- 1Posgrado en Ciencias del Mar y Limnología, Universidad Nacional Autónoma de México, México, Mexico
- 2Instituto de Geofísica, Universidad Nacional Autónoma de México, México, Mexico
- 3Instituto de Investigación Científica y Estudios Avanzados Chicxulub, Parque Científico y Tecnológico de Yucatán, Mérida, Mexico
- 4Instituto de Ciencias del Mar y Limnología, Universidad Nacional Autónoma de México, México, Mexico
- 5Aquatic Biogeochemistry Lab, Instituto de Ciencias del Mar y Limnología, Universidad Nacional Autónoma de México, México, Mexico
- 6Museo de Paleontología, Universidad Autónoma del Estado de Hidalgo, Ciudad del Conocimiento, Hidalgo, Mexico
- 7Unidad Académica Mazatlán, Instituto de Ciencias del Mar y Limnología, Universidad Nacional Autónoma de México, Mazatlán, Mexico
- 8Laboratoire d’Oceanographie et du Climat: Expérimentation et Approaches Numériques Centre. Sorbonne Université, Institut de Recherche pour le Développement, Centre National de la Recherche Scientifique, Muséum National d’histoire Naturelle, Bondy, France
- 9International Ocean Discovery Program, Texas A&M University, College Station, TX, United States
The Guaymas Basin (GB) is a highly productive region in the Gulf of California. Subseafloor sedimentary amorphous bio-opal and Ba/Ti records obtained from its northwestern and central areas reveal significant changes in exported productivity over the past 31,200 years. Millennial-scale variability reflects the influence of wind-driven upwelling, mesoscale eddies, and shifts in climate variability operating at orbital, millennial, and centennial timescales. Spatial heterogeneity in productivity recorded in International Ocean Discovery Program boreholes highlights regional differences in process dominance. We identify seven distinct productivity phases: From ∼31,200 to ∼26,500 cal yr BP, laminated sediments indicate strong seasonal variability and high productivity due to intense upwelling activity caused by northwesterly winds linked to a southward-shifted Intertropical Convergence Zone. The Last Glacial Maximum, from ∼26,500 to ∼19,000 cal yr BP., displayed pronounced fluctuations and a slight decline in productivity compared to the previous interval, owing to the reduced influence of the North Pacific High on the GB during this period. From ∼19,000 to ∼11,700 cal yr BP, there were shifts of high and low productivity, with opal minima coinciding with Heinrich events 2 and 1, as well as the Younger Dryas. Productivity declined slightly between ∼11,700 and ∼7,000 cal yr BP, featuring a short high-productivity period within that timespan (∼10,500 to ∼10,300 cal yr BP). From ∼7,000 to ∼4,200 cal yr BP, productivity decreased in the NW and increased in the central basin. This contrast reflects enhanced winter-spring coastal wind-driven upwellings and reduced eddy activity in the west. From ∼4,200 to ∼130 cal yr BP, productivity increased in both studied areas. The sedimentary Ba/Ti values in both holes generally indicate lower levels during the cold glacial period and higher levels during the warm interglacial period, suggesting reduced biological barite accumulation and less organic matter export from the surface under cold climate conditions. These changes correspond to documented climate transitions, highlighting GB’s sensitivity to global forcings (e.g., ice sheet retreat) and regional ocean-atmosphere interactions. Our findings underscore the key role of dynamic physical processes in shaping long-term productivity patterns in marginal seas at high resolution.
1 Introduction
Marine primary productivity is a fundamental component of the Earth’s biogeochemical cycles, sustaining marine ecosystems and influencing global carbon fluxes (Jiang et al., 2024). The average vertically integrated production in the Gulf of California (GoC) is estimated at ∼1.8 gCm−2 (Álvarez-Borrego, 2012). The GoC is one of the most productive regions on the planet (Lluch-Cota, 2000; Álvarez-Borrego, 2012). In particular, the high productivity of the overlying water column in the GB is remarkable, with diatoms being the dominant primary producers (Sancetta, 1995; Thunell, 1998; Pichevin et al., 2014; Teske et al., 2019; Aiello et al., 2024), resulting in the deposition of organic-rich diatomaceous sediments containing up to 4 wt.% organic carbon (Seewald et al., 2024), with an average productivity estimated to be >4 gCm−2 day−1 (Pike and Kemp, 1997; Thunell, 1998; Barron et al., 2014).
Marine productivity reconstructions are helpful in better understanding the relationship between ocean dynamics and climate drivers that shape ocean systems over time. The GB is a unique environment for investigating these processes due to its ocean dynamics and sensitivity to climatic fluctuations (Cheshire and Thurow, 2013; Price et al., 2013). The Pacific Ocean strongly influences the GoC, driving regional oceanic-specific physical processes in the GB, such as wind-driven upwelling and mesoscale eddies. The eddies are a key (if not the most important) component of the GoC circulation during the summer (Lavín et al., 2013), that transport and retain chemical properties and planktonic organisms, and that can enhance nutrient vertical flux and promote high productivity.
The GB sedimentary sequence provides high-resolution records of paleoclimatic changes during the late Pleistocene and Holocene (e.g., Barron et al., 2005). Over the past 31,000 years, variations in insolation, Intertropical Convergence Zone (ITCZ) latitudinal migration, and the influence of the North Pacific High (NPH) have interacted with the Tropical Pacific Ocean processes to shape productivity patterns in the GoC and GB (McClymont et al., 2012; Cheshire and Thurow, 2013; Barron et al., 2014). The GB sensitivity to regional variations and the large-scale circulation of the Eastern Tropical North Pacific Ocean (ETNP) makes it ideal for studying regional and global climatic shifts over different time scales (e.g., orbital to centennial scales). Besides, tectonic dynamics contribute to sedimentary variations reflecting changes in sea level, ocean circulation, and local geological processes (Lizarralde et al., 2007; Miller and Lizarralde, 2013; Lizarralde et al., 2023).
1.1 Climate setting
The GoC is an elongated oceanic basin that extends over 1,000 km where interactions between the ocean, atmosphere, and land are interconnected and influenced by the Pacific Ocean dynamics (Lavín and Marinone, 2003; Lavín et al., 2014). The GoC is also affected by the North American Monsoon (NAM), the position of the ITCZ, the NPH, and the hemispheric teleconnections (Amador et al., 2006; Pérez-Cruz, 2013; Barron et al., 2014) (Figure 1A).
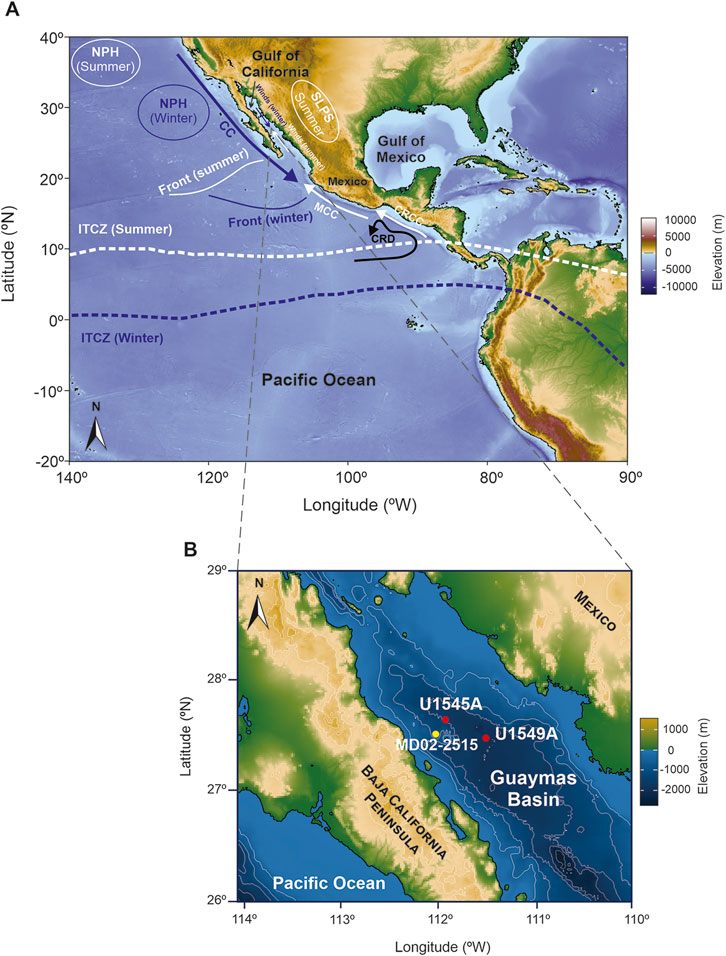
Figure 1. (A) Atmospheric and ocean dynamics in summer (white) and in winter (blue): NPH, North Pacific High-Pressure system; SLPS, Sonora Low-Pressure System; CC, California Current; MCC, Mexican Coastal Current; Costa Rica Coastal Current, CRCC; Costa Rica Dome, CRD. Continuous lines indicate the front between the CC and the Tropical Surface Water (Kessler, 2006). Dashed lines represent the average position of the Intertropical Convergence Zone. (B) Holes U1545-A, U1549-A and MD02-2515 (Pichevin et al., 2012).
The GoC has monsoon climate-driven seasonal wind reversal, characterized by two modes. These modes vary in intensity and duration owing to changes in insolation over different time scales (Douglas et al., 2007; Pérez-Cruz, 2013). Insolation is lowest during the winter-spring mode. The position of the NPH is around 30°N, driving NW winds along the gulf at speeds up to 12 ms−1 (Bordoni et al., 2004; Douglas et al., 2007; Pérez-Cruz, 2013). This promotes mixing and wind-driven upwelling, enhancing nutrient supply and productivity. The ITCZ is located at its southernmost annual position during the winter-spring mode. The Mexican Coastal Current (MCC), also known as the Western Coastal Current (WMC), weakens during this mode, and the Tropical Surface Water along the gulf becomes less evident (Badan-Dangon et al., 1985; García-Morales et al., 2017) (Figure 1A).
In contrast, during the summer-fall mode, insolation is maximum. The NPH and ITCZ move north to approximately 35° and 13°, respectively (Badan-Dangon et al., 1991; Bordoni et al., 2004), with wind direction reversing (Figure 1A). This leads to SE winds propagating over the gulf in pulses with wind speeds of ∼3–5 ms−1 (Badan-Dangon et al., 1991; Bordoni et al., 2004). Summer and fall winds are weak, and productivity declines. The MCC and Costa Rica Coastal Current (CRCC) promote the incursion of Tropical Surface Water into the GoC (Santamaría-del-Angel et al., 1994; Lluch-Cota, 2000; Lavín and Marinone, 2003; Espinosa-Carreón and Escobedo-Urías, 2017).
