- 1National Institute of Natural Hazards, Ministry of Emergency Management of China, Beijing, China
- 2Key Laboratory of Compound and Chained Natural Hazards Dynamics, Ministry of Emergency Management of China, Beijing, China
- 3Key Laboratory of Land Surface Pattern and Simulation, Institute of Geographic Sciences and Natural Resources Research, Chinese Academy of Sciences, Beijing, China
Lake sediments are an important carrier for recording natural disaster events. The key lies in correctly identifying the causes of abnormal lacustrine sediments. The study area, Qionghai Lake, is located at the southeastern edge of the Tibetan Plateau, at the junction of the Anning River Fault and the Zemu River Fault. Disasters such as earthquakes and floods are quite common. By conducting high-resolution analyses of chronology, sedimentary structure, and physical and chemical indicators on six short sediment cores collected from Qionghai Lake, we find that the common characteristics of flood- and earthquake-induced deposits are a sudden increase in the mean grain size, poor sorting property, and an abnormally high Mn content at the bottom of some event layers. The differential characteristics of earthquake-induced deposits are: that the contents of terrigenous elements such as Si and Rb remain at a relatively stable level; that the sedimentary structure lacks obvious bedding and is a homogeneous layer; and that the grain-size characteristics of some event layers show oscillatory changes. The differential characteristics of flood-induced deposits are a significant increase in the contents of indicator elements of terrigenous clastic sediments; and a gradually decreasing grain-size trend upwards, showing an obvious normal grading feature. In addition, the calculation of seismic intensity for earthquakes around Qionghai Lake shows that the threshold for seismically-induced deposit response in Qionghai Lake should be between 4.44–5.95 MMI.
1 Introduction
Lake sediments contain abundant information and typically form continuous depositional strata characterized by high sensitivity, high resolution, long-term records, and widespread regional distribution. Modern lacustrine sedimentology primarily focuses on the constant sedimentary record of paleoclimate evolution and its trends (Lan et al., 2020; Shah et al., 2020; Diaz et al., 2023). For example, Xiao et al. (2020) reconstructed vegetation, climate, and human influences in central Yunnan over the past 20,000 years using high-resolution environmental proxies such as pollen and charcoal.
Lake sediments not only serve as valuable tools for studying environmental and climate changes but also contribute to a deeper understanding of sedimentary processes. As important record carriers, lake sediments, like those in rivers and oceans, provide valuable data such as grain size and geochemical elements. Special sedimentary structures and anomalous elemental enrichments preserved in these sediments can be used to reconstruct past disaster events, such as floods and earthquakes. Frequent and catastrophic disaster events can cause significant social and economic losses, making the reconstruction of long-term disaster records highly important for regional disaster prevention and mitigation. However, historical records and instrumental data generally cover a short period, often limited to only a few thousand years, creating an urgent need for disaster research methods with longer time scales (Beck, 2009; Knapp et al., 2018; Azennoud et al., 2022). Lake sediments can record disaster events over much longer periods, thus largely compensating for the limitations of historical records and instrumental data (Strasser et al., 2013; Oswald et al., 2021; Banjan et al., 2023). In his study of the 1996 delta collapse and giant turbidite in Lake Brienz, suggested that lake sediment research could offer new perspectives for the assessment of natural disasters.
In recent years, lake sediments have demonstrated significant advantages in reconstructing past events such as ancient earthquakes and floods, serving as high-resolution archives of past anomalous events (Strasser et al., 2013; Ghazoui et al., 2019; Vandekerkhove et al., 2020; Biguenet et al., 2021; Daxer et al., 2022). Event-induced deposits (EID) are primarily classified into four main types: Mass-transport deposits (MTD), which are characterized by bulk transport (Strasser et al., 2006; Praet et al., 2020); Deformed structures (DS) (Monecke et al., 2004; Molenaar et al., 2021; Zhong et al., 2022); Lacustrine turbidites (LT), represented by lake turbidity currents (Van Daele et al., 2015; Li et al., 2021); and Seismically-induced dispersal redeposit (SIR), triggered by seismic activity that initiates, transports, and forms deposits. However, due to the similar sedimentary characteristics produced by different triggering factors, identifying the specific triggers of event-induced deposits remains a challenge (Rapuc et al., 2019). Previous studies have distinguished these events based on various characteristics: for example, flood deposits typically show significant terrigenous input and higher organic matter content (Wilhelm et al., 2013; Wilhelm et al., 2022), while geochemical analysis can differentiate sediment sources in event layers (Gastineau et al., 2023; Araya et al., 2024). Beck (2009) distinguished seismic and flood turbidites by examining the vertical evolution of grain size (sorting, skewness, median, and D99). Owen et al. (2011) suggested that earthquake-related deposits are spatially widespread, laterally continuous, and vertically repetitive.
