- 1GFZ, Helmholtz Centre for Geosciences, Potsdam, Germany
- 2Department of Earth Sciences, Freie Universität Berlin, Berlin, Germany
- 3Aix Marseille Université, Université de Toulon, CNRS, IRD, MIO, Marseille, France
- 4Department of Environmental Science, Aarhus University, Roskilde, Denmark
In recent decades, a growing body of research has focused on characterizing supraglacial microbial communities. These studies have unveiled that pigmented glacier ice algae, namely, Ancylonema nordenskiöldii and Ancylonema alaskanum, blooming during the summer melt seasons accelerate surface melting. Most techniques that quantify microbial processes on glacial surfaces require the melting of samples prior to their analysis and, so far, the structure and three-dimensional arrangement of microorganisms that bloom on bare ice surfaces have never been characterized. To address this gap, we developed, tested and validated a first characterization workflow for imaging the microorganisms colonizing the frozen ice surfaces on the Greenland Ice Sheet. While preserving their frozen state, we employed a suite of microscopic tools with progressively increasing resolution, starting from imaging algae on ice with hand-held, portable microscopes (up to ×150 magnification) all the way to high-resolution cryo-scanning electron microscopy (cryo-SEM, up to ×5.000 magnification). Our images provided us with the first visualization of the distribution, structure, interactions, and relationship between microbes, minerals, and their frozen substrate. Our innovative approach significantly advances the understanding of glacial microbial life within the ice matrix, shedding light on the spatial architecture of microbial cells in their on-ice state, thereby improving how we perceive and study these unique ecosystems.
1 Introduction
Microbial communities thriving on glacier surfaces become highly active during the summer melt season when they play a crucial role in increasing the absorption of solar radiation by decreasing the albedo of snow and ice surfaces, thus enhancing melting (e.g., Yallop et al., 2012; Lutz et al., 2016; Stibal et al., 2017; Ryan et al., 2018; Cook et al., 2020). Research about this significant impact has provided us with insights into the microbial community compositions and dynamics through sequencing or biomass and nutrient flux quantification (e.g., Yallop et al., 2012; Lutz et al., 2014; Lutz et al., 2018; Anesio et al., 2017; Holland et al., 2019; Williamson et al., 2021). Bare ice surfaces, once snow free, darken further due to the proliferation of glacier ice algae, a phenomenon that is particularly striking in the melt zone on the southwest margin of the Greenland Ice Sheet (GrIS), an area often referred to as the ‘Dark Zone’ (Box et al., 2012; Ryan et al., 2018; Cook et al., 2020; Feng et al., 2024). Glacier ice algae have been identified as the mostly unicellular Ancylonema alaskanum, formerly Mesotaenium berggrenii (Remias et al., 2009; Procházková et al., 2021) and the filamentous Ancylonema nordenskiöldii (Lutz et al., 2018; Hoham and Remias, 2020; Williamson et al., 2020). These species are notable for the large amounts of purple to dark brown phenolic pigments, primarily purpurogallin derivatives, that they accumulate and that protects them from potential damage by excess solar radiation (Remias et al., 2012; Yallop et al., 2012; Halbach et al., 2022a; Halbach et al., 2022b, Chevrollier et al., 2023; Leya et al., 2009), with the additional benefit of contributing to meltwater formation around the cells by dissipating heat from absorbed sunlight (Dial et al., 2018; Williamson et al., 2020). These pigments may also play additional biological roles, such as acting as antioxidants or contributing to microbial interactions within the ice matrix (Duval et al., 1999). Beyond algae, supraglacial ice habitats are teeming with a variety of other microeukaryotes, as well as bacteria, archaea, fungi, viruses, nematodes and higher organisms (e.g., Stibal et al., 2006; Lutz et al., 2015a; Edwards et al., 2013a; Anesio et al., 2017; Hotaling et al., 2021; Perini et al., 2019). In addition to biological communities, a range of light-absorbing particles (LAPs) such as mineral dust deposited on glaciers darken bare ice surfaces and can serve as an important nutrient source for microbial communities (e.g., Takeuchi, 2002; Dong et al., 2009; McCutcheon et al., 2021; McCutcheon et al., 2024; Wientjes et al., 2011; Dumont et al., 2014).
To study these complex communities, researchers have evaluated the microbial cell shapes or life cycle states through optical or fluorescence microscopy (e.g., Lutz et al., 2015b; Hoham and Remias, 2020), or by analysing their microbial diversity or functions via amplicon or metagenome sequencing (e.g., Edwards et al., 2013b; Zarsky et al., 2013; Lutz et al., 2018; Jaarsma et al., 2023; Remias et al., 2023). Furthermore, new insights were also gained by quantifying the associated mineral dust compositions via X-ray diffraction (e.g., Wientjes et al., 2011; Nagatsuka et al., 2014; Lutz et al., 2015a; McCutcheon et al., 2021) or analysing the aqueous compositions of the ice matrix through nutrient or trace metal concentration quantifications (e.g., Stibal et al., 2009; Telling et al., 2011; Lutz et al., 2015a; Holland et al., 2019; McCutcheon et al., 2021). The activity and interspecific relationships in glacier microbial communities were also recently assessed through fluorescence microscopic imaging of DAPI-stained (Nicholes, 2020) or BONCAT-treated samples (Bradley et al., 2022). These studies have described close associations between snow and glacier ice algae and bacteria, with bacteria frequently adhering to the algal cell walls (Nicholes, 2020), or between bacteria and fungi, where fungi likely acted as an end of season control for the blooms (Nicholes, 2020). Intriguingly, imaging revealed that healthier algal cells tended to be surrounded by fewer bacteria, hinting at a complex relationship between these organisms in their ice matrix (Nicholes, 2020). Imaging of cells has been done with light microscopy and several studies have also employed scanning electron microscopy (SEM) to image fixed snow and glacier ice algae cells (e.g., Weiss, 1983; Lutz et al., 2014; Fiołka et al., 2021; Perini et al., 2023). Despite the plethora of information gained about these algae and their associated communities or habitats, in all cases the original state on the snow or ice and its microbial communities was invariably lost, because samples were melted and often fixed to be subsequently re-deposited on a carrier used for microscopic imaging. Imaging of fixed or unfixed samples by conventional SEM has the additional drawback that it happens under vacuum conditions, which often results in distorted images with shrunken algal cells or cells with collapsed walls. In consequence, none of the above-mentioned microscopic studies could evaluate the microorganisms as they are present in/on the ice. Although protocols for collecting, preserving, imaging and analysing the mineral and chemical compositions of particles in many meter-long frozen ice cores or mm sized ice pieces have been developed (e.g., Weikusat et al., 2017 and references therein; Stoll et al., 2022; Bohleber et al., 2024; Faria et al., 2010), such protocols do not exist for imaging or analysing µm-sized microbes and minerals in sub-cm sized samples from surface ice environments.
From an ecological perspective, such a structural analysis of microbial communities thriving on terrestrial ice surfaces is critical, as spatial proximity and arrangements are key factors for assessing inter-specific interactions. For instance, bacterial cells tend to cluster more densely around primary producers (algae in our study), creating a gradient of cell concentration (Kuzyakov and Razavi, 2019) as it is the case in the rhizosphere and the phycosphere (Fujiwara et al., 2013; Seymour et al., 2017). Furthermore, production of organic acids or extracellular polymeric substances (EPS) (Nagar et al., 2021) through various cellular processes (Rousk and Bengtson, 2014; Wadham et al., 2019) facilitate coherence and binding to the minerals in the ice matrix, microbial adhesion, biofilm formation, and protection against environmental stressors (Dohnalkova et al., 2011). The microbial activity in this mineral-ice matrix further enhances the extraction of nutrients by increasing mineral solubilization (e.g., McCutcheon et al., 2021). Thus, observing microbes as well as ice crystals and minerals in their natural, in situ frozen state at a resolution where they can all be distinguished from each other is paramount. Yet, so far, we lack even the most essential insights into (a) the structural arrangement of microbial communities in situ on and within the frozen ice matrix and (b) the biotic and abiotic interactions occurring in this system.
To address this knowledge gap, we have developed a visualisation protocol for microbes and minerals on/in natural ice samples with the aim to: (i) establish and optimize a cryo-microscopic workflow tailored for surface ice samples, ensuring methodological robustness from field observations to laboratory high-resolution cryo-SEM imaging; (ii) analyze and compare images obtained from each observational technique to understand their applicability to diverse field and laboratory needs; (iii) investigate the spatial organization and potential ecological interactions between microbial communities inhabiting ice surfaces, to reveal whether microbes preferentially associate with specific minerals, organic matter, or ice structures.
2 Methods
We developed, tested and employed a workflow for the visualisation of microbial communities and minerals in/on natural ice samples from the southern margin of the Greenland Ice Sheet. The workflow includes on-ice in situ observations, collection, preservation, transport and cold-room light microscopy as well as cryo-SEM imaging (Figure 1). To highlight the advantages of our cryo-workflow, we compared our cryo-imaging modes with conventional SEM imaging of melted and refrozen samples. Note that in this study, cryo-microscopy is referring to imaging of naturally frozen ice samples, and not of samples that have been flash frozen (vitrified).