The oceanic circulation in the GoC exhibits distinct seasonal patterns and dynamic mesoscale features. According to numerical modeling (Lavín and Marinone, 2003), during the summer-fall mode, there is a general cyclonic circulation with water inflow along the eastern margin and outflow along the western margin (Figure 2A). This pattern reverses during winter (Figure 2B) (Ripa, 1997; Palacios-Hernández et al., 2002; Marinone, 2003). Beyond this general circulation, mesoscale structures such as eddies, meanders, and jets frequently occur (Figures 2C–E), playing a significant role in the region´s ocean dynamics (Fernández-Barajas et al., 1994; Pegau et al., 2002; Lavín et al., 2013; Lavín et al., 2014; Mercado-Santana et al., 2017; Acevedo-Acosta and Martínez-López, 2022).
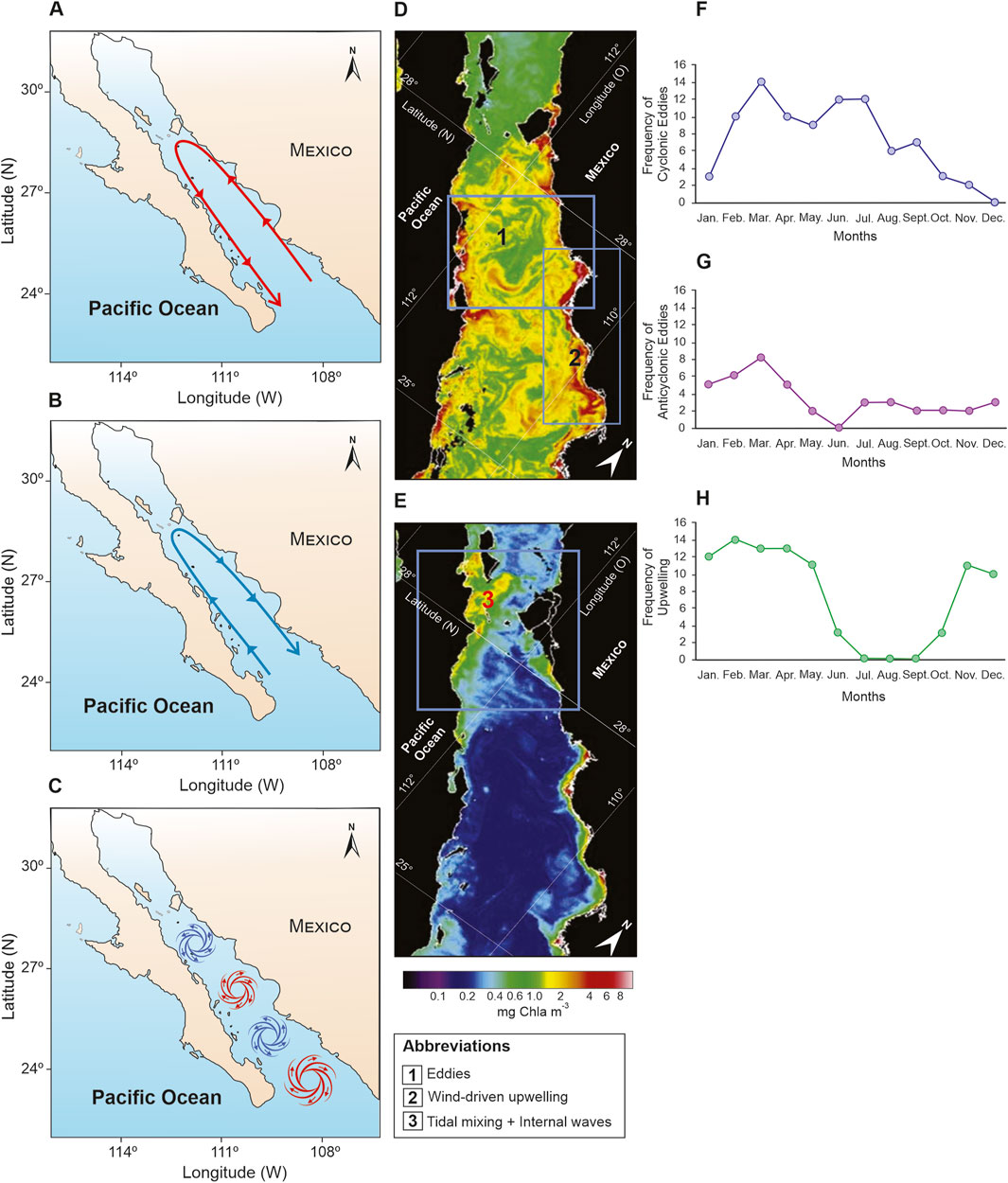
Figure 2. Ocean surface circulation patterns in the GoC. (A) Summer-fall mode. (B) Winter-spring mode. (C) Schematic representation of mesoscale eddies circulation in the GoC (modified from Acevedo-Acosta and Martínez-López, 2022). Satellite imagery of chlorophyll distribution illustrating key physical processes driving productivity in GB (Mercado-Santana et al., 2017; Multisensor imagery, Aqua/MODIS, Terra/MODIS, and VIIRS): (D) Wind-driven upwellings and mesoscale eddies (Chla sat, January 9, 2016), and (E) Tidal mixing and internal waves (Chla sat, August 5, 2016). Monthly frequency of mesoscale processes (García-Morales et al., 2017): (F) Cyclonic eddies, and (G) Anticyclonic eddies. (H) Wind-driven upwelling.
In the GB, prominent circulation features include mesoscale eddies (Figure 2C), which significantly influence basin productivity (Figure 2D) (Lavín et al., 2013; Lavín et al., 2014; García-Morales et al., 2017). The formation of these eddies has been studied using various techniques, such as satellite imagery of sea surface temperature (SST) and chlorophyll-a (Mercado-Santana et al., 2017; Figures 2D,E) (Badan-Dangon et al., 1985; Gaxiola-Castro et al., 1999; Pegau et al., 2002; García-Morales et al., 2017), satellite-tracked drifters (Lavín et al., 1997), and numerical models (Zamudio et al., 2008). Direct observations reveal that eddies are closely spaced along the GoC, alternating between cyclonic and anticyclonic rotations (Figure 2C) (Fernández-Barajas et al., 1994; Lavín et al., 2013). Cyclonic eddies are most noticeable from February to April and from June to July (Figure 2F), while anticyclonic eddies are more prominent from January to May (Figure 2G) (García-Morales et al., 2017). Anticyclonic eddies enhance productivity by pumping nutrient-rich waters along the continental slope and transporting chlorophyll-a-rich waters, especially when they interact with coastal upwelling systems (Merino and Monreal-Gómez, 2009; Dufois et al., 2016; Venkatachalam et al., 2017). These features play a crucial role in transporting suspended materials, facilitating vertical nutrient exchange between subsurface and surface layers, and influencing the distribution of phytoplankton, thus promoting high productivity along the western side of the gulf (García-Morales et al., 2017; López-Martínez et al., 2023). In contrast, the eastern margin primarily relies on wind-driven upwelling as the dominant driver of productivity during winter and spring (Figure 2H).
1.2 Sediment sequence
The relationship between winds, physical processes, plankton productivity, and the sinking of plankton to the seafloor that generates skeletal debris, bio-opal (opal), and calcium carbonate exemplifies how physical and climate drivers influence biogenic sedimentation (Thunell, 1998). The modern GB is recognized for the rapid sedimentation of predominantly diatomaceous ooze (Calvert, 1966; Douglas et al., 2007; Teske et al., 2021). The productivity exported is recorded in the sediments covering the seafloor, which consist of both organic-rich sediments derived from highly productive overlying waters and terrigenous material input from continental margins in summer, primarily via fluvial processes (Calvert, 1966; Baumgartner et al., 1991; Thunell et al., 1994; Thunell, 1998; Barbara et al., 2016).
The core sediments analyzed in this study mainly consist of a mix of biogenic and terrigenous materials, particularly siliceous diatom oozes containing clay-to-silt-sized siliciclastic particles (Persad and Marsaglia, 2023). This composition reflects the region’s high biogenic silica productivity, with diatoms being the primary siliceous microfossil.
1.3 Previous studies
During the late Quaternary, the global climate alternated between warm interglacial and cold glacial periods (Lisiecki and Raymo, 2005). These climate changes influenced the oceanic circulation, biological productivity, and the global carbon cycle (Bauska et al., 2021). Paleoceanographic and paleoclimate records in the GB during the past 50,000 years include various proxies (Barron et al., 2005; McClymont et al., 2012; Pichevin et al., 2012; 2014; Barron et al., 2014). Younger Dryas-like (YD) events have been identified in GB during glacial conditions, characterized by low productivity and high terrigenous input (Cheshire et al., 2005). Also, productivity shifts are recognized from Marine Isotope Stages MIS-3 to MIS-1 (Pichevin et al., 2012; Price et al., 2013; Barron et al., 2014; Velázquez-Aguilar et al., 2024). During MIS-3 and-1, high productivity is attributed to active wind-driven upwelling and well-mixed conditions. During MIS-2, particularly at the Last Glacial Maximum (LGM), the weakening of wind-driven upwelling resulted in low productivity (Price et al., 2013) and oligotrophic conditions (Barron et al., 2014; Velázquez-Aguilar et al., 2024). Other records indicate low productivity during the Heinrich-I event and the YD compared to the Holocene (MIS-1) (Barron et al., 2005; Barron et al., 2014; Pichevin et al., 2012; Velázquez-Aguilar et al., 2024). In the Holocene, proxies indicate high productivity in the GB during the Holocene Climate Optimum (HCO) (Velázquez-Aguilar et al., 2024).
Pichevin et al. (2012), Pichevin et al. (2014) analyzed silica fluxes and the Si/Corg ratio in sediments from the GoC and found that the Si/Corg ratio increases significantly during periods of intense upwelling. They found that these periods are associated with transient iron (Fe) limitation that results in a high export of silica relative to organic carbon, a diagnostic trait for diatoms growing under iron stress, which enhances their silica uptake. This suggests that the high opal burial observed in the GoC can be partially attributed to the presence of transient Fe limitation, and consequently, the diatom ∂30Si there increases with greater silicic acid utilization (Pichevin et al., 2014).
Overall, the paleoceanographic studies in this region outline that the environmental processes influencing GB productivity still need further investigation. This study aims to enhance our understanding of productivity changes in the northwestern and central basins over the past 31,200 years using geochemical proxies with opal and Ba/Ti values. We focus on significant shifts and their underlying causes. The study examines the role of oceanic processes, such as wind-driven upwelling and mesoscale eddies, in shaping productivity patterns. Additionally, the research assesses how climatic changes influenced the GB and GoC dynamics.
