- 1State Key Laboratory of Oil and Gas Reservoir Geology and Exploitation, Chengdu University of Technology, Chengdu, China
- 2Southwest Petroleum University, Chengdu, China
- 3China National Offshore Oil Corporation, Zhanjiang Branch, Zhanjiang, China
In relatively stable sedimentary basins, the formation of buried-basement hill reservoirs mainly occurs after the basin basement forms and before the overlying sedimentary cover layer is filled. Represented by the Beibu Gulf Basin in the South China Sea, buried-basement hill reservoirs are key targets for hydrocarbon exploration in deep sedimentary basins. Limited exploration and research in buried-basement hills hinder the understanding of hydrocarbon accumulation conditions, thereby restricting large-scale hydrocarbon exploration. Geophysical methods, including the balanced section method and stress field analysis, were used to restore the formation and burial processes of buried-hills in the Weixinan Depression of Beibu Gulf Basin. The formation process of these buried-basement hills consists of five stages including diapiric uplift (Caledonian period), thrust (Indosinian-Yanshanian period), multiple exposure (Late Caledonian, and Indosinian-Yanshanian period), multiple burials (Hercynian period and Early Himalayan Period), and finalization (Late Himalayan Period). Three controlling factors of these buried-basement hills are determined: ①differences in the degree of granite intrusion lead to differences in karst paleogeomorphology; ②thrust movement of NE-SW direction during the Hercynian period; ③ thrust movement of NW-SE direction during the Indosinian-Yanshanian. The main controlling factors for the development of buried-carbonate basement hill reservoirs are multi-stage tectonic stress, karstification, and lithology. The tight buried-carbonate hill reservoir mainly composed of dissolved pore and fracture is concentrated in horizontal underflow zone. The main controlling factors for the development of buried-granite (or metamorphic rock) hill reservoirs are tectonic stress, and karstification. The reservoir mainly composed of dissolved pore and fracture is concentrated in leaching zone located at the top of each buried-granite and metamorphic rocks hill.
1 Introduction
Basement oil and gas reservoirs refer to oil and gas accumulation in the crystalline basement of a basin, with reservoir rock types consisting of metamorphic or igneous rocks (Walters, 1953; P’an, 1982). As exploration deepens, the academic community has proposed that the formation of basement hydrocarbon reservoirs is linked to basin evolution (Kosachev et al., 2019). In recent years, basement reservoirs have been defined as hydrocarbon or fluid reservoirs formed in crystalline basement or sedimentary rocks prior to basin formation (Kutcherov and Krayushkin, 2010; Carvalho et al., 2013). The reservoir lithology can be metamorphic or igneous rocks, or sedimentary rocks (Ye et al., 2022; Parnell et al., 2017; Wang et al., 2023). The statistical results of hydrocarbon exploration cases worldwide indicate that hydrocarbon resources carried by basement are widely distributed worldwide (Gao et al., 2008; Azaiez et al., 2018; Rodríguez-Cuevas et al., 2024). The global number of basement oil and gas fields and the proportion of recoverable hydrocarbon reserves are 9.3%, 10.2%, and 2.7%, respectively (Wang et al., 2024). Buried-basement hill reservoirs are an major type of basement reservoirs (Salah and Alsharhan, 1998; Mazzullo et al., 2009). The formation of buried-basement hills often involves complex tectonic evolution, along with alterations in diagenetic fluids, which complicate the reservoir formation mechanism (Xing, 1993; Guo et al., 2017). Moreover, compared to carbonate and clastic reservoirs, the exploration risk of buried-basement hill reservoirs is high (Zhao et al., 2015; Zhao et al., 2018), the degree of exploration is low, and the understanding of reservoir formation conditions and distribution patterns is still unclear.
The tectonic zone between the Eurasian and Pacific Plates is a key area for basement oil and gas reservoir distribution (Liu et al., 2022). Due to the compression and subduction of the Indosinian Pacific Plate, numerous buried-basement hills formed in the South China Sea, including the Beibu Gulf Basin (Kerimov et al., 2019). In recent years, exploration has revealed the enormous potential of hydrocarbon resources in buried-basement hill reservoirs (Wang et al., 2024; Deng et al., 2024). The geological setting of Bohai Bay basin is similar to that of Beibu Gulf Basin, with complex stratigraphic structure and diverse types of buried-hills (Yi et al., 2022; Zhu et al., 2024). The tectonic dynamics, reservoir formation conditions and hydrocarbon accumulation model of buried-hill formation in Bohai Bay basin have been gradually revealed in recent years (Xu et al., 2024).
Compared with the Bohai Bay Basin, in the Beibu Gulf Basin, there are multiple lithologies present in the reservoir, and the differences in reservoir space types, porosity, and permeability among different lithologies are significant, which restricts the efficient exploration and development of this type of hydrocarbon resources. The complex structural history leads to complex diagenetic alteration in the later stage (Liu et al., 2017; Qin et al., 2020), with strong heterogeneity in the degree of alteration. There is a lack of research on the controlling factors of reservoir formation in different rock types of basement (Zhao et al., 2019). Extensive drilling, logging, and geophysical data from the Beibu Gulf Basin provide valuable insights into the genesis of buried-basement hill reservoirs. Using the latest 2D and 3D seismic and drilling data, the Weixinan Depression in the Beibu Gulf Basin was chosen as the research focus, revealing the formation mechanism of buried-hills under tectonic evolution conditions. By combining elements, isotopes, and microscopic temperature measurements, the controlling factors of buried-basement hill reservoirs are analyzed, providing a theoretical foundation for efficient exploration.
2 Geological setting
The Beibu Gulf Basin extends from the Guangdong-Guangxi Uplift in the north to the Hainan Uplift in the south and the Leizhou Peninsula in the east. It is a Cenozoic rift basin in the northern South China Sea. Based on the tectonic pattern formed during the Paleogene extensional stage, the basin is divided into three primary structural units from north to south: the northern depression zone, the Qixi Uplift, and the southern depression zone (Figure 1A). The Weixinan Depression is located in the northern depression of the Beibu Gulf Basin and is one of the two largest secondary structural units within the depression (Li et al., 2021). The stratigraphic column in the Weixinan Depression is composed of the Paleogene Changliu Formation, Liushagang Formation, Weizhou Formation, Neogene Xiayang Formation, Jiaowei Formation, Dengloujiao Formation, Wanglougang Formation, and Quaternary System from the bottom to the top (Figure 2). The basin has gone through two major stages of tectonic evolution: the rift stage in the Paleogene and the depression stage in the Neogene Quaternary. The rifting stage deposited continental clastic rocks including the Changliu Formation, Liushagang Formation, and Weizhou Formation, while the depression stage mainly consisted of marine clastic rock construction (Figure 1B).
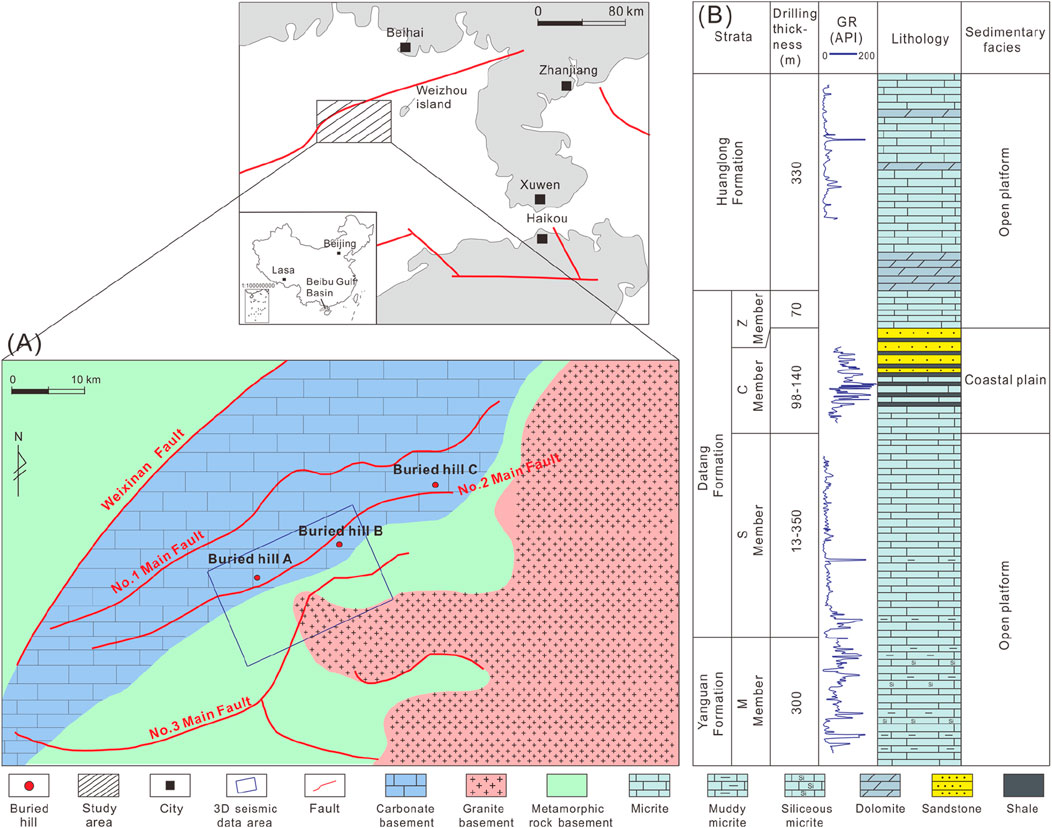
Figure 1. (A) Lithology of buried-basement hill and main fault distribution in Weixinan Depression of Northern Beibu Gulf Basin. (B) Stratigraphic column of buried-carbonate basement hill in Weixinan Depression of Northern Beibu Gulf Basin.