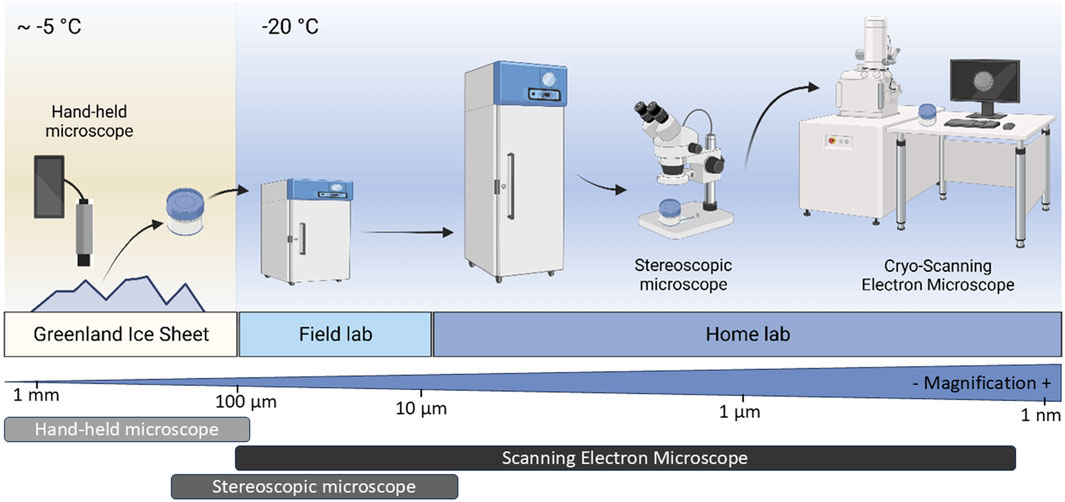
Figure 1. Multi-scale workflow for collection, preservation and cryo-imaging of ice surface samples. Figure 1 has been generated using BioRender under a Publication License, and Created in BioRender. (Mourot, 2025) https://BioRender.com/ph7nxib.
2.1 On-ice in situ imaging, sampling, sample preservation and transport
Surface dark ice was observed in situ and subsequently sampled from dark ice patches near the QAS-M weather station (PROMICE; Fausto et al., 2021) on the Southern Greenland Ice Sheet (Figure 2) in July 2020 and 2021. Imaging and subsequent sampling was done at dusk, when temperatures were lowest, and the ice sheet surface was frozen (at the time of sampling, the QAS-M surface logged temperature was 0°C). We identified an area with visible dark ice that was fully frozen and imaged the particles on the ice surface using two different hand-held, smartphone connected portable microscopes (a Ninyoon 4K and a Dino-Lite AF7915MZT microscope, both with magnification options of up to ×150). This in situ imaging helped us to identify small pieces of algae-rich ice (0.3–1 cm2) that could subsequently be carefully separated from the surface ice using clean tweezers and a chisel. The small pieces of ice were transferred into pre-cooled 30 mL glass jars that were encased inside frozen ice packs that preserved the frozen state of each sample. Once sealed the jars were double sealed in zip-lock bags and immediately transferred and stored at −20°C in a portable field freezer that was shipped to the home-laboratory in a −20°C container ship. During sampling we preserved the orientation of the ice pieces by keeping the sample containers always upright so as to allow us to evaluate positioning and orientation of biotic and abiotic particles with respect to the ice crystal surfaces. We selected −20°C as the adequate preservation, transport, storage and imaging temperature because the optimal temperature of most surface ice in Greenland lies between 0 and ∼ −30°C (Løkkegaard et al., 2023). We also collected small ice pieces that were melted and glutaraldehyde-fixed for conventional imaging. These samples were kept in the field laboratory until they were transported to the home laboratory where they were stored in a 4°C fridge until imaging at room temperature.
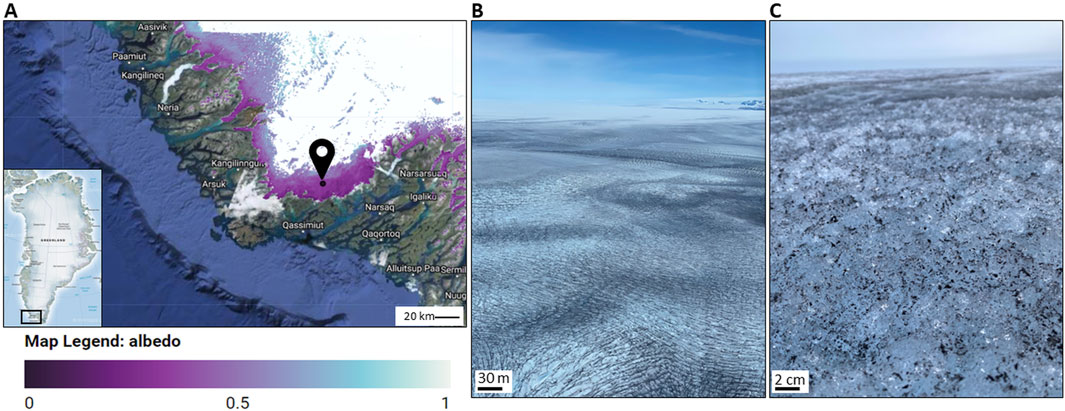
Figure 2. Sampling location on the Southern Greenland ice sheet margin (61°05′8708″N, 46°50′9442″W). (A) DeepPurple 2020/21 camp site near the PROMICE weather station QAS-M (Fausto et al., 2021), where on the 19th of July 2020 (one of the sampling days) an albedo of ∼ 0.4 was measured. Image produced using the Albedo Inspector (https://fsn1995.users.earthengine.app/view/albedoinspector) by Feng et al. (2023a), and Feng et al. (2023b), (B) Dark ice surface near the QAS-M weather station; (C) Close-up smartphone photograph of a dark particle-covered ice surface.
2.2 Cryo-imaging on a stereoscopic light microscope (light microscopy)
In the home-laboratory, all sample handling was carried out in a walk-in freezer set to −20°C. Samples were transferred from microscope to microscope in a pre-cooled, insulated Styrofoam box containing a large metal block that was cooled using liquid nitrogen (LN2). Our samples were never immersed in LN2 and we only used the LN2 vapour to preserve sample integrity. This approach also helped minimize humidity-induced water condensation and new ice deposition onto our samples. Inside the walk-in freezer, an ice piece from the field (inside a glass jars) was imaged frozen using a KERN ODC-86 camera attached to a Leica WILD M3B stereoscopic microscope and the associated Microscope VIS Pro 2.0 software (https://www.kern-sohn.com/). Samples were only observed for short lengths of time (<2 min) to avoid possible melting under the light of the stereoscopic microscope. We achieved magnifications of up 400 times with this microscope with no loss of focus. This imaging mode allowed us to compare the laboratory light microscopic images collected in the walk-in freezer with the in-field, on-ice images and to evaluate if sample integrity had been preserved. With light microscopy, due to the partial translucence of the ice crystals we could not just image the ice surfaces but also in part in the ice samples. For a comparison, we also imaged the melted and glutaraldehyde-fixed ice samples using room temperature light microscopy (Zeiss Axio A1 with a Zeiss A-plan ×20 objective) following the methods described by Lutz et al. (2014).
2.3 Cryo-scanning electron microscopy imaging (cryo-SEM)
For the highest resolution imaging (magnifications ×30 – ×5.000) of ice surface features, we used two different microscopes and transfer systems. For option 1, we transferred selected ice pieces from the glass jars onto a pre-cooled bronze stub, that was part of a home-designed transfer shuttle, using a pre-cooled polyurethane box and pre-cooled tweezers (Figure 3A). Ice samples were carefully secured with micro-screws, ensuring sample stability during imaging. Prior to adopting this method, alternative fixation techniques (e.g., freezing the sample onto the stub with a droplet of water, gentle melting and refreezing of the sample base directly on the cooled stub at −20°C, or using silver conductive paste) were tested but led to issues with compromised structural integrity of the sample. The transfer shuttle is equipped with a cover that can be closed or opened using a Quick-Loader port once mounted in the SEM (an FEI Quanta 3D SEM) room. The closed shuttle was installed into a Deben cold stage precooled to −20°C and after evacuating the microscope chamber within 3 min, the Quick-Loader port cover was removed. In a first step, low magnification cryo-SEM images were collected at 20 kV acceleration voltage in low-vacuum mode (1 mbar) using a dedicated low vacuum Everhart-Thornley detector. This allowed us to minimize ice sublimation and reduced sample damaging. Selected areas were subsequently imaged at high-vacuum but at a low acceleration voltage of 2 kV and a very low beam current of 17 pA in order to minimize charging of the uncoated samples. With this approach, our samples were preserved and could be imaged at −20°C, avoiding both temperature variations and reducing as much as possible ice sublimation.