The southeastern edge of the Tibetan Plateau is one of the most tectonically active and seismically intense regions in the world today (Wu et al., 2014; Shi et al., 2023a), particularly the western Sichuan region, at the junction of the Sichuan-Yunnan Block, Bayan Har Block, and South China Block, where major earthquakes have occurred every few decades (Xu et al., 2005; Li et al., 2008; Zhang et al., 2023). Xichang lies along the Anning River-Zemu River fault zone, a major fault along the eastern boundary of the Sichuan-Yunnan Block on the southeastern edge of the Tibetan Plateau. Historical seismic records and paleoseismic studies indicate that several significant earthquakes have occurred along this fault zone, such as the Ms7.5 earthquake in 1,536 and the Ms7.5 earthquake in 1850 (Wen et al., 2007; Ren and Lin, 2010; Yu et al., 2023a). Xichang features a typical mountain canyon landscape, with deeply incised river valleys and steep slopes. Intense geological activity in the high mountain canyon area creates favorable conditions for slope-related hazards (Dai et al., 2020), leading to frequent occurrences of landslides, collapses, and debris flows in the region (Zhou et al., 2021; Yi et al., 2022). The complex geological conditions and variable climate in the study area contribute to the frequent occurrence of various disasters, including earthquakes and floods. These events cause severe damage to local infrastructure, residents’ lives, and property, as well as socio-economic development, resulting in significant losses. Therefore, conducting in-depth disaster research in this region is of great practical importance.
This study conducts high-resolution analyses of the chronostratigraphy, sedimentary structures, and physical and chemical indicators from six short sediment cores taken from the northern, central, and southern parts of Qionghai Lake. Based on historical disaster records, the study identifies and characterizes earthquake and flood events in the lake sediments, revealing their sedimentary processes and laying the groundwork for lake disaster research.
2 Setting
2.1 Qionghai Lake and its basin
Qionghai Lake (102°16′E−102°28′E, 27°42′N–27°55′N) is located in Xichang City, Sichuan Province, in the mountainous canyon region on the southeastern edge of the Tibetan Plateau (Figure 1A). It is the second-largest freshwater lake in Sichuan and is classified as a tectonic freshwater lake on the plateau. Qionghai Lake belongs to the Yalong River system within the Yangtze River basin. The lake covers an area of approximately 30 km2, with an average depth of 10.3 m and a water storage capacity of about 3.2 × 108 m3 (Li et al., 2023; Ren and Zheng, 2024). The lake has a spiral shape, with a maximum length of about 10.3 km from north to south and a maximum width of about 5.6 km from east to west. The terrain around the lake basin is highly undulating, with a relatively gentle central basin and more complex topography in the northeastern direction (Dong et al., 2024) (Figures 1B,C).