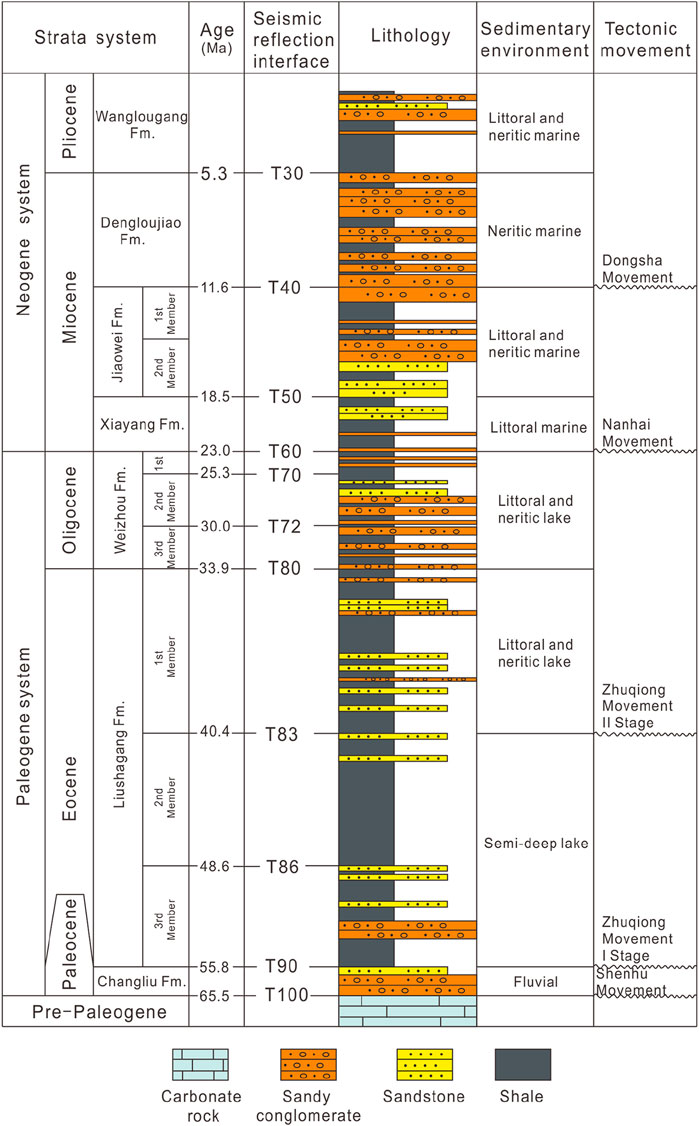
Figure 2. Stratigraphic column of overlying strata of buried-hill and tectonic evolution of Beibu Gulf Basin (modified from Li et al., 2018).
3 Samples and methods
3.1 Petrographic and geochemical analysis
First, 25 samples from drilling cores of the buried-hill reservoir were polished twice, then impregnated with blue epoxy and stained with Alizarin red to identify different rock fabrics. Next, petrographic relationships of the different fabrics were delineated using a CL8200MK5 cathodoluminescence (CL) microscope. Fluid-inclusion microthermometry was performed on 25 samples using a calibrated Linkam THMS600 cooling-heating stage. The thermal cycling method was used to determine fluid inclusion homogenization temperature (Th) and melting ice temperature (Tm). The measurement precision for Th is ±1°C, and ±0.1°C for Tm. Forty-one samples were selected for Sr isotope measurement using a Finnigan Triton thermal ionization mass spectrometer (TIMS). Forty-one samples were selected for stable isotopic (δ18O, δ13C) measurement using MAT253 isotope mass spectrometer. The δ18O and δ13C data were normalized to Vienna Pee Dee Belemnite (V-PDB) standard and corrected by fractionation factors proposed by Fairchild and Spiro (1987). All the above tests were conducted at Chengdu University of Technology.
3.2 Seismic data analysis
The 3D seismic survey network mainly covers the No.2 and No.3 Main Faults in the study area. The post-stack 3D seismic channel spacing is 12.5 m × 12.5 m, and the area is about 300 km2. The logging stratification and lithologic data of all wells in 3D seismic area are complete. The borehole seismic calibration of 15 wells in the 3D seismic area has been completed. The synthetic records are basically consistent with the characteristics of the well side channel wave group, with high correlation coefficient, good well seismic matching relationship, accurate horizon calibration results, and the interface of the buried-hill is moderately strong wave crest reflection, laying the foundation for the subsequent detailed interpretation of seismic horizon and the analysis of interval velocity field. Due to the weak reflection energy within the buried-hill, directly interpreting the three-dimensional reflection of high, steep faults from the original data volume is challenging. Therefore, this study enhances the seismic data by amplifying steep dip angle reflections and applying low-pass filtering. The enhanced seismic data highlights the fault reflections (high, steep reflection signals) within the buried-hill, aiding in fault characterization. The interpretation of high, steep faults within the buried-hill was completed by combining the original data volume, enhanced data volume, and fully stacked data volume after optimizing the gathers.
3.3 Analytical techniques of stress field
Numerical simulation of structural deformation is an interdisciplinary research area that integrates methods from geology, geophysics, geochemistry, mathematics, computational mechanics, and computer science. The principle of numerical simulation experiments on geological structure deformation is to follow the basic laws of geological development, rely on advanced computer processing systems, and use geological models constructed from solid earth science data as the experimental research basis. Structural fractures are primarily controlled by changes in the tectonic stress field. In stress concentration zones, when stress exceeds the fracture threshold, rock deformation and fractures occur, forming structural fractures. The formation and development of structural fractures mainly focus on two types of structures: first, fractures, which can cause local stress concentration along the periphery of the fractures and the intersection of multiple fractures; The second is folding. When the strata undergo folding deformation, stress concentration areas will be formed at the turning points, and crack development areas will be formed in areas with significant changes in folding curvature. In this study, to analyze the development of basement fractures in different structural units of the Beibu Gulf Basin, we selected drilling exploration wells for rock core observation and statistical fracture analysis. This was based on an analysis of the structural genesis system and the Cenozoic structural pattern. We constructed a geological model and applied numerical simulation methods for stress fields and fracture development across different periods of the basin. This allowed us to determine the extent and distribution of fractures in basement rocks across the main depressions of the basin. Based on the depth structure and elastic parameters of the top surface of the buried-hill (Figure 3A), the “thin-plate theory” is used for structural stress field analysis (Timoshenko and Woinowsky-Krieger, 1959; Zhou et al., 2011). Firstly, the elastic parameter data volume obtained from pre-stack seismic inversion is used to calculate the construction curvature and simulate stress distribution (Figure 3B).
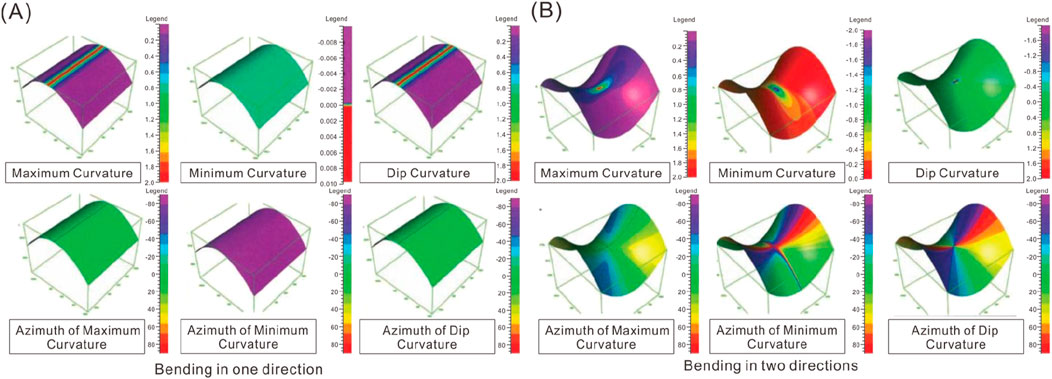
Figure 3. Schematic diagram of tectonic curvature and stress distribution applied in 3D seismic data. (A) Tectonic curvature and stress distribution in one direction. (B) Tectonic curvature and stress distribution in two direction.
