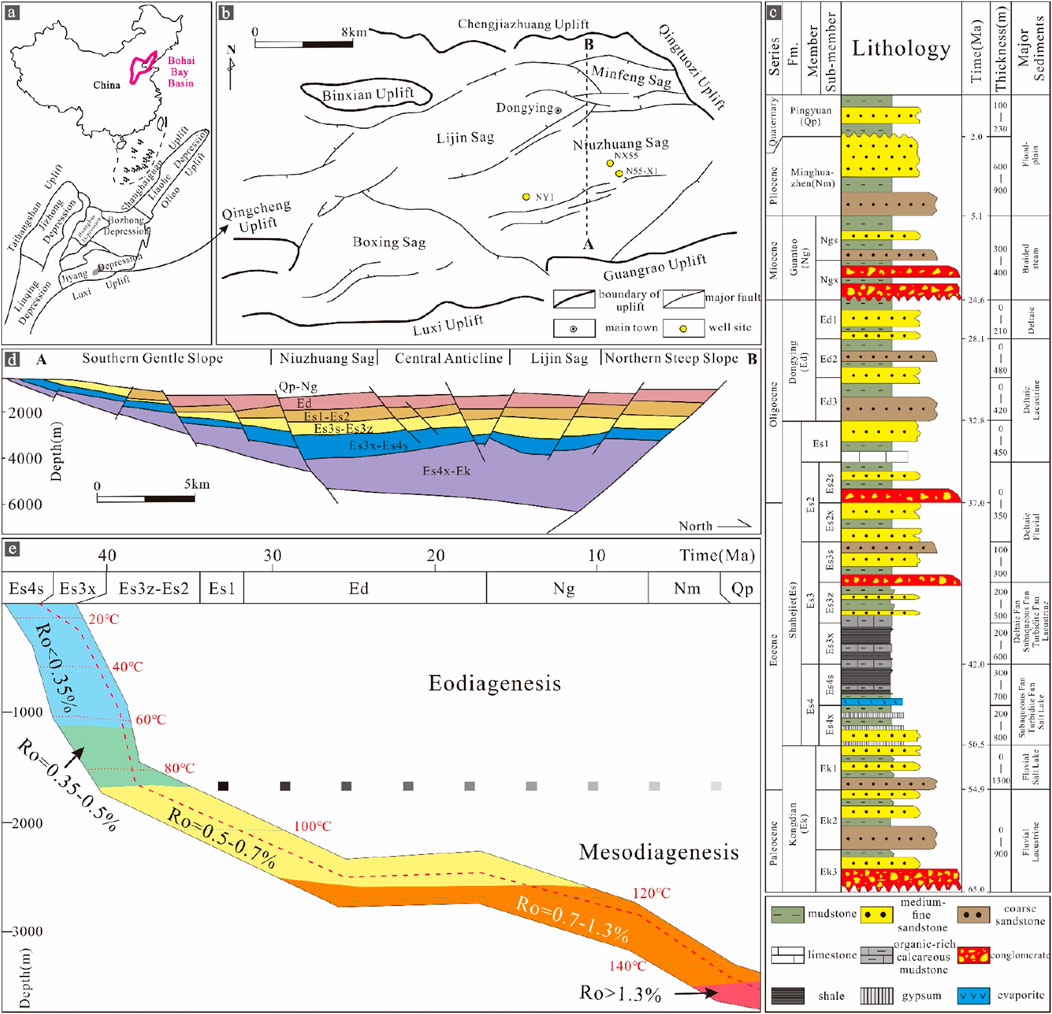
Figure 1. Basic information of the study area: (A) Location of the Bohai Bay Basin (Ma et al., 2021). (B) Structural features of the Dongying Depression and the sampling well locations in the study area. (C) Generalized Cenozoic–Quaternary stratigraphy of the Dongying Depression (Luan et al., 2019). (D) Cross-section AB showing the different tectonic–structural zones and key stratigraphic intervals (Guo et al., 2010). (E) Thermal and burial history of Es3x and Es4s and the general stages of diagenesis.
This study focuses on Es4s and Es3x. The Dongying Depression began to expand after the Mesozoic and reached its maximum diameter (>50 m) during this period, when a series of fan and braided deltas developed along the lake’s edges, and the centre of the deep-water lake was filled with thick black shale (approximately 400 ms thick), which was the main source rock for the oil-bearing depression. These organic-rich laminated shales and massive mudstones have total organic contents (TOCs) ranging from 0.47 to 12.4 wt%. The organic matter is predominantly planktonic algae ditch whip algae, coccoliths, and Bohai algae. The shale is thermally mature, as supported by vitrinite reflectance (Ro) measurements (0.69%–1.21%) and Tmax values (435°C–460°C). The above data indicate that the shales of Es3x to Es4s in the Dongying Depression are in a stage of mesodiagenesis (Figure 1E).
3 Materials and methods
All the shale samples were taken from the cores of three wells (Figure 1B), and the total core length was 204.6 m. A total of 137 slices were impregnated with standard epoxy and partly stained with Alizarin Red S and K-ferricyanide for the differentiation of carbonate minerals. Optical photomicrographs were taken with plane-polarized light (PPL), cross-polarized light (XPL) and reflected light (RL) using an Axio Scope A2m Zeiss Polarizing Microscope. Fluorescence light (FL) photomicrographs were taken with a Leica Model DMRX HC polarization fluorescence microscope. Cathodoluminescence (CL) analyses were carried out with an Olympus microscope equipped with a CL8200-MK5 CL instrument with a beam voltage of 17 kV and a current of 600 μA. Backscattered electron (BSE) and scanning electron microscopy (SEM) observations were performed with a Nova Nano SEM 450.
FSEM with a built-in Oxford X-Max 80 energy spectrometer. The voltage was 15 kV, the spot size was 5.5 nm, and the working distance was 5.0–6.5 mm. A portion of each sample was polished with argon ions to observe the mineral pore characteristics. A rock analyser (ROCK-EVAL. II) and a carbon and sulfur analyser (CS-230HC) were used for rock pyrolysis analysis and total organic carbon (TOC) analysis. The X-ray powder diffraction (XRD) analysis instrument used was a Phillips XPert PW3710 diffractometer with CuKα (λ = 1.54060 Å) radiation, a voltage of 40 kV and a tube current of 35 mA. Major and trace element contents were determined using an X-ray fluorescence (XRF) Phillips PW2400 spectrometer with Rh-Kα radiation and a power of 2400 W. In the carbon and oxygen isotope analysis, fibrous calcite, micritic lamellar calcite, and dispersed ankerite were sampled with a microscopically mounted drill assembly, whereas mudstone samples (without fibrous calcite) were powdery. The powder was reacted with ultrapure phosphoric acid at 25°C in an inert atmosphere. The extracted CO2 was carried by helium gas through a column and transferred to a MAT251 isotope ratio mass spectrometer, where the gas was ionized and the isotope ratio was determined. All analyses are converted to VPDB.
4 Results
4.1 Lithofacies descriptions
For convenience, the mineral composition, sedimentary structure characteristics and organic matter content of the mudstone were determined. The mineralogical data, such as the core and XRD data (Figure 2), were plotted on a ternary plot, and five lithologies were defined: laminated clay-rich carbonate mudstone (L1), laminated mixed mudstone (L2), laminated clay-rich siliceous mudstone (L3), massive argillaceous carbonate mudstone (L4), and laminated argillaceous siliceous mudstone (L5). Their respective characteristics are described in detail below.
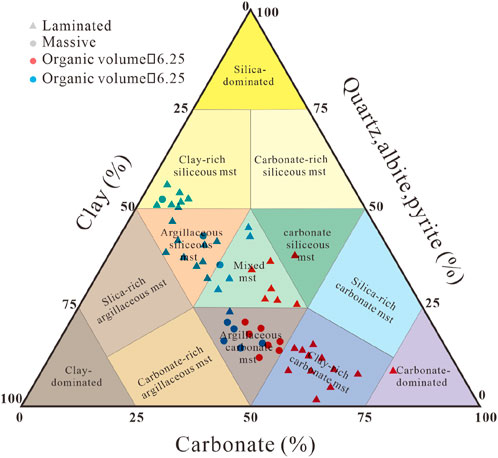
Figure 2. Three-end-member diagram of the mudstone mineral composition. The ternary diagram is simplified based on Diaz et al. (2013).
Laminated clay-rich carbonate mudstone (L1) contains many fibrous calcite laminae, which are usually symbiotic with the micritic calcite laminae that formed during deposition. There are also abundant carbonate minerals in the noncalcite laminae. The organic matter content is high, and the organic matter type is mostly type I. This type of lithofacies is generally regarded as the “sweet spot” of mudstone reservoirs. Laminated mixed mudstone (L2) contains a certain amount of fibrous calcite, but this type of lamina is significantly less dense than that in L1. The content of clay minerals increased significantly, and a small amount of siliceous lamina was noted. This change is related to the change in the sedimentary environment during lithofacies formation. This type of lithofacies is omitted in the subsequent analysis because the lithofacies minerals are diverse and can be considered a transitional type between L1 and L3 or L5. Its characteristics are repeated with other types of lithofacies, so it will not be further described. Laminated clay-rich siliceous mudstone (L3) contains a large amount of siliceous lamina, in which quartz, feldspar and certain amounts of carbonate minerals are distributed. The organic matter type of the lithofacies is II2–III, and the organic matter content is low. Massive argillaceous carbonate mudstone (L4) is characterized by a massive structure, in which the contents of clay and carbonate are high, mostly carbonate is distributed in clay minerals, the organic matter type is I1–II2, and the organic matter content is high. Laminated argillaceous siliceous mudstone (L5) also contains many siliceous laminae, but unlike those in L3, the siliceous laminae in this lithofacies are denser, the grains in the laminae are mainly quartz and clay minerals, the quartz particles are small, and the organic matter type is II1–II2 with moderate organic matter content.
4.2 Diagenetic minerals
4.2.1 Calcite
There are three main types of calcite: fibrous calcite, sparry calcite and micritic calcite. Sparry calcite is formed early by biological induction. The recrystallization of this calcite occurs during burial, and the degree of recrystallization is significantly greater in areas where fibrous calcite is densely developed (Figures 3A, B). Fibrous calcite occurs mainly in mud shales as fracture filler (Figures 3A, B). These fractures may be cracks in bedding, cracks caused by shrinkage during the diagenetic evolution of clay minerals, or structural fractures (Figure 3C). The CL characteristics of the two calcite types are significantly different, with fibrous calcite being brighter and showing a lower Fe content (Figures 3D, E). These two kinds of calcite are common in lithofacies L1 and L2.
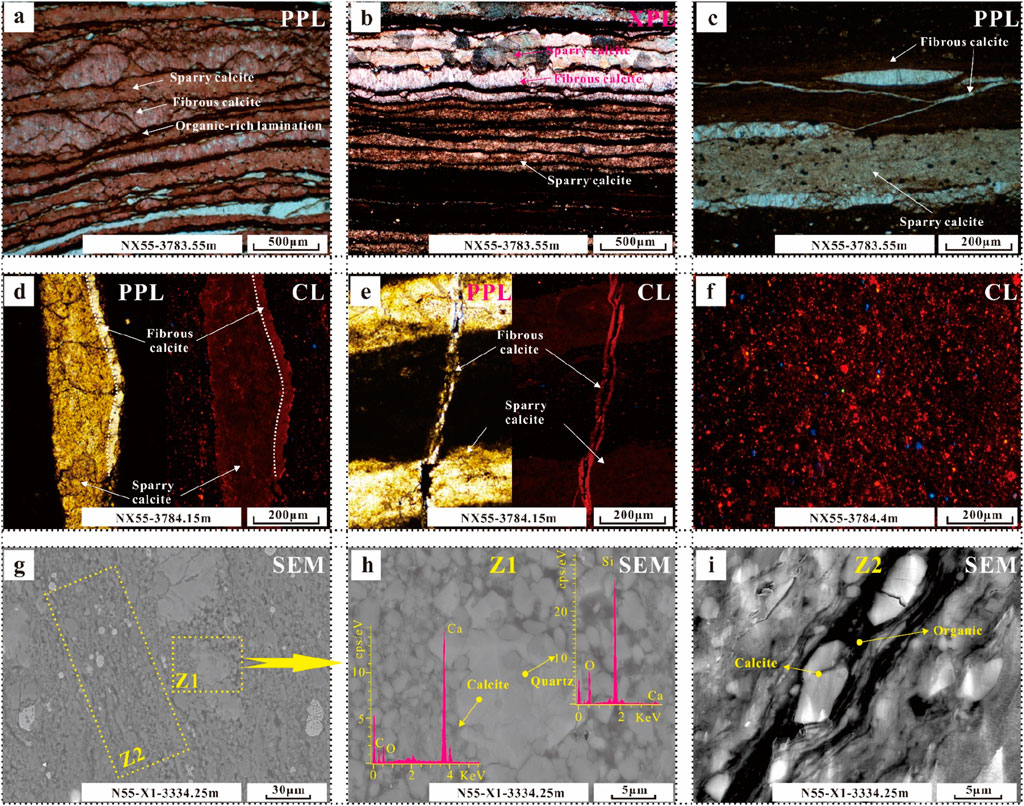
Figure 3. Photographs of the characteristics of calcite in different occurrences in various lithofacies. (A) Sparry calcite interbedded with fibrous calcite and occasionally exhibiting organic-rich laminae. (B) Some sparry calcite recrystallized to a high degree. (C) Fibrous calcite growing in cracks. (D) Contrast of CL between sparry calcite and fibrous calcite. (E) Sparry calcite in cracks. (F) Distribution of carbonate rocks in clay mineral beds. (G–I) Calcite of various occurrences and mineral associations in the clay mineral layers.
