- 1 Peatland Ecology Research Group, Centre for Northern Studies and Plant Sciences Department, Université Laval, Québec, QC, Canada
- 2 Bangor Wetlands Group, Wolfson Carbon Capture Laboratories, School of Environmental and Natural Science, Bangor University, Bangor, United Kingdom
- 3 School of Civil and Environmental Engineering, Yonsei University, Seoul, Republic of Korea
Phenolic compounds have been proposed to influence decomposition by inhibiting extracellular enzyme activities, as described in the enzymic latch mechanism (ELM). This study examined the effects of phenolic treatments on Sphagnum decomposition, productivity, and biomass accumulation within a Sphagnum farming system. A split-plot experiment with three phenolic treatments was implemented in two cultivation basins established with mosses dominated by the Acutifolia or Sphagnum subgenus. Phenolic treatments were wood pellets (wood), old roots from peat harrowing (root), and no addition (control). Phenolic additions did not result in a measurable reduction in decomposition rates nor was Sphagnum productivity or biomass affected by the experimental treatments. Both subgenera functioned as approximately similar small carbon dioxide (CO2) sinks, with values such as −2 ± 1 g CO2 m−2 d−1 (Acutifolia) and −0.2 ± 0.8 g CO2 m−2 d−1 (Sphagnum). Phenolic additions in both subgenera resulted in higher CO2 values as net ecosystem exchange compared to the control, which could be linked to emissions resulting from wood and root decomposition. In both subgenera, phenolic additions neither increased peat phenolic concentrations nor inhibited enzyme activities compared to the control. The current study did not validate the potential of phenolics in limiting decomposition as theorized in the ELM. The short duration of the experiment may have restricted the effect of phenolic products applied at the surface from reaching the ∼10 cm depth where peat was sampled. This could explain the absence of an inhibitory effect of phenolic products on enzyme activities. Therefore, it is recommended to conduct various sample analyses at different depths to better understand phenolic–enzyme interactions in a Sphagnum farming system.
1 Introduction
Sphagnum peat is important for growing substrates due to its low cost, easy availability, and unique characteristics for plant growth (Caron and Rochefort, 2013). However, the negative impact of peat extraction on peatland ecosystem services, such as carbon dioxide (CO2) sequestration, water regulation, and biodiversity, has increased attention on sustainable alternatives to minimize pressure on peatlands. To reduce the negative impact of peat extraction, a sustainable alternative for post-extracted peatlands is paludiculture, which involves the production of agricultural or forestry crops on rewetted peatlands (Wichtmann et al., 2016; Gaudig et al., 2017). The cultivation of Sphagnum biomass on a cyclical and renewable basis on rewetted peatlands is specifically termed Sphagnum farming, which is also a form of paludiculture (Pouliot et al., 2015; Gaudig et al., 2017). The production of undecomposed Sphagnum biomass has multiple end uses, primarily as a donor material for restoration and an alternative material for growing media (Emmel, 2008; Reinikainen et al., 2012; Jobin et al., 2014; Müller and Glatzel, 2021). Furthermore, Sphagnum farming can partially restore ecosystem services similar to pristine peatlands, due to its limited decomposition, enhanced CO2 sequestration, regulation of water and nutrients, and provision of habitats for a wide variety of biodiversity (Joosten et al., 2012; Luthardt and Wichmann, 2016).
Experiments involving Sphagnum cultivation are currently underway in degraded and cutover peatlands in various countries, including Canada, Germany, Finland, Chile, Denmark, Lithuania, Latvia, Ireland, the Netherlands, South Korea, Japan, and New Zealand. Several factors to optimize the Sphagnum yield have been tested, such as species selection based on decomposition and productivity comparison, regulation of external water supply, straw cover, and management of unwanted plant species (Guêné-Nanchen et al., 2017; Gaudig et al., 2017; Brown et al., 2017; Kim et al., 2021).
In general, biotic (enzymes and microorganisms) and abiotic (annual temperature, precipitation/water table, and pH) factors influence Sphagnum growth and decay dynamics (Coulson and Butterfield, 1978; Moore, 1989; Asada et al., 2003; Rydin and Jeglum, 2013). Manipulation of biotic and abiotic factors could be beneficial in enhancing the Sphagnum yield and limiting decomposition in a farming system. In the literature, the inhibition of extracellular enzymes and microbial activities by phenolics has been investigated as a means to limit decomposition, a process known as the enzymic latch mechanism (ELM) (Freeman et al., 2001). Essentially, the ELM is a group of constraints that exist in a cyclical process. In brief, oxygen limitation impedes phenol oxidase (POX) activity, which favors the accumulation of phenolic compounds. The accumulated phenolics work as potent inhibitors and restrict hydrolase enzyme activities. All the above-mentioned constraints lead to restricted decomposition based on the ELM (Freeman et al., 2001; Freeman et al., 2012). Considering the ELM, external phenolic supplementation could increase phenolic concentrations, thus strengthening the process by further putting constraints on enzyme activities, leading to limited decomposition. Increasing phenolic concentration might have an indirect positive effect on Sphagnum yield as phenolics play an important role in photosynthetic activity, hormone regulation, and protection from pathogens (Dixon, 2001; Wallis and Galarneau, 2020). Based on the ELM assumption, the phenolic potential to limit decomposition and enhance Sphagnum yield in Sphagnum farming would be highly relevant for restoring ecosystem services.
Over the last couple of decades, the role of phenolic additions in limiting decomposition based on the ELM has been investigated, but it remains controversial. Certain studies have shown no effect of phenolics on limiting decomposition (Harris et al., 2020; Urbanová and Hájek, 2021), while others have shown the role of phenolics in limiting enzyme activities, leading to limited decomposition (Freeman et al., 2001; Dunn et al., 2014; Pinsonneault et al., 2016; Kim et al., 2021; Alshehri et al., 2020). In addition, ELM and its strengthening through ecological engineering tools in the form of phenolic additions have not been fully explored in the context of Sphagnum cultivation.
Sphagnum species are rich in phenols, like Sphagnum acids, which act as a potent inhibition compound against enzymes (Wetzel, 1992; van Breemen, 1995; Freeman et al., 2001). Based on the ELM, external phenolic enrichment could be useful to further restrict the de novo synthesis of extracellular enzymes. Limited enzyme activities would deprive microbes of the substrates needed for growth, while a direct effect on microbial metabolism could decrease the de novo synthesis of extracellular enzymes (Fenner and Freeman, 2020). The sources of phenolic additions can be natural or commercial products. Natural products may include roots, wood chips, bark, and other wood by-products. Commercial products may include gallic acid, cinnamic acid, tannic acid, lignosulphonate acid, primary sludge, and secondary sludge from the paper and pulp industry (Dunn et al., 2014; Alshehri et al., 2020; Urbanová and Hájek, 2021). The amount of phenolic addition, along with the intrinsic biochemical structural properties of Sphagnum species, could make them more or less resistant to decomposition. For instance, Pipes and Yavitt (2022) showed that species with higher phenolic content tend to decay more slowly than those with lower phenolic content.
The three taxonomic subgenera of Sphagnum (Acutifolia, Sphagnum, and Cuspidata) can have differences in attributes like stem density, productivity, and decay patterns (Rochefort et al., 1990; Johnson and Damman, 1991). Supplementary Table 1 provides a brief comparison of the Acutifolia, Sphagnum, and Cuspidata subgenera based on various attributes. Species selection in Sphagnum farming depends on the end use of the biomass. For example, moss species from the Acutifolia and Sphagnum subgenera could be selected if the farming goal is to produce raw materials for horticultural growing substrates. Various plant cultivation experiments showed that several species of the Acutifolia and Sphagnum subgenera proved to be suitable components for growing substrates compared to species from the Cuspidata subgenus (Gaudig et al., 2014). If, however, the aim is to harvest Sphagnum diaspores for peatland restoration, then species from the Acutifolia and Sphagnum subgenera should be selected for Sphagnum farming as they are more tolerant to desiccation and produce better quality products.