Since Dahlstrom (1969) systematically proposed the balanced section method, this technique has gradually been widely adopted by geologists and has played a key role in verifying seismic interpretation and restoring structural deformation. The balanced section method analyzes the regional tectonic background, selects appropriate deformation mechanisms for interpreting the section, and applies geometric and kinematic principles to restore the deformation structures to the initial sedimentary, undeformed state. This method allows the characteristics of structural deformation at each stage of structural evolution to be visually represented on the section. By measuring the length changes of the section, strain variables and strain rates can be calculated, providing a more accurate basis for quantitative analysis of structural evolution history. To constrain the arbitrariness of balanced sections, geologists have established a series of rules in years of practical work to ensure the accuracy of balanced sections (Butler, 1983; Castelluccio et al., 2015; Mazur et al., 2018). These rules mainly include: (1) The area of the conservation profile remains unchanged before and after deformation, that is, the shortened area should be the same as the overlapping area of the strata. (2) The layer length before and after deformation remains unchanged, provided that the thickness of the rock layer does not change during the deformation process, only fractures and folds occur. (3) The conservation of displacement should be maintained along the same fault, and the displacement of each equivalent layer should be consistent.
4 Stratigraphic framework of buried-carbonate hill
According to the imaging logging results, the inclination of the Carboniferous strata in Buried-carbonate Basement Hill is mainly southeast, with a low dip angle and an average of about 21.6° (Figure 4). Speculation on the distribution characteristics of the inner strata of the carbonate buried-hill: The strata of the Buried-carbonate Basement Hill A are divided into S Member and M Member from bottom to top, with missing Z Member and C Member overlying. In contrast, the strata of Buried-carbonate Basement Hill C are divided into S Member, M Member, Z Member and C Member from bottom to top, and the underlying strata are currently not involved in drilling (Figure 1B).
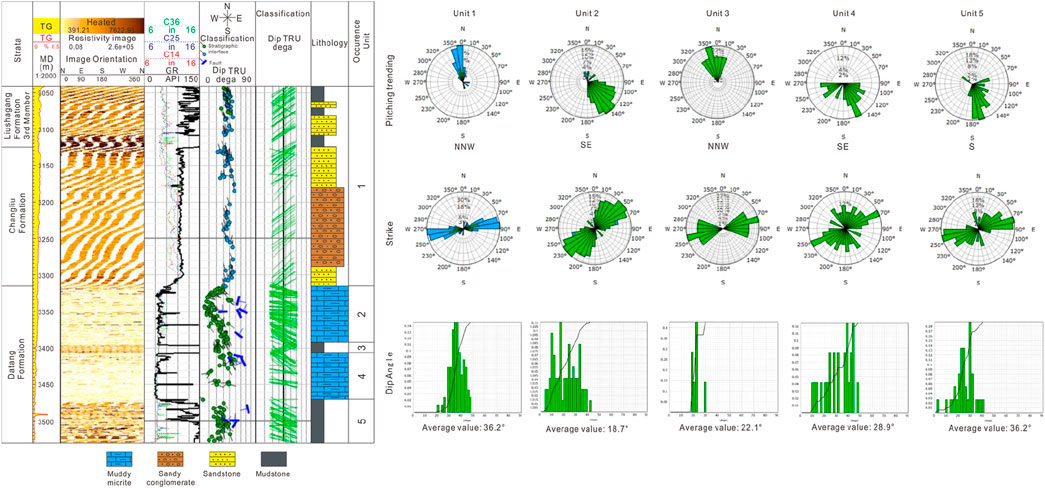
Figure 4. The occurrence of different stratigraphic units of buried-carbonate hill and overlying strata (Changliu Formation and Liu shagang Formation third Member) in Weixinan Depression (based on imaging logging data and drilling data).
5 Tectonic evolution of buried-hill
5.1 Analysis of stress field at the top of buried-hill
The stress field simulation based on three-dimensional seismic data is mainly through the elastic thin plate theory, which establishes the relationship between curvature and strain, and then establishes the relationship between strain and stress, so as to realize the prediction of in-situ stress. The thin plate bending theory holds that the stratum studied is uniform, continuous, isotropic and completely elastic, and that the formation of the stratum is completely formed by tectonic stress. In the stress prediction method based on curvature stress analysis, the key parameters are curvature, Young’s modulus, and Poisson’s ratio. To improve the accuracy of simulation results, a high-precision seismic layer velocity field is constructed based on multi-source information fusion. Time-depth conversion is performed on the interface of the submerged mountain top to obtain an accurate depth domain surface. The least squares method is then used to fit the trend surface and calculate its curvature. Young’s modulus and Poisson’s ratio data volumes are obtained through pre-stack inversion. The geostress is then calculated step by step, followed by outputting the structural stress field at the top of the buried-hill.
As mentioned earlier, the study area has developed both extensional controlled nearly EW oriented depression controlling faults that cut through the Paleogene (Wu et al., 2022), segmented NE oriented fault zones controlled by strike-slip, and a series of alternating NE oriented anticlines and synclines caused by compressive stress. Comprehensive analysis suggests that these structural phenomena are actually different manifestations of the same period of dextral shear deformation in different parts of the basin. Regional fault analysis shows that the inherited fault direction during the entire subsidence period in the study area is mainly NE-NNE, reflecting the overall NW in the study area (Figure 5). The simulation of tectonic stress field is based on the current deep tectonic surface, which is the comprehensive result of multiple tectonic processes. That is, the stress direction calculated by the strong stress plane diagram is the comprehensive result of multiple stress periods, approximately perpendicular to the direction of the current fault zone. The analysis of medium and weak stress fields can be used to analyze the stress characteristics of multiple tectonic periods, as shown in the three sets of stress directions in the figure (Figure 6A). Among them, the stress characteristics in the NW direction (green) are consistent with the stress direction of the first episode of the second belt rift. Characteristics of weak stress field at the top of buried-hill: In the area far away from the major fault, there are multiple sets of weak stress, whose direction is approximately consistent with the multi period stress direction in the study area. Characteristics of strong stress field at the top of buried-hill: Strong stress is concentrated near main faults (No. 2 and No. 3 main fault zones) (Figure 6), with a stress direction near the north-south direction (Figure 6B).
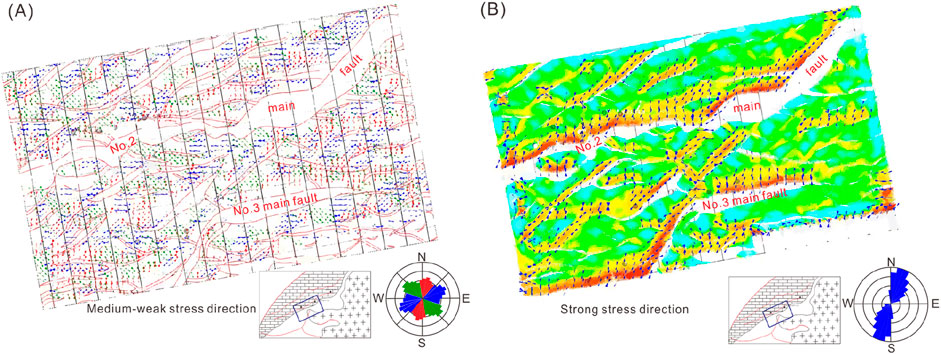
Figure 6. Characteristics of stress field at the top of buried-hills in Weixinan Depression. (A) Characteristics of medium and weak stress fields. (B) Characteristics of strong stress fields.
5.2 Seismic reflection characteristics of stratigraphic interfaces
Based on the seismic reflection characteristics of the main Paleogene interfaces in the Beibu Gulf Basin, and regional structural data, the geological structural attributes of each interface were analyzed, and the interfaces were classified. The first level unconformity is a tectonic cycle interface with tectonic transformation significance, corresponding to plate convergence events, with a large impact range. The secondary unconformity surface represents the interface for the transformation of the basin’s internal structural system, corresponding to periodic structural events within the basin. Therefore, T100 and T60 belong to the first order sequence boundary, which is a regional structural boundary with significant structural unconformity characteristics. They can be identified, tracked, and compared in the Beibu Gulf Basin and even in the entire northern margin of the South China Sea. The T100 interface represents the interface at which the basin enters the initial stage of rifting, and belongs to the unconformity interface of faulting (Figure 7). In the Beibu Gulf Basin, the reflection characteristics of the T100 interface are relatively obvious, but in some areas, the reflection characteristics of the T100 interface are unclear and difficult to identify and track. The T60 interface is the interface where the basin transforms from a rift to a depression (Figure 7), belonging to a post rift unconformity interface. It is also the interface where structural inversion occurs in the basin area, and the interface reflection is relatively clear throughout the entire basin.
During the formation of the Beibu Gulf Basin, the regional stress field underwent a clockwise rotation due to the combined forces of the Indian-Australian Plate, Pacific Plate, and the subduction of the ancient South China Sea. Based on the period of significant changes in the regional stress field, a second-order sequence boundary can be identified: T83, which represents the interface where the regional stress field transitions from NW-NNW to near NS direction. Before the T83 interface, the Indian Plate moved northward and began to collide with the Eurasian Plate, forming a series of early NE-NEE trending faults, mainly developing fluvial lacustrine sediments; After the T80 interface, it was subjected to the southward extrusion of the Indosinian Plate and the subduction of the ancient South China Sea, forming a series of late stage near EW trending faults (Figure 7), mainly developing coastal shallow lake delta sediments. In the late stage of fracturing, the basin transformed into marine facies, mainly developing coastal shallow sea to shallow sea sedimentary facies. In addition, the T86, T80, T72, T70 and other interfaces in the basin represent local tectonic change events and are third-order sequence interfaces (Figure 7).