2 Materials and methods
2.1 Core collection and sampling
During the IODP Expedition 385 in the GB, cores were recovered aboard the deep-sea R/V JOIDES Resolution (JR). We focus on two sites, U1545 and U1549, specifically Hole A drilled at each site. Hole U1545A is located in the northwestern part of the GB at 27°38.23′N, 111°53.34′W (Figure 1B) (Teske et al., 2021), and we studied the uppermost 42.29 m below the seafloor (mbsf) of sediments (cores 1H–5H), with a resolution of approximately 30 cm (n = 122). Hole U1549A is located in the central part of the GB at 27°28.33′N, 111°28.78′W (Figure 1B) (Teske et al., 2021), and we studied the uppermost 29.19 mbsf of sediments (cores 1H–4H) with a resolution of approximately 60 cm (n = 29).
2.2 Age models
The age models reported by Velázquez-Aguilar et al. (2024) are revised for this study. Age models are based on 17 accelerator mass spectrometry 14C dates of bulk sediment samples from Hole U1545A and 12 from Hole U1549A (Table 1). AMS radiocarbon analyses were conducted at the Rafter GNS Science at the National Isotope Centre, in New Zealand. The age models and sedimentation rates are calculated using Bayesian statistics with a Markov Chain-Montecarlo algorithm and the Marine20 calibration curve (Heaton et al., 2020). The calculations were performed using the R statistical software version 4.3.0 (R Core Team, 2020) and the rbacon library (Blaauw et al., 2011). Radiocarbon dates were corrected using a local reservoir age of ΔR = 301 ± 50 years (Goodfriend and Flessa, 1997). For Hole U1545A, ages were in stratigraphic order. The time frame spanned 31,260 to 490 cal years BP (Figure 3A), with an average sedimentation rate of ∼1.45 mmyr−1 (Figure 3B). It is worth mentioning that the sediments in the studied cores show no signs of disturbance or hiatus (Teske et al., 2021). Hole U1549A, ages ranged from 16,750 to 130 cal years BP (Figure 3C). The sample U1549-A-3H-1W_20–22 at 16.21 mbsf (Figure 3D) was in a gravity flow deposit (Teske et al., 2021), which could introduce an age error. To address it, we integrated the information on gravity flow depth into the Bacon software. This adjustment improved the accuracy and reliability of the age-depth relationship, providing a reliable calibration process and ensuring that it accurately accounts for temporal variations of accumulation rates.
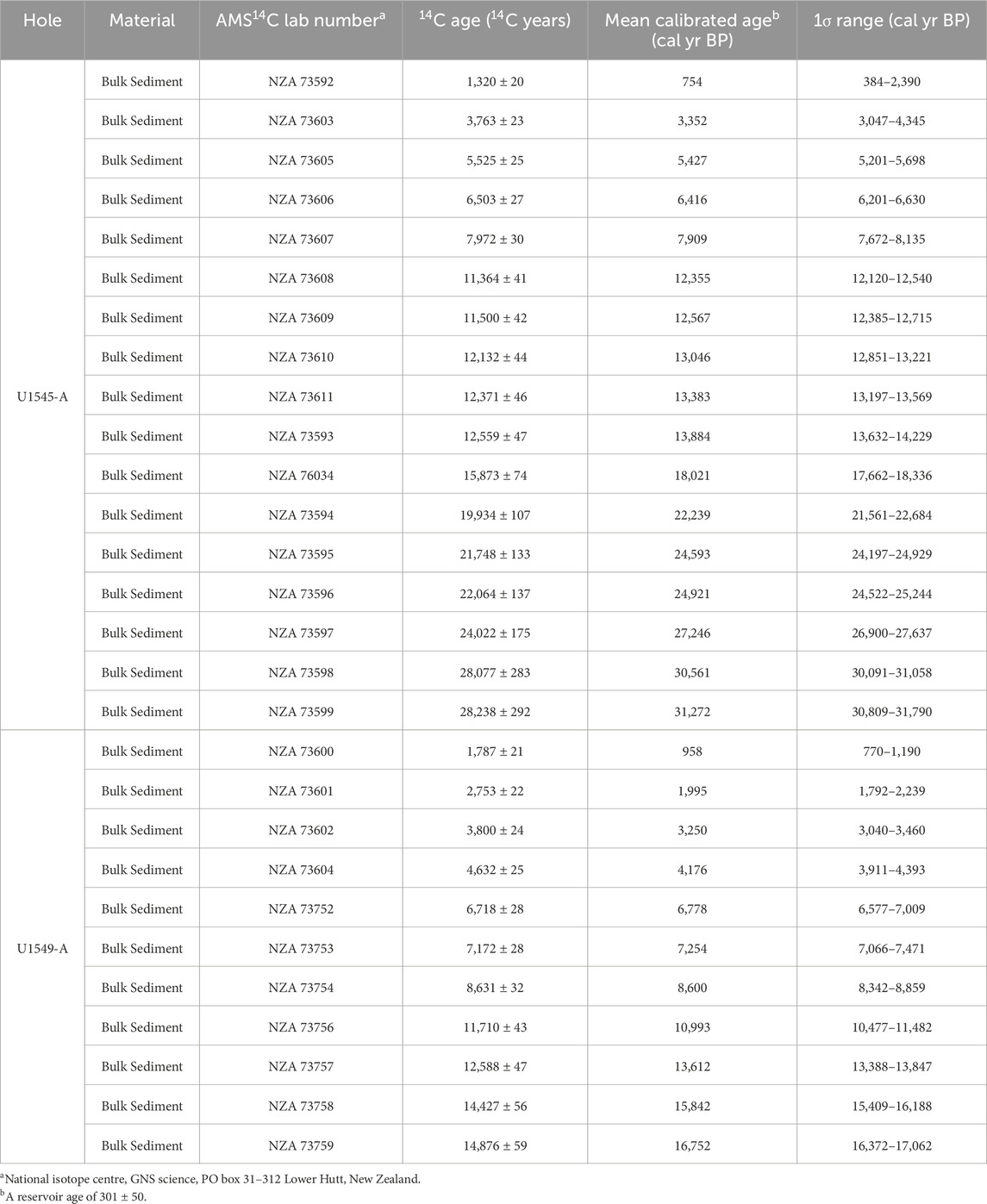
Table 1. Guaymas Basin, Gulf of California, Mexico, radiocarbon data (Velázquez-Aguilar et al., 2024).
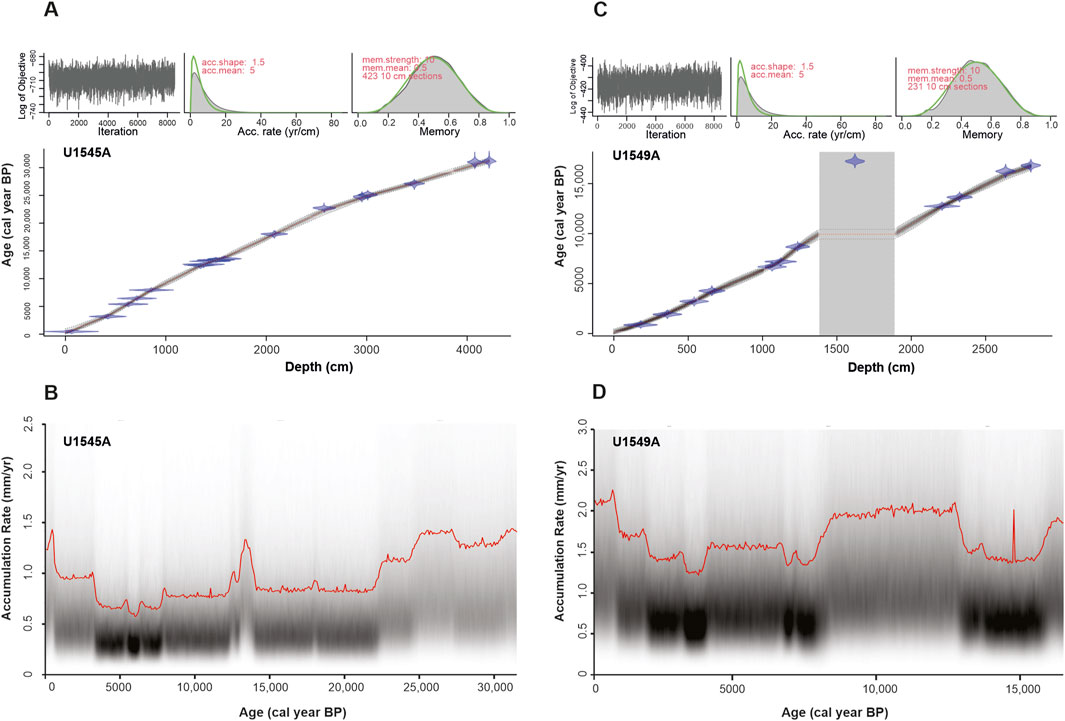
Figure 3. (A) Age-depth relationship and (B) sedimentation rate (red, mean values; grayscale, probability density) for Hole U1545A. (C) Age-depth relationship. (D) sedimentation rate (red -mean values; grayscale - probability density) for Hole U1549A.
2.3 Geochemical analyses
Exported biogenic silica (opal) is one of the major components in pelagic sediments, especially in high-nutrient environments where siliceous phytoplankton is abundant (Ragueneau et al., 1996). In the GB, the skeletal remains of siliceous organisms provide a record to identify changes in surface primary productivity (Sancetta, 1995; Barron et al., 2014). Opal records show a higher preservation efficiency than other productivity proxies (such as organic carbon) and better reflect changes in surface productivity (Ragueneau et al., 2000). Thus, they provide valuable insight into changes in surface ocean processes (Ragueneau et al., 1996). The composition of specific biogenic elements (diatoms) compared to the element concentrations in marine plankton in the GoC sediments suggests the accumulation of Si and Ba as opal and biogenic barite (Brumsack, 1989; Aiello et al., 2024).
In the sediments examined in this study, diatoms are the primary siliceous microfossils and the main contributors to opal records. Opal analysis was conducted using the wet-alkaline extraction procedure (Mortlock and Froelich, 1989) at the Laboratorio de Paleoceanografía y Paleoclimas, Instituto de Geofísica, UNAM. This method is commonly used for marine depositional environments with high opal concentrations in the sediments (>3% by weight) (Mortlock and Froelich, 1989; Hayes et al., 2021). Samples were freeze-dried for 48 h, ground with an agate mortar and pestle, weighed into 50 mL centrifuge tubes, and then treated with 10% hydrogen peroxide (H2O2) and hydrochloric acid (HCl) to eliminate organic matter and carbonates. Alkaline extraction was performed at 85°C for 5 h using a Na2CO3 solution (2M) that leached biogenic opal from sediments (DeMaster, 1981; Hayes et al., 2021). The silica content was determined by silicon-molybdenum blue spectrophotometry at the Aquatic Bio-geochemistry laboratory, Instituto de Ciencias del Mar y Limnología, UNAM, and the absorbance was read in a spectrophotometer at 812 nm.