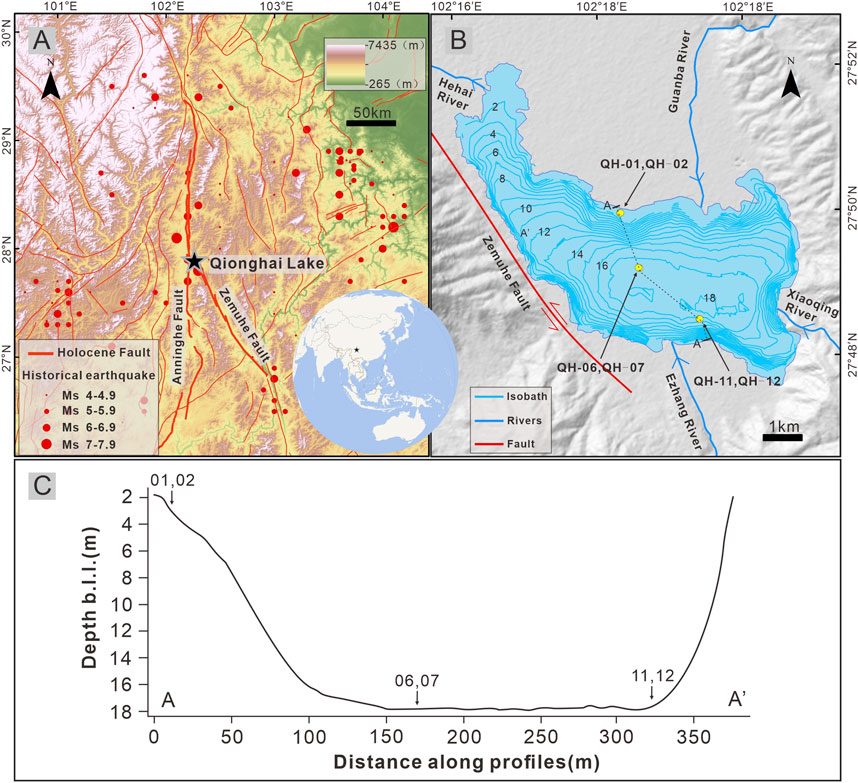
Figure 1. (A) Location and characteristics of the Anning River Fault, Zemu River Fault, Qionghai, and surrounding historical earthquakes (https://data.earthquake.cn/). Digital elevation model (DEM) from: https://panda.copernicus.eu/panda. (B) Bathymetric map of the Qionghai, location of lake core sampling sites, and major rivers in the Qionghai basin. (C) Cross-sectional profile of the lake basin along line AA’ (B), and core locations.
The Qionghai Lake basin features a well-developed river system, covering a total area of 307.67 km2 and collecting water from rivers such as the Xiao Qing River, Guanba River, and Ezhang River (Shi et al., 2023b) (Figure 1B). Among these, the Guanba River basin has the largest area at 121.60 km2, followed by the Ezhang River. The total annual soil erosion in the Qionghai Lake basin is 2.94 × 105 m3, with the Guanba River basin accounting for 53.8%, and the Ezhang River basin for 23.08%. The lake drains into the Hai River, which flows out from the northwestern corner of Qionghai Lake, then turns southwest to join the Anning River after merging with the Dong and Xi Rivers near the eastern and western parts of Xichang City. This region has a subtropical plateau mountain climate, with rainfall mainly concentrated in the summer and autumn seasons, accounting for over 90% of the annual precipitation (Liao et al., 2022). Rainfall is highly variable, with frequent heavy rainfall events. The area is surrounded by mountains on the east, south, and west, and geological hazards such as debris flows and floods are common (Wang et al., 2005; Wei and Chen, 2018). Notable events include the 1998 mountain flood in the Guanba River, considered a once-in-a-century event, and a large debris flow in the Ezhang River in 1853.
The Qionghai Lake basin is mainly composed of Mesozoic and Cenozoic strata, with mudstone, silty mudstone, marl, and shale as the primary rock types (Wei et al., 2014). The lake’s sediment primarily consists of brown and gray silty sand (Yu et al., 2023b).
2.2 Tectonic environment and seismic activity
Qionghai is located at the junction of the Anning River Fault and the Zemu River Fault. Both the Anning River Fault and the Zemu River Fault are large left-lateral strike-slip fault zones at the boundary between the Sichuan-Yunnan Block and the South China Block. They lie within the middle section of the Xianshuihe-Xiaojiang fault system and are the main active faults on the northeastern boundary of the Sichuan-Yunnan Block (Figure 1A). Under the collision and compression of the Indian Plate and the Eurasian Plate, the northward movement of the Indian Plate leads to intense uplift along the plate boundary and interior. Material from the Tibetan Plateau escapes eastward but is obstructed by the stable South China Block. The combined effects of these deep-seated dynamic processes result in active tectonic activity in the areas surrounding the Anning River-Zemu River fault zone (Li et al., 2020; Wu, 2020; Liu et al., 2023).
The Anning River Fault Zone is located in the Anning River Valley, with an overall strike close to NS. Most of the fault zone is concealed fault, extending north from Shimian and connecting with the Xianshuihe Fault Zone. It extends south through Mianning, Xichang, and Dechang, and connects with the Zemu River Fault Zone near Xichang. Since the Late Quaternary, the Anning River Fault has shown strong left-lateral strike-slip and reverse-slip characteristics, with the maximum left-lateral strike-slip rate since the Cenozoic being approximately 6.2 mm/year (Ran et al., 2008; Wu, 2020). The Zemu River Fault Zone is located in the Qionghai-Daqing area, with a general strike of NNW 330°. The northwestern end of the Zemu River Fault connects with the Anning River Fault, and the fault extends from Xichang through Puge, Ningnan, and stretches to the Qiaojia area in Yunnan, where it connects with the Xiaojiang Fault Zone. Like the Anning River Fault, the Zemu River Fault is predominantly a left-lateral strike-slip fault. The left-lateral strike-slip rate since the Holocene has reached (6.4 ± 0.6) mm/year (Du, 2000; Wen et al., 2008).