Micritic calcite is distributed mainly in regions with undeveloped laminae, and its Cl characteristics are similar to those of fibrous calcite laminae (Figure 3F). It is dispersed in clay minerals and has two different forms (Figure 3G): in Zone 1, calcite particles are large and often symbiotic with fine quartz particles (Figure 3H); in Zone 2, there is also some fine calcite in the bedding clay mineral layer (Figure 3I). This calcite is common in lithofacies L1, L2, L4 and L5.
4.2.2 Dolomite–ankerite
Dolomite-ankerite is abundant in only one section of well N55-X1, the lithofacies type is not as comprehensive as that of the NX55 well, and only L1 and L4 are characterized in the core of this calibre. Fibrous ankerite is layered (Figure 3A), and ankerite cements are found in some of the dissolved pores in the analcime (Figure 4B). The CL characteristics are obviously dark, resulting in a high Fe content (Figure 4C). One of the most common characteristics is the widely distributed foggy core/bright edge ferrite in clay minerals (Figure 4D), which is shaped like a diamond; its core is usually clay minerals or pyrite, and its core is obviously dissolved (Figures 4E, F). This ferrite is sometimes associated with organic materials (Figure 4G). The dolomite content in this section is also high (Figure 4H), with abundant clay minerals in the form of clumps and aggregates (Figure 4I).
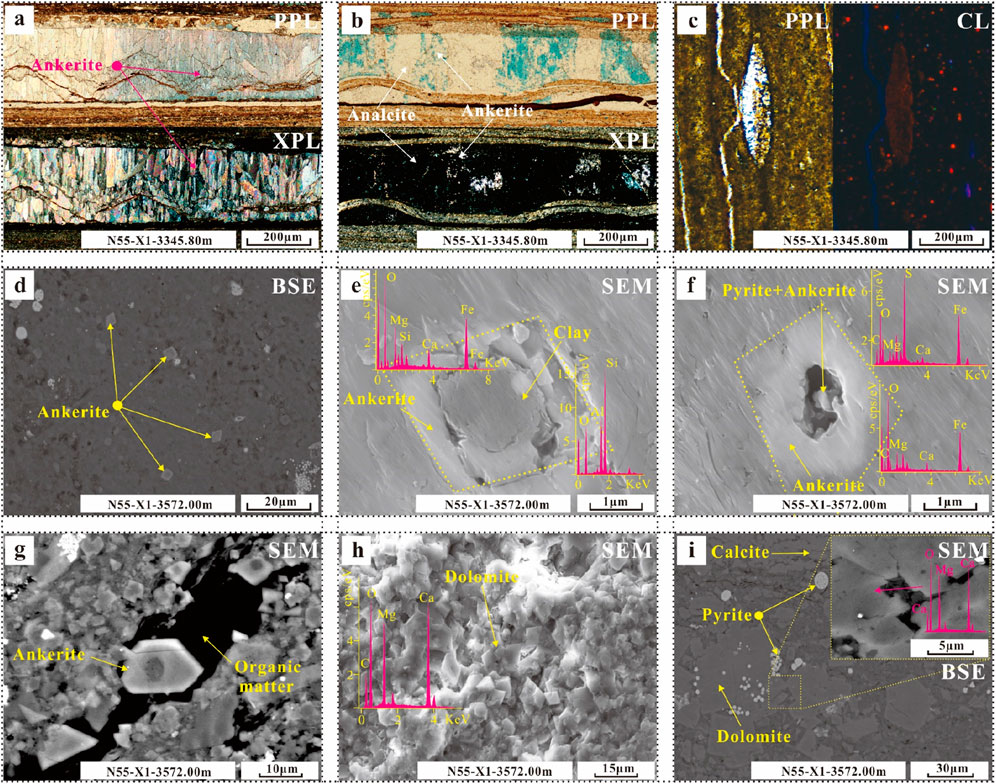
Figure 4. Photographs of the characteristics of dolomite–ankerite in different occurrences in various lithofacies. (A) Fibrous calcite metasomatized by ankerite. (B) The analogue was metasomatized by ankerite. (C) Characteristics of dolomite–ankerite under CL. (D–G) Distribution characteristics of ankerite with a foggy core/bright edge. (H, I) Dolomite distribution in the clay layers.
4.2.3 Quartz
There are two types of quartz: terrigenous quartz and authigenic quartz. Terrigenous clastic quartz is usually laminar and often contains water-escape structures formed by early undercompaction (Figures 5A, B). Some siliceous laminae contain organic matter (Figure 5C). Under CL, these laminae contain a certain amount of carbonate minerals (Figure 5D), the quartz includes blue and dark red high-temperature quartz, and there is no evidence of the luminescent diagenetic process of authigenic quartz (Figure 5E). This type of quartz is common in lithofacies L3 and L5.
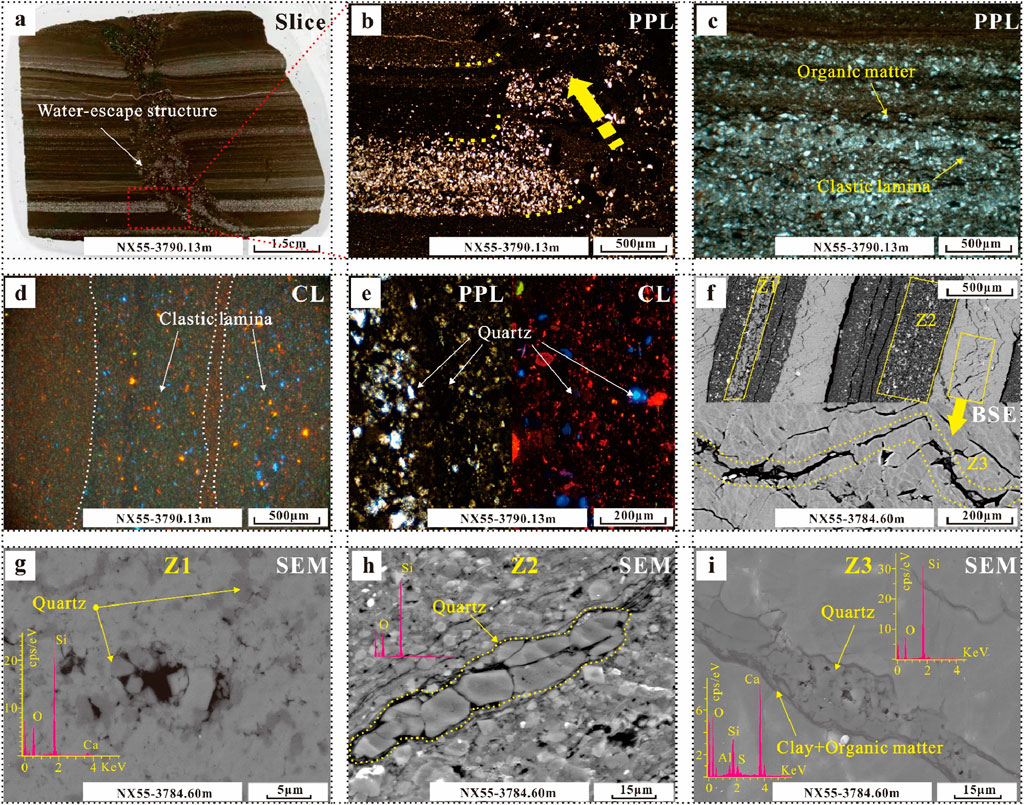
Figure 5. Photographs of the characteristics of different types of quartz in various lithofacies. (A, B) Quartz and a water-escape structure in a clastic lamina. (C) Distribution of quartz and organic matter in a lamina. (D, E) CL characteristics of quartz of different origins in the lamina. (F–I) Characteristics of quartz in different zones.
SEM observations revealed that there are autogenic quartz types with different characteristics in different zones of lithofacies L1 (Figure 5F): those with sparry calcite dissolution pores, clay minerals, and a black line in fibrous calcite. In Zone 1, authigenic quartz half-fills the dissolution pores of micritic calcite in the form of cement (Figure 5G). In Zone 2, it fills the shrinkage crack of clay minerals in the form of cement (Figure 5H); in Zone 3, the quartz exists in gaps along the growth edges of fibrous calcite (Figure 5I).
4.2.4 Feldspar
Feldspars include terrigenous feldspar and authigenic feldspar. The distribution of terrigenous feldspar is similar to that of terrigenous quartz, but the dissolution degree is greater (Figure 6A). Pores at the grain margins and within the grains are developed (Figures 6B, C). Small amounts of anorthite are also found in different lithofacies (Figures 6D, E). Terrigenous feldspar is common in lithofacies L3 and L5. Authigenic feldspar is mostly found in lithofacies L1 and L2, mainly albite and orthoclase, which are distributed in the dissolution gap between calcite and ankerite (Figures 6F–I).
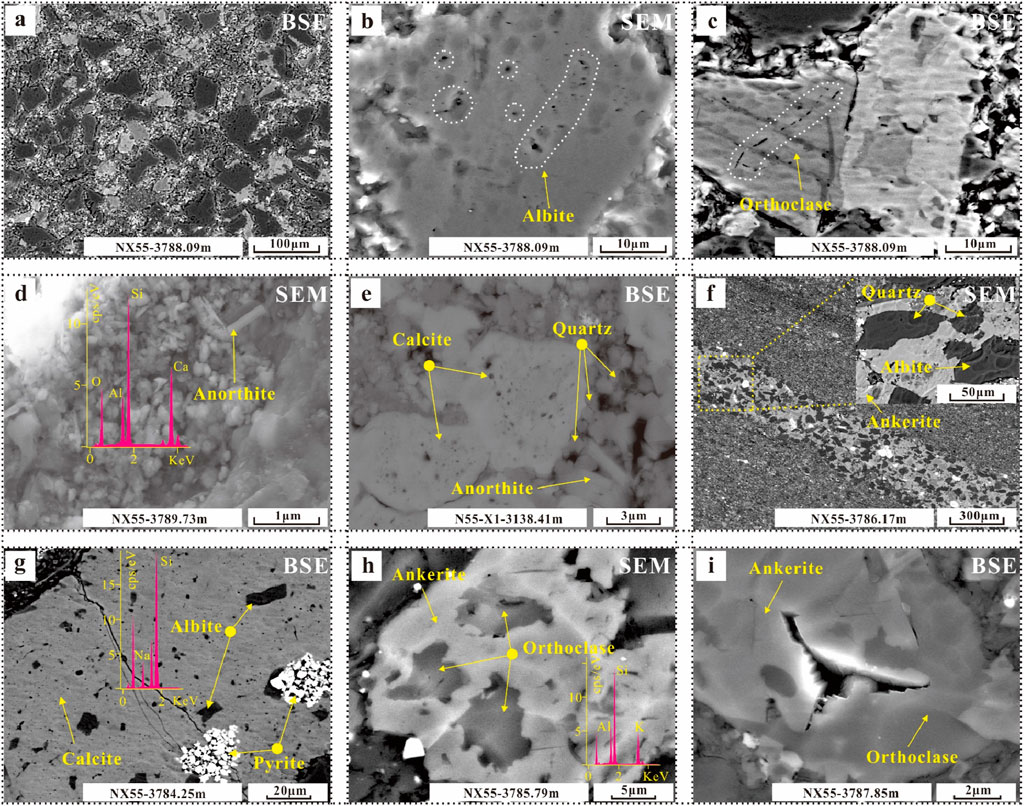
Figure 6. Photographs of the characteristics of feldspar in different occurrences in various lithofacies. (A) Distribution of terrigenous feldspar. (B) Dissolution on albite. (C) Dissolution on orthoclase. (D) Distribution of anorthite. (E) Mineral assemblage of calcite, quartz and anorthite. (F–I) Characteristics and distribution of authigenic feldspar.
4.2.5 Clay
Clay minerals constitute an important part of mudstone. In addition to the clay layers, clay minerals mostly exist in the form of cement in the intergranular pores of minerals (Figures 7A–D). In the clay mineral layer, the main forms of clay minerals are illite and illite/smectite layers (Table 1). In laminar lithofacies (e.g., L1, L2, L4, and L5), clay minerals are layered, and minerals such as organic matter and pyrite are distributed between the laminar clay minerals (Figures 7E, F). In L4, clay minerals form massive aggregates with other minerals (Figure 7G). Most clay shapes are pilar and fibrous (Figure 7H). There is also a small amount of acicular chlorite in these rocks (Figure 7I).