Barium, Ba/Al, Ba/Ca, Ba/Ti, and Ba/Fe values have been used as proxies of paleoproductivity in several environments (Dymond et al., 1992; Paytan et al., 1996; Lowery et al., 2018; Sajid et al., 2020). High Ba/Ti values have been linked to high Corg values because biogenic Ba is associated with organic matter (Dymond et al., 1992). Terrigenous elements, including Fe, Ti, Si, and K, originate mainly from the erosion of continental rocks and are useful indicators of sediment transport from land to the deep sea (Rothwell, 1989). Ti has been used as a proxy for reconstructing past hydrological changes. High concentrations of Ti indicate increased riverine discharges, while lower Ti values are linked to periods of aridity (Haug et al., 2001; Haug et al., 2003; Cheshire and Thurow, 2013; Pérez-Cruz, 2013).
Cores from holes U1545A and U1549A were scanned with a third-generation energy-dispersive Avaatech® X-ray fluorescence (XRF) core scanner (target X-ray tube: Rh) at the JR Science Operator XRF Facility at the Gulf Coast Repository of Texas A&M University. The split cores were covered with 4-μm-thick SPEX SamplePrep Ultralene foil to avoid contamination. Scans were conducted at different voltages to determine a range of elements: 10 kV (Al to Fe), with a beam current of 0.160 mA and a count time of 6 s; 30 kV (Co. to Zr) with a beam current of 1.250 mA and a count time of 6 s, and 50 kV (Ba), with a beam current of 0.750 mA and a count time of 10 s. X-ray spectra were processed with the bAxil software from BrightSpec, at a resolution of 2 cm in Hole U1545A and 5 cm in Hole U1549A.
2.4 Statistical data management
For uncertainties, we used quadratic propagation of all potential uncertainty sources (Ellison and Williams, 2012). These included sample mass, blank variability, and absorbance variability for opal. We analyzed four samples, two with high and two with low opal concentrations, each with six replicates. Uncertainties were propagated through all calculation steps, from sample preparation to final calculation. The Ba/Ti data uncertainties were calculated by analyzing 15 replicates of the certified international standard SARM-4 (Cantillo, 1992). This standard assessed the uncertainty of the data measurements using the Avaatech® XRF core scanner. SARM-4 was measured at 10 kV and 50 kV for Ti and Ba, respectively.
2.5 Opal comparison and interpolation
The opal comparison was performed for the last 16,500 cal yr BP, considering the overlapping timeframe of both holes, U1545A (n = 50) and U1549A (n = 27). To enable a direct comparison, linear interpolation was applied to both datasets to homogenize sample resolutions, ages, and variations in sedimentation rates, resulting in two curves with 77 data points. Once interpolated, we subtracted the opal values from Hole U1549A from those of Hole U1545A. This resulted in a new curve representing the opal differences.
3 Results
3.1 Hole U1545A
The opal records in Hole U1545A reveal notable variations (Figure 4A). Between ∼31,200 and ∼26,500 cal yr BP, the record exhibited significant fluctuations, with an average opal content of 32.2%. A peak of 46.5% was observed at ∼31,000 cal yr BP. High opal values were recorded between ∼30,000 and ∼28,000 cal yr BP, followed by an overall declining trend during this period. From ∼26,500 to ∼19,000 cal yr BP, the opal content was relatively lower than during the preceding interval, averaging 26.9%. Despite this, the period included high opal values, reaching around 38% at ∼24,750 cal yr BP. This period exhibited the most pronounced fluctuations in the entire record. The lowest opal value across the whole record, 13.8%, was recorded at ∼25,200 cal yr BP, highlighting the high variability during this period. Between ∼19,000 and ∼11,700 cal yr BP, the opal content fluctuated from 23.7% to 39.2%, averaging 33.5%. During this period, the opal increased from ∼18,500 to ∼17,000 cal yr BP, followed by a decline between ∼17,000 and ∼16,000 cal yr BP. The opal displayed a steady and consistent decrease from ∼16,000 to ∼11,000 cal yr BP.
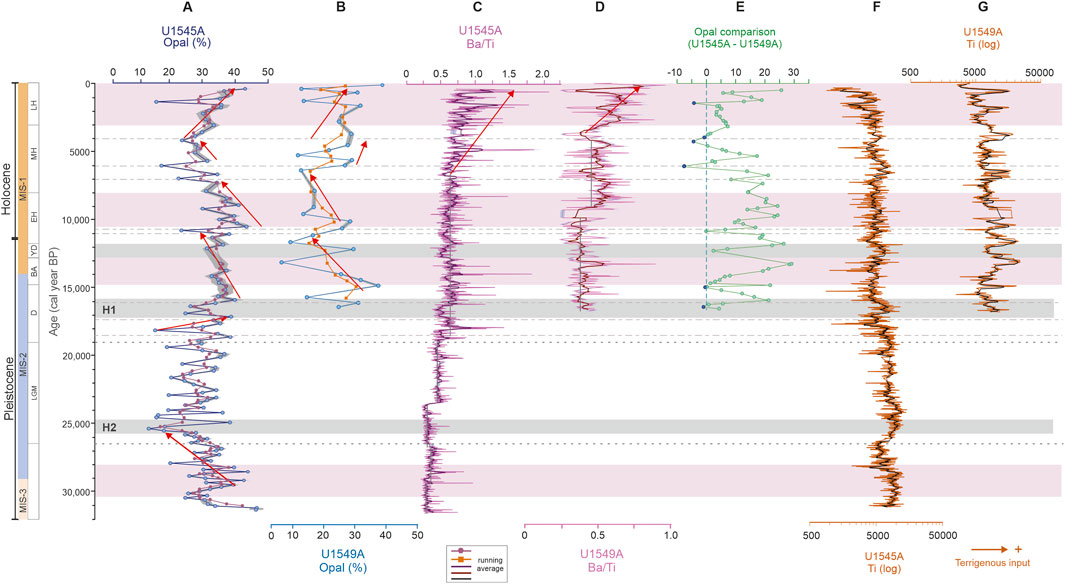
Figure 4. (A) Opal records (%) (dark blue) and uncertainties (gray) in Hole U1545A. Three-point running average (purple). (B) Opal records (%) (blue) and uncertainties (gray) in Hole U1549A. Three-point running average (orange). (C) Ba/Ti (log) in Hole U1545A (magenta), ten-point moving average (dark purple), and uncertainties (gray). (D) Ba/Ti (log) in Hole U1549A (lilac), ten-point moving average (dark red), and uncertainties (gray). (E) Opal comparison between holes U1545A (northwestern sector of GB) and U1549A (central GB). Intervals where opal content is higher in Holes U1545A (green dots) and U1549A (blue dots). Titanium log, a proxy for terrigenous supply (in orange) with a ten-point running average in black for (F) Hole U1545A and (G) Hole U1549A. Gray stripes identify Heinrich events and YD.
The opal content declined from ∼10,500 to ∼4,200 cal yr BP with an average of 33.4%. The highest values during this interval were recorded at ∼10,500 and ∼10,300 cal yr BP, reaching 43.4% and 40.5%, respectively. In contrast, the lowest values occurred at ∼7,000 and ∼6,000, dropping to 22.8% and 17.6%, respectively. This period was characterized by a gradual decrease in opal content, punctuated by these peaks and troughs. The opal content generally increased from ∼4,200 to ∼500 cal yr BP. However, there is a notable minimum peak of 15.6% at ∼1,400 cal yr BP.
The Ba/Ti record from Hole U1545A (Figure 4C) exhibits a series of distinct temporal variations and step-like changes. The lowest values occurred between ∼31,200 and ∼23,000 cal yr BP, with a minor increase observed from ∼28,500 to ∼26,500 cal yr BP. Following this interval, Ba/Ti values steadily rose from ∼23,000 to ∼18,000 cal yr BP. This upward trend persisted from ∼18,000 cal yr BP to ∼7,000 calyr BP, characterized by significant fluctuations and episodic peaks. Notably, two intervals of high values were observed from ∼14,700 to ∼11,700 cal yr BP and from ∼10,000 to ∼7,000 cal yr BP. From ∼7,000 to ∼500 cal yr BP, the Ba/Ti values increased, punctuated by episodic events characterized by high values. This period further covered the overall upward trend observed in the record.
3.2 Hole U1549A
The opal content record for Hole U1549A exhibits a trend similar to that observed in Hole U1545A (Figures 4A,B). Between ∼16,700 and ∼11,700 cal yr BP, the opal content showed wide fluctuations, averaging around 24%. The highest values were recorded at ∼ 14,900 and ∼14,500 cal yr BP, reaching 37.5% and 31.2%, respectively. At ∼11,700 cal yr BP, opal content decreased sharply to 9.2%. Opal content increased from ∼11,000 to ∼10,000 cal yr BP, followed by a declining trend from ∼9,600 to ∼6,500 cal yr BP. From 6,500 to the present, opal values gradually increased, indicating a recovery in biogenic silica deposition.
The Ba/Ti record from sediment cores in Hole U1549A reveals distinct trends. Between ∼16,700 and ∼9,200 cal yr BP, Ba/Ti values exhibit moderate fluctuations. The values slightly increase from ∼9,200 to ∼4,500 cal yr BP. This is followed by a decline in Ba/Ti from ∼4,500 to ∼3,000 cal yr BP. From ∼3,000 to ∼130 cal yr BP, the record displays an upward trend characterized by several prominent peaks (Figure 4D).
Based on quadratic error propagation analysis, both holes had low opal and Ba/Ti uncertainties (below 10%). Specifically, for Hole U1545A, opal uncertainties ranged from 3.8% to 7%, and for Hole U1549A, they ranged from 3.3% to 4.5%. For the Ba/Ti ratio, the uncertainties were 6.1% in both holes. The data’s low uncertainty values ensured their robustness and reliability, making them suitable for use in paleoceanographic and paleoclimatic reconstructions.
3.3 Opal comparison between holes U1545A and U1549A
Opal concentration records were higher in Hole U1545A, on the western side of the GB, than in Hole U1549A, central GB, over the past ∼16,700 years, except during episodic events at the end of the Deglaciation and the middle and late Holocene (Figure 4E). On average, opal concentrations were >11% higher in Hole U1545A, reaching maximum values up to 29% higher during the beginning of MIS-1 (Bølling–Allerød).
3.4 Ti records
The Ti record in Hole 1545A suggests a more significant terrigenous input into the Guaymas Basin via fluvial during the late Pleistocene than during the Holocene (Figure 4F). A decrease in terrigenous material is observed between ∼28,000 and ∼26,500 cal yr BP, followed by a significant increase during cold periods such as the LGM, Heinrich Event 2 (H2), and Heinrich Event 1 (H1). The Ti record from Hole U1549A displayed broader fluctuations throughout the sequence, with a slight decreasing trend toward the Holocene (Figure 4G).