This study aims to evaluate the impact of surface phenolic additions on limiting Sphagnum decomposition at the base of the acrotelm, enhancing Sphagnum productivity, and increasing CO2 uptake based on the ELM concept in the Sphagnum farming system. According to our objective, we aim to answer the following questions: 1) is there a difference in CO2 exchange between cultivated mosses from the Acutifolia and Sphagnum subgenera? 2) Do phenolic additions play a role in regulating CO2 exchange, peat phenolic concentrations, and enzyme activities? 3) Are phenolic additions an essential tool for optimizing the productivity and biomass of mosses from the Acutifolia and Sphagnum subgenera? In general, for the Acutifolia and Sphagnum subgenera, we anticipated the following: 1) phenolic additions would enhance peat phenolic concentration and limit enzyme activities; and 2) higher peat phenolic concentration, along with limited enzyme activities, would lead to lower CO2 emissions and higher CO2 uptake. Due to the limited scope, we did not intend to test the ELM; however, the study’s questions and hypothesis would be evaluated in the context of the ELM hypothesis.
2 Methods
2.1 Study site
This study was conducted in a Sphagnum farming site located approximately 13 km southeast of Rivière-du-Loup in Eastern Canada (47°49′N and 69°27′W). The Sphagnum farm covers an area of approximately 1 ha, established on a natural peatland area located beside a cutover bog. In 2013, six basins of 50 m × 10 m were built. Among the basins, three basins had a central irrigation canal, while the others had a peripheral irrigation canal. Water in the irrigation canals was supplied from a nearby main drainage canal and maintained between −5 cm and 0 cm from the Sphagnum surface using automatic sensor systems. Moss species from the Acutifolia and Sphagnum subgenera were reintroduced for cultivation using an adapted version of the Moss Layer Transfer Technique, which is widely and specifically used for the ecological restoration of post-extracted peatlands in North America (Rochefort et al., 2003). Gutierrez Pacheco et al. (2021) provided a complete description of the study site area, and Guêné-Nanchen and St-Hilaire (2022) provided more details on general Sphagnum cultivation, Sphagnum farm design, and construction.
2.2 Experimental design
To investigate the effect of phenolic additions in a Sphagnum farming system, two basins having central irrigation canals and dominant moss carpets of Acutifolia (Sphagnum rubellum) and Sphagnum (S. medium and S. papillosum) subgenera were selected in June 2021. A split block design was adopted with basins as the blocking factor, subgenus as the main plot factor (replicated four times across two basins), and phenolic supplements as the subplot factor. For phenolic treatments, two locally available products were chosen with different concentrations of soluble phenolics, namely wood pellets (1.2 mg g−1 phenolics) and old roots from harrowing before peat extraction (0.2 mg g−1 phenolics). The wood pellets (hereafter referred to as wood) are sourced from Granulco 100% natural softwood pellets, made from species of the Picea and Abies genera. Each pellet was approximately 40 mm in length and 6 mm in diameter. For root treatment, old roots (hereafter referred to as root) were chipped using a commercial chipping machine. The resulting root chips were fibrous, derived from Picea mariana, with sizes of 0.3 × 0.1 ± 0.05 mm. At both basins, phenolic treatments were randomly applied at 2 kg m−2 (fresh weight dosage) on top of the Acutifolia and Sphagnum subgenera plots of 4 m × 4 m sizes. In addition to phenolic additions, a control plot was also established for both subgenera for comparison within treatments. In the current study design, a total of 24 experimental units, two (subgenus) × four (subgenus replications) × three (phenolic treatments), were constructed (Supplementary Figure 1).
2.3 Samplings
2.3.1 Carbon dioxide exchange
In the middle of each experimental unit, 60 cm × 60 cm × 20 cm dimensions of stainless-steel collars having grooves on top were inserted into the Sphagnum carpet for carbon dioxide (CO2) sampling. To avoid disturbance during CO2 sampling, boardwalks and platforms were installed at each experimental unit. After approximately 1 year of phenolic addition, CO2 exchange (g CO2 m−2d−1) was measured from May to August/September 2022 using the closed chamber method (Alm et al., 1997). The net ecosystem exchange (NEE) of CO2 (g CO2 m−2d−1) was determined using a clear acrylic chamber (60 cm × 60 cm × 30 cm) connected with a portable infrared analyzer (IRGA; EGM-4 PP Systems, United States). The clear acrylic chamber was equipped with two fans (10 cm × 10 cm) operated with batteries, holes for gas exchange, and thermocouple wire. Prior to each measurement, any vascular vegetation was clipped to meet the criteria of this study. Finally, the chamber was placed in the collar groove, which was filled with water to prevent any gas exchange other than between the chamber and IRGA. The CO2 exchange inside the chamber was measured for 0–2 min with data recording at 15-s intervals. At the same time, photosynthetically active radiation, temperature inside the chamber, and relative humidity inside the chamber were also recorded. Ecosystem respiration (ER; g CO2 m−2d−1) was measured as a proxy for decomposition by covering the chamber with an opaque shroud. Gross ecosystem photosynthesis (GEP) was calculated as the difference between NEE and ER. Before and during each measurement, the chamber was lifted from the collar to allow headspace air equal to ambient CO2 concentration and temperature. The linear change in CO2 concentration over time was used to calculate NEE and ER. Fluxes were rejected in case of a non-linear trend in CO2 concentration (R 2 < 0.80). However, a flux with a constant CO2 concentration or a change of less than 2 ppm over time was retained, indicating an NEE close to 0. The conventional sign method was used, where negative values indicated CO2 uptake by the ecosystem from the atmosphere and positive values indicated CO2 emissions from the ecosystem to the atmosphere. To better understand NEE (NEE = GEP + ER), in brief, a negative NEE value indicates that CO2 captured (GEP, photosynthesis) by the Sphagnum species is higher than the CO2 release (respiration), while a positive NEE value indicates the opposite. At the end of the experiment, within each collar, several random height measurements were taken from the Sphagnum surface to the top of the collar for chamber volume correction. For statistical analysis, a minimum of five readings were required throughout the growing season from each experimental unit.
2.3.2 Sphagnum productivity and biomass
In the context of Sphagnum farming, it is important to understand the difference between acrotelm and catotelm layers to minimize the human-based error in the measurements. The acrotelm layer refers to the newly formed Sphagnum layer, while catotelm refers to the residual layer of peat.
At both basins, Sphagnum productivity (P; g m2 yr−1) was determined using the following equation:
where AI represents the Sphagnum mean annual increment (cm), D represents the density of the Sphagnum stem (stem m−2), W represents the dry weight of 1 cm of Sphagnum shoot (g cm−1 stem−1), and C represents the Sphagnum cover (%). Sphagnum mean annual increment (AI, cm) was measured with brush wires (Gunnarsson and Rydin, 2000) installed in each experimental unit in May 2022. A total of 360 brush wires, five (brush wires) × three (cluster) × two (subgenus) × three (phenolic treatments) × four (phenolic treatment replications), were installed in 24 experimental units. The height of the brush wire was noted twice, once at the beginning (initial height, in May) and again at the end of the season (final height, in October). Sphagnum height increment was calculated as the difference between the initial and final heights. At the end of the season, three Sphagnum biomass samples, each from an area of 35 cm2, were collected close to the three brush wire clusters. Samples were used to count the number of Sphagnum capitulum for estimating the density of Sphagnum stem (D, stem m−2). From the same sample, the top 3 cm of 40 Sphagnum stems without capitulum were oven-dried and weighed. To estimate the dry weight of 1 cm of Sphagnum shoot (W, g cm−1 stem−1), the oven dry weight was divided by three. Sphagnum cover (C, %) would be equal to 1 as our carpets had 100% Sphagnum cover.