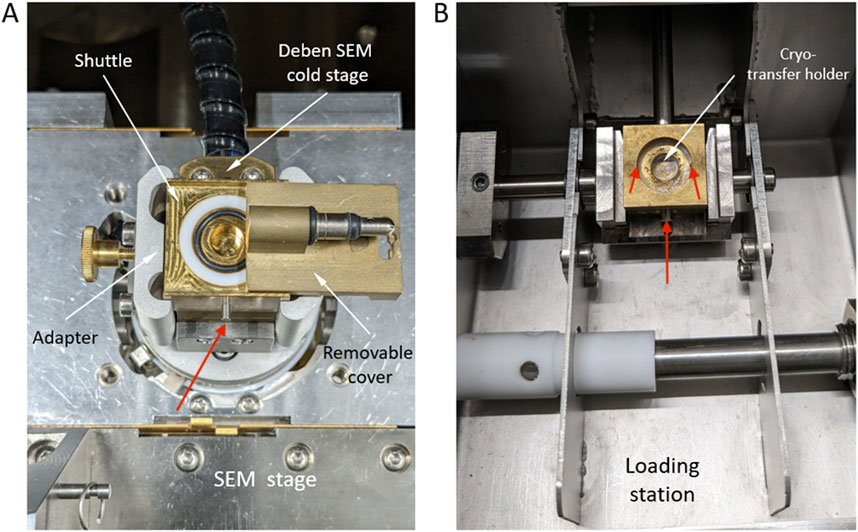
Figure 3. Cryo-transfer holder designed to preserve ice samples frozen at −20°C during their transition from the climate-controlled room to the cryo-SEM chamber, both at −20°C. (A) Cryo-transfer stage on which the ice samples to be imaged were secured through micro-screws (see red arrows) and the shuttle for the Quanta 3D SEM/FIB, (B) Cryo-transfer stage for the Zeiss Ultra Plus microscope.
For option 2, small ice samples were transferred by means of a commercial Leica VCT-100 liquid nitrogen cooled cryo-transfer system that was mounted on a Zeiss Ultra Plus SEM. This cryo-SEM was operated at 1.5 kV and at temperature of – 160°C). Again, we used a homemade stage (Figure 3B) which allowed us to fix small pieces of ice with one or several micro-screws. Due to the use of LN2 cooling, this method likely results in an alteration of the samples because of ice recrystallization and higher difficulties of avoiding moisture freezing at the sample surface during transfer. To test other possible artefacts, we also used field-fixed samples that we refroze at −20°C and subsequently imaged in cryo-mode at – 160°C on the same Zeiss Ultra Plus SEM instrument.
2.4 Conventional imaging of melted samples
We carried out conventional imaging of melted and redeposited samples from light microscopic imaging all the way to high-vacuum SEM imaging to compare with our new cryo-imaging modes. Melted surface ice samples were drop-cast onto glass slides and imaged using light microscopy (Leica WILD M3B stereoscopic microscope). A droplet of melted surface ice samples fixed with 2.5% glutaraldehyde was dispersed on an Al stub, air-dried and coated with 3 nm of carbon with a LEICA EM ACE600 vacuum coater and imaged at room temperature and under high vacuum with a conventional SEM (FEI Quanta 3D SEM, 20 keV).
3 Results
3.1 Hand-held microscopy: identifying biological and inorganic particles in situ on the ice prior to targeted sampling
Twelve different surface ice samples were imaged in situ on the ice using the hand-held microscopes. The images revealed noticeable variations in ice surface topography, with ice crystal features ranging from tens of micrometers to a few millimeters. In addition, variations in the distribution of biotic and abiotic particulates within the weathering crust were documented (Figure 4A). The ice contained particle aggregates that consisted of a mixture of what we assigned to be algae and minerals. Microscopy images revealed ice crystal surfaces that were densely covered by elongated, filamentous-like cells or aggregates of cells together with more angular particles (Figures 4B–D). This imaging enabled us to identify glacier ice algae through their characteristic rod-like and filamentous shapes and their distinct deep purple to brownish coloration. In addition, minerals were associated with these filamentous cells formed aggregates or clumps (e.g., Figure 4D, right). At the resolution of our in situ field deployable hand-held microscopes (see also Supplementary Figure S1), although the presence of filamentous glacier ice algae cells and of abiotic particles was evident, a definitive identification and a clear differentiation was not feasible. Furthermore, we often imaged aggregates that were too compact to discern any internal configurations or arrangements.
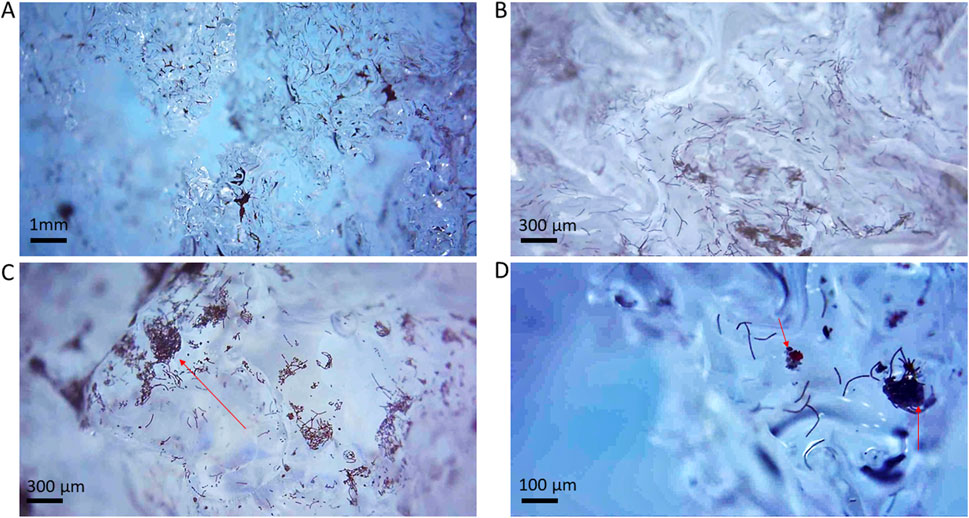
Figure 4. On-ice imaged glacier ice algae captured with a hand-held, smartphone-connected Ninyoon 4K Microscope (magnification between 10 and ∼ ×150). (A,B) Two different overviews of the ice crystal surfaces and interfaces covered by dark particles; (C) Zoomed image of the upper surface of an ice crystal covered in glacier ice algae. The red arrow designates the upper face of the ice grain, covered in glacier ice algae. (D) Close-up of an ice crystals surfaces displaying glacier ice algae and a snow algae cell (red). Red arrows indicate mineral particles. Further images are shown in Supplementary Figure S1.
3.2 Stereoscopic light microscopic imaging
The second step in our cryo-imaging workflow was the observations of the same samples in the home laboratory and using a stereoscopic microscope equilibrated at −20°C in a walk-in freezer. We evaluated potential changes in the ice surface during transport and handling. The obtained images (Figure 5) uncovered unique features of the ice surface ecosystem that were not discernible with the handheld microscopes. Notably, a considerable proportion of the glacier ice algae formed chains located on the edge of ice crystals, reaching out into the atmosphere (Figure 5; Supplementary Figure S2; Supplementary Video S1). Dense networks of filamentous algae that together formed ‘nets’ allowed entire chains of cells to remain suspended above the ice crystal surfaces were discernible (Figures 5A–C). These intricate networks appear to support larger structures, including mineral particles and in some cases binding also spherical, larger snow algae cells (Figure 5), the latter being recognizable by their spherical shapes and red colors (Figure 5C; Supplementary Figure S2). Another important observation in our light microscopic imaging was the presence of a translucent, at times slightly yellow mesh-like substance connecting these structures (Figure 5C). This seems akin to the mesh structure often described for extracellular polymeric substances (EPS, e.g., Dohnalkova et al., 2011; Neu and Lawrence, 2014) that bind cells and minerals together. However, we noted a significant difference in size, with our net-like structures that stretch up to 100-μm between anchored points.
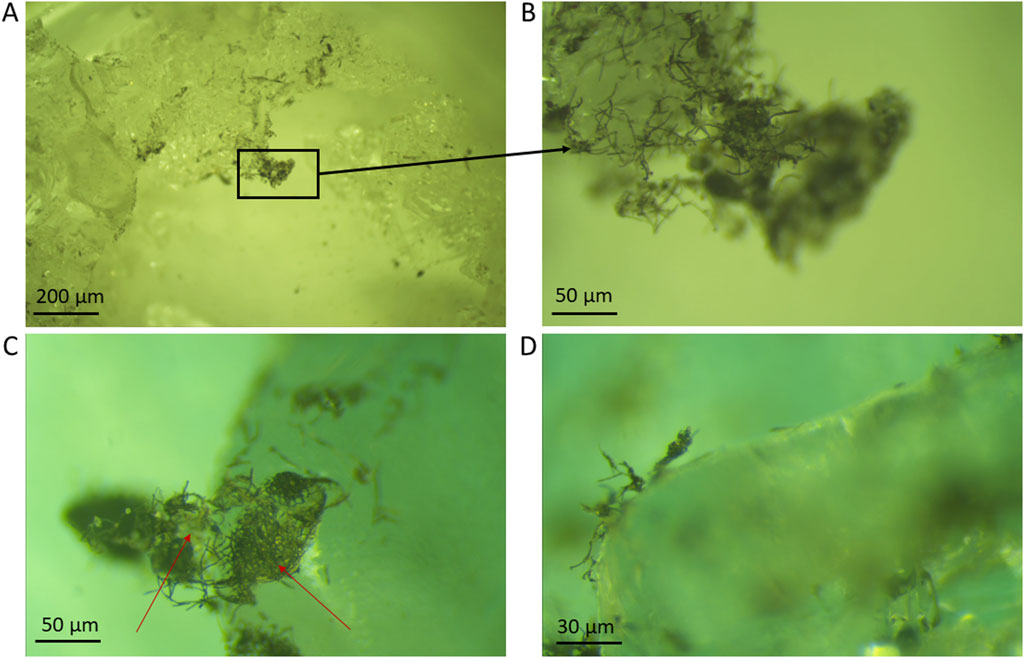
Figure 5. Surface ice samples imaged inside a climate-controlled room at −20°C using a stereoscopic microscope (magnification up to ×400). (A) Overview of edges of ice crystals covered by dark particles; (B) Detail image of the edge of the same ice crystal shown in (A), with glacier ice algae forming a net reaching out over the ice crystal’s edge. Red arrows indicate red, round cells identified as snow algae among the net. (C,D) Glacier ice algae and mineral aggregates covering and reaching over the edges of ice crystals. Red arrows in (C) indicate translucent, EPS-like material.