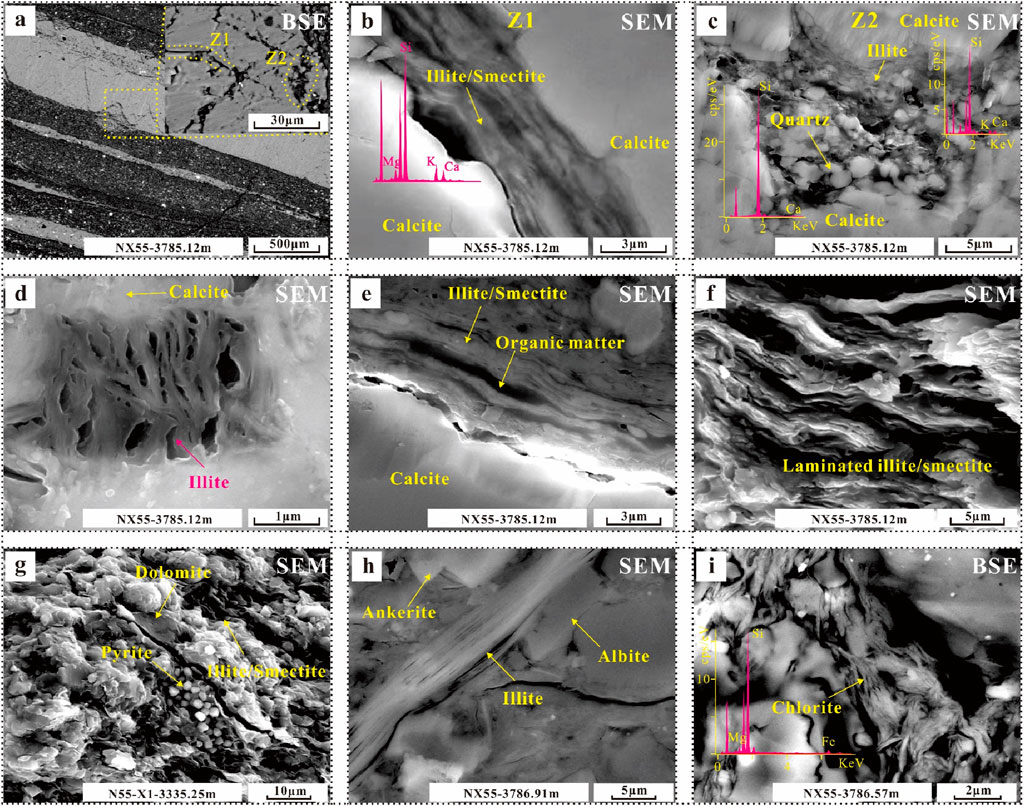
Figure 7. Photographs of the characteristics of clays in various lithofacies. (A) Characteristics of illite/smectite in different zones. (B) Clay minerals in a black line in fibrous calcite. (C) Clay minerals and authigenic quartz filling carbonate dissolution pores. (D) Illite filling carbonate dissolution pores. (E–H) Distribution, characteristics and associations of illite/smectite with other minerals. (I) Distribution and characteristics of chlorite.
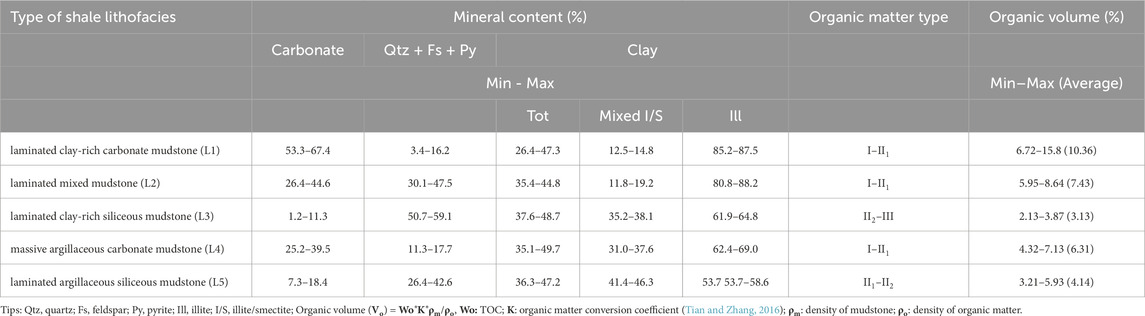
Table 1. Summary of organic geochemical parameters and mineral compositions of Es3x and Es4s from the Dongying Depression by XRD analysis.
4.2.6 Pyrite
Pyrite is widely distributed in this mudstone (Bilal et al., 2024), and there are two main types: strawberry pyrite in sparry calcite laminae (Figure 8A) and strawberry pyrite (Figures 8B, D), agglomerate pyrite (Figures 8B, C), or massive pyrite in clay minerals (Figure 8C). Intergranular pores are present in strawberry pyrite (Figure 8E), some of which contain organic matter (Figure 8F). The types of pyrite that form the cores of other minerals are described above.
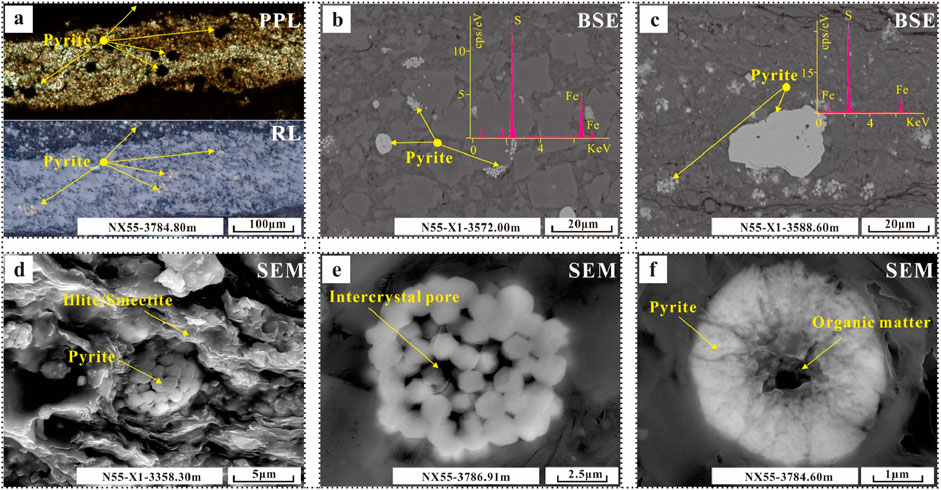
Figure 8. Photographs of the characteristics of pyrite in different occurrences in various lithofacies. (A) Distribution of pyrite in sparry calcite. (B) Distribution of strawberry and agglomerate pyrite. (D) Distribution of pyrite in clay minerals. (E) Pore characteristics of pyrite. (F) Organic matter in intergranular pores.
4.2.7 Organic matter
Organic matter is widely distributed in various lithofacies. In L1, the fluorescence reaction is very strong. From clay minerals to fibrous calcite, the fluorescence colour changes from brown to blue to green, which indicates that the organic matter has a high degree of evolution (Figure 9A). In L3, although the organic matter content is very low, the thin section fluoresces green, indicating considerable organic matter evolution (Figure 9B). In L4, the overall luminous intensity is weak, with brown fluorescence and a low degree of organic matter evolution (Figure 9C). In L5, the organic matter is brown–green and has undergone moderate evolution (Figure 9D).
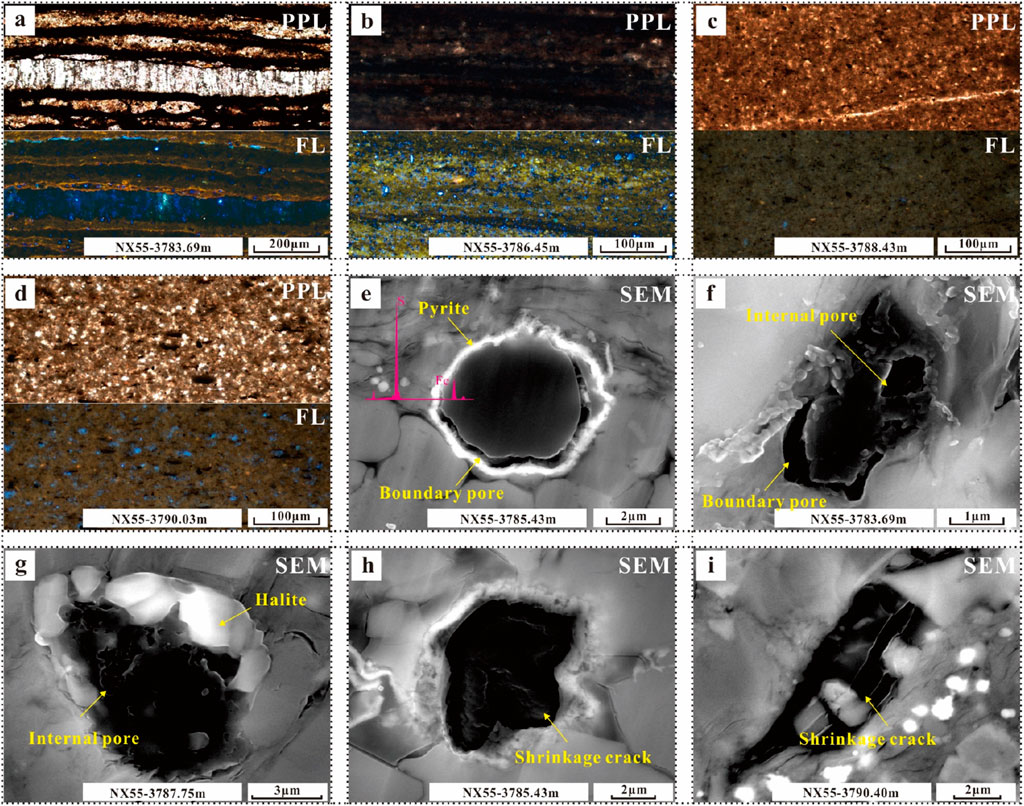
Figure 9. Photographs of the characteristics of organic matter in different occurrences in various lithofacies. (A) Distribution of organic matter in L1. (B) Distribution of organic matter in L3. (C) Distribution of organic matter in L4. (D) Distribution of organic matter in L5. (E) Pyrite is associated with the margin of organic matter. (F) Organic matter develops internal and boundary pores. (G) Organic development of irregular internal pores and margins associated with halite crystals. (H) Organic matter does not develop pores, but there are a few shrinkage cracks on its surface. (I) Shrinkage cracks throughout the organic matter.
SEM observations reveal that organic matter sometimes appears with pyrite and that the pyrite is evenly distributed around the organic matter (Figure 9E). Organic matter develops different pore types, which can be interpreted to determine the type of organic matter and its degree of evolution. Some highly evolved organic matter with well-developed internal and boundary pores is identified (Figure 9F). Some organic matter margins are associated with rock salt crystals (Figure 9G). Some organic matter has no pores but has developed small shrinkage cracks (Figure 9H) that grow through the organic matter as they develop (Figure 9I).
In this work, two methods were used to classify organic matter types: hydrogen index–oxygen index plots and hydrogen index-Tmax plots. During the pyrolysis of type I organic matter, a large amount of pyrolytic hydrocarbon S2 was released, whereas a small amount of type S3 and type III organic matter produced only a small amount of pyrolysis S2. The type of organic matter can be determined by placing the hydrogen and oxygen indices of mudstones on a map. The advantage of using the hydrogen index and Tmax chart to classify organic matter is that the influence of the maturity index Tmax on the hydrogen index is also considered. With increasing Tmax, the hydrogen index of organic matter gradually decreases along the trajectory curve.
The calculation formulae of the hydrogen and oxygen indices shown in Figure 10 are given in Table 2. The drop points on the plot reflect the different types of organic matter in the different lithofacies (Figures 10A, B). The corresponding relationship between the lithofacies and depth of each well suggests that there is a correspondence between the lithofacies and organic matter types: L1 corresponds to I-II1, L2 corresponds to I-II1, L3 corresponds to II2-III, L4 corresponds to I-II1, and L5 corresponds to II1-II2 (Table 1).
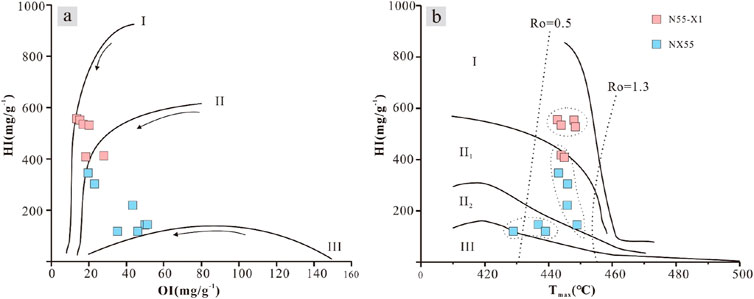
Figure 10. Determination of the organic matter type. (A) Hydrogen and oxygen indices of different mudstone samples. (B) Hydrogen indices and Tmax values of different mudstone samples (Wu and Gu, 1986).