5.3 Tectonic evolution and formation process of buried-carbonate hill
During the Jinning Movement, Precambrian metamorphic rocks formed the basin’s basement. During the Silurian period, influenced by the Caledonian Orogeny, northwest-southeast compression caused crustal uplift and thickening, leading to the reactivation of the geosyncline fold. The Weixinan Fault formed during this period and began to experience reverse movement. The magma in the southeastern study area transformed the metamorphic basement through diapiric intrusion/fault intrusion, resulting in the formation of paleogeomorphology with significant height variations following the uplift of the study area (Figure 8A). The southeastern part of the study is characterized by intense magma intrusion and thin overlying metamorphic rocks, which have started to undergo differential weathering and erosion. During the Hercynian period, uplift caused by early magma intrusion and a relative sea-level decline prevented deposition of the Devonian system in the study area, leading to continued weathering and erosion. Entering the Carboniferous period, influenced by the Hercynian Movement, the Weixinan Fault slid back, causing overall subsidence in the study area (Figure 8B). Coastal clastic rocks and marine carbonate rocks were continuously deposited on a large scale, including the Lower Carboniferous M Member, S Member, C Member, Z Member, and Upper Carboniferous Huanglong Formation. During this period, the paleogeomorphology caused by the Caledonian thrust were still maintained to a certain extent. The overlying metamorphic rocks in the area where Buried-hill A is located were completely eroded, exposing granite and undergoing atmospheric freshwater leaching and alteration. The undulating paleogeomorphology result in differences in the Carboniferous strata, with the depocenter located in the northwest of the study area and gradually tapering off towards the southeast. During the Late Permian Dongwu Movement, under the influence of NE-SW compressive stress, local thrust structures were formed due to active internal faults in the study area, and the overlying Carboniferous began to undergo differential erosion (Figure 8C).
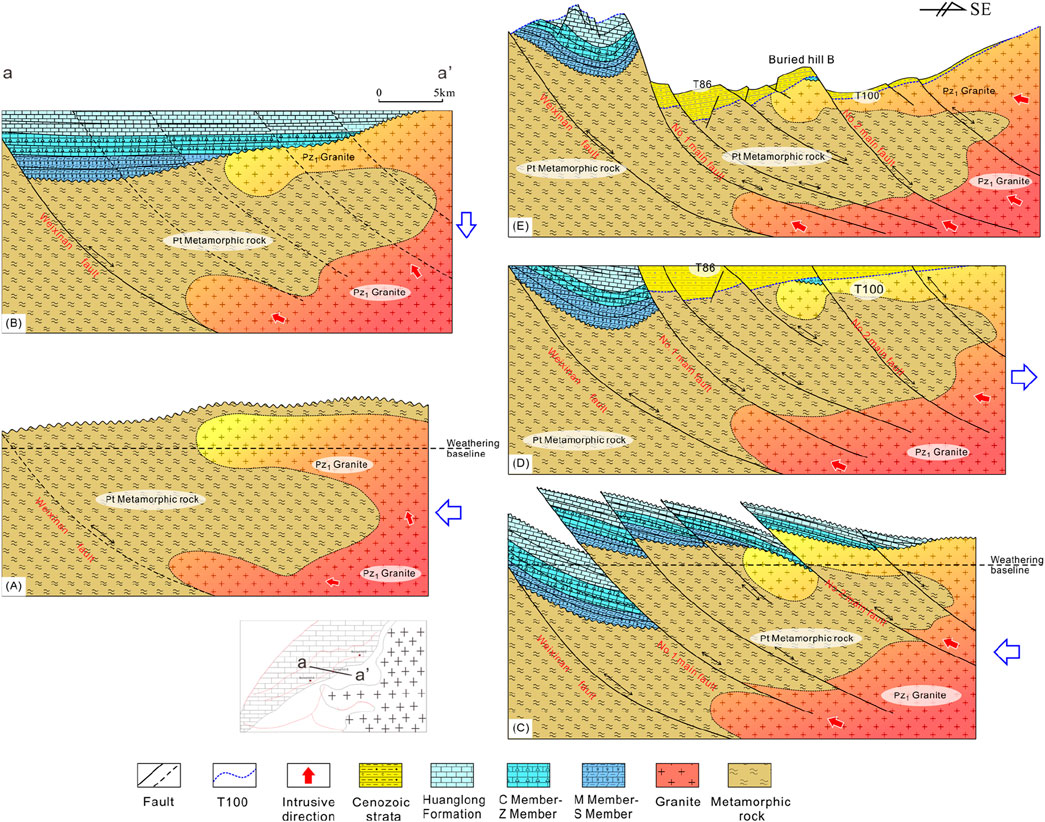
Figure 8. Tectonic evolution and formation process of buried-carbonate hill in Weixinan Depression. (A) The diapirism uplift of the basement leads to the exposure of granite and metamorphic rocks above the water surface. The first-stage buried-hills formed by weathering of granite and metamorphic rocks. (B) The sedimentary strata of the Carboniferous system led to the deep burial of the first-stage buried-hills (granite and metamorphic rocks). (C) The reverse thrust caused weathering of the Carboniferous carbonate rock, resulting in the formation of the second-stage of buried-hills (carbonate rock). (D) The Cenozoic sedimentary strata led to the deep burial of the second-stage of buried-hills. (E) The morphology of buried-hills was finally finalized in the Quaternary period.
During the Permian-Middle Triassic period, under the influence of the Indosinian Movement, strong compression occurred from the southeast to the northwest. The hidden faults formed during the Caledonian period were reactivated, and a series of strong northeast trending thrust structures were mainly formed by the Weixinan Fault, the No.1 main fault, the No.2 main fault, and the No.3 main fault. The Carboniferous system underwent stepped differential weathering and erosion. The stress primarily originates from the southeast direction, and the hanging plates of the No.1, No.2, and No.3 main faults being pushed to higher, more weathered positions, corresponding to stronger erosion. The lower plate of the No. 1 fault is positioned at a relatively lower elevation, corresponding to a lower degree of erosion. In the early stage of the Himalayan Movement from the Late Cretaceous to the Paleocene, there was regional northwest southeast tension, and the Weixinan Fault, the No.1 main fault, the No.2 main fault, and the No.3 main fault began to gradually reverse and slide back. The No.3 main fault experienced a late back slip, resulting in the interruption of Cenozoic sedimentation. The intensity of fault activity is strong in the southwest and gradually weakens towards the northeast, receiving alluvial fan sedimentation represented by the Changliu Formation (Figure 8D). In the late Himalayan period, the tensional-shear faulting ended, and the morphological characteristics of the Paleozoic buried-hills no longer changed (Figure 8E).
5.4 Tectonic evolution and formation process of buried-granite (and metamorphic rock) hill
During the Jinning Movement, the basement of the Weixinan Depression was composed of Proterozoic metamorphic rocks (Figure 8A). During the Silurian period, influenced by the Caledonian Orogeny, the crust was compressed in a northwest-southeast direction, resulting in uplift and thickening, with the geosyncline folds reactivating. The Weixinan Fault began to form and undergo reverse movement. The magma in the southeastern margin of the study area dissolved the metamorphic rock basement through basal intrusion/fault intrusion, while causing uplift. The uplifted granite and metamorphic rocks underwent differential weathering and erosion until the Devonian period (Figure 8A). Affected by the Carboniferous Hercynian Movement, the Weixinan Fault slid back, and the southwestern part of the study area sank and received Upper Carboniferous sediments (Figure 8B). During the Late Permian Dongwu Movement, NE-SW compressive stress induced internal fractures and formed local thrust structures, resulting in differential erosion of overlying Carboniferous carbonate rocks. During the Permian-Middle Triassic period, under the influence of the Indochina Movement, there was a strong compression from southeast to northwest, and the hidden faults formed during the Caledonian period were reactivated. A series of northeast trending thrust structures represented by the Weixinan Fault, the No.1 Main Fault, the No.2 Main Fault, and the No.3 Main Fault have caused the Carboniferous strata to be weathered again (Figure 8C).
During the Late Cretaceous Paleocene, there was regional NW-SE tension, and the Weixinan Fault, No.1 Main Fault, No.2 Main Fault, and No.3 Main Fault began to gradually reverse and slide back. The third main fault experienced a relatively late back slip, resulting in relatively thin Cenozoic sedimentary strata in the eastern study area (Figure 8D). Under the action of regional tension, many tensile fractures were formed inside the buried-hill (Figure 8D). In the late Himalayan period, the tensional-shear faulting ended, and the morphological characteristics of the Paleozoic buried-hills no longer changed (Figure 8E). In summary, there are two stages in the formation process of metamorphic rock buried-hills. The formation process of carbonate rocks buried-hills is limited to only one stage.