Despite our meticulous efforts to maintain environmental stability by setting the climate chamber to −20°C and limiting the duration of the observations with the light microscope and, thus, minimize light-induced heat exposure, some of the surfaces of ice crystals that were exposed for ∼ 4 min to constant light showed signs of partial melting (Supplementary Figure S3). This melting was attributed to the small but progressive increase in temperature of the stereoscopic microscope light source over time. Consequently, samples that showed signs of melting were excluded from further observation or from use for transfer and further imaging with the cryo-SEM. Based on these observations we suggest that continuous observation of natural ice samples using cryo-stereoscopic microscopy should be limited to maximum 2 min of continuous light exposure to avoid changes in the ice structure.
3.3 Cryo-scanning electron microscopic (cryo-SEM) imaging
Keeping the temperature of all imaged samples at −20°C, the cryo-SEM imaging enabled observation of microbes, ice crystals and mineral particles on the surfaces of our ice samples with barely any changes in our ice samples. The ice crystals were visible as smooth surfaces with rough edges, that were often covered with particles that were either biological cells - identified by their elongated or rounded shapes–or angular mineral particles (Figures 6A,B; Supplementary Figure S4). Additionally, we imaged the above inferred EPS material documented in the cryogenic stereoscopic light microscopy images (Figure 5). In the cryo-SEM images, such EPS appeared as structured made up of strings, nets, droplets and filaments (Figures 6C,D).
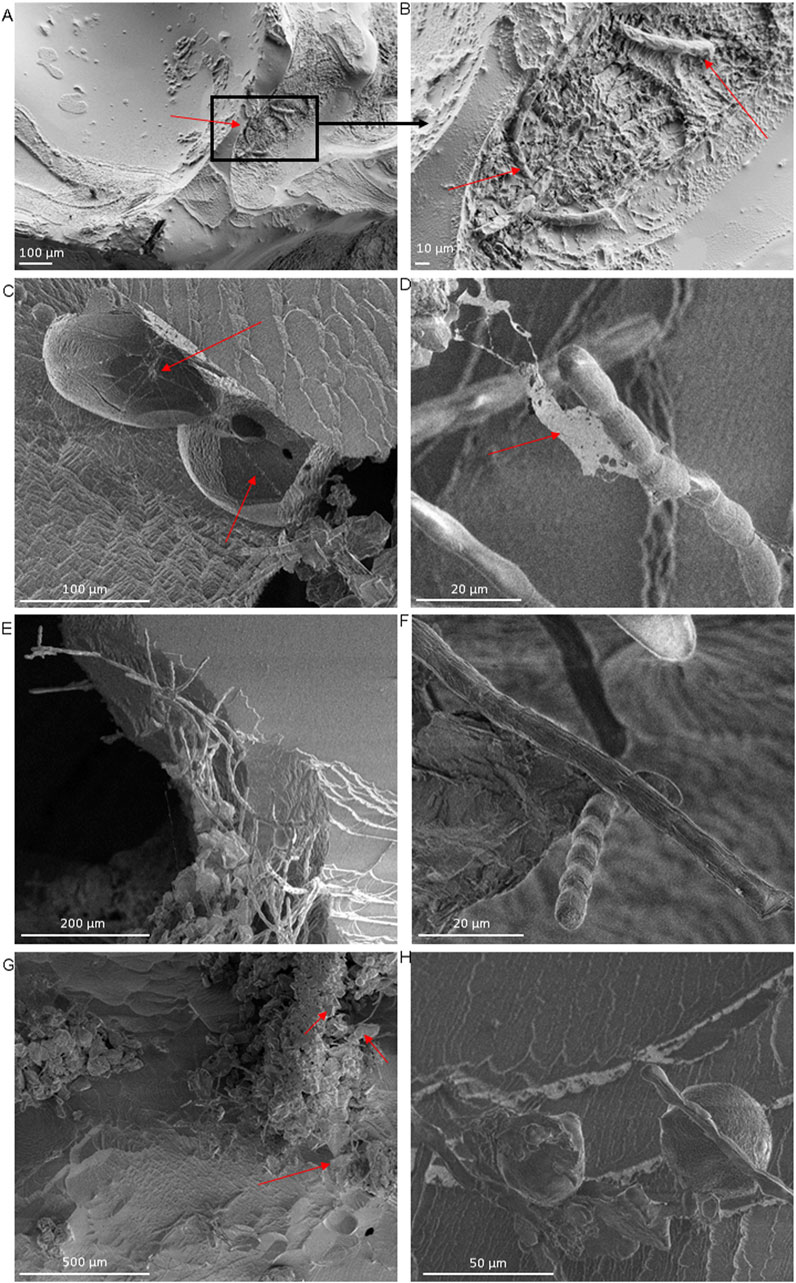
Figure 6. Surface ice samples from the Greenland ice sheet imaged using a cryo-scanning electron microscope at −20°C (magnification up to ×5.000). (A) Smooth ice crystal surfaces with sharp edges containing a mixture of cells and minerals indicated by red arrows. (B) Close up of (A) showing a microbial hotspot, with glacier ice algae indicated with red arrows; (C,D) Net-like structures, indicated using red arrows, draped over and spanning in between ice crystals and glacier ice algae; we attribute this structure to extracellular polymeric substances (EPS). (E,F) Filamentous glacier ice algae (likely Ancylonema nordenskiöldii (Remias et al., 2012)) covering and reaching over the edge of ice crystals; in E the filaments are also interspersed with small, angular mineral particles. (G) snow algae cells together with glacier ice algae filaments and minerals covering the ice surface (H) Hotspots of cellular and mineral particle aggregates indicated by red arrows on crests or in dips between ice crystals.
Cryo-SEM imaging further confirmed the prevalence of glacier ice algae along ice crystal edges (Figures 6E,F), an observation we had already noted in our on-ice in situ imaging prior to sampling (Figure 4; Supplementary Figure S1) and in the stereoscopic microscopic imaging of the returned samples (Figure 5; Supplementary Figure S2). Multiple observations of different areas in various ice samples revealed that when filamentous algal cell chains, typically comprising between five to ten individual cells, were present, only one or two cells of such a chain were directly in contact with and attached to the ice crystal surfaces. The remaining cells of a chain reached out into the void space above the ice crystals. In addition, we often observed nets formed by such filamentous chains of glacier ice algae. These nets often seem to bind mineral particles and single, round snow algal cells together (Figures 6E,G). This physical proximity among microorganisms of different taxa was a recurrent observation (Figure 6H). The main struggles we encountered during sample preparation led to frost deposition on the ice surface (Supplementary Figures S5A,B) and changes related to ice sublimation under vacuum conditions (Supplementary Figures S5C,D). To evaluate the possible beam damage to the algae due to prolonged beam exposure, we acquired images of the same algae filaments several minutes apart over a time frame of 20 min (Supplementary Figure S6) and our images demonstrate that neither the algae nor their substrate were affected.
3.4 Comparing conventional and cryo-imaging modes
Light microscopy observations of melted samples re-deposited on glass slides revealed that, although glacier ice algae were present and at times also in contact with mineral and other biological aggregates (Supplementary Figure S7A), such a co-location is likely accidental and a consequence of random re-deposition on the glass slides (Supplementary Figure S7B). In addition, and in contrast to our findings for the ice samples preserved and imaged in their native frozen state, the glacier ice algae and the minerals in the melted samples appeared mostly isolated as they lost their coherence in the melted medium. We also compared conventional SEM images of melted, fixed glacier ice surface samples with our cryo-SEM images and the results revealed that in addition to loosing co-localization, the cellular integrity was also lost, as most cell membranes of snow and glacier ice algae were affected by the high vacuum conditions of the conventional SEM (Supplementary Figures S8A,C). This is in contrary to their perfectly preserved 3D structures in our cryo-SEM workflow (Figure 6). Importantly, in the melted and re-deposited samples it was not feasible to assess the original relative localization between mineral and biological particles, as these are affected by the sample handling prior to the conventional scanning electron microscopic observations. Thus, not surprisingly, such an approach does not allow us to make any inference about the original 3D structure and arrangements of the ice surface microbial communities. Finally, we also compared/contrasted these conventional room temperature SEM images with cryo-SEM observations of such melted and glutaraldehyde-fixed samples that were subsequently refrozen at −20°C. The images (Supplementary Figure S9) of the so treated samples showed even clearer the compromised integrity of both the snow and glacier ice algae cells.