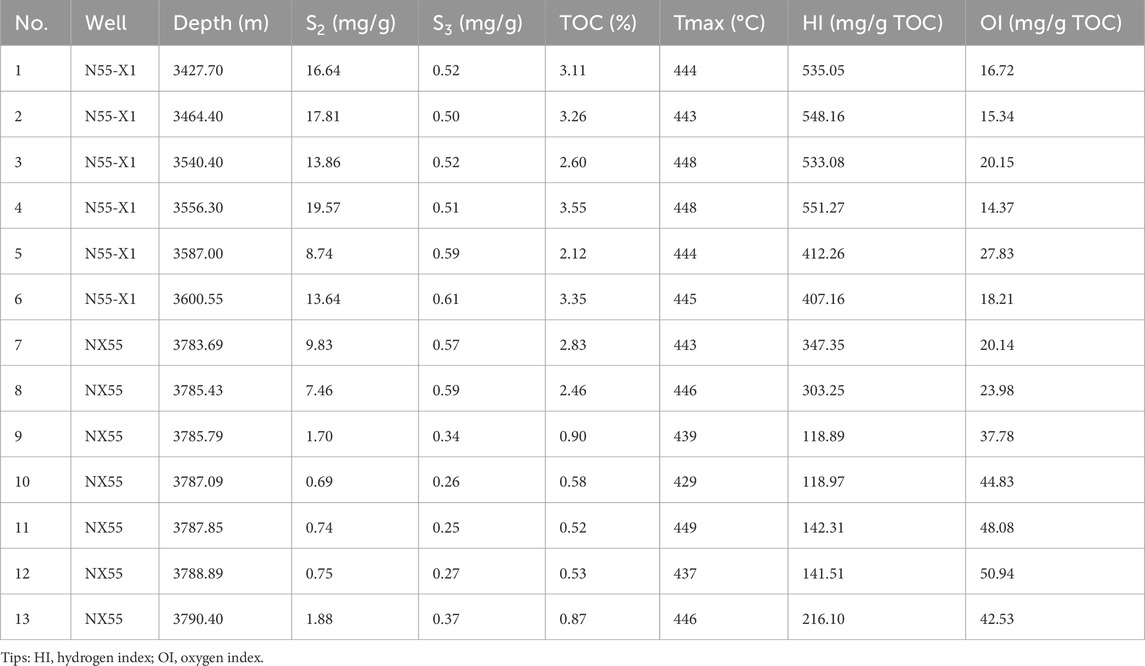
Table 2. Rock-Eval results, average TOCs, and hydrogen and oxygen indices of the samples from the N55–X1 and NX55 wells in the Dongying Depression.
5 Discussion
5.1 Sealing properties of diagenetic systems
Overpressure generally occurs in the Dongying Depression, and a large-scale overpressure system occurs in mainly the lower Es3 and upper Es4 members of the mudstone system (Liu and Xie, 2003; Zhang et al., 2010; Qiu, 2018). According to a large amount of drill stem test (DST) data, the depth of the abnormally high pressure in the Es3 and Es4 members is in the range of 2,200–4,400 m, the remaining pressure is in the range of 4–440 MP, and the pressure coefficient is in the range of 1.2–1.99 (He et al., 2012). Macroscopically, the pressure coefficient increases gradually from the edge to the interior of the depression. The occurrence of abnormal pressure is caused by the diagenesis and expulsion of hydrocarbons from organic matter.
In the absence of fracture development, the permeability of mudstone is generally less than 10–6 millidarcy (Jarvie, 2012), and the fluid in mudstone is difficult to transport. Eocene mudstone cores and thin beds in China are less fractured and have low permeability (Zhang et al., 2016). As a result, injecting external fluids into these mudstones is difficult.
The sealing property of mud shale can be used to analyse the openness of the diagenetic system. The Sr, Mn and Ca contents of each lithofacies in the NX55 well were calculated, and the Sr/Ca and Mn/Ca values were subsequently calculated (Figure 11). When the Sr/Ca value is high, the Mn/Ca value is low, and the diagenetic system tends to be closed. When the Sr/Ca value is low, the Mn/Ca value is high, and the diagenetic system tends to be open such that other fluids are involved in diagenesis (Tian and Zhang, 2016). Across the interval, the Sr/Ca values were high, and the Mn/Ca values were low. However, there are two sections with high Mn/Ca values in lithofacies L3 and L5. According to petrological evidence, the openness of the lithogenic system may be related to the following factors: terrigenous clastic grains are abundant, the porosity is high (L3), and early undercompaction leads to the development of drainage structures, which leads to the participation of other fluids in diagenesis (L5).
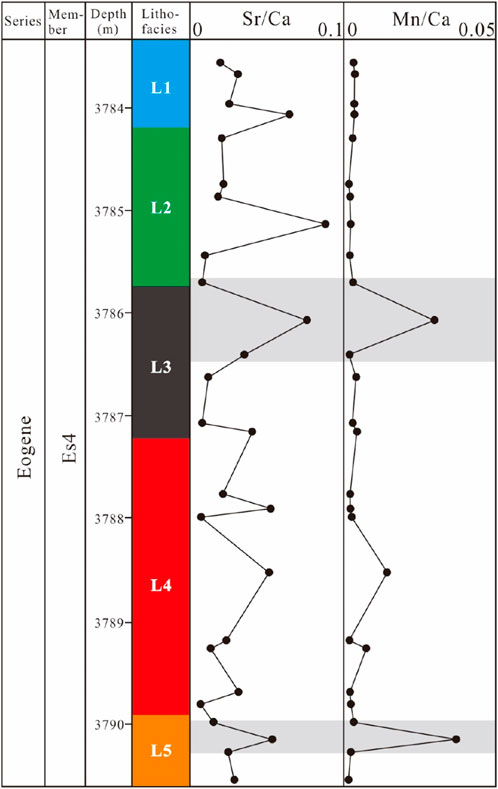
Figure 11. Lithofacies distribution and elemental geochemistry data of the NX55 well from 3,783.55 to 3,790.63 m.
Based on the formation overpressure system, lithologic characteristics and elemental geochemistry data of Es3x and Es4s, the mudstone in this section corresponds to a relatively closed diagenetic system as a whole.
5.2 CO2 and ion sources for authigenic carbonate
Stable carbon isotopes are highly stable in different deep-cycle carbon pools and can be used to indicate the carbon source of authigenic carbonate minerals during diagenesis (Siegel et al., 2004; Cao et al., 2018). The δ13C values range from 0.2 to 1.3 for sparry calcite, 3.2 to 6.4 for micritic calcite, 1.8 to 5.1 for fibrous calcite, 1.9 to 3.6 for ankerite, and 3.1 to 5.9 for micritic dolomite (Table 3). The δ13C values of these five different types of carbonate vary greatly, which makes it possible to determine the source of dissolved CO2 for authigenic carbonate minerals by analysing the distribution of δ13C (Warren, 2000; Kordi et al., 2017). In terms of the overall carbon isotope characteristics, the carbon isotope characteristics of the main authigenic carbonate are similar to those of the lacustrine facies micritic carbonate and the early carbonate cements in the mudstone, indicating that the carbonate ions needed for authigenic carbonate mineral precipitation are derived mainly from this source (Figure 12). The decrease in the carbon isotope value may be related to the decomposition of organic matter by sulfate-reducing bacteria (Irwin et al., 1977; Jiang et al., 2013), the thermal decarboxylation of organic matter (Jansa and Urrea, 1990; Sensuła et al., 2006), and the influence of diagenesis on the isotopic composition of authigenic carbonate minerals (Fritz and Smith, 1970).
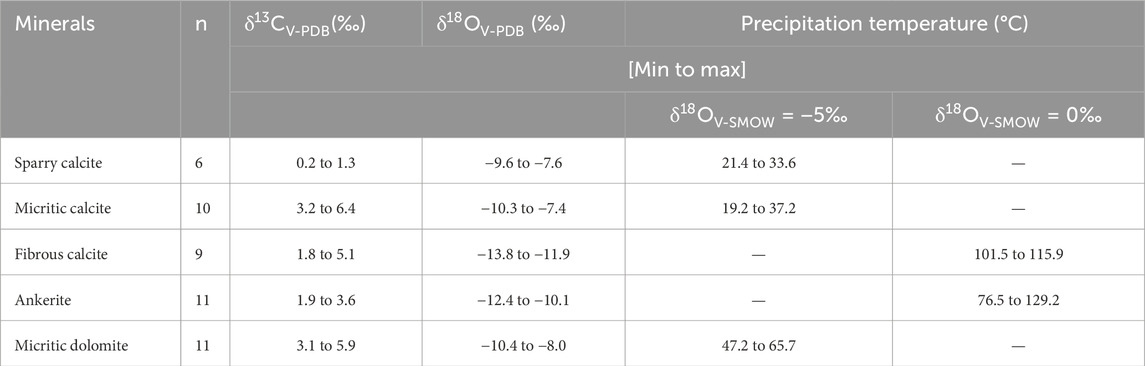
Table 3. Carbon and oxygen isotopic compositions of the sparry calcite, micritic calcite, fibrous calcite, ankerite and dolomite in the mudstone.
In the relatively closed environment of the diagenetic system, it is difficult for external fluid to enter the mudstone; therefore, the ions needed for the growth of authigenic carbonate minerals in the mudstone likely come from the mudstone itself. Petrological and geochemical findings indicate that fibrous carbonate rocks always exhibit laminar fractures, clay mineral shrinkage fractures and structural fractures in mudstones (Figure 3C). The δ18O values of fibrous carbonate rocks are always lower than those of micritic carbonate and sparry calcite (Table 3), indicating that they formed relatively late in the diagenetic process (Irwin et al., 1977; Sass et al., 1991). The above analysis reveals that the carbonate ions needed for authigenic carbonate mineral precipitation mainly originate from lacustrine carbonate sediments. Therefore, the Ca2+ and Mg2+ ions needed to form authigenic carbonate rocks should also have originated from these lacustrine carbonate deposits. Montmorillonite‒illite transformation also releases some Ca2+ and Mg2+ (Peltonen et al., 2009).
Due to the low porosity and permeability of mudstone, the Fe2+ supply in the pore water of this mudstone likely comes from montmorillonite‒illite transformation (Figure 7) (Bjørlykke et al., 1992; Xi et al., 2015; Haile et al., 2019) and the dissolution of unstable minerals in pyroclastics (Figures 6A–C) (Boles and Franks, 1979; Morse and Wang, 1997). Petrological evidence shows that both types of Fe2+ are derived from terrigenous pyroclasts and that the transformation of montmorillonite-illite typically occurs in mudstones. No cyclic band of carbonate luminescence was found under cathodoluminescence, which indicates that the source and content of Fe2+ in pore water are stable in a closed diagenetic environment (Ma et al., 2021). Therefore, montmorillonite‒illite transformation should be the main source of Fe2+ here.
5.3 Formation time and mechanism of authigenic carbonate
The fractionation coefficient of the oxygen isotopes is related to the temperature. The formation temperature of carbonate minerals can be calculated from the oxygen isotope values of the minerals and the oxygen isotope values of the palaeofluid. Similarly, the oxygen isotope values of palaeofluids can be obtained from the oxygen isotope values of carbonate minerals and precipitation temperatures (Zhang et al., 2021a). First, the oxygen isotope fractionation diagram of the calcite precipitation process and the oxygen isotope fractionation diagram of the dolomite precipitation process were drawn with the oxygen isotope fractionation formula presented by Friedman and O'Neil (1977), and the dolomite oxygen isotope fractionation formula presented by Land (1983), respectively. Thus, the precipitation temperatures of various carbonate minerals were calculated. The temperature and δ18OV-PDB values were used to calculate the formation fluid temperatures of each carbonate mineral (Table 3).
Micritic carbonate and sparry calcite formed earlier, and their genesis was controlled by sedimentary factors (Liu et al., 2014). The δ18OV-PDB values of micritic calcite range from −10.3‰ to −7.4‰, those of micritic dolomite range from −10.4 to −8.0, and those of sparry calcite range from −9.6‰ to −7.6‰ (Table 3). The δ18OV-SMOW values of formation fluids during the formation of micritic calcite range from −9‰ to −2.5‰, those of micritic dolomite range from −9‰ to −2.7‰, and those of sparry dolomite range from −8.1‰ to −3.9‰ during the sedimentary period (Figure 13). The oxygen isotope values of dolomite that formed during the same period are approximately 2%–3% higher than those of calcite, on average (Zhang et al., 2021b). The results show that the fluid environment of the dolomite was essentially the same as that of the calcite formed during the same period, indicating that the difference in the oxygen isotope values was caused mainly by the difference in oxygen isotope fractionation. The δ18OV-SMOW values of the early pore fluids in Es3x and Es4s range from −9‰ to −2.5‰, which reflects the characteristics of continental fresh to brackish water and is consistent with the sedimentary environment of the continental lake basin (Jiang et al., 2013). In addition to the carbonate of sedimentary origin, some carbonate cements precipitated from the pore fluid in the early diagenetic stage. The range of δ18O values for micritic calcite is wider than that for sparry calcite (Figure 13A), and the petrogenesis temperature should be higher.
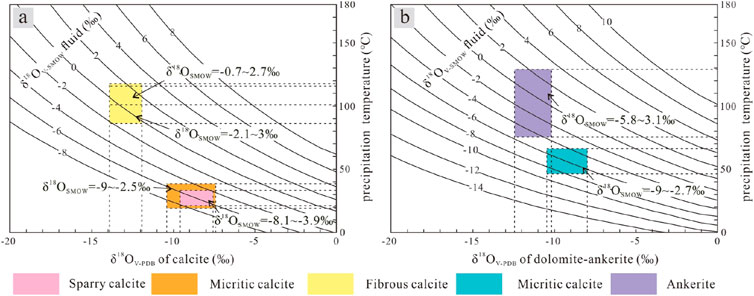
Figure 13. Oxygen isotope fractionation diagram of the carbonate mineral precipitation process in the Es3x and Es4s submembers in the Dongying Sag. (A) All kinds of calcite can be described with the following formula: 103lnα = 2.78 × 106 × T−2− 2.89 (Friedman and O'Neil, 1977). (B) All kinds of dolomite-ankerite can be described with the formula 103lnα = 3.2 × 106 × T−2 − 3.3 (Land, 1983).