6 Controlling factors for gas reservoir
6.1 Epidiagenetic-stage karstification
6.1.1 Petrological evidence
Microscopic petrological characteristics suggest that both buried-carbonate and granite (metamorphic) hills have been altered by prolonged atmospheric freshwater exposure after being uplifted above sea level. The lithology of buried-carbonate hills is composed of micrite, and numerous of intraparticle dissolution pores and fractures have been observed inside the host rock (micrite) (Figure 9A). The dissolved rock fabric has no selective characteristics and aligns with the characteristics of epidiagenetic karstification. The reservoir spaces in the granite (metamorphic rock) buried-hill reservoir are also composed of dissolution pores and fractures resulting from weathering leaching (Figures 9B, C). In thin-section, intense leaching causes unstable mineral particles such as feldspar to transform into clay mineral (Figure 9D), and fracture leads to mylonization of feldspar (Figure 9E), mica, and other minerals (Figure 9F). Rock core observations show that the development of karst pores and fractures increases from bottom to top, while cementation strengthens in the same direction. Under cathodoluminescence (CL) microscopy, multi-stage calcite vein exhibit bright red, distinct from the host rock (micrite) (Figures 9G, H).
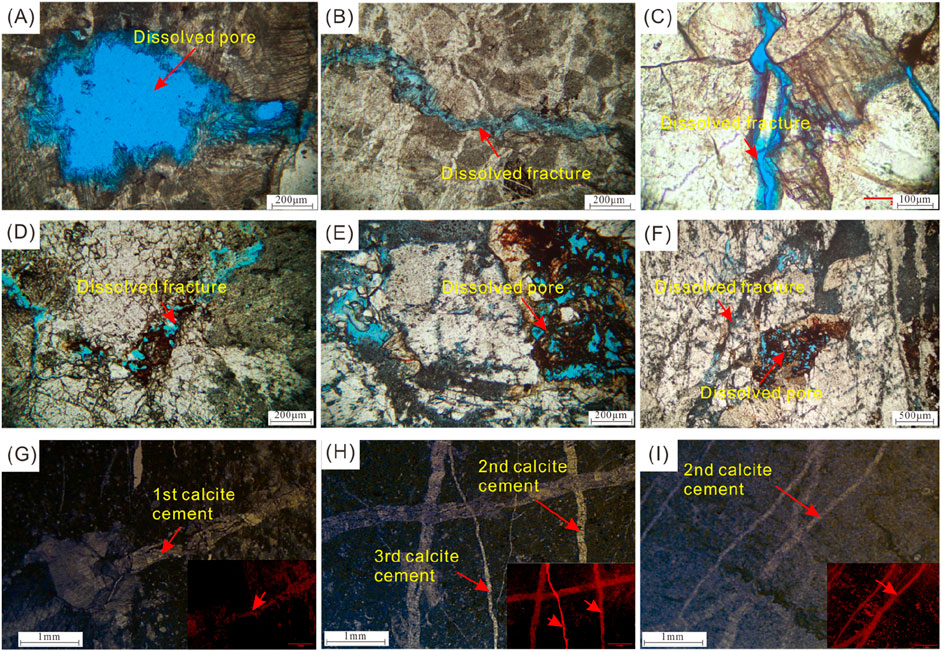
Figure 9. Petrological evidence of epidiagenetic-stage karstification in buried-basement hill in Weixinan Depression. (A) Intragranular dissolved pores in micrite. The pore size has exceeded 500 μm. (B) Dissolved fractures in micrite. The fracture width is 100 μm. (C) Dissolved fractures cutting through calcite crystals of the micrite matrix. The fracture width is 100 μm. (D) Dissolved fractures in granite cutting through feldspar grain. (E) Intragranular dissolved pores in feldspar grain of granite. (F) Intragranular dissolved pores and dissolved fractures in feldspar grains of granite. (G) The first-stage calcite cement of structural fracture in carbonate matrix. (H) The second-stage and third-stage calcite cement of structural fracture. (I) The second-stage calcite cement of structural fracture.
6.1.2 Carbon-oxygen stable isotope evidence
The abundance of δ13C and δ18O in marine carbonates is mainly influenced by factors such as sea level rise and fall, organic carbon sources and burial rates, and redox conditions in sedimentary diagenetic environments (Veizer et al., 1999; Berner, 2006). Thus, the carbon and oxygen isotope compositions of carbonate rocks vary depending on the sedimentary diagenetic environment and diagenetic fluid properties. In this study, according to the calculation formula proposed by Keith and Weber (1964), δ13C and δ18O were used to calculate the Z value and determine the diagenetic environment of calcite veins. The calculation formula is: paleo-salinity Z = 2.048 x (δ 13C + 50)+0.498 x (δ18O+50). When Z>120%, it is a marine diagenetic environment, and when Z<120%, it is a terrestrial diagenetic environment (Keith and Weber, 1964). The measured C-O isotopes of calcite vein and host rock are negatively biased compared to the original coeval seawater (Figure 10A), mainly due to the influence of atmospheric freshwater leaching. The calculated salinity Z value is mostly less than 120, suggesting marine limestone affected by fresh water. Moreover, the dilution effect of atmospheric fresh water on carbon and oxygen isotopes leads to negative measurement results (Figure 10A). The negative offset phenomenon in Vertical seepage zone is more significant than in horizontal runoff zone (Figure 10B).
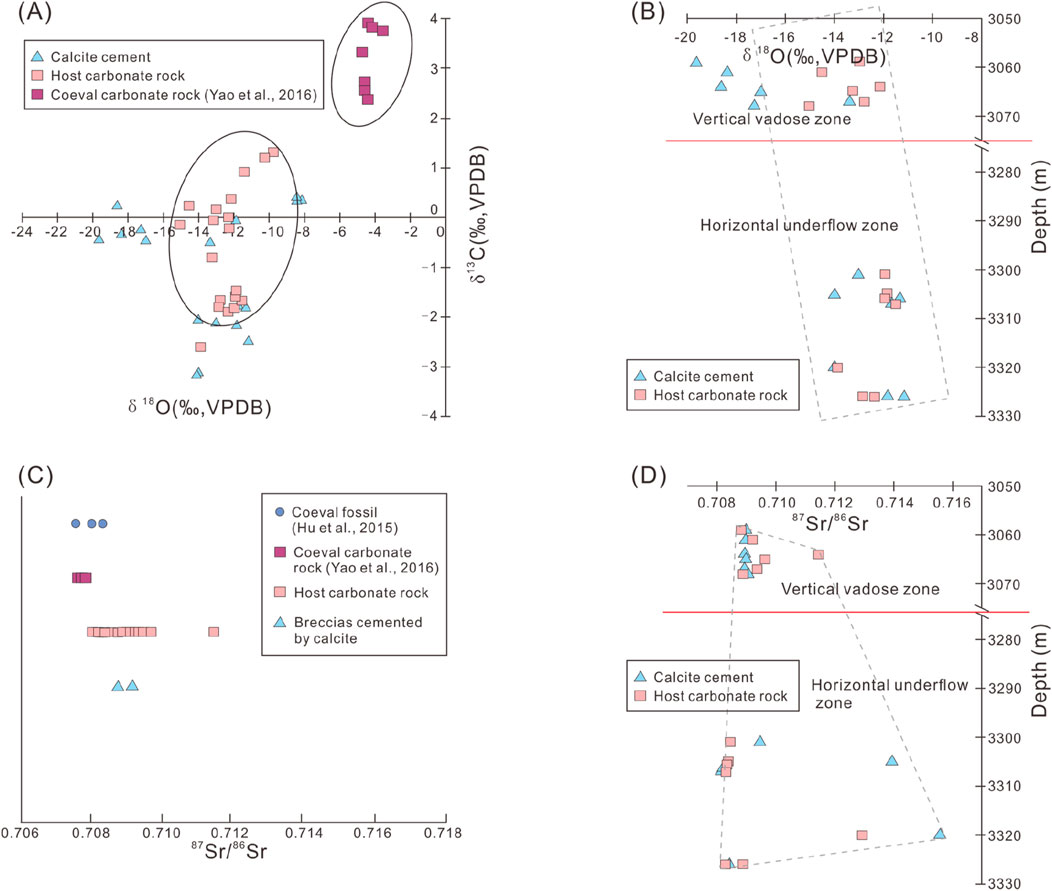
Figure 10. Inorganic geochemical characteristics of buried-hill reservoirs in Weixinan Depression. (A) Carbon and oxygen isotope crossplot. (B) Burial depth and oxygen isotope crossplot. (C) Strontium isotope distribution. (D) Burial depth and strontium isotope crossplot.(some data are from Hu et al., 2015; Yao et al., 2016).
6.1.3 Strontium isotope evidence
The host rock composed of micrite belongs to the Carboniferous Visean stage, and the coeval conodont and carbonate 87Sr/86Sr is between 0.7076 and 0.7080 (Chen et al., 2018). In contrast, the strontium isotope values of host rock and calcite cement are higher (Figure 10C). Previous studies indicate that mantle-derived fluids and connate seawaters have considerably low Sr isotopic compositions (Gu et al., 2023). The elevated 87Sr/86Sr ratios should be ascribed to the diagenetic fluids of more radiogenic Sr It is suggested that freshwater constitutes the main body of diagenetic fluids. The 87Sr/86Sr ratios in horizontal underflow zone is higher than that of vertical vadose zone (Figure 10D).