At each experimental unit, a total of three randomly spaced Sphagnum biomass (g m−2) samples were collected using 25 cm × 25 cm quadrats in October 2022. Within each quadrat, a Sphagnum biomass sample was extracted by cutting the Sphagnum carpet with a serrated knife from the top surface of the Sphagnum down to the top surface of the catotelm. The samples were sorted to conserve only Sphagnum biomass, oven-dried at 70°C for 72 h, and weighed for biomass dry weight.
2.3.3 Environmental conditions
After each CO2 exchange measurement, the water table level was measured manually in a PVC well (2-inch diameter). Near the collar, temperatures from the Sphagnum carpet surface to a depth of −2 cm, −5 cm, −10 cm, −15 cm, and −20 cm were monitored using a thermocouple probe (Digi-Sense, Cole-Parmer) connected with a digital temperature reader (Omega HH200).
At the end of the growing season, five to six handfuls (∼200 cm3 per handful) of randomly spaced fibrous peat samples from the bottom of the acrotelm layer, approximately 1–2 cm above the catotelm layer, were collected from each experimental unit. The average depth of peat sampling was approximately 10 cm from the top of the Sphagnum carpet surface. Subsequently, all peat samples were mixed to make a composite sample. During peat sampling, soil temperature for enzyme analysis was also recorded using thermocouple probes (Digi-Sense, Cole-Parmer) connected with a digital temperature reader (Omega HH200). During sampling, instruments and hands were rinsed with 70% isopropyl alcohol to avoid contamination. For pH and electrical conductivity (EC; µS/cm), samples were stored at −20°C until further processing. Later, samples were thawed in the laboratory and saturated with deionized water (Soil Medium Extract method) to measure peat pH and EC (AB200 pH and EC meter; Fisher Scientific, Hampton, New Hampshire, United States). The measurements were corrected for temperature and pH using the formula provided by Sjörs (1950). Samples for enzyme and soluble phenolic analysis were stored at 4°C. Enzyme analyses, such as hydrolase (β-D-glucosidase, arylsulfatase, N-acetyl-β-D-glucosaminidase, β-D-xylosidase, and phosphatase) and phenol oxidase, were measured at Bangor University using the methods explained by Dunn et al. (2014) within 2 weeks of sampling. The protocol for enzyme analysis is also provided in the Supplementary Material. Using the method described by Alshehri et al. (2020), peat-soluble phenolics were measured following the water extraction method.
2.4 Statistical analyses
All statistical analyses were conducted in R software (R Core Team, 2023) and reported with a significance level of 0.05. For our factorial experiment, the data were processed with a linear mixed-effect (LME) model in the lme4 package (Bates et al., 2015). For all the response variables, LME models were formulated with subgenus, phenolic treatments, and their interactions as fixed factors. Random effects need special attention in the presence or absence of repeated measurements and to properly calculate error terms. For example, NEE data were collected several times from the same sampling unit; therefore, its LME model included random effects as (1|Basin) + (1|Basin: Subgenus) + (1|Replicate: Basin: Subgenus) (1|Phenolic: treatments: Replicate: Basin: Subgenus). On the other side, pH data did not have pseudo-replications, so random effects in the LME model were as follows: (1|Basin) + (1|Basin: Subgenus) + (1|Replicate: Basin: Subgenus). All the models were visually inspected for normality and homogeneity of residuals. For analyses of variances, joint_tests in the emmeans package were used for all models (Lenth, 2023). Tukey multiple comparisons in emmeans and compact letter display (CLD) function in multcomp (Hothorn et al., 2008) packages were used to identify differences in significant main effects. For models with significant interaction effects, the joint_tests function with the by argument, followed by Tukey multiple comparisons and the CLD function, was used to detect differences among the different levels. To estimate the correlation between response variables, the cor command was used. All the graphics were produced using the ggplot2 package (Wickham, 2016).
3 Results
3.1 Carbon dioxide exchange
Broadly, Acutifolia and Sphagnum subgenera acted as CO2 sinks. The average NEE values between mosses of Acutifolia (−2 ± 1 g CO2 m−2 d−1) and Sphagnum subgenera (−0.2 ± 0.8 g CO2 m−2 d−1) were not different (Table 1). Among phenolic treatments, the wood treatment (2 ± 0.6 g CO2 m−2 d−1) resulted in greater positive mean values of NEE than the root (−1 ± 1 g CO2 m−2 d−1) and control (−4 ± 0.5 g CO2 m−2 d−1) treatments (Table 1; Figure 1A). For GEP, the wood treatment augmented the mean GEP values for the mosses of the Acutifolia subgenus, whereas root and control treatments did not (significant interaction Table 1; Figure 1B). For the mosses of the Sphagnum subgenus, mean GEP values among phenolic treatments were not different (Figure 1B). The mean ER was similar between mosses of Acutifolia (10 ± 1 g CO2 m−2 d−1) and Sphagnum (9 ± 1 g CO2 m−2 d−1) subgenera and did not vary among phenolic treatments (Table 1; Figure 1C).
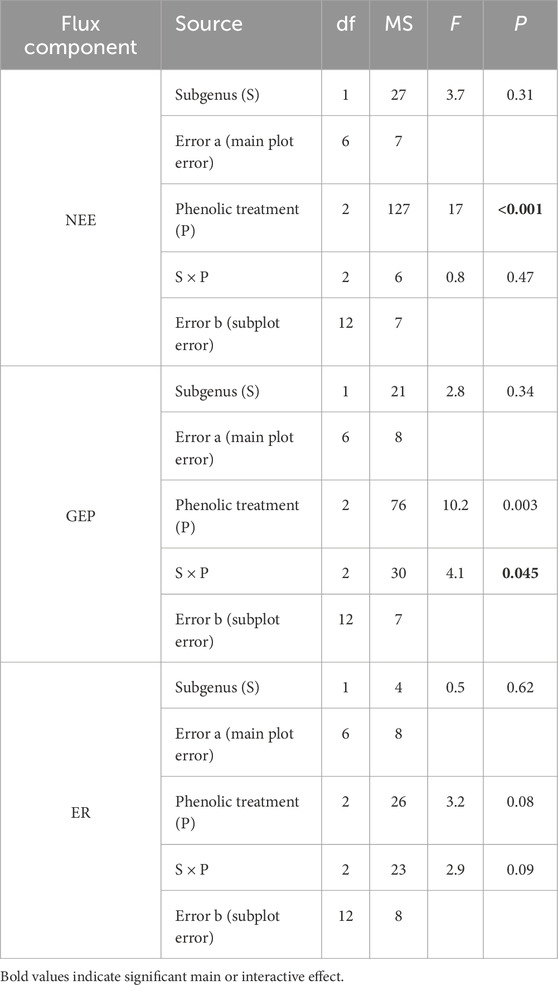
Table 1. Linear mixed-effect model to determine the effects of subgenus (Acutifolia and Sphagnum) and phenolic treatments (control = no addition, root = old roots from peat harrowing, and wood = wood pellets) on net ecosystem exchange (NEE; g CO2 m−2d−1), gross ecosystem photosynthesis (GEP; g CO2 m−2d−1), and ecosystem respiration (ER; g CO2 m−2d−1).
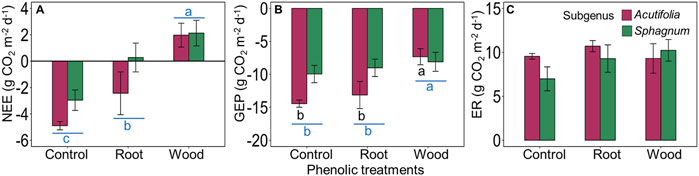
Figure 1. Effects of external phenolic additions (control = no addition, root = old roots from peat harrowing, and wood = wood pellets) on mean net ecosystem exchange [(A), NEE; g CO2 m−2d−1, n = 4], mean gross ecosystem photosynthesis [(B), GEP; g CO2 m−2d−1, n = 4], and mean ecosystem respiration [(C), ER; g CO2 m−2d−1, n = 4] in the Acutifolia and Sphagnum subgenera plots. Negative values indicate CO2 uptake by the ecosystem from the atmosphere, and positive values indicate a release of CO2 from the ecosystem to the atmosphere. Error bars represent the standard error of the mean. The presence of different blue lowercase letters indicates significant differences among phenolic treatments based on the significant main effect of phenolic treatments, whereas the presence of different black lowercase letters indicates significant differences among phenolic treatments for each subgenus level separately based on the significant two-way interactive effect of subgenus and phenolic treatments on observed variables (p < 0.05, Tukey’s HSD). See Table 1 for detailed statistical analysis.