The homogenization temperature of the fluid inclusions of the fibrous calcite in the upper submembers of Es3x and Es4s in the Dongying Depression is 86.4°C–117.4°C (Luan et al., 2019); this result is essentially the same as the precipitation temperature calculated by δ18O. Because the formation of fibrous calcite is related to hydrocarbon generation and expulsion, most of the inclusions that developed in the fibrous calcite are hydrocarbon inclusions, and the formation temperature of fibrous calcite should be slightly higher than this homogenization temperature. The δ18OSMOW values of formation fluids during the formation of fibrous calcite range from −2.1‰ to 3‰ (Figure 13A). Compared with those of fibrous calcite, the formation temperature and δ18OSMOW values of ankerite are greater, ranging from −5.8 to 3.1 (Figure 13B). The main occurrence of ankerite is laminated fibrous ankerite, cement or the “bright edge of the fog centre” (Figures 4A, B, D). From the petrological evidence, “fog heart bright side” ankerite or “fog heart centre” with clay minerals is given priority (Figures 4E, F), and its formation around the sedimentary clay minerals forms small clumps. The Fe ion needed for ankerite is provided by the transformation of the clay mineral; its formation time likely corresponds to the transformation of montmorillonite to illite or chlorite. Ankerite mainly exists in dissolution pores of carbonate minerals and silicoaluminate minerals (Figure 4B), and its formation is related to the discharge of organic acids caused by the maturation of organic matter. The laminated fibrous ankerite formation time should be equal to or after the laminated fibrous calcite formation time, as laminated fibrous ankerite does not require more diagenetic alteration (Figures 13A, B), which is not found in the mineral metasomatism mineral residue or mineral pseudomorphic changes under mineral cathodoluminescence, considering the different carbonate mineral generation types (Figures 4A, B). The formation of ankerite is likely due to the overpressure of fluid caused by the expulsion of hydrocarbons from organic matter (Zhang et al., 2016; Luan et al., 2019; Ma et al., 2020). The formation mechanism of ankerite is the same as that of fibrous calcite, and the difference in rock type is caused by the difference in the ionic environment.
5.4 Siliceous source and formation mechanism of authigenic silicates
The siliceous material in mudstone can be divided into terrigenous siliceous material and authigenic siliceous material formed during diagenesis, which can be distinguished by cathodoluminescence. Terrigenous quartz and feldspar usually have particle sizes larger than 5 μm and round, subround or angular shapes (Vos et al., 2014). Under cathodoluminescence, terrigenous quartz usually appears blue and dark red, terrigenous feldspar usually appears red, blue and green, and authigenic silica generally does not emit light. The cathodoluminescence observations of the samples reveal that there are small amounts of quartz and feldspar in all lithofacies, but most of them do not emit light, indicating that authigenic silicon is dominant in the silica content of the mudstone (Figures 3F, 5E). Zr and TiO2 in rocks are usually associated with heavy minerals and can be used as evidence to characterize detrital input into mudstone. Among the 5 different lithofacies, the contents of TiO2 in L3 and L5 are relatively high, and those of Zr in L3 and L4 are relatively high. The correlation coefficients are positive and negative but small (Figures 14C, D), indicating that terrigenous siliceous material accounts for a high proportion of these three lithofacies but is not the dominant source of siliceous material. In lithofacies L1 and L2, the contents of TiO2 and Zr are very low (Figures 14C, D), indicating that these two lithofacies are mainly autogenic siliceous lithofacies.
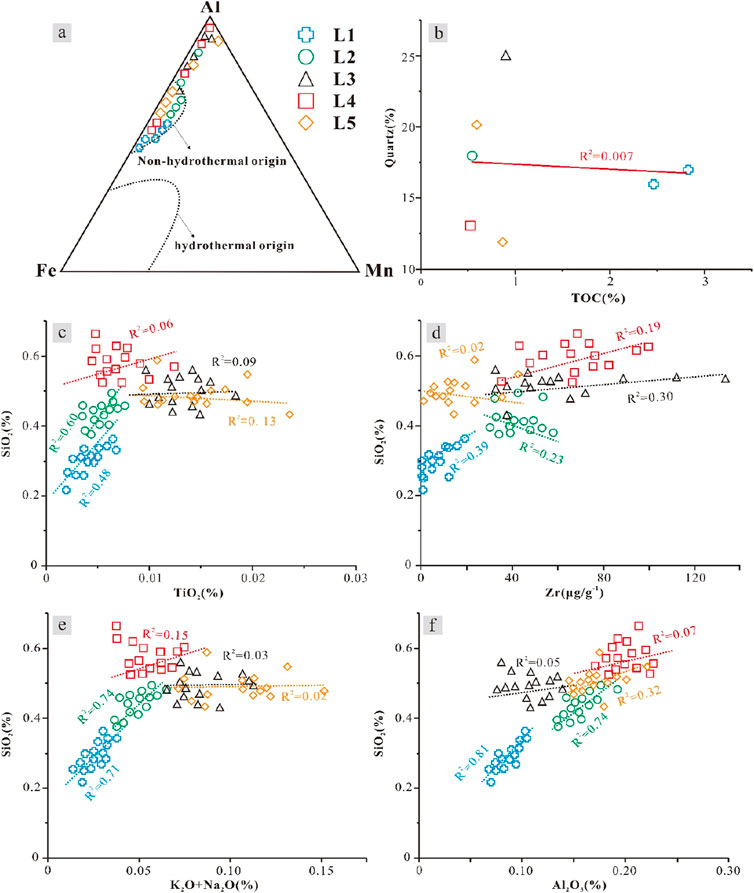
Figure 14. Explanation of the siliceous origins of various lithofacies. (A) Content diagram of Al, Fe, and Mn. (B) Relationship between the quartz content and TOC. Relationships between SiO2 and (C) TiO2, (D) Zr, (E) K2O + Na2O, and (F) Al2O3.
Authigenic siliceous material mainly comes from terrigenous siliceous dissolution and precipitation, biogenic siliceous transformation, clay mineral transformation, and hydrothermal siliceous input (Guo et al., 2021). Al‒Fe‒Mn plots are commonly used to characterize the formation environments of siliceous minerals (Adachi et al., 1985). None of the test samples of the five types of lithofacies were located in the range of hydrothermal origin (Figure 14A), suggesting that there is no hydrothermal siliceous source. No siliceous microfossils were found in any lithofacies, and the corresponding relationship between quartz content and TOC showed discrete data and low correlation (Figure 14B). Therefore, it was speculated that no silica was generated by the biological action or dissolution of the siliceous microfossils. In the diagrams of SiO2 and K2O + Na2O and SiO2 and Al2O3 (Figures 14E, F), L1 and L2 are highly positively correlated. Lithofacies L3, L4 and L5 have weak positive correlations, suggesting that the source of the authigenic siliceous material is related to the dissolution of feldspar and the transformation of clay minerals.
Based on the above evidence, three sources of siliceous materials are inferred: terrigenous direct input, reprecipitation of dissolved feldspar minerals, and siliceous release during the transformation of clay minerals. The cathodoluminescence and SEM images show that the three siliceous sources contribute to all the lithofacies. In L1 and L2, the siliceous materials formed by the transformation of clay minerals are dominant. In L3, terrigenous input siliceous materials are dominant. L4 and L5 are characterized by clay mineral transformation, terrigenous input and dissolution of feldspar minerals.
Clay mineral transformation is the main source of silica for authigenic quartz formation. Mudstone maturity is high in Es3x and Es4s in the Dongying Depression, and illite is the main component of clay. During the diagenetic process, the transformation of montmorillonite to illite results in silicon being released into the pore solution (Peltonen et al., 2009; Dowey and Taylor, 2020). Due to the low liquidity of the pore solution in the mudstone and the siliceous in situ precipitation crystallization of micron-scale silica particles, EDS reveals that this type of quartz contains a certain amount of Al (Figure 5H). This stage coincides with the beginning of the mass production of organic acids. Therefore, authigenic quartz precipitates in the dissolution pores of primary carbonate or early carbonate cements (Figures 3H, 5G). This phenomenon has occurred since the diagenetic evolution of mud shale entered the oil generation window, and the black line in the fibrous carbonate is actually the clay mineral boundary during the growth of such calcite (Figure 5F). The composition within the black line is mainly a variety of clay types with authigenic micron-scale quartz, indicating this diagenetic process (Figure 5G).
The dissolution of feldspar requires an acidic fluid environment derived from atmospheric precipitation under shallow burial conditions (<1,000 m) or from the thermal evolution of organic matter (carboxylic acid or CO2) in mudstone during burial-related diagenesis (2,000–4,000 m) (Bjørlykke et al., 1989; Surdam et al., 1989). The intense dissolution of feldspar minerals requires a diagenetic environment in which inert Al is complexed and transported (Wilkinson et al., 2004). Mudstone in the widespread presence of clay minerals and its conversion from montmorillonite to illite during the process of diagenetic transformation provides this kind of environment because the conversion of montmorillonite to illite consumes Al, and the release of organic acid is greater in mudstone and organic acid in complex anions that complex with Al (Zhang et al., 2021a) to increase the solubility of Al and dissolve feldspar (Figures 6B, C, H, I). Through simulation experiments, Surdam and Crossey (1985) suggested that carboxylic acid anions can increase the solubility of Al by 3 orders of magnitude compared with that of inorganic acid at 100°C. In addition to the formation of Si and H2O, many cations (Na, Ca, Mg and Fe) are released from the clay mineral transformation process. These cations must be consumed from the solution for montmorillonite‒illite transformation to continue, which should be achieved by authigenic quartz and authigenic carbonate rocks (Hower et al., 1976; Boles and Franks, 1979; Abercrombie et al., 1994; Bjørlykke, 1998). According to petrological evidence, authigenic feldspar is mainly albite, which is produced in dissolution pores of carbonate rocks (Figures 6F, G). The dissolution and precipitation of these different minerals also reflect the change in ion concentration in the pore solution.
5.5 Clay transformation process
Clay minerals are the key factors affecting the structure and diagenetic evolution of mudstone (Bozkaya and Yalçın, 2005; Lu et al., 2011). With increasing burial depth, temperature and pressure, clay minerals continuously remove interlayer water and ions, which is one of the main sources of pore fluid generation in mud shale. On the one hand, the transformation of montmorillonite to illite or chlorite increases the order of the clay minerals (Ma et al., 2020), and the volume shrinks to produce shrinkage cracks in mudstone, which provides space for the growth of authigenic carbonate and siliceous materials. On the other hand, this diagenesis provides a rich ionic environment and absorbs some of the supersaturated ions in the pore fluid, thus promoting the continuous generation or dissolution of various minerals in the mudstone-confined environment during diagenetic evolution. The highest content of clay minerals in the Es3x and Es4s submembers of the Dongying Depression is illite (Table 1). According to petrologic and energy spectrum evidence, potassium feldspar is strongly dissolved (Figures 6B, C, H, I), and the K+-rich environment needed for authigenic illite arises from the dissolution of potassium feldspar. Under certain temperature and pressure conditions, montmorillonite is converted into illite, and this process mainly begins at approximately 80°C (Peltonen et al., 2009).
Figure 15A shows that the clay mineral compositions of different lithofacies are different at the same burial depth and within the same area, indicating that the degree of smectite‒illite transformation is different in different lithofacies during the same diagenetic stage. The different characteristics of the five types of lithofacies mainly include their mineral compositions, sedimentary structures and organic matter contents (Table 1). According to the relationship between illite and I/S contents, L1 and L2 have the same clay mineral composition, whereas L3, L4 and L5 have the same clay mineral composition (Figure 15A). The illite content is positively correlated with the carbonate content (Figure 15b) and with the organic matter content (Figure 15C). Based on the above evidence, it can be inferred that smectite‒illite transformation is controlled by organic matter content and sedimentary structure characteristics. First, the expulsion of hydrocarbons from the thermal evolution of organic matter can cause overpressure of the pore fluid, which can promote the diagenetic evolution of clay minerals. Second, the development of lamellar fibrous carbonate driven by organic acids (Zhang et al., 2016) consumes many cations (Ca and Mg) generated during the diagenetic transformation of clay minerals, which leads to a positive shift in the chemical balance of the transformation of clay minerals and ensures that the reaction can continue. In lithofacies L4, although the organic matter content is very high, because of its massive tectonic sedimentary characteristics, it does not have bedding, such as lithofacies L1 and L2; similarly, overpressure cannot effectively occur after crack formation due to fluid pressure, and large authigenic carbonate grains are not produced. Thus, the ions in the pore fluid are unable to be consumed or are not greatly consumed, blocking smectite‒illite transformation. Although facies L3 and L5 have bedding fractures, their organic matter content is low, and it is difficult to produce large-scale organic matter generation and expulsion of hydrocarbons and organic acids. Unlike clastic reservoirs with good porosity and permeability, such as sandstones, pore fluids can migrate under multiple dynamics. The diagenetic system of this mudstone is relatively closed, and the supersaturated pore fluid formed during the diagenetic process must reach a state of undersaturation through “internal consumption”. In lithofacies L3, L4 and L5, although the degrees of transformation of smectite to illite are not as good as those of lithofacies L1 and L2, they also show positive correlations between the authentic illite content and the organic matter volume fraction and carbonate content (Figure 15C).