4 Discussion
We present here a workflow for the imaging of glacier ice surface samples in their natural, frozen state (Figure 7). We documented how such a workflow can be used to image microbes and minerals across different scales and how different cryo-processes for sample handling and different imaging tools can be combined for complementary cryogenic analyses. We assessed the strengths and limitations of microscopic techniques of progressively increasing magnification for imaging of ice-bound algae-rich particulates, from hand-held microscopy (Figure 4; Supplementary Figure S1; 50–150 ×) to stereoscopic light microscopy (Figure 5; Supplementary Figure S2, 400 ×), to high-resolution cryo-SEM (Figure 6; Supplementary Figure S4, 100 to 5,000 ×). Finally, we contrasted this new cryo-workflow with images obtained using a series of non-cryo imaging techniques (Supplementary Figures S7–S9).
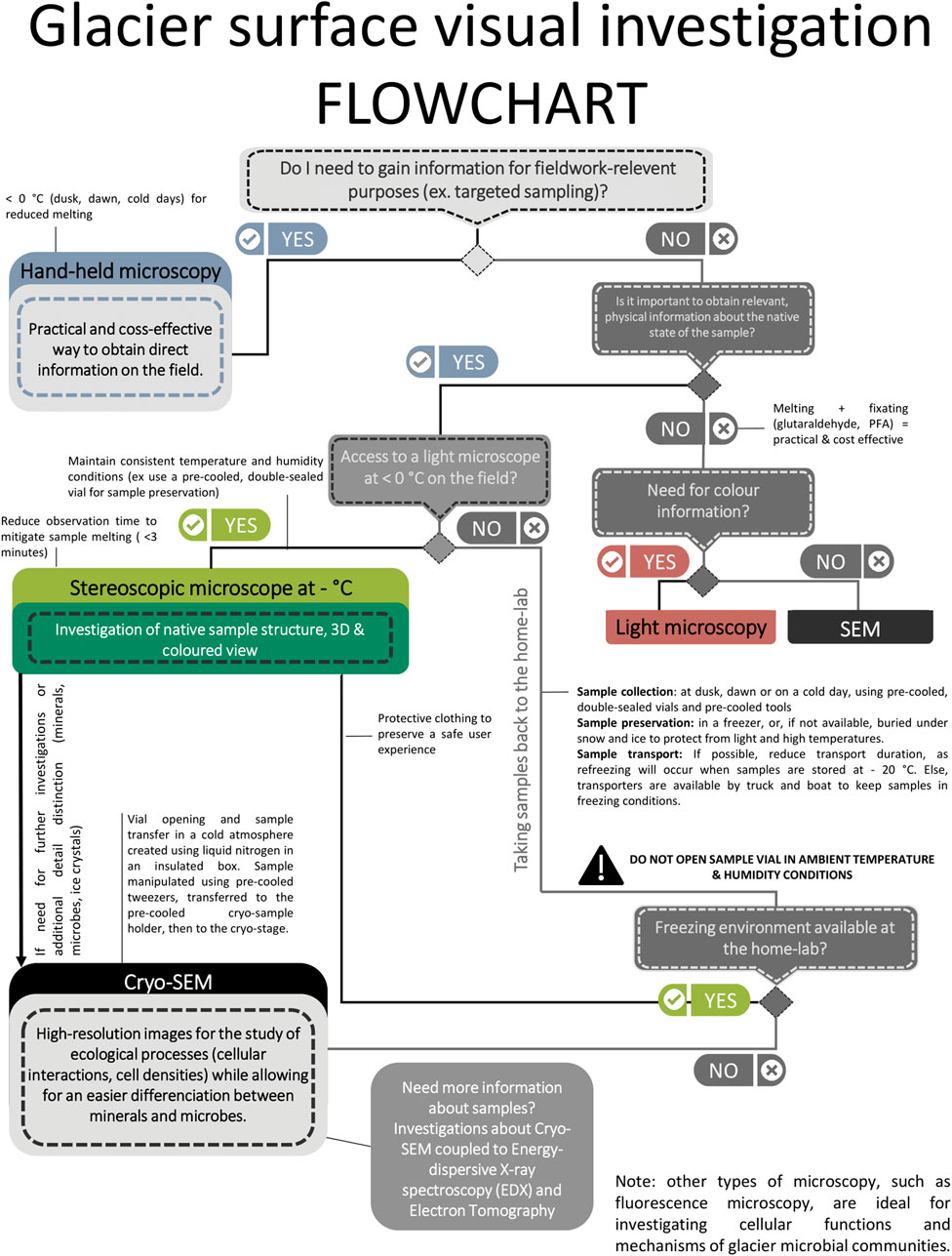
Figure 7. Multi-scale workflow for visualizing glacier microbial communities in their native state using various cryogenic microscopy techniques from the on-ice handheld microscopy in the field, through sample preservation and transport and all the way to the high resolution cryo-SEM imaging.
Important to note is that such a workflow and observations of microbes and minerals on ice surfaces are invariably also fraught with notable challenges. Prime issues among these are the need to maintain a constant temperature and humidity, because variations during transport, processing and imaging of ice samples can lead to recrystallization or new ice deposition (Supplementary Figures S5A–C; Baker, 2019). We documented that our workflow allows for samples to be preserved, transported and imaged at a constant temperature of −20°C, and that we limited variations in ambient humidity and thus preserved the integrity of our samples (Figures 4–6). It is also important to remember that when imaging natural ice sample with laboratory instruments, the time between sampling and observation is kept to a minimum. This time period, however, often depends on field logistics and safe sample transport from the remote glacier or ice sheet sampling site to the home-laboratory. Another significant challenge for imaging ice surfaces in their natural state is the need to prevent new ice or frost formation during sample transfer, in-between the field and the home laboratory and in-between imaging techniques. Our images demonstrated that we successfully used the pre-cooled, double-sealed glass jars for sample collection and transfer in a −20°C freezer to the home institution post collection. This allowed us to preserve not just the sample orientation but also to keep the temperature integrity and to minimize humidity changes, thus avoiding both melting and frost deposition. The next steps that had to be controlled was the transfer of samples in-between the various microscopic imaging instruments. For example, transferring a sample imaged inside the walk-in freezer at −20°C by light microscopy to the cryo-SEM had to also be done with no loss in sample integrity. Our images documented (Figure 6; Supplementary Figure S4) that by placing a sample onto a sealed, −20°C pre-cooled Deben cold-stage for the SEM (Figure 3), and by transporting this cold stage in a pre-cooled box to the SEM microscope allowed us to avoid melting, recrystallization, and humidity induced ice deposition. Specimen coating is not usually needed for cryo-SEM imaging, yet the absence of coating led sometimes to charging as documented through the development of brighter patches on some of the imaged ice structures (Supplementary Figures S4C, S5C,D, S6). Such charging, combined with the sensitivity of biological structures and the propensity of ice to melting due to electron impact and heating (i.e., beam induced damage), can result in loss of image quality and resolution. Nevertheless, imaging a sample under the SEM beam in cryo-mode for less than 15–20 min and careful control and maintenance of the cryo-stage temperature and of the vacuum settings are all crucial steps that prevent melting or sublimation of these specimens. Using our workflow, the ice sublimation rate was acceptable to image morphology of snow and glacier ice algae, ice crystals and mineral particles. These results when compared with the images from melted surface ice samples (Supplementary Figures S7–S9) underline the value of our proposed cryo-workflow as an innovative approach to investigate supraglacial microbial communities, preserving their native frozen state for more accurate characterization. It is to note that although the same ice samples were imaged using both stereoscopic microscopy and cryo-SEM, identical areas could not be consistently targeted due to the inherent limitations of each technique. Stereoscopic microscopy enabled the visualization of internal structures within the ice, while cryo-SEM provided high-resolution images of the sample surface, making direct correlation between specific regions challenging.
Our images document that our scale-spanning cryo-workflow works well from on-ice in situ imaging and all the way to high resolution cryo-SEM imaging. On-ice in situ imaging with a hand-held microscope directly in the field is a practical and cost-effective method (Figure 4; Supplementary Figure S1) and thus highly advisable. Such imaging does not just deliver in situ identification of the cryogenic algae and associated particles on the original surfaces, but is a pre-requisite and facilitates subsequent targeted sampling. Field microscopy allows for rapid, in situ assessments of microbial communities without the need for sample transportation or preparation, which is particularly crucial in remote glacial environments. Additionally, field-based imaging provides immediate ecological context, helping us to identify spatial distribution patterns of microbial cells relative to melt features and mineral particulates. However, field microscopy using smartphone-connected, hand-held microscopes also have limitations when imaging surface snow and ice. Their resolution is limited, they lack variable dept focus capabilities and, when temperatures are above freezing, imaging ice surfaces with such microscopes can also lead to additional melting dur to continual exposure to the bright microscope light source. Only imaging of frozen samples with cryogenic stereoscopic light microscopy (Figure 5) and even more so with cryo-electron microscopy (Figure 6) allowed us to collect detailed observations on the spatial organization of glacier ice algae, providing insights into biological, mineral, and ice matrix substrate structures. The algae observed in our study share morphological similarities with known supraglacial algal taxa such as Ancylonema nordenskiöldii, that develop under the form of filaments of assembled elongated cells and present a dark pigmentation primarily due to the presence of purpurogallin (e.g., Remias et al., 2012; Halbach et al., 2025). Genomic analyses of ice samples gathered at the same site during the same sampling campaign confirmed the dominance of Ancylonema nordenskiöldii and of A. alaskanum (Halbach et al., 2025; Rossel et al., 2025). In this way, it is possible to investigate possible ecological characteristics of glacier ice algae as they interact with other biological agents or minerals. The images acquired with the hand-held microscope as well as the stereoscopic microscope have the advantage of visualizing not just morphological features but also colours. Although both these microscopes are limited in resolution, combining observations based on algal shapes and colour helps to further evaluate habitat specific features. Nonetheless, stereoscopic microscopy with cryogenic conditions of observation has its own drawbacks, in that sample degradation can occurs after already a few minutes of light exposure (Supplementary Figure S3). In addition, this approach is far less user-friendly due to the necessity of observing samples in a −20°C walk-in freezer room. Finally, cryo-SEM imaging, although more time consuming and complicated in terms of sample handling, is the most advanced and highest resolution approach in our workflow and it can deliver excellent images and even analyses of surface ice samples containing mixed biological and non-biological particles in their native state. Combined with taxonomic knowledge of glacier ecosystems from the literature (e.g., Lutz et al., 2014; Anesio et al., 2017; Hoham and Remias, 2020; Hotaling et al., 2021), our cryo-SEM analyses enabled us to clearly distinguish between mineral and biological particles, not just based on structure and morphology but also on aggregation behaviour. It is however noteworthy that regardless of the cryo-SEM approach used, we were able to clearly differentiate not just the chains of glacier ice algae as well as spherical snow algae, but we could image the well-preserved structural arrangement between the algae, minerals, and the ice crystals substrate. Although this was outside the scope of this study, the cryo-SEM approach can also be used to verify differences in chemical compositions of the mineral types present on ice surfaces by using Energy-Dispersive X-ray (EDX) spectroscopic point analyses or mapping in cryo-mode.