3.2 Peat-soluble phenolics
The mean peat-soluble phenolic content was not found to be different between both subgenera and independent of the phenolic addition treatments (Table 2; Figure 2).
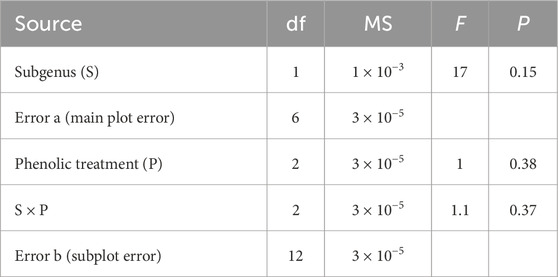
Table 2. Linear mixed-effect model to determine the effects of subgenus (Acutifolia and Sphagnum) and phenolic treatments (control = no addition, root = old roots from peat harrowing, and wood = wood pellets) on peat-soluble phenolics (mg g−1).
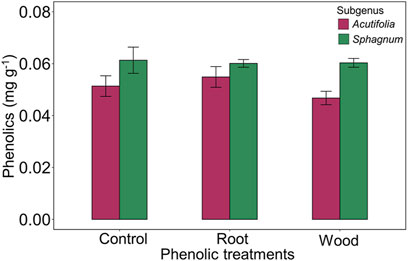
Figure 2. Effects of external phenolic additions (control = no addition, root = old roots from peat harrowing, and wood = wood pellets) on mean peat-soluble phenolics (mg g−1, n = 4) in the Acutifolia and Sphagnum subgenera plots. Error bars represent the standard error of the mean. See Tables 2 for detailed statistical analysis.
3.3 Enzyme activities
3.3.1 Hydrolase activities
Among hydrolase enzymes, the interaction effect of subgenus and phenolic treatments on arylsulfatase activities was significant (Table 3). This interaction effect resulted from the difference in phenolic treatments between the Acutifolia and Sphagnum subgenera. For the Acutifolia subgenus, mean arylsulfatase activities were higher in wood (0.2 ± 0.08 nmol g−1 min−1) treatment than in the control (0.08 ± 0.02 nmol g−1 min−1) and root (0.08 ± 0.005 nmol g−1 min−1) treatments (Figure 3B). The opposite was observed in the Sphagnum subgenus, where the root (0.3 ± 0.1 nmol g−1 min−1) treatment showed greater mean arylsulfatase activities than the wood (0.2 ± 0.1 nmol g−1 min−1) treatment, while the control (0.2 ± 0.02 nmol g−1 min−1) had similar mean arylsulfatase activities compared to both root and wood treatments (Figure 3B). Similarly, the interaction effects of subgenus and phenolic treatment on N-acetyl-β-D-glucosaminidase were significant (Table 3). This interaction effect was produced by the difference among phenolic treatments in the Acutifolia subgenus, where the wood (6 ± 1 nmol g−1 min−1) treatment resulted in more mean N-acetyl-β-D-glucosaminidase activities than the control (4 ± 0.3 nmol g−1 min−1) and the root (3 ± 0.01 nmol g−1 min−1, Figure 3D) treatments. However, no difference in mean N-acetyl-β-D-glucosaminidase activities among phenolic treatments was observed for the Sphagnum subgenus (Figure 3D). In the case of mean phosphatase activities, the wood (56 ± 9 nmol g−1 min−1) treatment showed higher values than the control (43 ± 2 nmol g−1 min−1) and root (26 ± 3 nmol g−1 min−1) treatments (Table 3; Figure 3E). β-D-glucosidase and β-D-xylosidase activities were not different between mosses from the Acutifolia and Sphagnum subgenera or among the phenolic addition treatments (Table 3; Figures 3A–C).
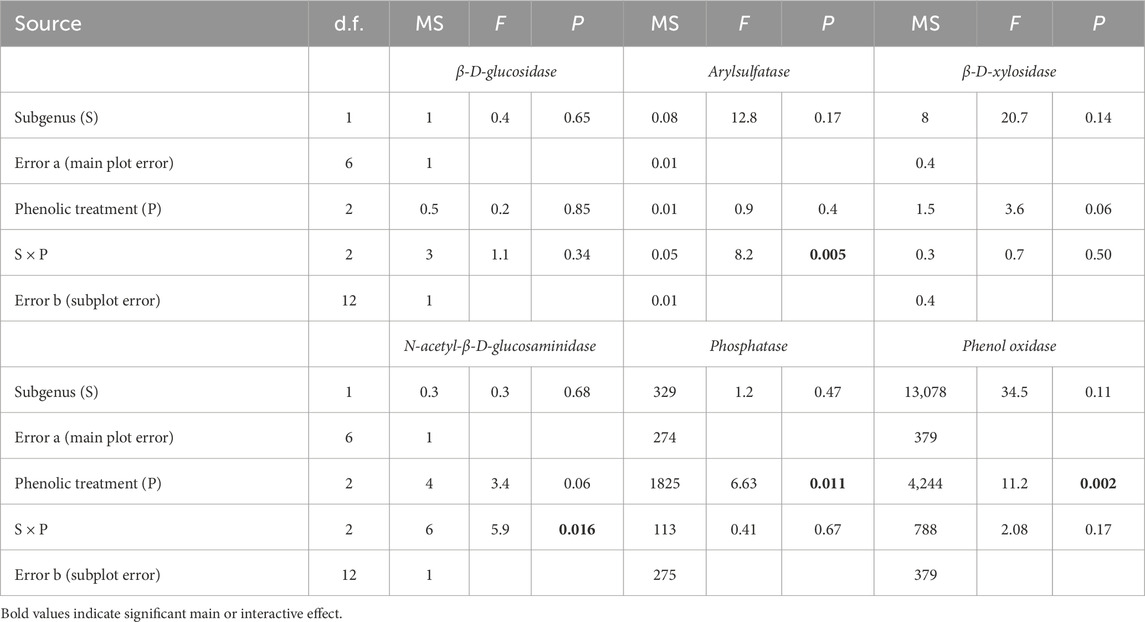
Table 3. Linear mixed-effect model to determine the effects of subgenus (Acutifolia and Sphagnum) and phenolic treatments (control = no addition, root = old roots from peat harrowing, and wood = wood pellets) on average hydrolase (nmol g−1 min−1, β-D-glucosidase, arylsulfatase, β-D-xylosidase, N-acetyl-β-D-glucosaminidase, and phosphatase) and phenol oxidase (nmol diqc g−1 min−1) activities.