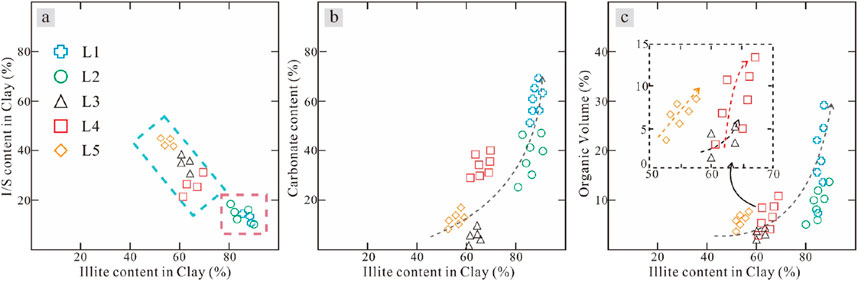
Figure 15. Relationships between illite content and (A) I/S content, (B) carbonate content, and (C) organic volume. Different lithofacies types are distinguished by different colours, which are the same as those in Figure 16.
5.6 Evolution of pyrite and organic matter
The strawberry pyrite (Figures 8A, D–F) observed was converted from mackinaite formed by BSR during early precipitation (Marin-Carbonne et al., 2014). The combination of massive pyrite and dolomite–ankerite (Figure 8C) may indicate thermochemical sulfate reduction (TSR) (Radke and Mathis, 1980; Machel et al., 1995). Pyrite formed during diagenesis is also one of the consumption pathways of Fe2+, which can also explain why Fe2+ does not enter the crystal lattice of dolomite during the rebonding process observed in petrology. The early precipitation of pyrite releases H+ (Jiang et al., 2018), which leads to a decrease in the pH of the early pore water and the dissolution of carbonate rocks in the early diagenetic stage. During the process of organic matter thermal evolution, transition metals and sulfides have a good catalytic effect on organic matter evolution (Mango, 1992; Wang et al., 2014). Fe2+ can affect the electron cloud distribution of pyrolysed kerogen, reduce the activation energy required for the reaction, increase the degradation rate, and promote hydrocarbon generation in organic matter.
With increasing vitrinite reflectance, organic matter matures and is discharged into hydrocarbons, an increasing number of organic pores are generated in the residual organic matter, and the size of the pores is constantly changing (Figure 9). The organic matter types in the five lithofacies are different (Tables 1, 2), which is caused by changes in the depositional environment. Like the organic pores in the Barnett shale (Loucks et al., 2009; Slatt and O'Brien, 2011), the vast majority of organic matter observed in the studied mudstone had more internal pores than marginal pores (Figures 9F, G, I), and small shrinkage fractures were found in organic matter without significant pore development (Figure 9H). However, some organic matter is surrounded by inorganic minerals, and edge pores are highly developed in the absence of internal pores (Figure 9E), indicating the catalytic effect of inorganic minerals on the evolution of organic matter. Clay minerals, carbonate minerals, quartz and pyrite in inorganic minerals (Zhang and Zhang, 1996; Liu et al., 2009), inorganic salts such as MgSO4 and NaHCO3 in pore fluids (Li et al., 2002), and trace elements such as nickel and zinc (Chen and Zha, 2007) have catalytic effects on kerogen pyrolysis and hydrocarbon generation, which is beneficial for the formation of pores. Hu et al. (2020), through experiments at the same temperature and pressure and physical and chemical conditions, reported that, based on the relationship between the quantity of hydrocarbon expulsion and organic matter types (type I > type II > type III), the combination of the organic matter contents of different lithofacies can control the thermal evolution of organic matter under pore fluid overpressure: L1 > L2 > L4 > L5 > L3.
5.7 Model illustrating lithofacies control on mineral coevolution
The diagenesis of various lithofacies has different evolution characteristics because of the different sedimentary structures, types and contents of organic matter and mineral types and can be roughly divided into four stages (Figure 16). The two models of the L1 lithofacies differ in terms of the carbonate rock type. Although they belong to the same lithofacies, they have different diagenetic evolution characteristics. The diagenetic evolution is as follows:
Stage 1: This stage is the beginning of diagenesis, and the influence of compaction is not obvious. All lithofacies maintain their respective sedimentary characteristics. L1 and L4, which have high organic matter contents, form mackinaite under BSR due to the formation of a closed reducing environment (Marin-Carbonne et al., 2014).
Stage 2: In areas where organic matter is generated during the biochemical gas-generating stage, a large amount of pore water is discharged from the mudstone due to compaction. However, due to the lithologic characteristics of mudstone, pore fluid overpresses when pore water is not discharged. As the pressure of the overlying strata continues to increase, water-escape structures may form (Figure 5A). At this stage, mackinaite converts into pyrite by absorbing Fe2+ ions in the pore water (Sweeney and Kaplan, 1973), and Ca2+ ions in the pore water can form early carbonate cements with organic matter evolution or HCO3− ions in the pore water (Figures 3H, I). This phenomenon results in a decrease in the pH of the pore fluid. Moreover, the Ca2+ and Mg2+ ions in these pore waters also participate in the recrystallization of the original carbonate minerals in the mudstone, among which dolomite has a high degree of self-recrystallization (Figure 4I).
Stage 3: Due to acidification of stage 2 pore fluids, feldspar minerals from terrigenous clasts in the mudstones dissolve, and K+ ions are released into the pore fluids. At this stage, due to the combined action of a K+-rich environment and temperature and pressure, smectite‒illite transformation takes place, releasing SiO2, H2O and metal cations (Na, Ca, Mg, and Fe) (Peltonen et al., 2009), and a large amount of SiO2 precipitates as microquartz in the cracks formed by smectite‒illite transformation (Figures 5G–I) (Towe, 1962). Some clay mineral masses release metal cations during diagenesis, causing carbonate rock to precipitate around these clay mineral masses, forming “the fog core bright side” ankerite (Figures 4D–F). Due to the organic acids formed by the thermal evolution of organic matter (Figure 16), the pH value of the pore fluid is further reduced, the Al complex produced by feldspar dissolution is consumed during smectite–illite transformation, and feldspar and analcime dissolution is more intense (Figures 4B, 6I). SiO2 from feldspar dissolution also contributes to the formation of microquartz (Figure 14E). Carbonate rocks that formed in the sedimentary and early diagenetic stages are dissolved by organic acids in the pore fluid. Recrystallization of the sparry calcite laminae occurs under the influence of overpressure. The transformation of clay mineral formation fluids that are rich in ions, fluid discharge from mineral recrystallization and organic matter thermal evolution form hydrocarbons and organic acids in the production of many “oil windows” within the shale formation with overpressure, and the fluid in the interior of the shale is prone to rupture via overpressure crack formation. These areas are usually laminated seams, exhibiting the transformation of clay minerals in terms of the formation of cracks, primary pores, and secondary pores produced during diagenesis. Due to the extremely high partial pressure of CO2, Ca, Mg and Fe ions form in the pore fluid due to early carbonate dissolution and clay mineral transformation, and fibrous carbonate forms in these overpressure fractures, which mainly occur in laminated seams (Figures 3A, 4A). Due to insufficient organic matter content, L3 and L5 cannot form large-scale fluid overpressure at this stage and thus do not develop large-scale fibrous carbonate. Although L4 has a high organic matter content, there are no laminated seams suitable for the development of fibrous carbonate. L3, L4 and L5 are unable to form fibrous carbonate, resulting in a small consumption of metal cations formed during the transformation of clay minerals and a slower conversion rate of smectite to illite than that observed for L1 and L2.
Stage 4: The production of organic acids decreases but continues at the peak of hydrocarbon expulsion due to the thermal evolution of organic matter, and the fibrous carbonate in L1 and L2 gradually develops into laminae. Recrystallization of the sparry calcite lamina continues. In the clay mineral layer, the transformation of smectite to illite results in an increase in the overall order of the clay minerals, resulting in many shrinkage fractures, in which carbonate and quartz precipitate (Figure 3C). Due to the decrease in organic acid production and increase in the pH value of the pore fluid, the authigenic albitite precipitates in the secondary pores dissolve during diagenesis (Figures 6F, G).
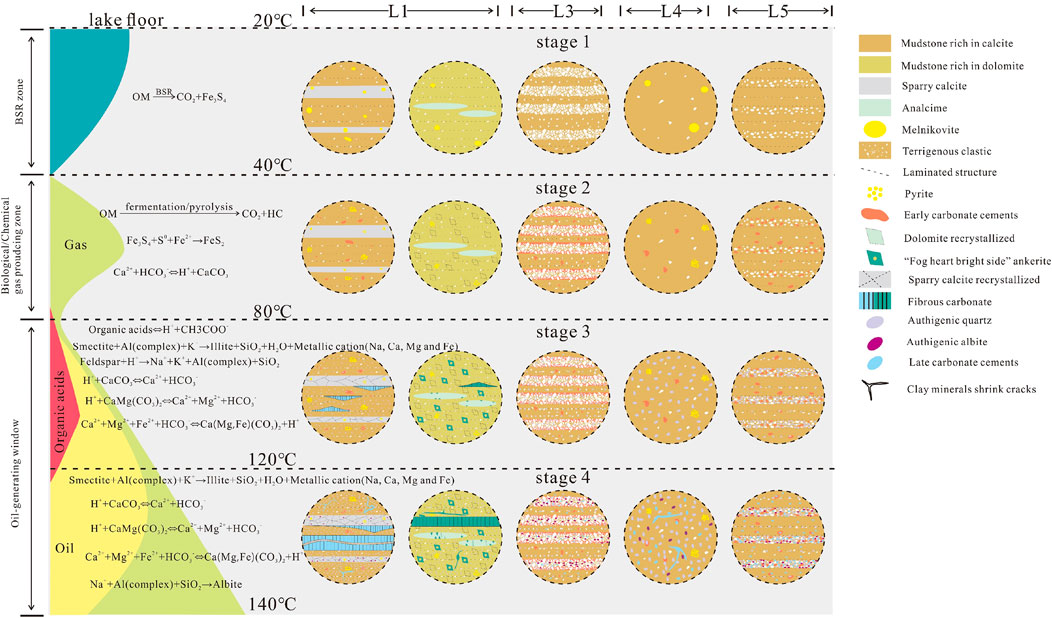
Figure 16. Model diagram of the organic matter evolution stage and various lithofacies evolution stages.
6 Conclusion
In a closed diagenetic environment, the diagenetic characteristics of different lithofacies are controlled by the sedimentary structure, mineral types, and organic matter content and evolution. The coevolution of minerals is realized mainly through the ion exchange of different minerals during diagenesis. The main medium of ion exchange is pore fluid, and the change in ion concentration is driven by the transformation of clay minerals and organic acids generated during the thermal evolution of organic matter. The CO2 needed for the formation of fibrous carbonate comes from carbonate of sedimentary origin in the mudstone and carbonate cement precipitated from pore fluid in the early diagenetic stage. The cations come from the dissolution of carbonate and the transformation of clay minerals in the early diagenetic stage, and fibrous carbonates form in laminar fractures formed during deposition. The transformation of smectite-illite requires a rich K+ environment provided by the dissolution of orthoclase and feldspar in Al complexes by the consumption of clay minerals and the transformation and emission of large amounts of SiO2 and metal cations. SiO2 forms a large amount of microquartz and metal cations that participate in the formation of authigenic carbonate, quartz, albite, illite, and chlorite. Compared with L1, L4 lacks the lamellar structure required for fibrous carbonate formation. Due to the low organic matter contents in L3 and L5, it is difficult to form overpressure fractures and pore fluid environments rich in organic acids during the thermal evolution of organic matter in these lithofacies, resulting in different types of diagenetic phenomena among the various lithofacies.
Data availability statement
The original contributions presented in the study are included in the article/supplementary material, further inquiries can be directed to the corresponding authors.
Author contributions
ZZ: Conceptualization, Data curation, Formal Analysis, Investigation, Methodology, Software, Validation, Writing–original draft, Writing–review and editing. ZJ: Conceptualization, Funding acquisition, Project administration, Resources, Supervision, Writing–review and editing. YY: Formal Analysis, Methodology, Supervision, Writing–review and editing.
Funding
The author(s) declare that no financial support was received for the research, authorship, and/or publication of this article.
Conflict of interest
The authors declare that the research was conducted in the absence of any commercial or financial relationships that could be construed as a potential conflict of interest.
Generative AI statement
The author(s) declare that no Generative AI was used in the creation of this manuscript.
Publisher’s note
All claims expressed in this article are solely those of the authors and do not necessarily represent those of their affiliated organizations, or those of the publisher, the editors and the reviewers. Any product that may be evaluated in this article, or claim that may be made by its manufacturer, is not guaranteed or endorsed by the publisher.