4 Discussion
Our results indicate that bio-opal exported productivity in the GB sediments has changed over the past 31,200 years, highlighting the impacts of physical processes that drive nutrient enrichment, including mesoscale eddies, wind-driven upwelling, and shifts in climate variability that fluctuate on orbital, millennial, and centennial time scales. Variations in productivity between holes may arise from the differing interactions of these processes in both regions. Seven distinct conditions were observed on the millennial scale: ∼31,200 to ∼26,500 cal yr BP (late MIS-3 to early MIS-2), ∼26,500 to ∼19,000 cal yr BP (LGM), ∼19,000 to ∼11,700 cal yr BP (Deglaciation, BA, YD), ∼11,700 to ∼10,500 cal yr BP (the onset of the Holocene), ∼10,500-4,200 cal yr BP (early and middle Holocene), and between ∼4,200 and ∼130 cal yr BP (late Holocene).
In Hole U1545A, during the late MIS-3-MIS-2, wide fluctuations were observed from ∼31,200 to ∼26,500 cal yr BP. Productivity was higher around the beginning of MIS-2 (Figure 5C), and high productivity levels persisted until 26,000 cal yr BP. We suggest that enhanced wind-driven upwelling fostered nutrient availability and increased productivity during this interval. The migration of the ITCZ during this period, which was positioned further south (Leduc et al., 2009), resulted in winter-like conditions with intensified northwestern winds. The laminated sediments found in the cores of Hole U1545A during this period show seasonal changes (Teske et al., 2021), which could indicate that active monsoon conditions were presented and played a role in the observed high productivity patterns. Other studies in the area have reported similar productivity conditions (Barron et al., 2014; Pichevin et al., 2014). These results are a consequence of the conditions that occur during the winter formation of the Gulf of California Water (Velázquez-Aguilar et al., 2024) and when wind-driven upwelling along the eastern margin of the Gulf intensifies.
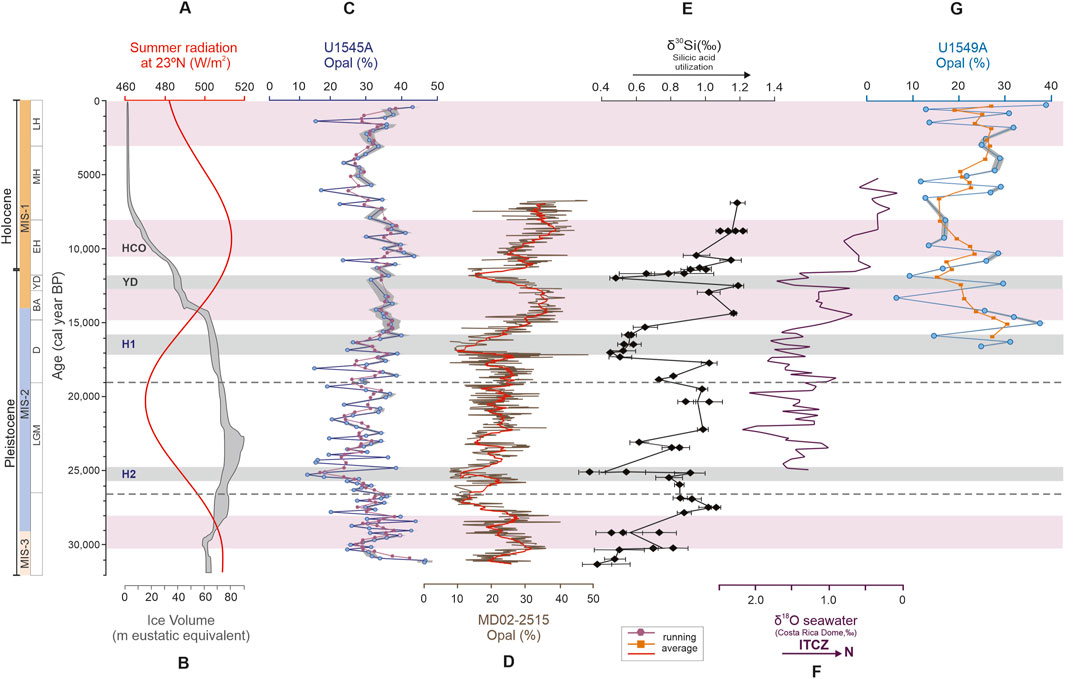
Figure 5. (A) Summer solar radiation at 23°N (W/m2) (Berger and Loutre, 1991). (B) Ice volume over North America represented by eustatic sea-level equivalents in meters (Hughes et al., 2013). (C) Opal records (%) in Hole U1545A in blue, with a three-point running average in purple and uncertainty in gray. (D) Opal record (%) for site MD02-2515 displayed in gray, with a running average in red (Pichevin et al., 2012). (E) δ30Si (‰) record as a proxy for silicic acid utilization (Pichevin et al., 2012; Pichevin et al., 2014). (F) δ18O in the Costa Rica Dome as a proxy for ITCZ position (Leduc et al., 2009). (G) Opal records (%) in Hole U1549A in blue, with a three-point running average in orange and uncertainty represented in gray.
The opal record from Hole 1545A exhibits pronounced fluctuations and a slight decline in average productivity during LGM between ∼26,500 and ∼19,000 cal yr BP compared to the previous interval, as indicated by the opal content average. We propose that the combined effects of low insolation in the Northern Hemisphere (Berger and Loutre, 1991; Figure 5A), expanded North American ice volume (Figure 5B), the southern extension of polar ice sheets, and a likely weakening and southern migration of the NPH (Lora et al., 2017; Leduc et al., 2009), may have reduced the influence of the NPH over GB during this period, leading to significant productivity fluctuations. At certain intervals, this likely resulted in weaker winds, diminished upwelling and mesoscale eddies, enhanced precipitation and riverine discharges (Cheshire et al., 2005; Cheshire and Thurow, 2013), and the formation of less saline waters (Velázquez-Aguilar et al., 2024). These changes may have intensified the stratification and limited the vertical nutrient flux in the GB, ultimately affecting productivity. Notably, the bio-opal record from U1545A had parallel trends from MD02-2515 (Pichevin et al., 2012) (Figure 5D), with opal minima coinciding with Heinrich events H2 and H1. The lowest productivity in our record occurred during H2. Furthermore, these intervals correlate with increased terrigenous input in Hole 1545A (Figure 4F), likely linked to high riverine discharge that may have impacted the ∂30Si (Figure 5E) during this period.
Conditions in the GB differ from those recorded during the LGM off Oregon, where productivity increased (Lopes et al., 2015). We attribute this to a southward displacement of the North Pacific Subpolar Gyre (NPSG), which enhanced upwelling along the Oregon margin. The expanded and intensified NPSG, driven by a southward shift of midlatitude westerlies and increased wind stress from polar easterlies (Gray et al., 2020), amplified upwelling along the northern gyre margin (Oregon margin). This gyre reorganization reflects broader LGM atmospheric shifts, including the southern migration of the NPH pressure system (Lora et al., 2017). Therefore, the GB experienced changes in circulation patterns, altered upwelling dynamics, and modified nutrient distribution.
Our records from both holes in the northwestern (U1545A) and the central (U1549A) sectors in GB showed similar trends from ∼17,000 to ∼11,700 cal yr BP. However, the opal content in Hole U1545A remains high, particularly during the Bølling–Allerød. Low productivity was observed at ∼16,800 cal yr BP and ∼12,900 to ∼11,700 cal yr BP, corresponding to H1 and the YD. Barron et al. (2014) also observed this decrease in silica burial, which correlated with low ratios of Si to organic carbon (Si/Corg) and δ30Si (Pichevin et al., 2014) (Figure 5E). In both holes, productivity is relatively higher than in the previous period (Figures 4A,B). We propose that the formation of nearby mesoscale eddies in the northwestern basin facilitated nutrient transport, increasing productivity in that region. During this time, ITCZ shifted southward (Figure 5F; Leduc et al., 2009), while climate conditions contributed to the gradual movement of the NPH to around 30°N (Gardner et al., 1997; Cheshire and Thurow, 2013) and the retreat of the polar cell (Cheshire and Thurow, 2013). The consistent increase in SST over the ETNP (Seo et al., 2016; Ford et al., 2018) and the Eastern Pacific Warm Pool (Benway et al., 2006) promotes the strengthening of both the poleward MCC and CRCC, similar to what is observed today during the summer months in the GoC. This, in turn, enhances the formation and intensification of mesoscale eddies (Lavín et al., 2013) and their influence along the GoC, thereby boosting productivity in the northwestern GB (Figure 5C).
Productivity declined slightly, in steps, from ∼10,500 to ∼4,200 cal yr BP. However, our records also show high productivity from ∼10,500 to ∼10,300 cal yr BP during the HCO (Figure 5C). We suggest that intensified wind-driven upwelling and eddies at the onset of the Holocene enhanced nutrient transport and distribution, contributing to increased productivity. These conditions are likely associated with summer insolation in the Northern Hemisphere reaching a maximum at 10,000 cal yr BP (Berger and Loutre, 1991), the contraction of the atmospheric polar cell, driven by higher insolation (Douglas et al., 2007; Cheshire and Thurow, 2013), the strong influence of the Hadley and Ferrel cells and the more northerly mean position of the ITCZ followed by its gradual southern migration (Koutavas and Lynch-Stieglitz, 2004; Leduc et al., 2009). Such atmospheric conditions may have intensified the northwestern winds, increased evaporation, and further enhanced wind-driven upwelling along the eastern margin of the GoC and the formation of the Gulf of California Water (Velázquez-Aguilar et al., 2024). Additionally, the strength of NPH and the northward migration of the ITCZ (McClymont et al., 2012) could have influenced the poleward movements of the MCC and the CRCC. This, in turn, may have contributed to the intensification of mesoscale eddies and their influence along the GoC, further fostering high opal content in the GB northwestern region (Figure 5C), as the mesoscale eddies facilitated the redistribution of phytoplankton debris from coastal upwelling areas.
Our records reveal inverse productivity trends between regions during the middle Holocene, from ∼7,000 cal yr BP to ∼4,200 cal yr BP, characterized by a decline in productivity in the NW area but a marked increase in the central basin (Figure 5G). This is evidenced by a comparison of the opal records in both holes (Figure 4E), where productivity was slightly higher in Hole U1549A. The conditions may have been influenced primarily by enhanced winter-spring coastal wind-driven upwellings on the eastern side of the Gulf. This increase in upwelling promoted the siliceous productivity in that area. Coastal wind-driven upwellings occur on the east margin during winter and spring due to local winds (Badan-Dangon et al., 1991); in contrast, the western side experiences weaker upwellings and less intense eddies. Similar conditions were suggested between ∼6,200 and ∼5,400 cal yr BP (Barron et al., 2005; Pride et al., 2010).
From ∼4,200 cal yr BP to the present, our records show a consistent pattern of increased productivity. Insolation levels are low during this period, and the ITCZ is positioned further south. Some studies suggest that the NPH gradually transitioned toward present conditions (Gardner et al., 1997; Barron and Bukry, 2007), resulting in northwestern winds and wind-driven upwelling in the eastern margin. Additionally, warmer SST in the ETNP (Benway et al., 2006) may have strengthened the poleward CRCC and MCC, as indicated by the presence of Tropical Surface Water in the basin (Velázquez-Aguilar et al., 2024). This likely led to the formation of strong eddies that could transport nutrient-rich water as they drifted away into the gulf.