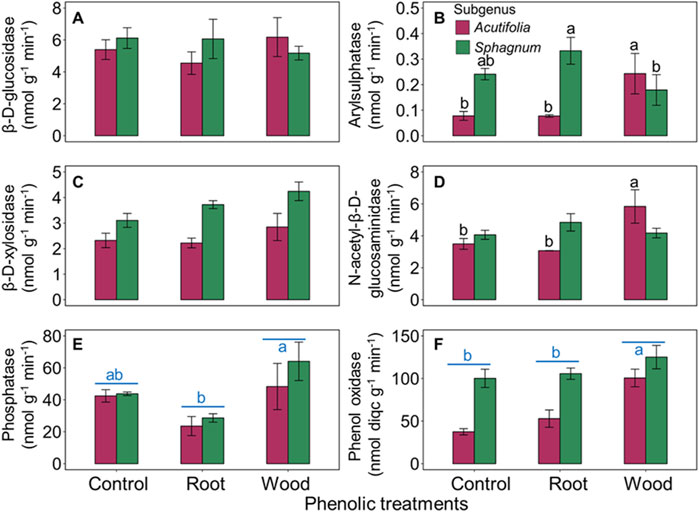
Figure 3. Effects of external phenolic additions (control = no addition, root = old roots from peat harrowing, and wood = wood pellets) on mean hydrolase [(A–E), nmol g−1 min−1, n = 4] and phenol oxidase [(F), nmol diqc g−1 min−1, n = 4] enzyme activities in the Acutifolia and Sphagnum subgenera plots. Error bars represent the standard error of the mean. The presence of different blue lowercase letters indicates significant differences among phenolic treatments based on the significant main effect of phenolic treatments. The presence of different black lowercase letters indicates significant differences among phenolic treatments for each subgenus level separately based on the significant two-way interactive effect of subgenus and phenolic treatments on observed variables (p < 0.05, Tukey’s HSD). See Table 3 for detailed statistical analysis.
3.3.2 Phenol oxidase activities
The mean phenol oxidase (POX) activities were similar between Acutifolia (64 ± 9 nmol diqc g−1 min−1) and Sphagnum (110 ± 6 nmol diqc g−1 min−1) subgenera (Table 3). Among phenolic treatments, the wood (113 ± 9 nmol diqc g−1 min−1) treatment resulted in greater mean POX activities than the root (79 ± 12 nmol diqc g−1 min−1) and control (69 ± 13 nmol diqc g−1 min−1) treatments, and the latter two treatments had similar mean POX activities (Table 3; Figure 3F).
3.4 Sphagnum productivity and biomass
The mean Sphagnum productivity between the Acutifolia (378 ± 39 g m−2 yr−1) and Sphagnum (363 ± 32 g m−2 yr−1) subgenera was similar (Table 4). Similarly, the mean values of Sphagnum biomass were not different between the Acutifolia (998 ± 87 g m−2) and Sphagnum (809 ± 83 g m−2) subgenera (Table 4). Productivity and biomass were also similar among phenolic treatments (Table 4; Figure 4).
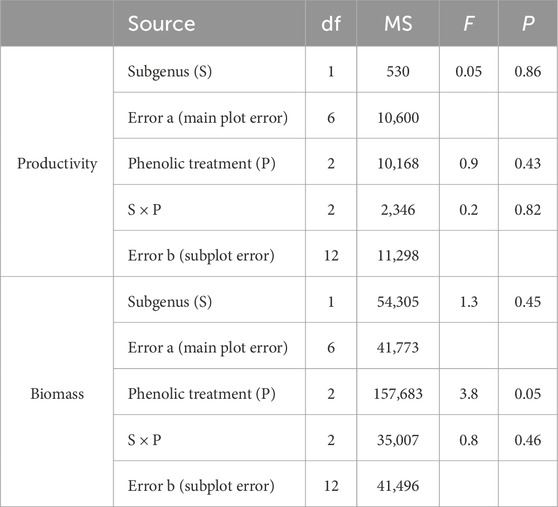
Table 4. Linear mixed-effect model to determine the effects of subgenus (Acutifolia & Sphagnum) and phenolic treatments (control = no addition, root = old roots from peat harrowing, and wood = wood pellets) on Sphagnum productivity (g m−2 yr−1) and biomass (g m−2).
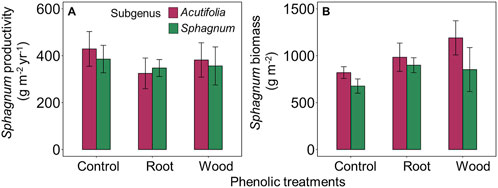
Figure 4. Effects of external phenolic additions (control = no addition, root = old roots from peat harrowing, and wood = wood pellets) on mean Sphagnum productivity [(A), g m−2 yr−1, n = 4] and mean Sphagnum biomass [(B), g m−2, n = 4] in the Acutifolia and Sphagnum subgenera plots. Error bars represent the standard error of the mean. See Tables 4 for detailed statistical analysis.
3.5 Relationship between CO2 exchange, enzyme activities, and environmental variables
Overall, the Acutifolia and Sphagnum subgenera did not show a strikingly different pattern in response to phenolic additions. In the Acutifolia subgenus, NEE was positively correlated with arylsulfatase (r = 0.7, p = 0.02), β-D-xylosidase (r = 0.6, p = 0.03), and N-acetyl-β-D-glucosaminidase (r = 0.6, p = 0.03) activities (Figure 5A; Supplementary Figure 2A). Similarly, β-D-xylosidase activities at the Sphagnum subgenus also exhibited a positive correlation with NEE (Figure 5A, r = 0.9, p < 0.001). In the Acutifolia subgenus, POX activities showed a positive correlation with NEE (Figure 5B, r = 0.9, p < 0.001), whereas POX activities in the Sphagnum subgenus were not correlated with either NEE or ER (Figure 5B; Supplementary Figure 2B, p > 0.05). For both subgenera, pH was negatively correlated with peat-soluble phenolics (Supplementary Figure 2). However, peat-soluble phenolics were not correlated with NEE, ER, POX, or hydrolase enzyme activities (Supplementary Figure 2, p > 0.05).
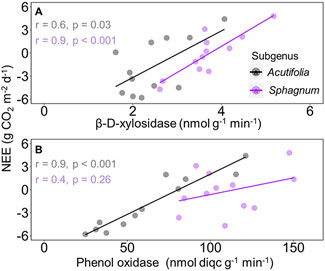
Figure 5. Pearson correlation coefficient of β-D-xylosidase [(A), nmol g−1 min−1] and phenol oxidase [(B), nmol diqc g−1 min−1] activities with net ecosystem exchange (NEE, g CO2 m−2d−1) at Acutifolia and Sphagnum subgenus levels. For NEE, negative values indicate CO2 uptake by the ecosystem from the atmosphere, and positive values indicate a release of CO2 from the ecosystem to the atmosphere. P < 0.05 represents a significant correlation between variables.
4 Discussion
Decomposition in peatlands is a very complex mechanism. Inhibiting extracellular enzymes with phenolic additions could be a low-cost, effective method to limit CO2 emissions based on the ELM hypothesis. The literature is limited in understanding the potential of phenolics in limiting decomposition, enhancing Sphagnum productivity, and increasing biomass in the Sphagnum farming system.
The first question addressed in this paper investigated the difference in CO2 exchange between cultivated mosses from the Acutifolia and Sphagnum subgenera as CO2 fluxes of cultivated mosses in a Sphagnum farming system remain unclear. Our results indicate that both subgenera, Acutifolia and Sphagnum, despite stable water table levels, exhibited identical results of ecosystem respiration, which was used as a proxy for decomposition. Likewise, NEE values were also similar between Acutifolia (−2 ± 1 g CO2 m−2 d−1) and Sphagnum (−0.2 ± 0.8 g CO2 m−2 d−1) subgenera, representing a small sink of CO2. In support of our results, Günther et al. (2017) estimated CO2 exchange in a Sphagnum farming system in Germany for two growing seasons during the establishment phase and found no difference in the NEE values between S. papillosum (−15 ± 1 g CO2 m−2 d−1) and S. palustre (−15 ± 1 g CO2 m−2 d−1). The higher NEE values (more CO2 release than CO2 uptake) observed in the current study, compared to those reported by Günther et al. (2017), could be attributed to differences in the length of the growing season between Germany and Canada. In Germany, Sphagnum growth is favored due to longer and more suitable weather conditions, whereas in Canada, shorter growing seasons followed by snow prevent the growth of Sphagnum. Within the scope of the first question, it was clear that mosses from the Acutifolia and Sphagnum subgenera did not show prominent differences in terms of CO2 exchange.