The identification of EPS-like thin networks and agglomerated glacier ice algae filaments in our images (Figures 5, 6) raises the question of whether microbial life on ice forms biofilms. While the existence of biofilm has been demonstrated in other supraglacial habitats, through mostly macroscopic observations, such as cryoconite granules (Smith et al., 2016), at melting snow-ice interfaces (Lutz et al., 2014), or as macrostructures (Jaarsma et al., 2023), their presence and formation on ice surfaces remain underexplored. The documented localized and microscopic scale aggregations of microbial cells and EPS in our work (Figure 6) is relevant as it could play a role in surface adhesion and microbial resilience, yet future investigations are needed to elucidate the structural and functional dynamics of these microbial assemblages under rapidly changing glacial conditions.
We documented that combining various cryo-imaging approaches gives best images that aid our understanding the ecology of supraglacial microbial communities. The on-ice field observations allowed us to establish the presence of algal taxa on ice surface samples (Figure 4). The subsequent stereoscopic light microscopic and cryo-SEM imaging confirmed this but also revealed that the algae were preferentially located on the edges of ice crystal and most often extending away from the ice crystals into the air (Figures 5, 6). We documented that only a fraction of the algal chains was in direct contact with the ice crystals themselves. At the time of sampling (dusk, when all surfaces are frozen), most of the chains were not attached to the ice substrate but perched into the air. We cannot determine if such a partial attachment confers the glacier ice algae with any ecological advantages, such as enhanced temperature regulation, increased solar radiation capture, or effective wind dispersion (Harding et al., 2011). However, under the ‘drier’ conditions of our dusk sampling, the positioning of the algae perched on top the ice crystals may easier facilitate the aerial dispersal.
Our sampling was done at the end of the day, avoiding conditions where the weathering crust was water saturated. We captured glacier ice algae in their frozen state, providing a unique perspective on microbial spatial arrangements on glacier surfaces. However, the spatial patterning of microorganisms is highly variable during melt days, which can record more than 10 cm surface melting on the ablation zone. Although glacier ice algae were observed embedded within the frozen ice matrix at the time of sampling, their metabolic activity and nutrient uptake are likely dependent on transient liquid water films that form during melt events. In consequence, further work is needed to understand the arrangement of glacier ice algae on ice crystals through ice melting.
5 Conclusion
We have detailed pathways for three-dimensional imaging of frozen particle-rich ice samples from the field to the high resolution of a cryo-SEM microscope. We highlight the advantages and disadvantages of each approach for investigating localisation and distribution of particles and interactions between microbes, minerals and the ice substrate and tested and validated a workflow on real surface ice samples from the Greenland Ice Sheet. We showed how such multi-modal cryo-imaging approaches can significantly advance our knowledge of glacier ecosystems.
Data availability statement
The raw data supporting the conclusions of this article will be made available by the authors, without undue reservation.
Author contributions
RM: Conceptualization, Data curation, Investigation, Methodology, Visualization, Writing – original draft, Writing – review and editing. VR: Conceptualization, Investigation, Methodology, Software, Supervision, Validation, Visualization, Writing – review and editing. AMA: Funding acquisition, Project administration, Supervision, Validation, Writing – review and editing. MT: Funding acquisition, Project administration, Supervision, Validation, Writing – review and editing. LGB: Conceptualization, Funding acquisition, Methodology, Project administration, Supervision, Validation, Writing – review and editing.
Funding
The author(s) declare that financial support was received for the research and/or publication of this article. The presented work is part of the DeepPurple project, which has received funding from the European Research Council (ERC) under the European Union’s Horizon 2020 research and innovation program (Grant Agreement No. 856416). We also acknowledge the European Regional Development Fund and the State of Brandenburg for access to the Potsdam Imaging and Spectral Analysis Facility (PISA) at the GFZ.
Acknowledgments
This work would not have been possible without the contributions of Eva Doting, Laura Halbach, Lasse Twiggs Degn (RISO), and Sandy Herrmann (GFZ), who supported the field campaign organization. We thank Pablo Forjanes, Stefanie Lutz and Sathish Mayanna (GFZ) for their contributions with images in the Supplementary Material. We also thank Wilfried Steiner and Reik Sünkel (GFZ) for their help with designing and manufacturing the cryo-SEM stage.
Conflict of interest
The authors declare that the research was conducted in the absence of any commercial or financial relationships that could be construed as a potential conflict of interest.
The author(s) declared that they were an editorial board member of Frontiers, at the time of submission. This had no impact on the peer review process and the final decision.
Generative AI statement
The author(s) declare that no Generative AI was used in the creation of this manuscript.
Publisher’s note
All claims expressed in this article are solely those of the authors and do not necessarily represent those of their affiliated organizations, or those of the publisher, the editors and the reviewers. Any product that may be evaluated in this article, or claim that may be made by its manufacturer, is not guaranteed or endorsed by the publisher.
Supplementary material
The Supplementary Material for this article can be found online at: https://www.frontiersin.org/articles/10.3389/feart.2025.1572881/full#supplementary-material
References
Anesio, A. M., Lutz, S., Chrismas, N. A. M., and Benning, L. G. (2017). The microbiome of glaciers and ice sheets. Npj Biofilms Microbiomes 3 (1), 10. doi:10.1038/s41522-017-0019-0
Baker, I. (2019). Microstructural characterization of snow, firn and ice. Philosophical Trans. R. Soc. A Math. Phys. Eng. Sci. 377 (2146), 20180162. doi:10.1098/rsta.2018.0162
Bohleber, P., Larkman, P., Stoll, N., Clases, D., Gonzalez de Vega, R., Šala, M., et al. (2024). Quantitative insights on impurities in ice cores at the micro-scale from calibrated LA- ICP-MS imaging. Geochem. Geophys. Geosystems G. 25 (3), 25. doi:10.1029/2023gc011425
Box, J. E., Fettweis, X., Stroeve, J. C., Tedesco, M., Hall, D. K., and Steffen, K. (2012). Greenland ice sheet albedo feedback: thermodynamics and atmospheric drivers. Cryosphere 6, 821–839. doi:10.5194/tc-6-821-2012
Bradley, J. A., Trivedi, C. B., Winkel, M., Mourot, R., Lutz, S., Larose, C., et al. (2022). Active and dormant microorganisms on glacier surfaces. Geobiology 21 (2), 244–261. doi:10.1111/gbi.12535
Chevrollier, L.-A., Cook, J. M., Halbach, L., Jakobsen, H., Benning, L. G., Anesio, A. M., et al. (2023). Light absorption and albedo reduction by pigmented microalgae on snow and ice. J. Glaciol. 69 (274), 333–341. doi:10.1017/jog.2022.64
Cook, J. M., Tedstone, A. J., Williamson, C., McCutcheon, J., Hodson, A. J., Dayal, A., et al. (2020). Glacier algae accelerate melt rates on the south-western Greenland Ice Sheet. Cryosphere 14 (1), 309–330. doi:10.5194/tc-14-309-2020