The Anning River and Zemu River Fault Zones are located on the eastern margin of the Tibetan Plateau in China and are the core areas of seismic activity in the Sichuan-Yunnan region. The fault zones exhibit significant seismic activity, with high slip rates (Fan et al., 2023) and complex stress accumulation patterns (Wu et al., 2017) in their geological structure. Historical earthquake records and paleoseismic studies show that tectonic activity in the study area is active, with multiple strong earthquakes occurring in the past (Ren and Lin, 2010; Li et al., 2020) (Table 1; Figure 1A). According to the National Earthquake Data Center (https://data.earthquake.cn/index.html), three earthquakes of magnitude 7 or higher have occurred near Xichang. Since the Ms7.5 earthquake along the Anning River Fault in 1,536 and the Ms7.5 earthquake along the Zemu River Fault in 1850, no other strong earthquakes of magnitude 7 or higher have occurred. Therefore, the earthquake hazard in this region requires close attention.
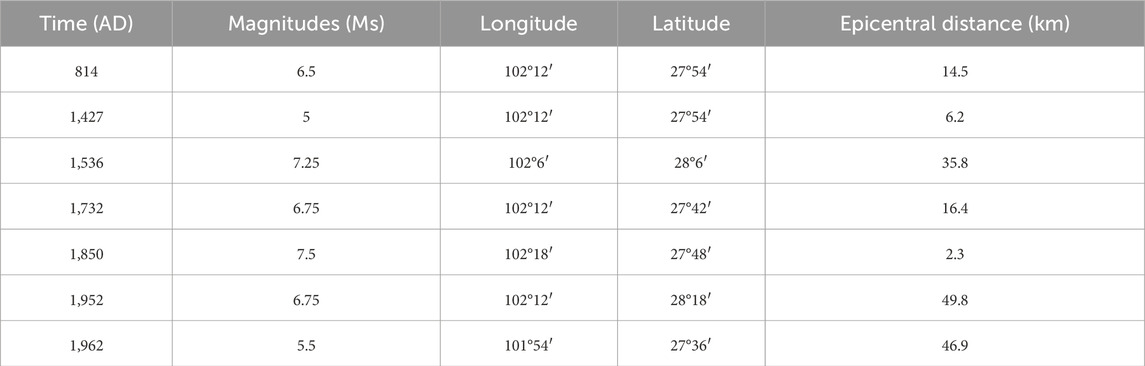
Table 1. Historical earthquakes with epicenters within 50 km of Qionghai and magnitudes ≥ Ms 5.0: Time, magnitude (Ms), geographic coordinates (longitude/latitude), and epicentral distance from Qionghai.
3 Materials and methods
3.1 Bathymetry
The multibeam echosounder is an advanced underwater topographic measurement device capable of accurately mapping the terrain of the water bottom. This technology works by emitting multiple sound beams to the bed and receiving the reflected echoes, which provide detailed information about the underwater terrain (Horvei and Nilsen, 2010; Colbo et al., 2014). The multibeam echosounder offers significant advantages, including high accuracy, high resolution, and wide coverage. It can rapidly survey underwater topography and process data in real time, making it suitable for a variety of environmental conditions, from shallow waters to deep seas. Moreover, due to its versatility, this technology is widely applied in marine surveys, environmental monitoring, and engineering construction, making it an essential tool for modern underwater exploration (Ierodiaconou et al., 2018; Montereale-Gavazzi et al., 2018; Maleika, 2020).
To investigate the water depth and morphological features of Qionghai Lake, a systematic depth measurement was conducted using the multibeam echosounder. The survey was completed in August 2022, using a rubber boat equipped with a Haizhuo MS400P multibeam echosounder. The echosounder was configured with 512 beams, with a beam angle of 2 ° × 1 ° and a maximum opening angle of 143°. The operating frequency was 100 kHz, and the depth measurement range covered from 0.2 to 120 m. To ensure data accuracy, the vertical sound velocity data of the lake water, obtained with the Haizhuo SVS1500 sound velocity profiler, were used to calibrate the multibeam data. All data were processed using Hypack software, and the final lake depth map was obtained (Figure 1B).