The second question in this study evaluated the role of externally added phenolics in understanding the decomposition of mosses from the Acutifolia and Sphagnum subgenera. The results from this study indicated that phenolic treatments did not enhance peat-soluble phenolics, limit extracellular enzyme activities, or reduce ecosystem respiration compared to the control for the tested Sphagnum species. The NEE outcome showed that between the two phenolic treatments, Sphagnum species in wood-treated subplots served as a CO2 source, while those in the root-treated subplots served as a CO2 sink. The decomposition of wood pellets might have contributed to higher NEE values (more CO2 release than CO2 uptake) than root additions. This suggests that wood, as an external phenolic supplement, is not a suitable cost-effective method for reducing emissions in short-duration experiments (one to two growing seasons), despite having a higher soluble phenolic content (1.2 mg g−1) than the root product (0.2 mg g−1). Alshehri et al. (2020) conducted a phenolic addition experiment at a greenhouse scale where wood chips of Larix laricina, P. mariana, and Thuja occidentalis were used as phenolic additions. Wood chips were added using two methods, namely, surface addition and mixed treatment (wood chips mixed within the top 10 cm of the peat surface). Consistent with our results, Alshehri et al. (2020) did not find statistically significant differences in peat phenolics with surface addition treatments. However, differences in peat phenolics were found in the mixed treatment, excluding L. laricina, compared to the control. Surface addition also did not show any difference in enzyme activities, but the mixed treatment for P. mariana showed lower enzyme activity than the control. For CO2 exchange, L. laricina and P. mariana under surface addition acted as CO2 sinks. Such results indicate the complex nature of the decomposition process, which could vary based on the method of phenolic addition and micro-site conditions. For a better understanding of the decomposition process, it is important to conduct hit-and-trial laboratory-based or greenhouse experiments with phenolic additions ranging from surface application to different depths within the Sphagnum carpet. Another experiment conducted in a controlled microcosm exhibited that wood insertion in peat (Quercus robur and Liriodendron tulipifera wood pieces of 2 cm3 were inserted 5 cm below the peat surface under anaerobic conditions) enhanced polyphenols, which reduced extracellular enzyme activities and acted as a quadruple lock against decomposition (Fenner and Freeman, 2020). In the present study, the absence of a detectable effect of phenolic addition on peat-soluble phenolics and extracellular enzyme activities could be attributed to the possibility that recalcitrant compounds from the phenolic products applied at the surface did not reach the base of the acrotelm, where peat sampling was conducted.
To some extent, short-term field experiments do not support the role of phenolics in limiting extracellular enzyme activities. For example, Urbanová and Hájek (2021), in a laboratory-based study, found that added phenolics did not exhibit extracellular enzyme activities and indicated that phenolics could be a source of labile carbon. The researchers observed that oxidative enzyme activities were similar in both oxic and anoxic environments. Other studies investigated different aspects of biogeochemical processes that could help understand enzyme activity response. For instance, Wang et al. (2017) examined the role of iron (Fe) in a field-based mesocosm and found that a lower water table inhibited extracellular enzyme activities. The lower water table resulted in Fe oxidation, which increased Fe-protected phenolics and acted as an iron gate against the opening of the ELM. Wang et al. (2017) highlighted that the iron gate mechanism is more important in mineral-rich or vascular plant-dominated wetlands. Similarly, van Bodegom et al. (2005) conducted a laboratory-based study to understand the interaction of iron with phenol oxidase and CO2 exchange in a waterlogged soil. The results showed that added Fe2+ significantly increased POX activities and CO2 production, measured as a proxy for decomposition.
The last question in this experimental study addressed the fate of phenolic addition in optimizing the productivity and biomass of the Sphagnum species under investigation. The results of this study interpreted that phenolic additions did not enhance Sphagnum productivity and biomass. Alshehri et al. (2020) revealed that phenolic enrichment in the form of wood chips of L. laricina, P. mariana, and T. occidentalis did not enhance Sphagnum productivity and biomass. The role of phenolics has not been widely tested for Sphagnum productivity and biomass; therefore, current study results were compared with those of the studies that reported Sphagnum productivity and biomass in any manner. In the current study, productivity values between the Acutifolia (378 ± 39 g m−2 yr−1) and the Sphagnum (363 ± 32 g m−2 yr−1) subgenera were not different but aligned within the range of data reported by another study (Table 5). The biomasses of the Acutifolia (983 ± 201 g m−2) and Sphagnum (819 ± 130 g m−2) subgenera, estimated from the same experimental site in 2017 (PERG unpublished data), were similar to the biomasses of both subgenera estimated in this study. This implies that Sphagnum biomass might have reached a point where biomass production equals decomposition, leading to constant biomass production. The results from this short-duration study pointed out that surface phenolic additions are not an ideal tool for promoting Sphagnum species productivity and biomass.
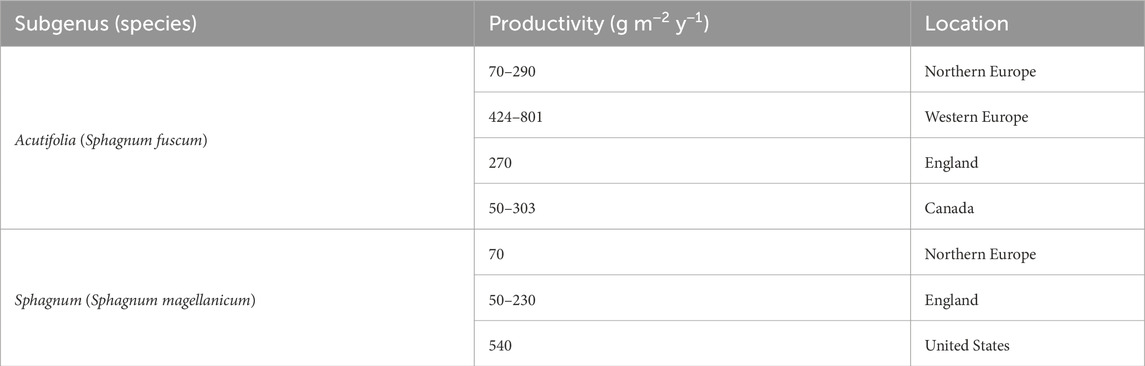
Table 5. Overview of Acutifolia and Sphagnum moss species productivity (g m−2 y−1) data from different locations. Table adapted from Rochefort et al. (1990).
Overall, we hypothesized that phenolic additions would enhance peat phenolic concentrations, leading to lower enzyme activities, lower CO2 emissions, and higher CO2 uptake. In contrast to our hypothesis, applied phenolic treatments did not increase peat phenolic abundance, constrain enzyme activities, limit CO2 emissions, or increase CO2 uptake. In the present study, extracellular enzyme activities at both subgenera showed a positive correlation with NEE (higher enzyme activities leading to higher CO2 emissions), which is consistent with the ELM hypothesis. However, this response could not be directly attributed to effects on enzyme activities as these were not inhibited by the phenolic additions or anoxic conditions. Our results conflict with the findings of Freeman et al. (2004), which showed that hydrolase and POX activities can be inhibited when the abundance of phenolic compounds increases. Broadly, for both subgenera, our results clearly showed no evidence of phenolic enrichment in response to our treatments. It is also essential to look for the conceptual relationship between the observed variables that could lead to better interpretation. In the peatlands, a slow rate of decomposition is well-established, but how much it is impacted by phenolic additions remains uncertain. Further research is needed to identify potential phenolic products that could increase peat phenolic concentration and limit CO2 emissions or enzyme activities, leading to enhanced productivity in a Sphagnum farming system.