Dial, R. J., Ganey, G. Q., and Skiles, S. M. (2018). What color should glacier algae be? An ecological role for red carbon in the cryosphere. FEMS Microbiol. Ecol. 94 (3). doi:10.1093/femsec/fiy007
Dohnalkova, A. C., Marshall, M. J., Arey, B. W., Williams, K. H., Buck, E. C., and Fredrickson, J. K. (2011). Imaging hydrated microbial extracellular polymers: comparative analysis by electron microscopy. Appl. Environ. Microbiol. 77 (4), 1254–1262. doi:10.1128/AEM.02001-10
Dong, Z., Li, Z., Wang, F., and Zhang, M. (2009). Characteristics of atmospheric dust deposition I n snow on the glaciers of the eastern Tien Shan, China. J. Glaciol. 55 (193), 797–804. doi:10.3189/002214309790152393
Dumont, M., Brun, E., Picard, G., Michou, M., Libois, Q., Petit, J.-R., et al. (2014). Contribution of light-absorbing impurities in snow to Greenland’s darkening since 2009. Nat. Geosci. 7 (7), 509–512. doi:10.1038/ngeo2180
Duval, B., Shetty, K., and Thomas, W. H. (1999). Phenolic compounds and antioxidant properties in the snow alga Chlamydomonas nivalis after exposure to UV light. J. Of Appl. Phycol. 11 (6), 559–566. doi:10.1023/a:1008178208949
Edwards, A., Douglas, B., Anesio, A. M., Rassner, S. M., Irvine-Fynn, T. D., Sattler, B., et al. (2013a). A distinctive fungal community inhabiting cryoconite holes on glaciers in Svalbard. Fungal Ecol. 6, 168–176. doi:10.1016/j.funeco.2012.11.001
Edwards, A., Pachebat, J. A., Swain, M., Hegarty, M., Hodson, A. J., Irvine-Fynn, T. D. L., et al. (2013b). A metagenomic snapshot of taxonomic and functional diversity in an alpine glacier cryoconite ecosystem. Environ. Res. Lett. 8 (3), 035003. doi:10.1088/1748-9326/8/3/035003
Faria, S. H., Freitag, J., and Kipfstuhl, S. (2010). Polar ice structure and the integrity of ice-core paleoclimate records. Quat. Sci. Rev. 29, 338–351. doi:10.1016/j.quascirev.2009.10.016
Fausto, R. S., Van As, D., Mankoff, K. D., Vandecrux, B., Citterio, M., Ahlstrøm, A. P., et al. (2021). PROMICE automatic weather station data. Cryosphere – Glaciol. doi:10.5194/essd-2021-80
Feng, S., Cook, J. M., Anesio, A. M., Benning, L. G., and Tranter, M. (2023a). Long time series (1984- 2020) of albedo variations on the Greenland ice sheet from harmonized Landsat and Sentinel 2 imagery. J. Glaciol. 69 (277), 1225–1240. doi:10.1017/jog.2023.11
Feng, S., Cook, J. M., Naegeli, K., Anesio, A. M., Benning, L. G., and Tranter, M. (2024). The impact of bare ice duration and geo-topographical factors on the darkening of the Greenland Ice Sheet. Geophys. Res. Lett. 51, e2023GL104894. doi:10.1029/2023GL104894
Feng, S., Cook, J. M., Onuma, Y., Naegeli, K., Tan, W., Anesio, A. M., et al. (2023b). Remote sensing of ice albedo using harmonized Landsat and Sentinel 2 datasets: Validation. Int. J. Remote Sens. 45, 7724–7752. doi:10.1080/01431161.2023.2291000
Fiołka, M. J., Takeuchi, N., Sofińska-Chmiel, W., Wójcik-Mieszawska, S., Irvine-Fynn, T., and Edwards, A. (2021). Morphological and spectroscopic analysis of snow and glacier algae and their parasitic fungi on different glaciers of Svalbard. Sci. Rep. 11 (1), 1. doi:10.1038/s41598-021-01211-8
Fujiwara, K., Iida, Y., Iwai, T., Aoyama, C., Inukai, R., Ando, A., et al. (2013). The rhizosphere microbial community in a multiple parallel mineralization system suppresses the pathogenic fungus Fusarium oxysporum. MicrobiologyOpen 2 (6), 997–1009. doi:10.1002/mbo3.140
Halbach, L., Chevrollier, L.-A., Cook, J. M., Stevens, I. T., Hansen, M., Anesio, A. M., et al. (2022a). Dark ice in a warming world: advances and challenges in the study of Greenland Ice Sheet’s biological darkening. Ann. Glaciol. 63 (87–89), 95–100. doi:10.1017/aog.2023.17
Halbach, L., Chevrollier, L.-A., Doting, E. L., Cook, J. M., Jensen, M. B., Benning, L. G., et al. (2022b). Pigment signatures of algal communities and their implications for glacier surface darkening. Sci. Rep. 12 (1), 17643. doi:10.1038/s41598-022-22271-4
Halbach, L., Kitzinger, K., Hansen, M., Littmann, S., Benning, L. G., Bradley, J. A., et al. (2025). Single-cell imaging reveals efficient nutrient uptake and growth of microalgae darkening the Greenland Ice Sheet. Nat. Commun. 16 (1), 1521. doi:10.1038/s41467-025-56664-6
Harding, T., Jungblut, A. D., Lovejoy, C., and Vincent, W. F. (2011). Microbes in high arctic snow and implications for the cold biosphere. Appl. Environ. Microbiol. 77 (10), 3234–3243. doi:10.1128/AEM.02611-10
Hoham, R. W., and Remias, D. (2020). Snow and glacial algae: a review. J. Phycol. 56 (2), 264–282. doi:10.1111/jpy.12952
Holland, A. T., Williamson, C. J., Sgouridis, F., Tedstone, A. J., McCutcheon, J., Cook, J. M., et al. (2019). Dissolved organic nutrients dominate melting surface ice of the Dark Zone (Greenland Ice Sheet). Biogeosciences 16, 3283–3296. doi:10.5194/bg-16-3283-2019
Hotaling, S., Lutz, S., Dial, R. J., Anesio, A. M., Benning, L. G., Fountain, A. G., et al. (2021). Biological albedo reduction on ice sheets, glaciers, and snowfields. Earth-Science Rev. 220, 103728. doi:10.1016/j.earscirev.2021.103728
Jaarsma, A. H., Zervas, A., Sipes, K., Campuzano Jiménez, F., Smith, A. C., Svendsen, L. V., et al. (2023). The undiscovered biosynthetic potential of the Greenland Ice Sheet microbiome. Front. Microbiol. 14, 1285791. doi:10.3389/fmicb.2023.1285791
Kuzyakov, Y., and Razavi, B. S. (2019). Rhizosphere size and shape: temporal dynamics and spatial stationarity. Soil Biol. Biochem. 135, 343–360. doi:10.1016/j.soilbio.2019.05.011
Leya, T., Rahn, A., Lütz, C., and Remias, D. (2009). Response of arctic snow and permafrost algae to high light and nitrogen stress by changes in pigment composition and applied aspects for biotechnology. FEMS Microbiol. Ecol. 67 (3), 432–443. doi:10.1111/j.1574-6941.2008.00641.x
Løkkegaard, A., Mankoff, K. D., Zdanowicz, C., Clow, G. D., Lüthi, M. P., Doyle, S. H., et al. (2023). Greenland and Canadian Arctic ice temperature profiles database. Cryosphere 17, 3829–3845. doi:10.5194/tc-17-3829-2023
Lutz, S., Anesio, A. M., Edwards, A., and Benning, L. G. (2015a). Microbial diversity on Icelandic glaciers and ice caps. Front. Microbiol. 6, 307. doi:10.3389/fmicb.2015.00307
Lutz, S., Anesio, A. M., Field, K., and Benning, L. G. (2015b). Integrated ‘omics’, targeted metabolite and single-cell analyses of arctic snow algae functionality and adaptability. Front. Microbiol. 6, 1323. doi:10.3389/fmicb.2015.01323
Lutz, S., Anesio, A. M., Jorge Villar, S. E., and Benning, L. G. (2014). Variations of algal communities cause darkening of a Greenland glacier. FEMS Microbiol. Ecol. 89 (2), 402–414. doi:10.1111/1574-6941.12351
Lutz, S., Anesio, A. M., Raiswell, R., Edwards, A., Newton, R. J., Gill, F., et al. (2016). The biogeography of red snow microbiomes and their role in melting arctic glaciers. Nat. Commun. 7 (1), 11968. doi:10.1038/ncomms11968
Lutz, S., McCutcheon, J., McQuaid, J. B., and Benning, L. G. (2018). The diversity of ice algal communities on the Greenland Ice Sheet as revealed by oligotyping. Microb. Genomics 4 (3), e000159. doi:10.1099/mgen.0.000159
McCutcheon, J., Lutz, S., Williamson, C., Cook, J. M., Tedstone, A. J., Vanderstraeten, A., et al. (2021). Mineral phosphorus drives glacier algal blooms on the Greenland Ice Sheet. Nat. Commun. 12 (1), 570. doi:10.1038/s41467-020-20627-w
McCutcheon, J., McQuaid, J. B., Canha, N., Barr, S. L., Lutz, S., Roddatis, V., et al. (2024). “Locally derived mineral dust fertilizes the glacier ice algal blooms in the ‘Dark Zone’ of the Greenland Ice Sheet,” in GOLDSCHMIDT - 2024 goldschmidt conference. Available online at: https://conf.goldschmidt.info/goldschmidt/2024/meetingapp.cgi/Paper/22760.
Mourot, R. (2025). Figure 1. Multi-scale workflow for collection, preservation and cryo-imaging of ice surface samples. Created in BioRender. Available at: https://BioRender.com/ph7nxib.