6.2 Faulting activity
The South China Sea, which contains the Beibu Gulf Basin, has experienced multiple tectonic movements since the Mesozoic. These tectonic movements have resulted in the multi-stage development of faults (Figure 11), influencing the formation and evolution of buried-hills in the Beibu Gulf Basin. They are the dominant factor in the evolution of buried-hill structures, affecting both the internal structural styles and external morphology of the buried-hills.
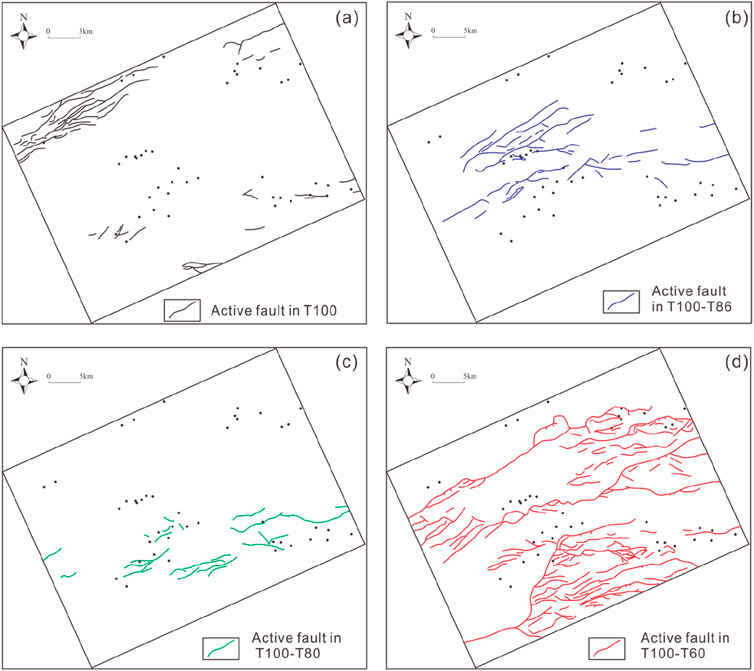
Figure 11. Distribution of active faults in different periods and drilling well locations in Weixinan Depression. (A) Active fault in T100. (B) Active fault in T100-T86. (C) Active fault in T100-T80. (D) Active fault in T100-T60.
Tectonic processes such as uplift, compression, and extension form large-scale faults, providing pathways for fluid transport in water-rock interactions. Fault activity produces numerous cracks and fractured zones in rocks. These cracks create channels for fluid migration, allowing hydrothermal fluids, groundwater, and other fluids to move through rock layers. Hydrothermal fluids, carrying abundant minerals, rise or diffuse along fault zones. They undergo chemical reactions with surrounding rocks, dissolving mineral components and transforming pore structures, thus increasing porosity and permeability.
The rate of fault activity significantly affects reservoir properties and is a primary factor in fracture development. A higher rate of fault activity generates numerous fractures, increases pore space, promotes dissolution to form secondary pores, and thus enhances porosity. Simultaneously, more and wider fractures interconnect to become efficient flow channels, increasing reservoir permeability but also enhancing permeability heterogeneity. The impact of lower fault activity rates on these aspects is relatively weak.
7 Reservoir formation mechanism of buried-basement hill
The vertical zonation of buried-basement hill reservoir has been proposed by several literature (Salah and Alsharhan, 1998; Luo et al., 2005; Plotnikova, 2006), but the specific zonation scheme has not been unified (Zhang et al., 2024). Salah and Alsharhan (1998) divided the buried-basement hill into four zones based on the degree of alteration. On the basis of well logging interpretation, another more specific scheme was proposed (Luo et al., 2005), which is to divide the buried-hill reservoir into three zones: Top weathered zone, Middle fracture zone, and Lower tight zone. However, Xu et al. (2024) proposed that fractures only develop in the bottom and top zones, with the middle zone having low permeability. The vertical zonation of the buried-hill reservoir should be a joint result of basement lithology, faulting events, and diagenetic events (Wang et al., 2023; Zhang et al., 2024). By observing the core and logging curve shapes of different types of basement rock, the vertical zonation scheme of buried-hills that conforms to different types of basement was summarized in this study. For buried-carbonate basement hill reservoirs, the lithology is easily dissolved carbonate rocks with obvious vertical zonation characteristics (Figure 12). Based on previous studies and logging responses in this study (He et al., 2013), the vertical zonation of buried-carbonate hill are divided into four zones (Figure 13): surface karst zone, vertical vadose zone, horizontal underflow zone and deep slow flow zone (Xie et al., 2024). In surface karst zone, the filling of breccias and calcite cement leads to the underdevelopment of pores. The cementation effect of vertical vadose zone is strong, and the dissolved pores are almost completely cemented. The reservoir mainly composed of dissolved pore and fracture is concentrated in horizontal underflow zone. In addition to epidiagenetic karst, other diagenesis, including dolomitization, recrystallization and so on, can almost be ignored in the lithological transformation of buried-carbonate hills. Because the induction of these diagenesis requires harsh conditions, the range and intensity of action are extremely limited. The lithologic logging results of drilling also confirmed this view (Figure 12).
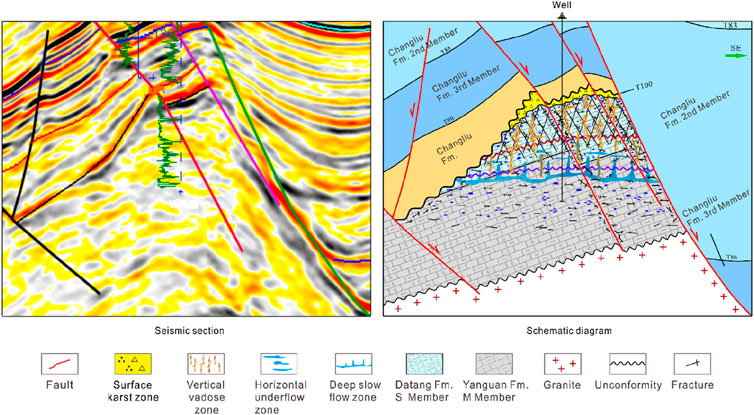
Figure 13. Formation model of buried-carbonate basement hill reservoir in Weixinan Depression (modified from Xie et al., 2024).
For aluminosilicate-rich rocks represented by granite and metamorphic rocks, Zhang et al. (2024) proposed a unified classification scheme for weathering crust structure. This classification scheme is composed of fractured basement zone, disintegration zone, and leaching zone from bottom to the top (Figure 14). Due to the minerals that form granite and metamorphic rocks is aluminosilicate minerals, their chemical properties are much more stable than those of carbonate rocks. Structural fractures play a decisive role in chemical alteration and dissolution of aluminosilicate minerals. From the bottom of the leaching zone to the surface of the buried-granite and metamorphic rocks basement hill, the chemical alteration of aluminosilicate minerals along various fractures is enhanced continuously, forming clay minerals and dissolved pores, and the rock sbecome loose and sandy. The reservoir mainly composed of dissolved pore and fracture is concentrated in leaching zone located at the top of each buried-granite and metamorphic rocks hill (Figure 14).
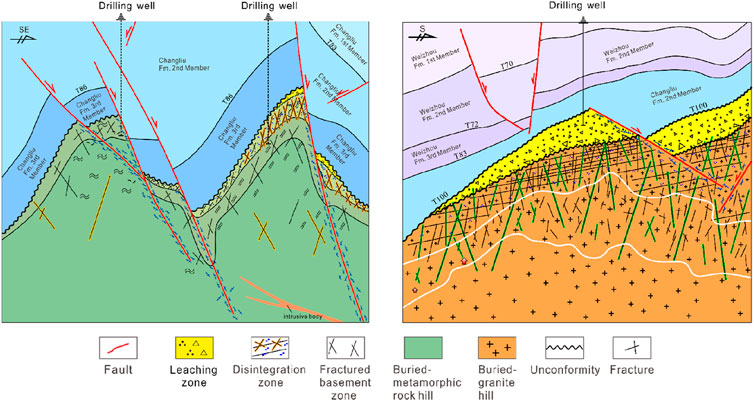
Figure 14. Formation model of buried-granite (or metamorphic rock) basement hill reservoir in the Weixinan Depression.
8 Conclusion
The petrographic features, seismic section and isotope evidence of the Pre-Paleogene, Paleogene and Neogene strata were studied to determine the Formation process of buried-hill and formation mechanism of reservoir in the Weixinan Depression of Northern Beibu Gulf Basin.
(1) The stress field at the top of the buried-basement hills has been revealed. Multiple sets of medium-weak stresses are observed far from the main fault area, and their directions are approximately consistent with the multi-stage stress directions in the Weixinan Depression of the Beibu Gulf Basin. Strong stress is concentrated near the main faults (No.2 and No.3 Main Fault), with the stress direction being nearly north-south.