3.2 Lake core sampling
In August 2022, lake core sampling was conducted at Qionghai Lake using the VC-D deep-water sediment coring device from SDI, United States. The VC-D coring device employs high-frequency vibration principles for drilling and sampling, combined with a uniquely designed gravity ring and float structure, which allows for vertical drilling in deep-water or fast-flowing environments, ensuring the accuracy of the sampling points. Based on preliminary multibeam bathymetric results (Figure 1B), sampling locations were selected: two cores, QH-01 and QH-02, were extracted from the northern part of the lake at a depth of approximately 2 m near the shoreline; two cores, QH-06 and QH-07, were extracted from the central part of the lake at a depth of about 17 m in a flat basin; and two cores, QH-11 and QH-12, were extracted from the southern part of the lake at a depth of approximately 16 m (Table 2). The northern and southern cores represent different source inputs, specifically from the Guanba River and the Ezhang River, respectively. The northern sampling points are located near river deltas, where the sedimentation rate is higher; the central sampling points are in deeper, flatter lake basin areas; and the southern sampling points are near steep slopes, with deep water. The six lake cores are primarily composed of red-brown silty sand.
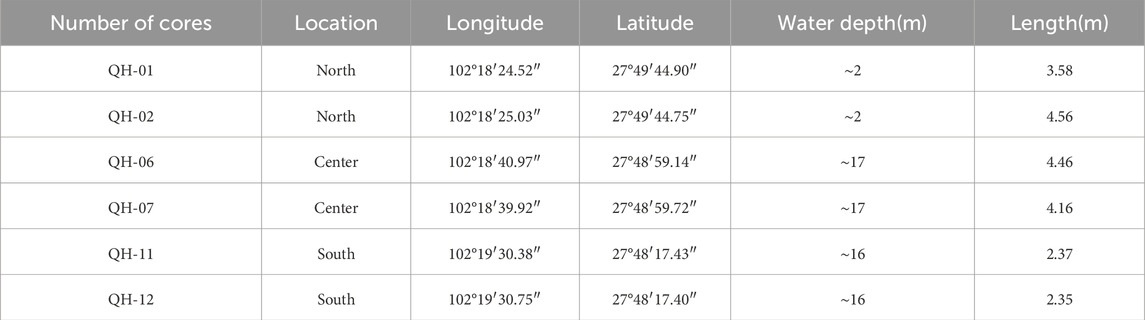
Table 2. Number, location, geographic coordinates (longitude/latitude), water depth, and length of six sedimentary lake cores in Qionghai.
After CT scanning of all cores, they were cut along the central axis using a Geotek Core Splitter at the Institute of Geographic Sciences and Natural Resources Research, Chinese Academy of Sciences, and described in detail. Subsequently, half of the cores were selected for XRF scanning, subsampling, grain size analysis, and dating analysis at appropriate positions. The remaining half of the cores were preserved for future research.
3.3 CT scanning
Before sectioning the cores, CT scanning was conducted at iRock Technologies. Core CT scanning is an important non-destructive method for analyzing sediment cores using computed tomography (CT). This technique utilizes X-rays to penetrate the core and generates high-resolution three-dimensional images by detecting differences in the X-ray absorption properties of various materials. This allows for a clear depiction of internal density variations, layering structures, and possible microfeatures such as fractures and pores within the core (Eltom, 2020). The scanning was performed using the Uct760 system, with scan voltages set at 100 kV (low energy) and 140 kV (high energy) to accommodate the stratified distribution of materials with varying densities and compositions, ensuring clear image contrast. The scanning resolution achieved by the equipment was 0.55 × 0.55 × 0.625 mm. The three-dimensional images obtained from the CT scanning provide direct visual insights into the core’s layering characteristics, sedimentary structures, and interfaces between different sediment layers, further revealing anomalous events within the sediments.
3.4 X-ray fluorescence scanning
X-ray fluorescence (XRF) scanning is a non-destructive, efficient elemental analysis technique that identifies and quantitatively analyzes the elemental composition and distribution by exciting atoms in the sample with X-rays, causing them to emit characteristic fluorescence. This technique is characterized by simultaneous multi-element detection, rapid efficiency, and high sensitivity (Ross et al., 2013; Bertrand et al., 2024).