Nagar, S., Antony, R., and Thamban, M. (2021). Extracellular polymeric substances in Antarctic environments: a review of their ecological roles and impact on glacier biogeochemical cycles. Polar Sci. 30, 100686. doi:10.1016/j.polar.2021.100686
Nagatsuka, N., Takeuchi, N., Nakano, T., Shin, K., and Kokado, E. (2014). Geographical variations in Sr and Nd isotopic ratios of cryoconite on Asian glaciers. Environ. Res. Lett. 9 (4), 045007. doi:10.1088/1748-9326/9/4/045007
Neu, T. R., and Lawrence, J. R. (2014). Advanced techniques for in situ analysis of the biofilm matrix (Structure, composition, dynamics) by means of laser scanning microscopy. Methods Mol. Biol. 1147, 43–64. doi:10.1007/978-1-4939-0467-9_4
Nicholes, M. J. (2020). Bacterial dynamics on the surface of the Greenland ice sheet. (doctoral dissertation). University of Bristol, Bristol, United Kingdom: University of Bristol. Available online at: https://research-information.bris.ac.uk/en/studentTheses/bacterial-dynamics-on-the-surface-of-the-greenland-ice-sheet.
Perini, L., Gostinčar, C., Anesio, A. M., Williamson, C., Tranter, M., and Gunde-Cimerman, N. (2019). Darkening of the Greenland ice sheet: fungal abundance and diversity are associated with algal bloom. Front. Microbiol. 10, 557. doi:10.3389/fmicb.2019.00557
Perini, L., Gostinčar, C., Likar, M., Frisvad, J. C., Kostanjšek, R., Nicholes, M., et al. (2023). Interactions of fungi and algae from the Greenland ice sheet. Microb. Ecol. 86 (1), 282–296. doi:10.1007/s00248-022-02033-5
Procházková, L., Řezanka, T., Nedbalová, L., and Remias, D. (2021). Unicellular versus Filamentous: The Glacial Alga Ancylonema alaskana comb. et stat. nov. and Its Ecophysiological Relatedness to Ancylonema nordenskioeldii (Zygnematophyceae, Streptophyta). Microorganisms 20 (5), 1103. doi:10.3390/microorganisms9051103
Remias, D., Holzinger, A., Aigner, S., and Lütz, C. (2012). Ecophysiology and ultrastructure of Ancylonema nordenskiöldii (Zygnematales, Streptophyta), causing brown ice on glaciers in Svalbard (high arctic). Polar Biol. 35 (6), 899–908. doi:10.1007/s00300-011-1135-6
Remias, D., Holzinger, A., and Lütz, C. (2009). Physiology, ultrastructure and habitat of the ice alga Mesotaenium berggrenii (Zygnemaphyceae, Chlorophyta) from glaciers in the European Alps. Phycologia 48 (4), 302–312. doi:10.2216/08-13.1
Remias, D., Procházková, L., Nedbalová, L., Benning, L. G., and Lutz, S. (2023). Novel insights in cryptic diversity of snow and glacier ice algae communities combining 18S rRNA gene and ITS2 amplicon sequencing. FEMS Microbiol. Ecol. 99 (12), fiad134. doi:10.1093/femsec/fiad134
Rossel, P. E., Antony, R., Mourot, R., Dittmar, T., Anesio, A. M., Tranter, M., et al. (2025). Dynamics of organic matter in algal blooms on the Greenland ice sheet. Sci. Rep. 15 (1), 8288. doi:10.1038/s41598-025-92182-7
Rousk, J., and Bengtson, P. (2014). Microbial regulation of global biogeochemical cycles. Front. Microbiol. 5, 103. doi:10.3389/fmicb.2014.00103
Ryan, J. C., Hubbard, A., Stibal, M., Irvine-Fynn, T. D., Cook, J., Smith, L. C., et al. (2018). Dark zone of the Greenland Ice Sheet controlled by distributed biologically-active impurities. Nat. Commun. 9 (1), 1065. doi:10.1038/s41467-018-03353-2
Seymour, J. R., Amin, S. A., Raina, J. B., and Stocker, R. (2017). Zooming in on the phycosphere: the ecological interface for phytoplankton–bacteria relationships. Nature Microbiology 2, 17065. doi:10.1038/nmicrobiol.2017.65
Smith, H. J., Schmit, A., Foster, R., Littman, S., Kuypers, M. M., and Foreman, C. M. (2016). Biofilms on glacial surfaces: hotspots for biological activity. Npj Biofilms Microbiomes 2 (1), 16008. doi:10.1038/npjbiofilms.2016.8
Stibal, M., Anesio, A. M., Blues, C. J. D., and Tranter, M. (2009). Phosphatase activity and organic phosphorus turnover on a high Arctic glacier. Biogeosciences 6, 913–922. doi:10.5194/bg-6-913-2009
Stibal, M., Box, J., Cameron, K., Langen, P., Yallop, M., Mottram, R., et al. (2017). Algae drive enhanced darkening of bare ice on the Greenland ice sheet: algae drive Greenland bare ice darkening. Geophys. Res. Lett. 44. doi:10.1002/2017GL075958
Stibal, M., Šabacká, M., and Kaštovská, K. (2006). Microbial communities on glacier surfaces in Svalbard: impact of physical and chemical properties on abundance and structure of cyanobacteria and algae. Microb. Ecol. 52 (4), 644–654. doi:10.1007/s00248-006-9083-3
Stoll, N., Hörhold, M., Erhardt, T., Eichler, J., Jensen, C., and Weikusat, I. (2022). Microstructure, micro-inclusions, and mineralogy along the EGRIP (East Greenland Ice Core Project) ice core – Part 2: implications for palaeo-mineralogy. Cryosphere 16, 667–688. doi:10.5194/tc-16-667-2022
Takeuchi, N. (2002). Optical characteristics of cryoconite (surface dust) on glaciers: the relationship between light absorbency and the property of organic matter contained in the cryoconite. Ann. Glaciol. 34, 409–414. doi:10.3189/172756402781817743
Telling, J., Anesio, A. M., Tranter, M., Irvine-Fynn, T., Hodson, A., Butler, C., et al. (2011). Nitrogen fixation on arctic glaciers, svalbard. J. Geophys. Res. 116, G03039. doi:10.1029/2010JG001632
Wadham, J. L., Hawkings, J. R., Tarasov, L., Gregoire, L. J., Spencer, R. G. M., Gutjahr, M., et al. (2019). Ice sheets matter for the global carbon cycle. Nat. Commun. 10 (1), 3567. doi:10.1038/s41467-019-11394-4
Weikusat, I., Jansen, D., Binder, T., Eichler, J., Faria, S. H., Wilhelms, F., et al. (2017). Physical analysis of an antarctic ice core—towards an integration of micro- and macrodynamics of polar ice. Philosophical Trans. R. Soc. A Math. Phys. Eng. Sci. 375 (2086), 20150347. doi:10.1098/rsta.2015.0347
Weiss, R. L. (1983). Fine structure of the snow alga (chlamydomonas nivalis) and associated Bacteria1. J. Phycol. 19 (2), 200–204. doi:10.1111/j.0022-3646.1983.00200.x
Wientjes, I. G. M., Van De Wal, R. S. W., Reichart, G. J., Sluijs, A., and Oerlemans, J. (2011). Dust from the dark region in the western ablation zone of the Greenland ice sheet. Cryosphere 5 (3), 589–601. doi:10.5194/tc-5-589-2011
Williamson, C. J., Cook, J., Tedstone, A., Yallop, M., McCutcheon, J., Poniecka, E., et al. (2020). Algal photophysiology drives darkening and melt of the Greenland Ice Sheet. Proc. Natl. Acad. Sci. 117 (11), 5694–5705. doi:10.1073/pnas.1918412117
Williamson, C. J., Turpin-Jelfs, T., Nicholes, M. J., Yallop, M. L., Anesio, A. M., and Tranter, M. (2021). Macro- nutrient stoichiometry of glacier algae from the southwestern margin of the Greenland ice sheet. Front. Plant Sci. 12, 673614. doi:10.3389/fpls.2021.673614
Yallop, M. L., Anesio, A. M., Perkins, R. G., Cook, J., Telling, J., Fagan, D., et al. (2012). Photophysiology and albedo-changing potential of the ice algal community on the surface of the Greenland ice sheet. ISME J. 6 (12), 2302–2313. doi:10.1038/ismej.2012.107
Keywords: microscopy, cryo-SEM, glacier ice algae, multi-scale, microbial ecology
Citation: Mourot R, Roddatis V, Anesio AM, Tranter M and Benning LG (2025) Cryo multi-scale microscopy visualization tools for studying microbial communities on glacier surfaces. Front. Earth Sci. 13:1572881. doi: 10.3389/feart.2025.1572881
Received: 07 February 2025; Accepted: 01 May 2025;
Published: 21 May 2025.
Edited by:
Nozomu Takeuchi, Chiba University, JapanReviewed by:
Casey Brigham Engstrom, Simon Fraser University, CanadaNaoko Nagatsuka, Japan Agency for Marine-Earth Science and Technology (JAMSTEC), Japan
Copyright © 2025 Mourot, Roddatis, Anesio, Tranter and Benning. This is an open-access article distributed under the terms of the Creative Commons Attribution License (CC BY). The use, distribution or reproduction in other forums is permitted, provided the original author(s) and the copyright owner(s) are credited and that the original publication in this journal is cited, in accordance with accepted academic practice. No use, distribution or reproduction is permitted which does not comply with these terms.
*Correspondence: Rey Mourot, cmV5Lm1vdXJvdEBtaW8ub3N1cHl0aGVhcy5mcg==; Liane G. Benning, YmVubmluZ0BnZnouZGU=