5 Conclusion
This study examined the role of surface application of phenolics in limiting decomposition at the base of the acrotelm (∼10 cm below the Sphagnum surface) in a Sphagnum farming system. Broadly, both subgenera showed indistinguishable responses to phenolic enrichment. The phenolic additions neither increased peat phenolic concentration nor inhibited extracellular enzyme activities—as hypothesized in this study and by the ELM. During the field study period (1.5 growing seasons), phenolic addition did not prove to be a cost-effective strategy for reducing decomposition or optimizing the productivity and biomass accumulation of mosses from the Acutifolia and Sphagnum subgenera. The results of this study did not provide strong evidence for understanding the role of phenolic addition in limiting decomposition. Primarily, we assumed that phenolic products, especially wood pellet decomposition, contributed to greater carbon dioxide emissions. It is also assumed that the surface application of phenolic products did not induce the inhibitory effects on extracellular enzyme activities at the Sphagnum carpet depth from where all sampling was taken. Consequently, further research is needed to explore other sources of phenolic products and alternative application methods. For surface application, long-term monitoring and sampling from various depths will be required to understand peat phenolic concentrations, enzyme activities, and carbon dioxide flux. Additionally, more evidence is required to identify phenolic products that could increase peat phenolic concentrations and limit decomposition, thereby optimizing productivity in a Sphagnum farming system.
Data availability statement
The raw data supporting the conclusions of this article will be made available by the authors, without undue reservation.
Author contributions
TA: conceptualization, formal analysis, investigation, methodology, software, validation, visualization, writing – original draft, and writing – review and editing. LR: conceptualization, funding acquisition, resources, supervision, and writing – review and editing. CF: conceptualization, supervision, and writing – review and editing. CD: writing – review and editing. HK: writing – review and editing. MG-N: writing – review and editing.
Funding
The author(s) declare that financial support was received for the research and/or publication of this article. Financial support for this study was provided by the National Sciences and Engineering Research Council of Canada (NSERC) through a Collaborative Research and Development Grant supported by the Canadian Sphagnum Peat Moss Association (CSPMA) and its members (grant no. CRDPJ 769 517951 – 17).
Acknowledgments
The authors thank the Canadian Sphagnum Peat Moss Association and its members for providing the facilities to conduct research work. They also appreciate Maria Strack for knowledge transfer related to carbon flux. They are also grateful for the Peatland Ecology Research Group (PERG) colleagues and research assistants who helped during the field or laboratory work. In particular, they are grateful to Andrew Albert Rutland, Meike Lemmer, Aamir Shehzad Khan, Laura Catalina Riano Pena, Charles Gignac, Kathy Pouliot, Brigitte Vimard Mathieu Ferland, Myriam Tougas-Dumesnil, Louis-Philippe Thériault, Siska Shooner, and Christina Tougas.
Conflict of interest
The authors declare that the research was conducted in the absence of any commercial or financial relationships that could be construed as a potential conflict of interest.
Generative AI statement
The author(s) declare that no Generative AI was used in the creation of this manuscript.
Publisher’s note
All claims expressed in this article are solely those of the authors and do not necessarily represent those of their affiliated organizations, or those of the publisher, the editors and the reviewers. Any product that may be evaluated in this article, or claim that may be made by its manufacturer, is not guaranteed or endorsed by the publisher.
Supplementary material
The Supplementary Material for this article can be found online at: https://www.frontiersin.org/articles/10.3389/feart.2025.1554757/full#supplementary-material
References
Alshehri, A., Dunn, C., Freeman, C., Hugron, S., Jones, T. G., and Rochefort, L. (2020). A potential approach for enhancing carbon sequestration during peatland restoration using low-cost, phenolic-rich biomass supplements. Front. Environ. Sci. 8, 48. doi:10.3389/fenvs.2020.00048
Alm, J., Talanov, A., Saarnio, S., Silvola, J., Ikkonen, E., Aaltonen, H., et al. (1997). Reconstruction of the carbon balance for microsites in a boreal oligotrophic pine fen, Finland. Oecologia 110, 423–431. doi:10.1007/s004420050177
Asada, T., Warner, B. G., and Banner, A. (2003). Growth of mosses in relation to climate factors in a hypermaritime coastal peatland in British Columbia, Canada. Bryologist 106 (4), 516–527. doi:10.1639/0007-2745(2003)106[516:GOMIRT]2.0.CO;2
Bates, D., Mächler, M., Bolker, B., and Walker, S. (2015). Fitting linear mixed-effects models using lme4. J. Stat. Softw. 67 (1), 1–48. doi:10.18637/jss.v067.i01
Brown, C. M., Strack, M., and Price, J. S. (2017). The effects of water management on the CO2 uptake of Sphagnum moss in a reclaimed peatland. Mires Peat 20 (05), 1–15. doi:10.19189/MaP.2016.OMB.258
Caron, J., and Rochefort, L. (2013). Use of peat in growing media: state of the art on industrial and scientific efforts envisioning sustainability. ISHS Acta Hortic. Int. symposium responsible Peatl. Manag. Grow. media Prod. 982, 15–22. doi:10.17660/ActaHortic.2013.982.1
Coulson, J. C., and Butterfield, J. (1978). An investigation of the biotic factors determining the rates of plant decomposition on blanket bog. J. Ecol. 66, 631–650. doi:10.2307/2259155
Dixon, R. A. (2001). Natural products and plant disease resistance. Nature 411, 843–847. doi:10.1038/35081178
Dunn, C., Jones, T. G., Girard, A., and Freeman, C. (2014). Methodologies for extracellular enzyme assays from wetland soils. Wetlands 34 (1), 9–17. doi:10.1007/s13157-013-0475-0
Emmel, M. (2008). “Growing ornamental plants in Sphagnum biomass,” in ISHS acta horticulture proceedings of the international symposium on growing media 779, 173–178. doi:10.17660/ActaHortic.2008.779.20
Fenner, N., and Freeman, C. (2020). Woody litter protects peat carbon stocks during drought. Nat. Clim. Change 10, 363–369. doi:10.1038/s41558-020-0727-y
Freeman, C., Fenner, N., and Shirsat, A. H. (2012). Peatland geoengineering: an alternative approach to terrestrial carbon sequestration. Philos. Trans. R. Soc. A Math. Phys. Eng. Sci. 370 (1974), 4404–4421. doi:10.1098/rsta.2012.0105
Freeman, C., Ostle, N., and Kang, H. (2001). An enzymic “latch” on a global carbon store: a shortage of oxygen locks up carbon in peatlands by restraining a single enzymes. Nature 409 (6817), 149. doi:10.1038/35051650
Freeman, C., Ostle, N. J., Fenner, N., and Kang, H. (2004). A regulatory role for phenol oxidase during decomposition in peatlands. Soil Biol. biochem. 36 (10), 1663–1667. doi:10.1016/j.soilbio.2004.07.012
Gaudig, G., Fengler, F., Krebs, M., Prager, A., Schulz, J., Wichmann, S., et al. (2014). Sphagnum farming in Germany – a review of progress. Mires Peat 13 (08), 1–11. Available online at: http://www.mires-and-peat.net/pages/volumes/map13/map1308.php.
Gaudig, G., Krebs, M., and Joosten, H. (2017). Sphagnum farming on cut-over bog in NW Germany: long-term studies on Sphagnum growth. Mires Peat 20, 1–19. doi:10.19189/MaP.2016.OMB.238
Guêné-Nanchen, M., Pouliot, R., Hugron, S., and Rochefort, L. (2017). Effect of repeated mowing to reduce graminoid plant cover on the moss carpet at a Sphagnum farm in North America. Mires Peat 20 (6), 1–12. doi:10.19189/MaP.2016.OMB.250
Guêné-Nanchen, M., and St-Hilaire, B. (2022). Sphagnum farming in Canada: state of knowledge. CSPMA and APTHQ. Québec, Quebec.