In Hole U1545A, located in the northwestern basin, Ba/Ti values gradually increased from 31,000 to 18,000 cal yr BP. Both holes (U1545A and I1545A) showed a moderate synchronous rise in values from 18,000 to 11,000 cal yr BP, which continued in Hole 1545A until the early Holocene. A distinct pattern emerges in the early Holocene, as Hole U1549 in the central basin records peak Ba/Ti values, with both sites then displaying a gradually increasing trend from the middle to late Holocene.
The sedimentary Ba/Ti values in both holes generally revealed lower values during the cold glacial period and higher values during the interglacial period. This suggests reduced BioBa accumulation and less organic matter export from the surface during cold climate conditions, as indicated in other Pacific regions (Narita et al., 2002; Jaccard et al., 2005). However, variations at millennial and centennial time scales of the Ba/Ti in the sediments of the GB may have been attributed to enhanced preservation of organic matter due to low O2 without necessarily higher productivity.
5 Conclusion
This study discusses how oceanic and atmospheric processes have modulated the productivity in the GB over the last 31,200 years and the mechanisms that may have influenced climate and productivity linked to changes in the summer insolation in the Northern Hemisphere owing to shifts in the tilt and precession, the shifts of the NPH and the ITCZ, and the circulation patterns of the ETNP.
The productivity GB, known for being one of the most productive areas in the world, is likely related both to local processes associated with the dynamics of the Pacific Ocean and to regional and global climate variability. It is a complex system involving many mechanisms and factors in varying oceanic and climatic conditions.
High productivity is particularly pronounced at the end of MIS-3, HCO, and the late Holocene. This increase in productivity is associated with the prevalence of mesoscale eddies and wind-driven upwellings, which enhance nutrient distribution and likely impact the NW region. The opal records suggest that eddies have significantly influenced this area. Meanwhile, wind-driven upwellings along the eastern margin likely impacted the central region more. We observed a slight decline in productivity during the LGM and a decrease during H2, H1, and YD.
Our findings show that local productivity varies with physical process interactions. The oceanographic conditions and biological productivity in the GB are governed by the Eastern Tropical Pacific circulation through synergistic mechanisms: atmospheric forcing, where trade winds and their northward extension modulate the NPH; intensifying wind-driven upwelling and nutrient supply; mesoscale eddies that redistribute nutrients (adding spatiotemporal complexity); and biochemical processes, such as the presence of transient Fe limitation, which is superimposed on the basin’s dynamics.
Determining which process might be the most significant or whether the observed patterns result from their combined actions remains challenging. Future research should focus on detailed multidisciplinary oceanographic studies to enable substantial comparisons with fossil records and provide constraints into past and present oceanic dynamics. Findings underscore the key role of dynamic physical processes in shaping long-term productivity patterns in GoC marginal basins.
Data availability statement
The datasets presented in this study can be found in online repositories. The names of the repository/repositories and accession number(s) can be found below: https://doi.pangaea.de/10.1594/PANGAEA.974742.
Author contributions
AA-C: Formal Analysis, Methodology, Software, Writing – original draft, Writing – review and editing. LP-C: Conceptualization, Funding acquisition, Investigation, Writing – original draft, Writing – review and editing. JU-F: Funding acquisition, Resources, Writing – review and editing. MM-G: Writing – review and editing. MM-I: Writing – review and editing. MV-A: Writing – review and editing. RV-F: Writing – review and editing. J-AS-C: Formal analysis, Writing – review, and editing. AS: Writing – review and editing. TH: Writing – review and editing.
Funding
The author(s) declare that financial support was received for the research and/or publication of this article. This study was financially supported by the IICEAC Project 418908. LP-C acknowledges DGAPA PAPIIT, UNAM Project Number IN116623, for the partial financial support for this research. AA-C acknowledges the Posgrado en Ciencias del Mar y Limnología, UNAM, and the financial support provided by the Consejo Nacional de Humanidades, Ciencias y Tecnologías (CONAHCYT) for the PhD grant no. 491392.
Acknowledgments
This research used samples and data from the International Ocean Discovery Program (IODP). We thank the shipboard scientists, the IODP technical staff, and the R/V JOIDES Resolution crew for recovering the cores and for their invaluable assistance during the IODP Expedition 385. We sincerely thank the reviewers, Cristina Lopes, Fabienne Marret, and Alberto Sánchez-González, for their insightful critiques, valuable recommendations, and constructive suggestions, which greatly improved the quality of the manuscript and the interpretation of the data. We thank Marysol Valdez Hernández for her assistance in creating the figures used in this article.
Conflict of interest
The authors declare that the research was conducted in the absence of any commercial or financial relationships that could be construed as a potential conflict of interest.
Generative AI statement
The authors declare that no Generative AI was used in the creation of this manuscript.
Publisher’s note
All claims expressed in this article are solely those of the authors and do not necessarily represent those of their affiliated organizations, or those of the publisher, the editors and the reviewers. Any product that may be evaluated in this article, or claim that may be made by its manufacturer, is not guaranteed or endorsed by the publisher.
Abbreviations
GB, Guaymas Basin; GoC, Gulf of California; IODP, International Ocean Discovery Program; NPH, North Pacific High-Pressure system; ETNPO, Eastern Tropical North Pacific Ocean; ITCZ, Intertropical Convergence Zone; MCC, Mexican Coastal Current; CRCC, Costa Rica Coastal Current; SST, Sea Surface Temperature; MIS, Marine Isotope Stage; LGM, Last Glacial Maximum; HCO, Holocene Climate Optimum; UNAM, Universidad Nacional Autónoma de México; XRF, X-ray fluorescence; cal yr BP, Calibrated Years Before the Present; YD, Younger Dryas.
References
Acevedo-Acosta, J. D., and Martínez-López, A. (2022). Anomalously low diatom fluxes during 2009–2010 at alfonso basin, gulf of California. Prog. Oceanogr. 206, 102837. doi:10.1016/j.pocean.2022.102837
Aiello, I. W., Höfig, T. W., Riboulleau, A., Teske, A. P., Lizarralde, D., Ash, J. L., et al. (2024). Mineralization kinetics of biosiliceous sediments in hot subseafloors. Geochim. Cosmochim. Acta. 380, 71–82. doi:10.1016/j.gca.2024.07.005
Álvarez-Borrego, S. (2012). Phytoplankton biomass and production in the gulf of California: a review. Bot. Mar. 55 (2), 119–128. doi:10.1515/bot.2011.105
Amador, J. A., Alfaro, E. J., Lizano, O. G., and Magaña, V. O. (2006). Atmospheric forcing of the eastern tropical pacific: a review. Prog. Oceanogr. 69 (2–4), 101–142. doi:10.1016/j.pocean.2006.03.007
Badan-Dangon, A., Dorman, C. E., Merrifield, M. A., and Winant, C. D. (1991). The lower atmosphere over the gulf of California. J. Geophys. Res. Oceans 96 (C9), 16877–16896. doi:10.1029/91JC01433
Badan-Dangon, A., Koblinsky, C., and Baumgartner, T. (1985). Spring and summer in the gulf of California - observations of surface thermal patterns. Oceanol. Acta. 8 (1), 13–22. Available online at: https://www.semanticscholar.org/paper/Spring-and-summer-in-the-gulf-of-california-of-Badan-Dangon-Koblinsky/91318f7e94a8f6c89b6d94860c8ea8f3570dc1d3.
Barbara, L., Schmidt, S., Urrutia-Fucugauchi, J., and Pérez-Cruz, L. (2016). Fuerte River floods, an overlooked source of terrigenous sediment to the Gulf of California. Cont. Shelf Res. 128, 1–9. doi:10.1016/j.csr.2016.09.006
Barron, J. A., and Bukry, D. (2007). Development of the California current during the past 12,000 Yr based on diatoms and silicoflagellates. Palaeogeogr. Palaeoclimatol. Palaeoecol. 248 (3), 313–338. doi:10.1016/j.palaeo.2006.12.009
Barron, J. A., Bukry, D., and Cheshire, H. (2014). Response of diatom and silicoflagellate assemblages in the central gulf of California to regional climate change during the past 55 kyrs. Mar. Micropaleontol. 108, 28–40. doi:10.1016/j.marmicro.2014.02.004
Barron, J. A., Bukry, D., and Dean, W. E. (2005). Paleoceanographic history of the Guaymas Basin, gulf of California, during the past 15,000 Years based on diatoms, silicoflagellates, and biogenic sediments. Mar. Micropaleontol. 56 (3–4), 81–102. doi:10.1016/j.marmicro.2005.04.001
Baumgartner, T. R., Ferreira-Bartrina, V., and Moreno-Hentz, P. (1991). “Varve formation in the central Gulf of California: a reconsideration of the origin of the dark laminae from the 20th century varve record,” in The gulf and peninsular province of the californias. Editors. J. P. Dauphin, and B. R. T. Simoneit, American Association of Petroleum Geologists.
Bauska, T. K., Marcott, S. A., and Brook, E. J. (2021). Abrupt changes in the global carbon cycle during the last glacial period. Nat. Geosci. 14 (2), 91–96. doi:10.1038/s41561-020-00680-2
Benway, H. M., Mix, A. C., Haley, B. A., and Klinkhammer, G. P. (2006). Eastern pacific warm Pool paleosalinity and climate variability: 0–30 kyr. Paleoceanography 21 (3), 2005PA001208. doi:10.1029/2005PA001208
Berger, A., and Loutre, M. F. (1991). Insolation values for the climate of the last 10 million years. Quat. Sci. Rev. 10 (4), 297–317. doi:10.1016/0277-3791(91)90033-Q
Blaauw, M., Christen, J. A., and Aquino-Lopez, M. (2011). Rbacon: age-depth modelling using bayesian statistics. R. package version 2.5.8. Available online at: https://CRAN.R-project.org/package=rbacon (Accessed February, 2024).
Bordoni, S., Ciesielski, P. E., Johnson, R. H., McNoldy, B. D., and Stevens, B. (2004). The low-level circulation of the North American monsoon as revealed by QuikSCAT. Geophys. Res. Lett. 31 (10), 2004GL020009. doi:10.1029/2004GL020009
Brumsack, H. J. (1989). Geochemistry of recent TOC-rich sediments from the gulf of California and the black sea. Geol. Rundsch. 78 (3), 851–882. doi:10.1007/BF01829327
Calvert, S. E. (1966). Accumulation of diatomaceous silica in the sediments of the gulf of California. Geol. Soc. Am. Bull. 77 (6), 569. doi:10.1130/0016-7606(1966)77[569:aodsit]2.0.co;2
Cantillo, A. Y. (1992). Standard and reference materials for marine science. NOAA. Available online at: https://repository.library.noaa.gov/view/noaa/2881.