References
Abercrombie, H. J., Hutcheon, I. E., Bloch, J. D., and de Caritat, P. (1994). Silica activity and the smectite–illite reaction. J. Geol. 22, 539–542. doi:10.1130/0091-7613(1994)022<0539:saatsi>2.3.co;2
Adachi, M., Yamamoto, K., and Sugisaki, R. (1985). Hydrothermal chert and associated siliceous rocks from the northern Pacific their geological significance as indication od ocean ridge activity. J. Sediment. Geol. 47, 125–148. doi:10.1016/0037-0738(86)90075-8
Barth, T., and Bjørlykke, K. (1993). Organic acids from source rock maturation: generation potentials, transport mechanisms and relevance for mineral diagenesis. Appl. Geochem. 8, 325–337. doi:10.1016/0883-2927(93)90002-X
Bilal, A., Yang, R., Fan, A., Mughal, M. S., Li, Y., Basharat, M., et al. (2022a). Petrofacies and diagenesis of thanetian lockhart limestone in the upper indus basin (Pakistan): implications for the ceno-tethys ocean. J. Carbonates Evaporites 37, 78. doi:10.1007/s13146-022-00823-z
Bilal, A., Yang, R., Li, Y., Zhang, J., and Janjuhah, H. T. (2024). Microfacies shift in the late paleocene-early eocene patala formation in the upper indus basin(Pakistan): implications for development of the ceno-tethys ocean. Mar. Petroleum Geol. 161, 106693. doi:10.1016/j.marpetgeo.2024.106693
Bilal, A., Yang, R., Mughal, M. S., Janjuhah, H. T., Zaheer, M., and Kontakiotis, G. (2022b). Sedimentology and diagenesis of the early–middle eocene carbonate deposits of the ceno-tethys ocean. J. Mar. Sci. Eng. 10 (11), 1794. doi:10.3390/jmse10111794
Bjørlykke, K. (1998). “Clay mineral diagenesis in sedimentary basins: a key to the prediction of rock properties: examples from the North Sea Basin,” in Clay minerals in the modern society. Mineralogical society (London), 15–34. United King-dom. doi:10.1180/claymin.1998.033.1.03
Bjørlykke, K., Nedkvitne, T., Ramm, M., and Saigal, G. C. (1992). Diagenetic processes in the brent group (middle jurassic) reservoirs of the north sea: an overview. J. Geol. Soc. 61, 263–287. doi:10.1144/GSL.SP.1992.061.01.15
Bjørlykke, K., Ramm, M., and Saigal, G. C. (1989). Sandstone diagenesis and porosity modification during basin evolution. J.Geologische Rundsch. 78, 243–268. doi:10.1007/bf01988363
Boles, J. R., and Franks, S. G. (1979). “Clay diagenesis in Wilcox sandstones of Southwest Texas; implications of smectite diagenesis on sandstone cementation,”J. J. Sediment. Res., 49. 55–70. doi:10.1130/0091-7613(1979)7<602:SERATM>2.0
Bozkaya, Ö., and Yalçın, H. (2005). Diagenesis and very low-grade metamorphism of the Antalya unit: mineralogical evidence of Triassic rifting, Alanya-Gazipas, A central Taurus belt, Turkey. J. J. Asian Earth Sci. 25, 109–119. doi:10.1016/j.jseaes.2004.02.001
Cao, Q., Zhou, W., Chen, W. L., Deng, H. C., Yan, C. H., and Deng, K. (2015). Analysis of pore types,sizes and genesis in continental shale gas reservoir of Chang 7 of Yanchagn Formation,Odros Basin. J. J. Mineralogy Petrology. 35, 90–97. doi:10.19719/j.cnki.1001-6872.2015.02.011
Cao, Z., Lin, C., Dong, C., Ren, L., Han, S., Dai, J., et al. (2018). Impact of sequence stratigraphy, depositional facies, diagenesis and CO2 charge on reservoir quality of the lower cretaceous Quantou Formation. Southern Songliao Basin, China. Mar. Petroleum Geol. J. 93, 497–519. doi:10.1016/j.marpetgeo.2018.03.015
Chen, D. X., Pang, X. Q., Zhang, J., and Li, M. (2007). Application of quantitative grain fluorescence techniques to study subtle oil migration pathway of lithological reservoir. J. Front. Earth Sci. 1, 498–504. doi:10.1007/s11707-007-0061-y
Chen, J., and Xiao, X. M. (2014). Evolution of nanoporosity in organic-rich shales during thermal maturation. J. Fuel 129, 173–181. doi:10.1016/j.fuel.2014.03.058
Chen, Z. H., and Zha, M. (2007). Correlation between inorganic elements and abnormal vitrinite reflectance in lacustrine source rocks. J. Geochim. 36, 275–278. doi:10.1631/jzus.2007.A1858
Cobbold, P. R., Zanella, A., Rodrigues, N., and Løseth, H. (2013). Bedding-parallelfibrous veins (beef and cone-in-cone): worldwide occurrence and possible significance in terms of fluid overpressure, hydrocarbon generation and mineralization. J. Mar. Petroleum Geol. 43, 1–20. doi:10.1016/j.marpetgeo.2013.01.010
Diaz, H. G., Fuentes, C. C., Calvin, C., Yang, Y., MacPhail, K., and Lewis, R. (2013). Evaluating the impact of mineralogy on reservoir quality and completion quality of organic shale plays. Salt Lake City, Utah: AAPG Rocky Mountain Section Meeting, 22–24. Available at: https://www.mendeley.com/catalogue/8a34396f-2142-3633-8c82-4e52fc74c936/.
Dowey, P. J., and Taylor, K. G. (2020). Diagenetic mineral development within the upper jurassic haynesville-bossier shale, USA. J. Sedimentol. 67, 47–77. doi:10.1111/sed.12624
Durney, D. W., and Ramsay, J. G. (1973). “Incremental strains measured by syntectonic crystal growths,” in Gravity and tectonics. Editors K. A. De Jong, and K. Scholten (New York: Wiley), 67–96.
Friedman, I., and O'Neil, J. R. (1977). Data of geochemistry: compilation of stable isotope fractionation factors of geochemical interest, 440. Washington, DC: US Government Printing Office. Available at: https://refhub.elsevier.com/S0264-8172(19)30010-8/sref24.
Fritz, P., and Smith, D. G. W. (1970). The isotopic composition of secondary dolomites. J. Geochimica Cosmochimica Acta 34, 1161–1173. doi:10.1016/0016-7037(70)90056-6
Guo, W., Dong, D. Z., Li, M., Sun, S. S., Quan, G. Z., and Zhang, S. R. (2021). Quartz genesis in organic-rich shale and its indicative significance to reservoir quality: a case study on the first sub-member of the first Member of Lower Silurian Longmaxi Formation in the southeastern Sichuan Basin and its periphery. J. Nat. Gas. Ind. 41, 65–74. doi:10.3787/j.issn.1000-0976.2021.02.008
Guo, X. W., He, S., Liu, K. Y., Song, G. Q., Wang, X. J., and Shi, Z. H. (2010). Oil generation as the dominant overpressure mechanism in the cenozoic dongying depression, Bohai Bay Basin, China. J. AAPG Bull. 94, 1859–1881. doi:10.1306/05191009179
Haile, B., Czarniecka, U., Xi, K., Smyrak-Solpra, A., Jahren, J., Braathen, A., et al. (2019). Hydrothermally induced diagenesis: evidence from shallow marine-deltaic sediments, Wilhelmøya, Svalbard. J. Geosci. Front. 10, 629–649. doi:10.1016/j.gsf.2018.02.015
He, S., Song, G. Q., Wang, Y. S., Hao, X. F., Wang, B. J., Li, N., et al. (2012). Distribution and major control factors of the present-day large-scale overpressured system in Dongying depression. J. Earth Science—Journal China Univ. Geosciences 37, 1029–1042. doi:10.3799/dqkx.2012.110
Hower, J., Eslinger, E. V., Hower, M. E., and Perry, E. A. (1976). Mechanism of burial meta-morphism of argillaceous sediment: 1. Mineralogical and chemical evidence. J. Geol. Soc. Am. Bull. 87, 725–737. doi:10.1130/0016-7606(1976)87<725:mobmoa>2.0.co;2
Hu, J. J., Tang, Y. J., He, D. X., Bo, N., and Li, M. J. (2020). Comparison and exploration of hydrocarbon expulsion patterns of different types of source rocks. J. J. Geomechanics 26, 941–951. doi:10.12090/j.issn.1006-6616.2020.26.06.075
Hu, W. X., Yao, S. P., Lu, X. C., Wu, H. G., Sun, F. N., and Qin, X. (2019). Effects of organic matter evolution on oil reservoir property during diagenesis of typical continental shale sequences. J. Oil and Gas Geol. 40, 947–956. doi:10.11743/ogg20190501
Huang, Z. K., Chen, J. P., Wang, Y. J., Xue, H. T., Deng, C. P., and Wang, M. (2013). Characteristics of micropores in mudstones of the cretaceous Qingshankou Formation,Songliao Basin. J. Acta Pet. Sin. 34, 30–36. doi:10.7623/syxb201301004
Irwin, H., Curtis, C., and Coleman, M. (1977). Isotopic evidence for source of diagenetic carbonates formed during burial of organic-rich sediments. J. Nat. 269, 209–213. doi:10.1038/269209a0
Jansa, L. F., and Urrea, V. H. N. (1990). Geology and diagenetic history of overpressured sand-stone reservoirs, venture gas field, offshore Nova scotia. Canada (1). J. AAPG Bull. 74, 1640–1658. doi:10.1306/0C9B2551-1710-11D7-8645000102C1865D
Jarvie, D. M. (2012). “Shale resource systems for oil and gas: part 1-shale-gas resource systems,”. Shale reservoirs-giant resources for the 21st century. Editor J. A. Breyer (Washington, DC: AAPG Memoir), 97, 69–88. doi:10.1306/13321446M973489
Jiang, L., Worden, R. H., and Yang, C. B. (2018). Thermochemical sulphate reduction can improve carbonate petroleum reservoir quality. J. Geochim. Cosmochim. Acta. 223, 127–140. doi:10.1016/j.gca.2017.11.032
Jiang, Z. X., Liang, C., Wu, J., Zhang, J. G., Zhang, W. Z., Wang, Y. S., et al. (2013). Several issues in sedimentological studies on hydrocarbon-bearing fine-grained sedimentary rocks. J. Acta Pet. Sin. 34, 1031–1039. doi:10.7623/syxb201306001
Kordi, M., Morad, S., Turner, B., and Salem, A. M. (2017). Sequence stratigraphic controls on formation of dolomite: insights from the carboniferous um bogma formation, SinaiEgypt. J. Pet. Sci. Eng. 149, 531–539. doi:10.1016/j.petrol.2016.10.067
Land, L. S. (1983). The application of stable isotopes to studies of the origin of dolomite and to problems of diagenesis of clastic sediments. J. SEPM Short. Course (SC10) 4, 1–22. doi:10.2110/scn.83.01.0000
Li, J. Y. (2015). Pore characteristics and their evolution in paleogene mud shales, dongying sag, Bohai Bay Basin. J. Petroleum Geol. Exp. 37, 566–574. doi:10.11781/sysydz201505566
Li, S. Y., Lin, S. J., Guo, S. H., and Liu, L. F. (2002). Effects of inorganic salts on the hydrocarbon generation from kerogens. J. Geochim. 31, 15–20. doi:10.1080/12265080208422884
Liu, H. P., Zhang, Z. L., Ji, Z. K., and Li, B. (2009). Effects of inorganic is salts on the hydrocarbon generation of mixed ester catalyzed by natural minerals at low temperature. J. Acta Sedimentol. Sin. 26, 886–890. doi:10.14027/j.cnki.cjxb.2008.05.010
Liu, S. B., Huang, S. J., Shen, Z. M., Lv, Z. X., and Song, R. C. (2014). Diagenetic fluid evolution and water-rock interaction model of carbonate cements in sandstone: an example from the reservoir sandstone of the Fourth Member of the Xujiahe Formation of the Xiaoquan-Fenggu area, Sichuan Province, China. J. Sci. China Earth Sci. 57, 1077–1092. doi:10.1007/s11430-014-4851-2
Liu, X. F., and Xie, X. N. (2003). Study on fluid pressure system in Dongying depression. J. Earth Sci. J. China Univ. Geosciences 28, 78–86.