Gunnarsson, U., and Rydin, H. (2000). Nitrogen fertilization reduces Sphagnum production in bog communities. New Phytol. 147 (3), 527–537. doi:10.1046/j.1469-8137.2000.00717.x
Günther, A., Jurasinski, G., Albrecht, K., Gaudig, G., Krebs, M., and Glatzel, S. (2017). Greenhouse gas balance of an establishing Sphagnum culture on a former bog grassland in Germany. Mires Peat 20, 1–16. doi:10.19189/MaP.2015.OMB.210
Gutierrez Pacheco, S., Lagacé, R., Hugron, S., Godbout, S., and Rochefort, L. (2021). Estimation of daily water table level with bimonthly measurements in restored ombrotrophic peatland. Sustainability 13 (10), 5474. doi:10.3390/su13105474
Harris, L. I., Moore, T. R., Roulet, N. T., and Pinsonneault, A. J. (2020). Limited effect of drainage on peat properties, porewater chemistry, and peat decomposition proxies in a boreal peatland. Biogeochemistry 151 (1), 43–62. doi:10.1007/s10533-020-00707-1
Hothorn, T., Bretz, F., and Westfall, P. (2008). Simultaneous inference in general parametric models. Biom. J. 50 (3), 346–363. doi:10.1002/bimj.200810425
Jobin, P., Caron, J., and Rochefort, L. (2014). Developing new potting mixes with Sphagnum fibers. Can. J. Soil Sci. 94, 585–593. doi:10.4141/cjss2013-103
Johnson, L. C., and Damman, A. W. H. (1991). Species-controlled Sphagnum decay on a South Swedish raised bog. Oikos 61, 234–242. doi:10.2307/3545341
Joosten, H., Tapio-Biström, M. L., and Tol, S. (2012). Peatlands-guidance for climate change mitigation through conservation, rehabilitation and sustainable use. Mitigation of climate change in agriculture (MICCA) programme. Food Agric. Organ. U. N.
Kim, J., Rochefort, L., Hogue-Hugron, S., Alqulaiti, Z., Dunn, C., Pouliot, R., et al. (2021). Water table fluctuation in peatlands facilitates fungal proliferation, impedes Sphagnum growth and accelerates decomposition. Front. Earth Sci. 8 (April), 1–9. doi:10.3389/feart.2020.579329
Lenth, R. (2023). Emmeans: estimated marginal means, aka least-squares means_. R. package version 1.8.5. doi:10.32614/CRAN.package.emmeans
Luthardt, V., and Wichmann, S. (2016). “Ecosystem services of peatlands,” in Paludiculture - productive use of wet peatlands. Editors W. Wichtmann, C. Schröder, and H. Joosten (Stuttgart: Schweizerbart Science Publishers), 13–20.
Moore, T. R. (1989). Growth and net production of Sphagnum at five fen sites, subarctic eastern Canada. Can. J. Bot. 67, 1203–1207. doi:10.1139/b89-156
Müller, R., and Glatzel, S. (2021). Sphagnum farming substrate is a competitive alternative to traditional horticultural substrates for achieving desired hydro-physical properties. Mires Peat (27). doi:10.19189/MaP.2021.OMB.StA.2157
Pinsonneault, A. J., Moore, T. R., and Roulet, N. T. (2016). Temperature the dominant control on the enzyme-latch across a range of temperate peatland types. Soil Biol. biochem. 97, 121–130. doi:10.1016/j.soilbio.2016.03.006
Pipes, G. T., and Yavitt, J. B. (2022). Biochemical components of Sphagnum and persistence in peat soil. Can. J. Soil Sci. 102 (3), 785–795. doi:10.1139/cjss-2021-0137
Pouliot, R., Hugron, S., and Rochefort, L. (2015). Sphagnum farming: a long-term study on producing peat moss biomass sustainably. Ecol. Eng. 74, 135–147. doi:10.1016/j.ecoleng.2014.10.007
R Core Team (2023). _R: a language and environment for statistical computing_. Vienna, Austria: R Foundation for Statistical Computing.
Reinikainen, O., Korpi, J., Tahvonen, R., Näkkilä, J., Silvan, N., and Silvan, K. (2012). Harvesting of Sphagnum biomass and its use as a growing medium constituent. Proc. 14th Int. Peat Congr. Stockh. Swed., No. 137, International Peat Society, Jyväskylä, Finland.
Rochefort, L., Vitt, D. H., and Bayley, S. E. (1990). Growth, production, and decomposition dynamics of Sphagnum under natural and experimentally acidified conditions. Ecol. 71 (5), 1986–2000. doi:10.2307/1937607
Rochefort, L., Quinty, F., Campeau, S., Johnson, K., and Malterer, T. (2003). North American approach to the restoration of Sphagnum dominated peatlands. Wetl. Ecol. Manag. 11, 3–20. doi:10.1023/A:1022011027946
Sjörs, H., and Sjors, H. (1950). On the relation between vegetation and electrolytes in north Swedish mire waters. Oikos 2 (2), 241–258. doi:10.2307/3564795
Urbanová, Z., and Hájek, T. (2021). Revisiting the concept of ‘enzymic latch’ on carbon in peatlands. Sci. Total Environ. 779, 146384. doi:10.1016/j.scitotenv.2021.146384
Van Bodegom, P. M., Broekman, R., Van Dijk, J., Bakker, C., and Aerts, R. (2005). Ferrous iron stimulates phenol oxidase activity and organic matter decomposition in waterlogged wetlands. Biogeochemistry 76 (1), 69–83. doi:10.1007/s10533-005-2053-x
van Breemen, N. (1995). How Sphagnum bogs down other plants. Trends Ecol. Evol. 10 (7), 270–275. doi:10.1016/0169-5347(95)90007-1
Wallis, C. M., and Galarneau, E. R.-A. (2020). Phenolic compound induction in plant-microbe and plant-insect interactions: a meta-analysis. Front. Plant Sci. 11, 580753. doi:10.3389/fpls.2020.580753
Wang, Y., Wang, H., He, J. S., and Feng, X. (2017). Iron-mediated soil carbon response to water-table decline in an alpine wetland. Nat. Commun. 8 (May), 15972–15979. doi:10.1038/ncomms15972
Wetzel, R. G. (1992). Gradient-dominated ecosystems: sources and regulatory functions of dissolved organic matter in freshwater ecosystems. Hydrobiologia 229, 181–198. doi:10.1007/bf00007000
W. Wichtmann, C. Schröder, and H. Joosten (2016). Paludiculture - productive use of wet peatlands. Climate protection, biodiversity, regional economic benefits (Stuttgart: Schweizerbart Science Publishers), 272.
Wickham, H. (2016). ggplot2: elegant graphics for data analysis. New York: Springer-Verlag. Available online at: https://ggplot2.tidyverse.org.
Keywords: paludiculture, Sphagnum farming, phenolic additions, phenol oxidase, hydrolase, carbon dioxide exchange
Citation: Asif T, Rochefort L, Freeman C, Dunn C, Kang H and Guêné-Nanchen M (2025) Phenolic supplements: testing an approach to limit Sphagnum decomposition in a Sphagnum farming system. Front. Earth Sci. 13:1554757. doi: 10.3389/feart.2025.1554757
Received: 02 January 2025; Accepted: 14 April 2025;
Published: 13 May 2025.
Edited by:
Evan S. Kane, Michigan Technological University, United StatesReviewed by:
Karl Romanowicz, University of Oregon, United StatesMagdalena Wiedermann, Lund University, Sweden
Copyright © 2025 Asif, Rochefort, Freeman, Dunn, Kang and Guêné-Nanchen. This is an open-access article distributed under the terms of the Creative Commons Attribution License (CC BY). The use, distribution or reproduction in other forums is permitted, provided the original author(s) and the copyright owner(s) are credited and that the original publication in this journal is cited, in accordance with accepted academic practice. No use, distribution or reproduction is permitted which does not comply with these terms.
*Correspondence: Line Rochefort, bGluZS5yb2NoZWZvcnRAZnNhYS51bGF2YWwuY2E=