Cheshire, H., and Thurow, J. (2013). High-resolution migration history of the Subtropical High/Trade Wind system of the northeastern Pacific during the last ∼55 years: implications for glacial atmospheric reorganization. Paleoceanography 28 (2), 319–333. doi:10.1002/palo.20031
Cheshire, H., Thurow, J., and Nederbragt, A. J. (2005). Late quaternary climate change record from two long sediment cores from Guaymas Basin, gulf of California. J. Quat. Sci. 20 (5), 457–469. doi:10.1002/jqs.944
DeMaster, D. J. (1981). The supply and accumulation of silica in the marine environment. Geochim. Cosmochim. Acta. 45 (10), 1715–1732. doi:10.1016/0016-7037(81)90006-5
Douglas, R., Gonzalez-Yajimovich, O., Ledesma-Vazquez, J., and Staines-Urias, F. (2007). Climate forcing, primary production and the distribution of Holocene biogenic sediments in the gulf of California. Quat. Sci. Rev. 26 (1–2), 115–129. doi:10.1016/j.quascirev.2006.05.003
Dufois, F., Hardman-Mountford, N. J., Greenwood, J., Richardson, A. J., Feng, M., and Matear, R. J. (2016). Anticyclonic eddies are more productive than cyclonic eddies in subtropical gyres because of winter mixing. Sci. Adv. 2 (5), e1600282. doi:10.1126/sciadv.1600282
Dymond, J., Suess, E., and Lyle, M. (1992). Barium in deep-sea sediment: a geochemical proxy for paleoproductivity. Paleoceanography 7 (2), 163–181. doi:10.1029/92PA00181
Ellison, S. L. R., and Williams, A. (2012). “Eurachem/CITAC guide CG 4”: Quantifying uncertainty in analytical measurement. Available online at: https://repository.oceanbestpractices.org/handle/11329/1449.
Espinosa-Carreón, T. L., and Escobedo-Urías, D. (2017). South region of the gulf of California large marine ecosystem upwelling, fluxes of CO2 and nutrients. Environ. Dev. 22, 42–51. doi:10.1016/j.envdev.2017.03.005
Fernández-Barajas, M. E., Monreal-Gómez, M. A., and Molina-Cruz, A. (1994). Thermohaline structure and geostrophic flow in the gulf of California, during 1992. Cien. Mar. 20 (2), 267–286. doi:10.7773/cm.v20i2.958
Ford, H. L., McChesney, C. L., Hertzberg, J. E., and McManus, J. F. (2018). A deep eastern equatorial pacific thermocline during the last glacial maximum. Geophys. Res. Lett. 45 (21). doi:10.1029/2018GL079710
García-Morales, R., López-Martínez, J., Valdez-Holguin, J., Herrera-Cervantes, H., and Espinosa-Chaurand, L. (2017). Environmental variability and oceanographic dynamics of the central and southern coastal zone of Sonora in the gulf of California. Remote Sens. 9 (9), 925. doi:10.3390/rs9090925
Gardner, J. V., Dean, W. E., and Dartnell, P. (1997). Biogenic sedimentation beneath the California current system for the past 30 kyr and its paleoceanographic significance. Paleoceanography 12 (2), 207–225. doi:10.1029/96PA03567
Gaxiola-Castro, G., Álvarez-Borrego, S., Lavín, M. F., Zirino, A., and Nájera-Martínez, S. (1999). Spatial variability of the photosynthetic parameters and biomass of the gulf of California phytoplankton. J. Plankton Res. 21 (2), 1–13. doi:10.1093/plankt/21.2.231
Goodfriend, G. A., and Flessa, K. W. (1997). Radiocarbon reservoir ages in the gulf of California: roles of upwelling and flow from the Colorado river. Radiocarbon 39 (2), 139–148. doi:10.1017/S0033822200051985
Gray, W. R., Wills, R. C. J., Rae, J. W. B., Burke, A., Ivanovic, R. F., Roberts, W. H. G., et al. (2020). Wind-driven evolution of the North Pacific subpolar gyre over the last deglaciation. Geophys. Res. Lett. 47, e2019GL086328. doi:10.1029/2019GL086328
Haug, G. H., Gunther, D., Peterson, L. C., Sigman, D. M., Hughen, K. A., and Aeschlimann, B. (2003). Climate and the collapse of Maya civilization. Science 299 (5613), 1731–1735. doi:10.1126/science.1080444
Haug, G. H., Hughen, K. A., Sigman, D. M., Peterson, L. C., and Rohl, U. (2001). Southward migration of the intertropical convergence zone through the Holocene. Science 293 (5533), 1304–1308. doi:10.1126/science.1059725
Hayes, C. T., Costa, K. M., Anderson, R. F., Calvo, E., Chase, Z., Demina, L. L., et al. (2021). Global Ocean sediment composition and burial flux in the deep sea. Glob. Biogeochem. Cycles 35 (4), e2020GB006769. doi:10.1029/2020GB006769
Heaton, T. J., Butzin, P. K. M., Bard, E., Reimer, R. W., Austin, W. E. N., Ramsey, C. B., et al. (2020). Marine20—the marine radiocarbon age calibration curve (0–55,000 cal BP). Radiocarbon 62 (4), 779–820. doi:10.1017/RDC.2020.68
Hughes, Ph. D., Gibbard, Ph. L., and Ehlers, J. (2013). Timing of glaciation during the last glacial cycle: evaluating the concept of a global ‘last glacial maximum’ (LGM). Earth Sci. Rev. 125, 171–198. doi:10.1016/j.earscirev.2013.07.003
Jaccard, S. L., Haug, G. H., Sigman, D. M., Pedersen, T. F., Thierstein, H. R., and Röhl, U. (2005). Glacial/interglacial changes in subarctic North Pacific stratification. Science 308 (5724), 1003–1006. doi:10.1126/science.1108696
Jiang, H.-B., Hutchins, D. A., Zhang, H.-R., Feng, Y.-Y., Zhang, R.-F., Sun, W.-W., et al. (2024). Complexities of regulating climate by promoting marine primary production with ocean iron fertilization. Earth Sci. Rev. 249, 104675. doi:10.1016/j.earscirev.2024.104675
Kessler, W. S. (2006). The circulation of the eastern tropical pacific: a review. Prog. Oceanogr. 69 (2–4), 181–217. doi:10.1016/j.pocean.2006.03.009
Koutavas, A., and Lynch-Stieglitz, J. (2004). “Variability of the marine ITCZ over the eastern pacific during the past 30,000 years,” in The Hadley circulation: present, past and future. Editors H. F. Diaz, and R. S. Bradley (Dordrecht: Springer Netherlands), 21, 347–369. doi:10.1007/978-1-4020-2944-8_13
Lavín, M. F., Beier, E., and Badan, A. (1997). “Estructura Hidrográfica y Circulación del Golfo de California: Escalas Estacional e Interanual,” in Contribuciones a la Oceanografía Física en México. Editor M. F. Lavín, México: Unión Geofísica Mexicana, 141–171.
Lavín, M. F., Castro, R., Beier, E., Cabrera, C., Godínez, V. M., and Amador-Buenrostro, A. (2014). Surface circulation in the gulf of California in summer from surface drifters and satellite images (2004–2006). J. Geophy. Res. Oceans 119 (7), 4278–4290. doi:10.1002/2013JC009345
Lavín, M. F., Castro, R., Beier, E., and Godínez, V. M. (2013). Mesoscale eddies in the southern gulf of California during summer: characteristics and interaction with the wind stress. J. Geophy.l Res. Oceans 118 (3), 1367–1381. doi:10.1002/jgrc.20132
Lavín, M. F., and Marinone, S. G. (2003). “An overview of the physical oceanography of the gulf of California,” in Nonlinear processes in geophysical fluid dynamics. Editors O. U. Velasco Fuentes, J. Sheinbaum, and J. Ochoa (Dordrecht: Springer Netherlands), 173–204. doi:10.1007/978-94-010-0074-1_11
Leduc, G., Vidal, L., Tachikawa, K., and Bard, E. (2009). ITCZ rather than ENSO signature for abrupt climate changes across the tropical pacific? Quat. Res. 72 (1), 123–131. doi:10.1016/j.yqres.2009.03.006
Lisiecki, L. E., and Raymo, M. E. (2005). A pliocene-pleistocene stack of 57 globally distributed benthic δ18O records. Paleoceanography 20 (1), 2004PA001071. doi:10.1029/2004PA001071
Lizarralde, D., Axen, G. J., Brown, H. E., Fletcher, J. M., González-Fernández, A., Harding, A. J., et al. (2007). Variation in styles of rifting in the gulf of California. Nature 448 (7152), 466–469. doi:10.1038/nature06035
Lizarralde, D., Teske, A., Höfig, T. W., and González-Fernández, A.IODP Expedition 385 Scientists (2023). Carbon released by sill intrusion into young sediments measured through scientific drilling. Geol 51 (4), 329–333. doi:10.1130/G50665.1
Lluch-Cota, S. E. (2000). Coastal upwelling in the eastern gulf of California. Oceanol. Acta 23 (6), 731–740. doi:10.1016/S0399-1784(00)00121-3
Lopes, C., Kucera, M., and Mix, A. C. (2015). Climate change decouples oceanic primary and export productivity and organic carbon burial. PNAS 112 (2), 332–335. doi:10.1073/pnas.1410480111
López-Martínez, J., Farach Espinoza, E. B., Herrera Cervantes, H., and García Morales, R. (2023). Long-term variability in Sea Surface temperature and chlorophyll a concentration in the gulf of California. Remote Sens. 15 (16), 4088. doi:10.3390/rs15164088
Lora, J. M., Mitchell, J. L., Risi, C., and Tripati, A. E. (2017). North pacific atmospheric rivers and their influence on western North America at the last glacial maximum. Geophys. Res. Lett. 44 (2), 1051–1059. doi:10.1002/2016GL071541
Lowery, Ch. M., Bralower, T. J., Owens, J. D., Rodríguez-Tovar, F. J., Jones, H., Smit, J., et al. (2018). Rapid recovery of life at ground zero of the end-cretaceous mass extinction. Nature 558 (7709), 288–291. doi:10.1038/s41586-018-0163-6
Marinone, S. G. (2003). A three-dimensional model of the mean and seasonal circulation of the gulf of California. J. Geophys. Res. Oceans 108 (C10), 2002JC001720. doi:10.1029/2002JC001720
McClymont, E. L., Ganeshram, R. S., Pichevin, L. E., Talbot, H. M., Van Dongen, B. E., Thunell, R. C., et al. (2012). Sea-Surface temperature records of termination 1 in the gulf of California: challenges for Seasonal and interannual analogues of tropical pacific climate change. Paleoceanography 27 (2), 2011PA002226. doi:10.1029/2011PA002226
Mercado-Santana, J. A., Santamaría-del-Ángel, E., González-Silvera, A., Sánchez-Velasco, L., Gracia-Escobar, M. F., Millán-Núñez, R., et al. (2017). Productivity in the gulf of California large marine ecosystem. Environ. Dev. 22, 18–29. doi:10.1016/j.envdev.2017.01.003
Merino, M., and Monreal-Gómez, M. A. (2009). “Ocean currents and their impact on marine life marine ecology,” in Encyclopedia of life support systems (EOLSS), developed under the auspices of the UNESCO (Oxford: Eolss Publishers), 1, 47–52.