(2) The buried-basement hills have undergone multiple periods of uplift, exposure, and burial. The formation of buried-basement hills involves several stages: diapiric uplift, thrusting, exposure, multiple burials, and finalization. Three factors control the formation of buried-basement hills: ① differences in the degree of granite intrusion, which lead to variations in karst paleogeomorphology; ②NE-SW thrusting during the Hercynian period; ③NW-SE thrusting during the Indosinian-Yanshanian.
(3) The reservoir spaces of tight buried-basement hills are composed of dissolved pores, dissolved fractures and structural fractures. The main controlling factors for the development of buried-carbonate basement hill reservoirs are multi-stage tectonic stress, karstification, and lithology. Lithology of buried-carbonate hill determines the degree of dissolution, while tectonic movements cause the uplift and erosion of the Carboniferous carbonate strata. Early-stage fractures provide favorable seepage space for subsequent karst processes, while late-stage fractures increase reservoir permeability. The main factors controlling the development of buried-granite (or metamorphic rock) hill reservoirs are tectonic stress and karstification.
Data availability statement
The raw data supporting the conclusions of this article will be made available by the authors, without undue reservation.
Author contributions
AL: Conceptualization, Methodology, Writing–original draft. FX: Funding acquisition, Investigation, Software, Visualization, Writing–original draft. CF: Data curation, Formal Analysis, Project administration, Writing–review and editing. ML: Investigation, Methodology, Writing–original draft. FJ: Methodology, Project administration, Supervision, Writing–review and editing. GX: Project administration, Resources, Supervision, Validation, Writing–review and editing. XX: Resources, Validation, Visualization, Writing–review and editing. XZ: Conceptualization, Investigation, Methodology, Writing–review and editing. BX: Data curation, Formal Analysis, Investigation, Writing–review and editing.
Funding
The author(s) declare that financial support was received for the research and/or publication of this article. This study was funded by the National Natural Science Foundation Youth Foundation Project of China (No. 42302186).
Conflict of interest
Author CF, ML and FJ are employed by China National Offshore Oil Corporation.
The remaining authors declare that the research was conducted in the absence of any commercial or financial relationships that could be construed as a potential conflict of interest.
Generative AI statement
The author(s) declare that no Generative AI was used in the creation of this manuscript.
Publisher’s note
All claims expressed in this article are solely those of the authors and do not necessarily represent those of their affiliated organizations, or those of the publisher, the editors and the reviewers. Any product that may be evaluated in this article, or claim that may be made by its manufacturer, is not guaranteed or endorsed by the publisher.
References
Azaiez, H., Gabtni, H., Bédir, M., and Campbell, S. (2018). Aeromagnetic study of buried basement structures and lineaments of sahel region (eastern Tunisia, north africa). Arabian J. Geosciences 11 (7), 140. doi:10.1007/s12517-018-3476-8
Berner, R. A. (2006). Geocarbsulf: a combined model for phanerozoic atmospheric O2 and CO2. Geochimica Cosmochimica Acta 70 (23), 5653–5664. doi:10.1016/j.gca.2005.11.032
Butler, R. W. H. (1983). Balanced cross-sections and their implications for the deep structure of the northwest Alps. J. Struct. Geol. 5 (2), 125–137. doi:10.1016/0191-8141(83)90038-X
Carvalho, I. S., Mendes, J. C., and Costa, T. (2013). The role of fracturing and mineralogical alteration of basement gneiss in the oil exhsudation in the Sousa Basin (Lower Cretaceous), Northeastern Brazil. J. S. Am. Earth Sci. 47, 47–54. doi:10.1016/j.jsames.2013.06.001
Castelluccio, A., Andreucci, B., Zattin, M., Ketcham, R. A., Szaniawski, R., Mazzoli, S., et al. (2015). Coupling sequential restoration of balanced cross sections and low-temperature thermochronometry: the case study of the western carpathians. Lithosphere 7 (4), 367–378. doi:10.1130/L436.1
Chen, J., Montanez, I. P., Qi, Y., Shen, S., and Wang, X. (2018). Strontium and carbon isotopic evidence for decoupling of pco(2) from continental weathering at the apex of the late paleozoic glaciation. Geology 46 (5), 395–398. doi:10.1130/G40093.1
Dahlstrom, C. (1969). Balanced cross sections. Can. J. Earth Sci. 6 (4), 743–757. doi:10.1139/e69-069
Deng, Y., Hu, D., Zhu, J., Liu, G., Chen, K., and Tong, C. (2024). Hydrocarbon accumulation regularities, newfields and new types of exploration,and resource potentials in Beibuwan Basin. Acta Pet. Sin. 45 (1), 202–225. doi:10.7623/syxb202401012
Fairchild, J., and Spiro, B. (1987). Petrological and isotopic implications of some contrasting late Precambrian carbonate, NE Spitsbergen. Sedimentology 34 (6), 793–989. doi:10.1111/j.1365-3091.1987.tb00587.x
Gao, X., Pang, X., Li, X., Chen, Z., Shan, J., Liu, F., et al. (2008). Complex petroleum accumulating process and accumulation series in the buried-hill trend in the rift basin:an example of Xinglongtai structure trend, liaohe subbasin. Sci. China Ser. D Earth Sci. 51 (Suppl. II), 108–116. doi:10.1007/s11430-008-6022-9
Gu, Y., Wang, Z., Yang, C., Luo, M., Jiang, Y., Luo, X., et al. (2023). Effects of diagenesis on quality of dengying formation deep dolomite reservoir, Central Sichuan Basin, China: insights from petrology, geochemistry and in situ U-Pb dating. Front. Earth Sci. 10, 1041164. doi:10.3389/feart.2022.1041164
Guo, Z., Ma, Y., Liu, W., Wang, L., Tian, J., Zeng, X., et al. (2017). Main factors controlling the formation of basement hydrocarbon reservoirs in the Qaidam Basin, western China. J. Petroleum Sci. Eng. 149, 244–255. doi:10.1016/j.petrol.2016.10.029
He, J., Fang, S. X., Hou, F. H., Yan, R. H., Zhao, Z. J., Yao, J., et al. (2013). Vertical zonation of weathered crust ancient karst and the reservoir evaluation and prediction-A case study of M55-M51 sub-members of Majiagou Formation in gas fields, central Ordos Basin, NW China. Petroleum Explor. Dev. 40 (5), 534–542. doi:10.11698/PED.2013.05.04
Hu, Z., Li, Y., Li, B., Huang, S., and Han, X. (2015). An overview of the strontium isotopic composition of phanerozoic seawater. Adv. Earth Sci. 30 (1), 37–49. doi:10.11867/j.issn.1001-8166.2015.01.0037
Keith, M. L., and Weber, J. N. (1964). Carbon and oxygen isotopic composition of selected limestones and fossils. Geochimica Cosmochimica Acta 28 (10-11), 1787–1816. doi:10.1016/0016-7037(64)90022-5
Kerimov, V. Y., Leonov, M. G., Osipov, A. V., Mustaev, R. N., and Hai, V. N. (2019). Hydrocarbons in the basement of the South China Sea (Vietnam) Shelf and structural–tectonic model of their formation. Geotectonics 53 (1), 42–59. doi:10.1134/S0016852119010035
Kosachev, I., Kayukova, G., and Mikhailova, A. N. (2019). Features of composition of oil extracts from the rocks of basement and sedimentary stratums of the novo-elkhovskoye field of tatarstan. Petroleum Sci. Technol. 37, 1016–1024. doi:10.1080/10916466.2018.1564771
Kutcherov, V. G., and Krayushkin, V. A. (2010). Deep-seated abiogenic origin of petroleum: from geological assessment to physical theory. Rev. Geophys. 48 (1), RG1001. doi:10.1029/2008RG000270
Li, C., Fan, C., Hu, L., Hu, Q., and Chen, L. (2021). Tectonic evolution characteristics and genesis of Weixi’nan low uplift in Beibu Gulf Basin. Mar. Orig. Pet. Geol. 26 (4), 319–325. doi:10.3969/j.issn.1672-9854.2021.04.004
Li, C., Yang, X., Fan, C., Hu, L., Dai, L., and Zhao, S. (2018). On the evolution process of the Beibu Gulf Basin and forming mechanism of local structures. Acta Geol. Sin. 92 (10), 2028–2039. doi:10.3969/j.issn.0001-5717.2018.10.005
Liu, L., Sun, Y., Chen, C., Lou, R., and Wang, Q. (2022). Fault reactivation in No.4 structural zone and its control on oil and gas accumulation in Nanpu sag, Bohai Bay Basin, China. Petroleum Explor. Dev. 49 (4), 824–836. doi:10.1016/s1876-3804(22)60313-6
Liu, N., Qiu, N., Chang, J., Shen, F., Wu, H., Lu, X., et al. (2017). Hydrocarbon migration and accumulation of the Suqiao buried-hill zone in Wen'an slope, Jizhong subbasin, Bohai Bay basin, China. Mar. Petroleum Geol. 86, 512–525. doi:10.1016/j.marpetgeo.2017.05.040
Luo, J., Morad, S., Liang, Z., and Zhu, Y. (2005). Controls on the quality of Archean metamorphic and Jurassic volcanic reservoir rocks from the Xinglongtai buried hill, western depression of Liaohe basin, China. AAPG Bull. 89 (10), 1319–1346. doi:10.1306/05230503113
Mazur, S., Ggaa, U., Kufrasa, M., and Krzywiec, P. (2018). Application of two-dimensional gravity models as input parameters to balanced cross-sections across the margin of the East European Craton in SE Poland. J. Struct. Geol. 116, 223–233. doi:10.1016/j.jsg.2018.05.013
Mazzullo, S. J., Wilhite, B. W., and Woolsey, I. W. (2009). Petroleum reservoirs within a spiculite-dominated depositional sequence: cowley formation (mississippian: lower carboniferous), south-central Kansas. AAPG Bull. 93 (12), 1649–1689. doi:10.1306/06220909026
P’an, C. H. (1982). Petroleum in basement rocks. AAPG Bull. 66 (10), 1597–1643. doi:10.1306/03b5a994-16d1-11d7-8645000102c1865d
Parnell, J., Baba, M., Bowden, S., and Muirhead, D. (2017). Subsurface biodegradation of crude oil in a fractured basement reservoir, Shropshire, UK. J. Geol. Soc. 174, 655–666. doi:10.1144/jgs2016-129
Plotnikova, I. N. (2006). Nonconventional hydrocarbon targets in the crystalline basement, and the problem of the recent replenishment of hydrocarbon reserves. J. Geochem. Explor. 89 (1-3), 335–338. doi:10.1016/j.gexplo.2005.12.012
Qin, C., Wang, H., Jiang, P., Yang, X., and Zou, K. (2020). Spacial distribution and evolution of axial deltaic system in continental rift basin:A case study of Weixinan sag. Beibuwan Basin 49 (3), 542–551. doi:10.13247/j.cnki.jcumt.001094
Rodríguez-Cuevas, M., Cardona, A., Monsalve, G., Zapata, S., and Valencia-Gómez, J. C. (2024). Fracture evaluation of the plutonic basement in the upper magdalena basin: implications for the development of naturally fractured reservoirs in the Northern Andes. Geol. J. 59, 1968–1997. doi:10.1002/gj.4980
Salah, M. G., and Alsharhan, A. S. (1998). The Precambrian basement: a major reservoir in the rifted basin, Gulf of Suez. J. Petroleum Sci. Eng. 19 (1998), 201–222. doi:10.1016/S0920-4105(97)00024-7
Timoshenko, S. P., and Woinowsky-Krieger, S. P. (1959). Theory of plates and shells. New York: McGraw-Hill.