Loucks, R. G., Reed, R. M., Ruppel, S. C., and Jarvie, D. M. (2009). Morphology, genesis, and distribution of nanometer-scale pores in siliceous mudstones of the Mississippian Barnett Shale. J. J. Sediment. Res. 79, 848–861. doi:10.2110/jsr.2009.092
Lu, J., Milliken, K. L., Reed, R. M., and Hovorka, S. (2011). Diagenesis and sealing capacity of the middle Tuscaloosa mudstone at the Cranfield carbon dioxide injection site, Mississippi, USA. J. Environ. Geosci. 18, 35–53. doi:10.1306/eg.09091010015
Luan, G. Q., Dong, C. M., Karem, A., Lin, C. Y., Ma, C. F., Ren, L. H., et al. (2019). Origin of bedding-parallelfibrous calcite veins in lacustrine black shale: a case study from Dongying Depression, Bohai Bay Basin. J. Mar. Petroleum Geol. 102, 873–885. doi:10.1016/j.marpetgeo.2019.01.010
Lundegard, P. D., Land, L. S., and Galloway, W. E. (1984). Problem of secondary porosity: frio formation (oligocene), Texas gulf coast. Tex. Gulf Coast J. Geol. 12, 399. doi:10.1130/0091-7613(1984)12<399:pospff>2.0.co;2
Ma, C. F., Dong, C. M., Derek, E., Wang, Q., Huang, Z., Liu, H. L., et al. (2020). Insights from electron backscatter diffraction into the origin offibrous calcite veins in organic-rich shale from lower Es3 to upper Es4, Jiyang Depression, China. Mar. Petroleum Geol. 113, 104131. doi:10.1016/j.marpetgeo.2019.104131
Ma, P. J., Lin, C. Y., Jens, J., Dong, C. M., Ren, L. H., and Helge, H. (2021). Cyclic zoning in authigenic saddle dolomite-ankerite: indications of a complex interplay between fault-rupturing and diagenetic alteration. J. Chem. Geol. 599 (2021), 119831. doi:10.1016/j.chemgeo.2020.119831
Machel, H. G., Krouse, H. R., and Sassen, R. (1995). Products and distinguishing criteria of bacterial and thermochemical sulfate reduction. J. Geol. Soc. Spec. Publ. 10, 373–389. doi:10.1016/0883-2927(95)00008-8
Mango, F. D. (1992). Transition metal catalysis in the generation of petroleum and natural gas. J. Geochimica Cosmochimica Acta 56, 553–555. doi:10.1016/0016-7037(92)90153-A
Marin-Carbonne, J., Rollion-Bard, C., Bekker, A., Rouxel, O., Agangi, A., Cavalazzi, B., et al. (2014). Coupled Fe and S isotope variations in pyrite nodules from Archean shale. J. Sci. Lett. 392, 67–79. doi:10.1016/j.epsl.2014.02.009
Marshall, J. D. (1982). Isotopic composition of displacive fibrous calcite veins: reversals in pore-water composition trends during burial diagenesis. J. Sediment. Res. 52, 615–630. doi:10.1306/212F7FB3-2B24-11D7-8648000102C1865D
Milliken, K. L., Rudnicki, M., Awwiller, D. N., and Zhang, T. (2013). Organic matter–hosted pore system, marcellus formation (devonian), Pennsylvania. J. Aapg Bull. 97, 177–200. doi:10.1306/07231212048
Montgomery, S. L., Jarvie, D. M., Bowker, K. A., and Pollastro, R. M. (2005). Mississippian Barnett shale, Fort Worth Basin, north-central Texas: gas-shale play with multi-trillion cubic foot potential. J. AAPG Bull. 89, 155–175. doi:10.1306/09170404042
Morse, J. W., and Wang, Q. (1997). Pyrite formation under conditions approximating those in anoxic sediments: II. Influence of precursor iron minerals and organic matter. J. Mar. Chem. 57, 187–193. doi:10.1016/S0304-4203(97)00050-9
Nie, H. K., Ma, X., Yu, C., Ye, X., Bian, R. K., and Liu, Z. B. (2017). Shale gas reservoir characteristics and its exploration potential-Analysis on the Lower Jurassic shale in the eastern Sichuan Basin. J. Oil Gas Geol. 38, 438–447. doi:10.11743/ogg20170303
Peltonen, C., Marcussen, Ø., Bjørlykke, K., and Jahren, J. (2009). Clay mineral diagenesis and quartz cementation in mudstones: the effects of smectite to illite reaction on rock properties. Mar. Petroleum Geol. 26, 887–898. doi:10.1016/j.marpetgeo.2008.01.021
Qiu, Y. B. (2018). Overpressure structure in Dongying sag and its controlling factors. J. China Pet. Explor. 23, 28–34. doi:10.3969/j.issn.1672-7703.2018.03.004
Radke, B. M., and Mathis, R. L. (1980). On the formation and occurrence of saddle dolomite. J. Sediment. Res. 50, 1149–1168. doi:10.1306/212F7B9E-2B24-11D7-8648000102C1865D
Ross, D. J. K., and Bustin, R. M. (2009). Investigating the use of sedimentary geochemical proxies for paleoenvironment interpretation of thermally mature organic-rich strata: examples from the Devonian–Mississippian shales, Western Canadian Sedimentary Basin. J. Chem. Geol. 260, 1–19. doi:10.1016/j.chemgeo.2008.10.027
Sass, E., Bein, A., and Almogi-Labin, A. (1991). Oxygen-isotope composition of diagenetic calcite in organic-rich rocks: evidence for18O depletion in marine anaerobic pore water. J. Geol. 19, 839–842. doi:10.1130/0091-7613(1991)019<0839:oicodc>2.3.co;2
Seewald, J. S. (2003). Organic-inorganic interactions in petroleum-producing sedimentary basins. J. Nat. 426, 327–333. doi:10.1038/nature02132
Sensuła, B., Bottger, T., Pazdur, A., Piotrowska, N., and Wagner, R. (2006). Carbon and oxygen isotope composition of organic matter and carbonates in recent lacustrine sediments. J. Geochronometria. 25, 77–94. doi:10.1016/j.geobios.2004.10.004
Siegel, D. I., Lesniak, K. A., Stute, M., and Frape, S. (2004). Isotopic geochemistry of the Saratoga springs: implications for the origin of solutes and source of carbon dioxide. J. Geol. 32, 257–260. doi:10.1130/G20094.1
Slatt, R. M., and O'Brien, N. R. (2011). Pore types in the Barnett and Woodford gas shales: contribution to understanding gas storage and migration pathways in fine-grained rocks. J. AAPG Bull. 95, 2017–2030. doi:10.1306/03301110145
Song, M., Liu, H., Wang, Y., and Liu, Y. (2020). Enrichment rules and exploration practices of paleogene shale oil in jiyang depression, Bohai basin, China. Petroleum Explor. Dev. 47, 225–235. doi:10.1016/S1876-3804(20)60043-X
Sun, H. Q. (2017). Exploration practice and cognitions of shale oil in Jiyang Depression. J. China Pet. Explor. 22, 1–14. doi:10.3969/j.issn.1672-7703.2017.04.001
Surdam, R. C., and Crossey, L. J. (1985). Organic-inorganic reactions during progressive burial: key to porosity and permeability enhancement and preservation. J. Philosophical Trans. R. Soc., 315, 135–156. doi:10.1016/0198-0254(86)91076-9
Surdam, R. C., Crossey, L. J., Hagen, E. S., and Heasler, H. P. (1989). Organic-inorganic interactions and sandstone diagenesis. J. Am. Assoc. Petroleum Geol. Bull. 73. doi:10.1306/703c9ad7-1707-11d7-8645000102c1865d
Sweeney, R. E., and Kaplan, I. R. (1973). Pyrite framboid formation; laboratory synthesis and marine sediments. J. Econ. Geol. 68, 618–634. doi:10.2113/gsecongeo.68.5.618
Tian, J. C., and Zhang, X. (2016). Sedimentary geochemistry (in chinses). Beijing: Geological Publishing House.
Towe, K. M. (1962). Clay mineral diagenesis as a possible source of silica cement in sedimentary rocks. J. J. Sediment. Petrology 32, 26–28. doi:10.1306/74d70c3b-2b21-11d7-8648000102c1865d
U.S. Energy Information Administration (2020). International energy outlook. (Accessed 25 November 2020). Available at: https://www.eia.gov/outlooks/ieo/.
Vos, K., Vandenberghe, N., and Elsen, J. (2014). Surface textural analysis of quartz grains by scanning electron microscopy (SEM): from sample preparation to environmental interpretation. J. Earth-Science Rev. 128, 93–104. doi:10.1016/j.earscirev.2013.10.013
Wang, Q. T., Lu, H., Shen, C. C., Liu, J. Z., Peng, P., and Hsu, C. S. (2014). Impact of inorganically bound sulfur on late shale gas generation. J. Energy and Fuels 28, 785–793. doi:10.1021/ef401468w
Warren, J. (2000). Dolomite: occurrence, evolution and economically important associations. J. Earth Sci. Rev. 52, 1–81. doi:10.1016/S0012-8252(00)00022-2
Weger, R., Murray, S., McNeill, D., Swart, P. K., Eberli, G. P., Blanco, L. R., et al. (2019). Paleothermometry and distribution of calcite beef in the vaca muerta formation, neuquén basin, Argentina. J. AAPG Bull. 103, 931–950. doi:10.1306/10021817384
Wilkinson, M., Haszeldine, R. S., and Milliken, K. L. (2004). “Cross-formational flux of aluminium and potassium in gulf coast (USA) sediments,”. Clay mineral cements in sandstones. Editors R. Worden, and S. Morad (Washington, DC: J. International Association of Sedimentologists Special Publication), 34, 147–160. doi:10.1002/9781444304336.ch7
Wu, H. G., Hu, W. X., Tang, Y., Cao, J., Wang, X. L., Wang, Y. C., et al. (2017). The impact of organic fluids on the carbon isotopic compositions of carbonate-rich reservoirs: case study of the Lucaogou Formation in the Jimusaer Sag, Junggar Basin, NW China. J. Mar. Petroleum Geol. 85, 136–150. doi:10.1016/j.marpetgeo.2017.05.003
Wu, L. Y., and Gu, X. Z. (1986). The application of pyrolysis Technique in source rock research. J. Acta Pet. Sin. J. 7, 13–19. doi:10.7623/syxb198602003
Wu, S. T., Zhu, R. K., Cui, J. G., Cui, J. W., Bai, B., Zhang, X. X., et al. (2015). Characteristics of lacustrine shale porosity evolution, triassic chang 7 member, Ordos Basin,NW China. J. Petroleum Explor. Dev. 42, 185–195. doi:10.1016/S1876-3804(15)30005-7
Xi, K. L., Cao, Y. C., Jahren, J., Zhu, R., Bjørlykke, K., Haile, B. G., et al. (2015). Diagenesis and reservoir quality of the lower cretaceous quantou formation tight sandstones in the southern Songliao Basin, China. J. Sediment. Geol. 330, 90–107. doi:10.1016/j.sedgeo.2015.10.007
Xi, Z. D., Tang, S. H., Zhang, S. H., Yi, Y. X., Dang, F., and Ye, Y. P. (2019). Characterization of quartz in the Wufeng Formation in northwest Hunan Province, south China and its implications for reservoir quality. J. Petroleum Sci. Eng. 179, 979–996. doi:10.1016/j.petrol.2019.04.051
Yang, X. R., Yan, D. T., W, X. S., Zhang, L. W., Zhang, B., Xu, H. W., et al. (2018). Different formation mechanism of quartz in siliceous and argillaceous shales: a case study of Longmaxi Formation in South China. J. Mar. Petroleum Geol. 94, 80–94. doi:10.1016/j.marpetgeo.2018.03.036
Zhang, J. G., Jiang, Z. X., Jiang, X. L., Wang, S. Q., Liang, C., and Wu, M. H. (2016). Oil generation induces sparry calcite formation in lacustrine mudrock, Eocene of east China. J. Mar. Petroleum Geol. 71, 344–359. doi:10.1016/j.marpetgeo.2016.01.007
Zhang, J. L., and Zhang, P. Z. (1996). A Discussion of pyrite catalysis on the hydrocarbon generation process. Adv. Earth Sci. 11, 282–287.
Zhang, S., Liu, H. M., Wang, M., Fu, A. B., Bao, S. Y., Wang, W. Q., et al. (2018). Pore evolution of shale oil reservoirs in Dongying Sag. J. Acta Pet. Sin. 9, 754–766. doi:10.7623/syxb201807003
Zhang, S. C., Zhang, H. L., Zha, M., Zhu, R. F., and Liu, Q. (2010). Control of pressure system development on reservoir formation in the Dongying sag. J. Petroleum Explor. Dev. 37, 289–296.
Zhang, Y. W., Jang, S. B., and Li, F. (2021a). Influence of sandstone – shale contact on feldspar diagenesis in the sandstone reservoir of the Shahejie Formation in the Dongying depression, Bohai Bay basin. J. Acta Geol. Sin. 95, 883–894. doi:10.19762/j.cnki.dizhixuebao.2021126
Zhang, Z., Pang, J., Yang, Y. T., Cao, Y. H., Qi, M. H., Zhang, Y. Y., et al. (2021b). Carbon and oxygen isotope characteristics and genesis of carbonate in sandstone of the 4th Member if Xujiahe Formation in the central western Sichuan depression, Sichuan basin, China. J. Acta Geol. Sin. 95. doi:10.19762/j.cnki.dizhixuebao.2022260
Zhao, X. Z., Zhou, L. H., Pu, X. G., Jin, M. F., Han, Z. W., Xiao, G. T., et al. (2018). Geological characteristics of shale rock system and shale oil exploration breakthrough in a lacustrine basin: a case study from the Paleogene 1st sub-member of Kong 2 Member in Cangdong sag,Bohai Bay Basin, China. J. Petroleum Explor. Dev. 45, 377–388. doi:10.1016/S1876-3804(18)30043-0
Keywords: mudstone, diagenesis, authigenic mineral, eocene, east China
Citation: Zhang Z, Jiang Z and Yang Y (2025) Coevolution of minerals in lacustrine mudstone during diagenesis: a case study of the dongying depression in the Bohai Bay Basin. Front. Earth Sci. 13:1502476. doi: 10.3389/feart.2025.1502476
Received: 26 September 2024; Accepted: 03 February 2025;
Published: 16 April 2025.
Edited by:
George Kontakiotis, National and Kapodistrian University of Athens, GreeceReviewed by:
Kunyue Ling, Chinese Academy of Sciences (CAS), ChinaAhmer Bilal, Shandong University of Science and Technology, China
Copyright © 2025 Zhang, Jiang and Yang. This is an open-access article distributed under the terms of the Creative Commons Attribution License (CC BY). The use, distribution or reproduction in other forums is permitted, provided the original author(s) and the copyright owner(s) are credited and that the original publication in this journal is cited, in accordance with accepted academic practice. No use, distribution or reproduction is permitted which does not comply with these terms.
*Correspondence: Zongxuan Zhang, enp4MTk5NDc1QDE2My5jb20=; Zaixing Jiang, amlhbmd6eEBjdWdiLmVkdS5jbg==