Miller, N. C., and Lizarralde, D. (2013). Thick evaporites and early rifting in the Guaymas Basin, gulf of California. Geol 41 (2), 283–286. doi:10.1130/G33747.1
Mortlock, R. A., and Froelich, Ph. N. (1989). A simple method for the rapid determination of biogenic opal in pelagic marine sediments. Deep. Sea. Res. A 36 (9), 1415–1426. doi:10.1016/0198-0149(89)90092-7
Narita, H., Sato, M., Tsunogai, S., Murayama, M., Ikehara, M., Nakatsuka, T., et al. (2002). Biogenic opal indicating less productive northwestern North Pacific during the glacial ages. Geophys. Res. Lett. 29 (15), 22–31. doi:10.1029/2001GL014320
Palacios-Hernández, E., Beier, E., Lavín, M. F., and Ripa, P. (2002). The effect of the seasonal variation of stratification on the circulation of the northern gulf of California. J. Phys. Oceanogr. 32 (3), 705–728. doi:10.1175/1520-0485(2002)032<0705:teotsv>2.0.co;2
Paytan, A., Kastner, M., and Chavez, F. P. (1996). Glacial to interglacial fluctuations in productivity in the equatorial pacific as indicated by marine barite. Science 274 (5291), 1355–1357. doi:10.1126/science.274.5291.1355
Pegau, W. S., Boss, E., and Martínez, A. (2002). Ocean color observations of eddies during the summer in the gulf of California. Geophys. Res. Lett. 29 (9), 6. doi:10.1029/2001GL014076
Pérez-Cruz, L. (2013). Hydrological changes and paleoproductivity in the gulf of California during middle and late Holocene and their relationship with ITCZ and north American monsoon variability. Quat. Res. 79 (2), 138–151. doi:10.1016/j.yqres.2012.11.007
Persad, L. D., and Marsaglia, K. M. (2023). Data report: detailed lithologic columns for IODP expedition 385 and DSDP leg 64 sites in the Guaymas Basin, gulf of California, Mexico. Proc. Int. Ocean. Discov. Program Exped. Rep. 385 (202). doi:10.14379/iodp.proc.385.202.2023
Pichevin, L., Ganeshram, R. S., Reynolds, B. C., Prahl, F., Pedersen, Th. F., Thunell, R., et al. (2012). Silicic acid biogeochemistry in the gulf of California: insights from sedimentary Si isotopes. Paleoceanography 27 (2), 2011PA002237. doi:10.1029/2011PA002237
Pichevin, L. E., Ganeshram, R. S., Geibert, W., Thunell, R., and Hinton, R. (2014). Silica burial enhanced by iron limitation in oceanic upwelling margins. Nat. Geosci. 7 (7), 541–546. doi:10.1038/ngeo2181
Pike, J., and Kemp, A. E. S. (1997). Early Holocene decadal-scale ocean variability recorded in gulf of California laminated sediments. Paleoceanography 12 (2), 227–238. doi:10.1029/96PA03132
Price, A. M., Mertens, K. N., Pospelova, V., Pedersen, T. F., Raja, S., and Ganeshram, R. S. (2013). Late quaternary climatic and oceanographic changes in the northeast pacific as recorded by dinoflagellate cysts from Guaymas Basin, gulf of California (Mexico). Paleoceanography 28 (1), 200–212. doi:10.1002/palo.20019
Pride, C., Thunell, R., Sigman, D., Keigwin, L., Altabet, M., and Tappa, E. (2010). Nitrogen isotopic variations in the Gulf of California since the Last Deglaciation: response to global climate change. Paleoceanography 14 (3), 397–409. doi:10.1029/1999PA900004
Ragueneau, O., Leynaert, A., Tréguer, P., DeMaster, D. J., and Anderson, R. F. (1996). Opal studied as a marker of paleoproductivity. Eos, Trans. AGU. 77 (49), 491. doi:10.1029/96EO00325
Ragueneau, O., Tréguer, P., Leynaert, A., Anderson, R. F., Brzezinski, M. A., DeMaster, D. J., et al. (2000). A review of the Si cycle in the modern ocean: recent progress and missing gaps in the application of biogenic opal as a paleoproductivity proxy. Glob. Planet. Change 26 (4), 317–365. doi:10.1016/S0921-8181(00)00052-7
R Core Team (2020). R: a language and environment for statistical computing. Vienna: R Foundation for Statistical Computing. Available online at: https://www.R-project.org/.
Ripa, P. (1997). Toward a physical explanation of the seasonal dynamics and thermodynamics of the gulf of California. J. Phys. Oceanogr. 27 (5), 597–614. doi:10.1175/1520-0485(1997)027<0597:tapeot>2.0.co;2
Rothwell, R. G. (1989). Minerals and mineraloids in marine sediments: an optical identification guide. Springer Science & Business Media.
Sajid, Z., Ismail, M. S., Zakariah, M. N. A., Tsegab, H., Gámez Vintaned, J. A., Hanif, T., et al. (2020). Impact of paleosalinity, paleoredox, paleoproductivity/preservation on the organic matter enrichment in black shales from triassic turbidites of semanggol basin, peninsular Malaysia. Minerals 10 (10), 915. doi:10.3390/min10100915
Sancetta, C. (1995). Diatoms in the gulf of California: seasonal flux patterns and the sediment record for the last 15,000 years. Paleoceanography 10 (1), 67–84. doi:10.1029/94PA02796
Santamaría-del-Angel, E., Álvarez-Borrego, S., and Müller-Karger, F. E. (1994). The 1982–1984 el niño in the gulf of California as seen in coastal zone color scanner imagery. J. Geophys. Res. Oceans 99 (C4), 7423–7431. doi:10.1029/93JC02147
Seewald, J. S., Wheat, C. G., Reeves, E. P., Tivey, M. K., Sievert, S. M., Stakes, D., et al. (2024). Spatial evolution and temporal stability of hydrothermal processes at sediment-covered spreading centers: constraints from Guaymas Basin, gulf of California. Geochim. Cosmochim. Acta. 367, 87–106. doi:10.1016/j.gca.2023.12.006
Seo, I., Lee, Y., Lee, Y. I., Yoo, C. M., and Hyeong, K. (2016). Climatic evolution of the central equatorial pacific since the last glacial maximum. Geochem. Geophys. Geosyst. 17 (8), 3454–3468. doi:10.1002/2016GC006371
Teske, A., Lizarralde, D., Höfig, T. W., Aiello, I. W., Ash, J. L., and Bojanova, D. P. (2021). “The expedition 385 scientists, Guaymas Basin tectonics and biosphere,” in Proceedings of the International Ocean Discovery Program. Editors A. Teske, D. Lizarralde, and T. W. Höfig (College Station, TX: International Ocean Discovery Program) 385. Site U1545. doi:10.14379/iodp.proc.385.106.2021
Teske, A., McKay, L. J., Ravelo, A.Ch., Aiello, I., Mortera, C., Núñez-Useche, F., et al. (2019). Characteristics and evolution of sill-driven off-Axis hydrothermalism in Guaymas Basin—the ringvent site. Sci. Rep. 9 (1), 13847. doi:10.1038/s41598-019-50200-5
Thunell, R. C. (1998). Seasonal and annual variability in particle fluxes in the gulf of California: a response to climate forcing. Deep-Sea Res. I Oceanogr. Res. Pap. 45 (12), 2059–2083. doi:10.1016/S0967-0637(98)00053-3
Thunell, R. C., Pride, C. J., Tappa, E., and Muller-Karger, F. E. (1994). Biogenic silica fluxes and accumulation rates in the gulf of California. Geol 22 (4), 303–306. doi:10.1130/0091-7613(1994)022<0303:bsfaar>2.3.co;2
Velázquez-Aguilar, M., Pérez-Cruz, L., Urrutia-Fucugauchi, J., Marsaglia, K. M., Coria-Monter, E., Monreal-Gómez, M. A., et al. (2024). Evolution of ocean circulation and water masses in the Guaymas Basin (gulf of California) during the last 31,000 Years revealed by radiolarians and silicoflagellates in IODP expedition 385 sediment cores. Front. Earth Sci. 12, 1301999. doi:10.3389/feart.2024.1301999
Venkatachalam, S., Ansorge, I. J., Mendes, A., Melato, L. I., Matcher, G. F., and Dorrington, R. A. (2017). A pivotal role for ocean eddies in the distribution of microbial communities across the antarctic circumpolar current. PLoS One 12 (8), e0183400. doi:10.1371/journal.pone.0183400
Keywords: biogenic opal, Ba/Ti, wind-driven upwellings, mesoscale eddies, eastern tropical north pacific ocean, climate
Citation: Aldama-Cervantes A, Pérez-Cruz L, Urrutia-Fucugauchi J, Monreal-Gómez MA, Merino-Ibarra M, Velázquez-Aguilar M, Venegas-Ferrer R, Sanchez-Cabeza JA, Sifeddine A and Höfig TW (2025) Advancing knowledge on the paleoproductivity and climate in the Guaymas Basin, Gulf of California, over the past 31,200 years: geochemical proxies in IODP expedition 385 sediments. Front. Earth Sci. 13:1568130. doi: 10.3389/feart.2025.1568130
Received: 28 January 2025; Accepted: 21 April 2025;
Published: 12 May 2025.
Edited by:
Teresa Drago, Portuguese Institute for the Sea and Atmosphere, PortugalReviewed by:
Alberto Sánchez-González, National Polytechnic Institute (IPN), MexicoFabienne Marret, University of Liverpool, United Kingdom
Cristina Lopes, Portuguese Institute for Sea and Atmosphere (IPMA), Portugal
Copyright © 2025 Aldama-Cervantes, Pérez-Cruz, Urrutia-Fucugauchi, Monreal-Gómez, Merino-Ibarra, Velázquez-Aguilar, Venegas-Ferrer, Sanchez-Cabeza, Sifeddine and Höfig. This is an open-access article distributed under the terms of the Creative Commons Attribution License (CC BY). The use, distribution or reproduction in other forums is permitted, provided the original author(s) and the copyright owner(s) are credited and that the original publication in this journal is cited, in accordance with accepted academic practice. No use, distribution or reproduction is permitted which does not comply with these terms.
*Correspondence: Ligia Pérez-Cruz, cGVyZXpjcnV6QGlnZW9maXNpY2EudW5hbS5teA==
†Present address: Tobias W. Höfig, Project Management Julich, Julich Research Centre GmbH, Rostock, Germany