The XRF scanning was conducted at the Institute of Geographic Sciences and Natural Resources Research, Chinese Academy of Sciences, using the MSCL-S core multi-sensor logging system produced by Geotek, United Kingdom. This system was used to scan the lake cores and obtain elemental composition data. The surface of the cut lake cores was first smoothed to ensure scanning accuracy, followed by the application of a 4 µm UI-tralence film to prevent sample contamination and drying. Scanning was conducted at 1 cm intervals, with each point being tested for 30 s. Different scanning voltages were used for different elements: 10 kV for Al, Si, P, S, Cl, Ar, K, Ca, Sc, Ti, and Mn; and 30 kV for Fe, Rb, Sr, and Zr. The elemental data obtained from the scans are presented in semi-quantitative form, with units of counts per second (cps), reflecting the relative variation trends of the chemical elements. Data with counts below 100 cps, which are deemed unreliable, were excluded in the subsequent data analysis.
3.5 Grain size analysis
The grain size analysis was conducted at the Sediment and Environmental Analysis Laboratory of the National Institute of Natural Hazards. The HELOS laser particle size analyzer, manufactured by Sympatec, Germany, was used for testing. This analyzer operates on the principle of laser diffraction, where a laser beam irradiates the particle suspension, and the scattering angle and intensity of the particles are analyzed to rapidly and accurately determine the particle size distribution (Kusnierz and Lomotowski, 2015; Magno et al., 2018). The laser particle size analyzer offers significant advantages, including a wide measurement range, high resolution, ease of operation, and intuitive results. It is capable of simultaneously characterizing the distribution of particles ranging from microns to nanometers within a sample.
For this analysis, half of the lake core samples were selected, with subsampling conducted at 1 cm intervals. To ensure data reliability, the repeatability error was controlled within 1%. The sampling unit used was the QUIXEL wet method, with measurements taken through three lenses (R1, R4, and R7). The corresponding particle size ranges covered by these lenses were: R1 (0.18–35 μm), R4 (1.8–350 μm), and R7 (18–3,500 μm). During the testing process, ultrasonic treatment was applied to prevent particle aggregation, and grain size-related parameters were calculated using a graphical method.
3.6 Chronology
Given that the samples from the southern and northern cores are located near the river inflow areas, sediment erosion may have occurred. Therefore, the QH-06 core, located closer to the lake basin center, was selected for chronological analysis. Due to the high sedimentation rate at Qionghai Lake, and considering that this study primarily focuses on cores above 3 m, both 210Pb and 137Cs dating methods were employed to calibrate the sediment age. During the experiment, samples from the 60–70 cm section of the QH-06 core were collected at 1 cm intervals, while samples from the 0–60 cm and 70–100 cm sections were collected at 2 cm intervals. The samples were first dried at 60°C in an oven until they reached a constant weight, then ground to a particle size of less than 260 mesh using a mortar and pestle. The dry samples were weighed and sealed in dedicated plastic tubes for 20 days to allow the decay of 222Rn over approximately five half-lives, thus minimizing the impact of 222Rn on the 210Pb measurement. The measurements of 210Pb and 137Cs content were conducted at the National Institute of Natural Hazards, using a high-purity germanium γ-spectrometer. By measuring the specific activity difference between 210Pb and 226Ra, the specific activity of 210Pbex was calculated (Figure 2). The sediment age and sedimentation rate of the core were then inferred by combining the 137Cs peak location (Figure 2) and the characteristics of various radioactive isotopes (Chen et al., 2009; Liu et al., 2022; Constantinescu et al., 2023) (Figures 2, 3C).