Veizer, J., Ala, D., Azmy, K., Bruckschen, P., Buhl, D., Bruhn, F., et al. (1999). 87Sr/86Sr, δ13C and δ18O evolution of phanerozoic seawater. Chem. Geol. 161 (30), 59–88. doi:10.1016/S0009-2541(99)00081-9
Walters, R. F. (1953). Oil production from fractured pre-Cambrian basement rocks in central Kansas. AAPG Bull. 37 (2), 300–313. doi:10.1306/5ceadc59-16bb-11d7-8645000102c1865d
Wang, H., Jiang, Y., Yang, C., Zhang, B., Gu, Y., Luo, X., et al. (2023). Hydrothermal silicification in Ediacaran Dengying Formation fourth member deep dolomite reservoir, Central Sichuan Basin,China:Implications for reservoir quality. Geol. J. 2023, 4257–4270. doi:10.1002/gj.4757
Wang, Z., Jiang, Q., Wang, J., Long, G., Cheng, H., Shi, Y., et al. (2024). Hydrocarbon accumulation characteristics in basement reservoirsand exploration targets of deep basement reservoirs in onshore China. Petroleum Explor. Dev. 51 (1), 31–43. doi:10.1016/s1876-3804(24)60003-0
Wu, K., Li, Y., Fan, C., Dong, F., and Hong, M. (2022). Characteristics of unconformity structure and its implication for petroleum accumulation during fault-depression transition period in the wushi sag, beibuwan basin. Geotect. Metallogenia 46 (2), 272–281. doi:10.16539/j.ddgzyckx.2022.02.006
Xie, B., Xu, F. H., Fan, C. W., Man, Y., Jiang, F., Xu, G. S., et al. (2024). Karst identification and development model of buried hill limestone reservoir in Weixinan Depression, Beibuwan Basin. J. Jilin Univ. Earth Sci. Ed. 54 (6), 2029–2046. doi:10.13278/j.cnki.jjuese.20240223
Xing, Y. (1993). A study of carbonate paleo buried hill reservoir in Beibu Gulf W10-3N field. Petroleum Explor. Dev. 20 (6), 100–108. CNKI:SUN:SKYK.0.1993-06-014.
Xu, C., Yang, H., Wang, F., and Peng, J. (2024). Formation conditions of deep to ultra-deep composite buried-hill hydrocarbon reservoirs in Bohai Sea, China. Petroleum Explor. Dev. 51 (6), 1–13. doi:10.11698/PED.20230576
Yao, L., Wang, X. D., Lin, W., Li, Y., Kershaw, S., and Qie, W. K. (2016). Middle Viséan (Mississippian) coral biostrome in central Guizhou, southwestern China and its palaeoclimatological implications. Palaeogeogr. Palaeoclimatol. Palaeoecol. 448 (2016), 179–194. doi:10.1016/j.palaeo.2015.08.031
Ye, T., Chen, A., Niu, C., Wang, Q., and Hou, M. (2022). Characteristics, controlling factors and petroleum geologic significance of fractures in the archean crystalline basement rocks: a case study of the south jinzhou oilfield in liaodong bay depression, north China. J. Petroleum Sci. Eng. 208, 109504. doi:10.1016/j.petrol.2021.109504
Yi, J., Li, H., Shan, X., Hao, G., Yang, H., Wang, Q., et al. (2022). Division and identification of vertical reservoir units in archaeozoic metamorphic buried hill of bozhong sag, Bohai Bay Basin, east China. Petroleum Explor. Dev. 49 (6), 1282–1294. doi:10.1016/s1876-3804(23)60349-0
Zhang, Y. J., Wang, G. M., and Yin, Z. Y. (2024). Classification scheme of weathering crusts structures for aluminosilicate rich rocks. Acta Pet. Sin. 45 (9), 1372–1384. doi:10.7623/syxb202409005
Zhao, S., Yang, X., Chen, L., Zhou, G., Jiao, L., and Zhao, Y. (2019). Hydrocarbon accumulation conditions and exploration potential of carbonate buried hills in Weixinan sag in western South China Sea. China Offshore Oil Gas 31 (2), 51–61. doi:10.11935/j.issn.1673-1506.2019.02.006
Zhao, S., Zhao, Y., Yang, X., Jiao, L., and Chen, C. (2018). An analysis on the characteristics and main controlling factors of reservoir in carbonate buried hill in the Weixi'nan Sag, Beibuwan Basin. Haiyang Xuehao 40 (9), 43–53. doi:10.3969/j.issn.0253-4193.2018.09.004
Zhao, X., Jin, F., Wang, Q., and Bai, G. (2015). Buried-hill play, Jizhong subbasin, Bohai Bay basin: a review and future propespectivity. AAPG Bull. 99 (1), 1–26. doi:10.1306/07171413176
Zhou, Z. H., Wong, K. W., Xu, X. S., and Leung, A. Y. T. (2011). Natural vibration of circular and annular thin plates by Hamiltonian approach. J. Sound Vib. 330 (5), 1005–1017. doi:10.1016/j.jsv.2010.09.015
Zhu, Y., Yan, Z., Qin, K., Li, Z., Xiong, R., and Sun, K. (2024). Lithological and physical characteristics and their controlling factors of the Archaean metamorphic rocks: a case study of the Damintun Sag, in Liaohe depression of Bohai Bay Basin, China. Geoenergy Sci. Eng. 241 (2024), 213088. doi:10.1016/j.geoen.2024.213088
Keywords: paleozoic, buried-basement hill, tectonic evolution, controlling factor, Beibu Gulf Basin, Weixinan depression
Citation: Li A, Xu F, Fan C, Li M, Jiang F, Xu G, Xiong X, Zhang X and Xie B (2025) Formation process and controlling factors of tight buried-basement hill reservoirs:a case study of Weixinan Depression, Northern Beibu Gulf Basin, South China. Front. Earth Sci. 13:1552826. doi: 10.3389/feart.2025.1552826
Received: 29 December 2024; Accepted: 21 March 2025;
Published: 11 April 2025.
Edited by:
Hu Li, Sichuan University of Science and Engineering, ChinaReviewed by:
Binfeng Cao, Institute of Geology and Geophysics (CAS), ChinaYuqiang Jiang, Southwest Petroleum University, China
Copyright © 2025 Li, Xu, Fan, Li, Jiang, Xu, Xiong, Zhang and Xie. This is an open-access article distributed under the terms of the Creative Commons Attribution License (CC BY). The use, distribution or reproduction in other forums is permitted, provided the original author(s) and the copyright owner(s) are credited and that the original publication in this journal is cited, in accordance with accepted academic practice. No use, distribution or reproduction is permitted which does not comply with these terms.
*Correspondence: Fanghao Xu, eHVmYW5naGFvMTdAY2R1dC5lZHUuY24=