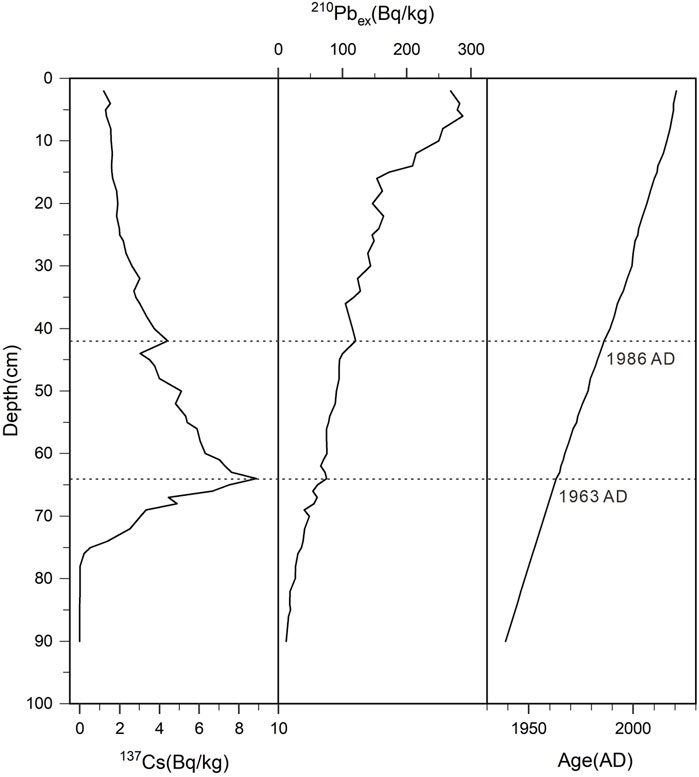
Figure 2. Specific activities of 137Cs and 210Pbex and their dating results in the upper section of Core QH-06.
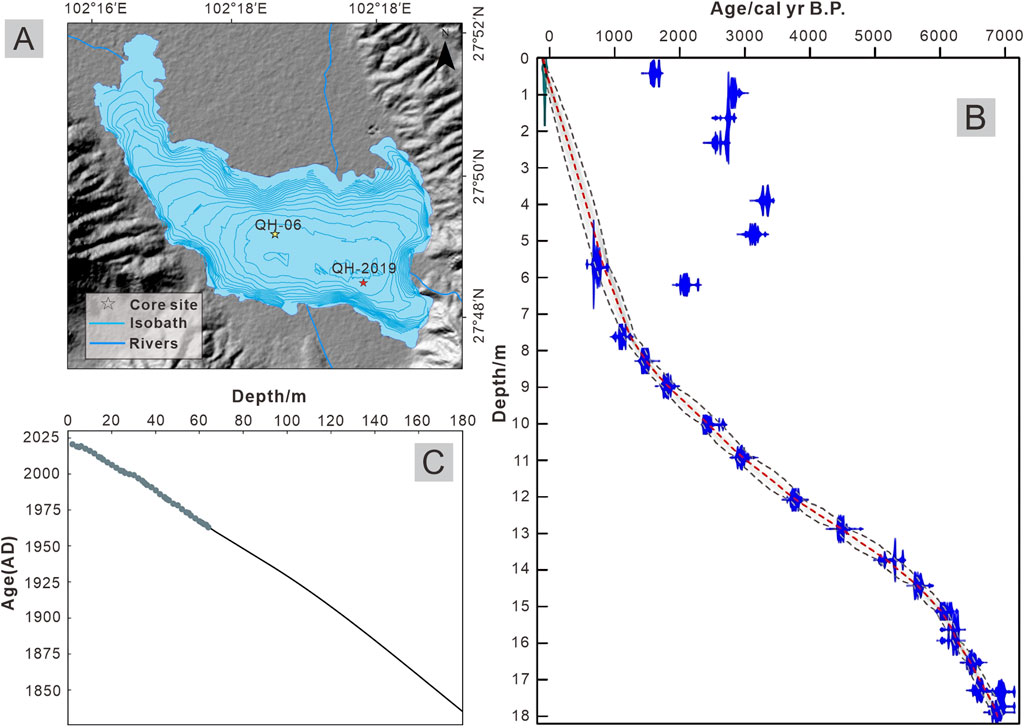
Figure 3. (A) Map of the location of the sampling points, QH-06 is the lake core of the current 137Cs/210Pb dating sample, and QH-2019 is the lake core used for the 14C sample by Liu Xingqi’s team. (B) Age-depth model built by Liu Xingqi’s team based on the 14C dating results. (C) Age-depth model based on 137Cs/210Pb dating data from lake core QH-06, constructed using spline interpolation and extending the length to 180 cm.
4 Result
4.1 Lake bathymetry
The multibeam bathymetric results show that the maximum water depth of the lake exceeds 18 m, with the deepest point located in the southern part of the central basin of Qionghai Lake. The basin features a relatively flat bottom, with gentle slopes at the northwestern ends and steeper slopes at the southeastern ends (Figures 1B,C). A survey conducted by the Xikang Provincial Water Conservancy Bureau in 1952 reported a maximum water depth of 34 m and an average depth of 14 m. This significantly differs from the current measurements, suggesting a substantial reduction in the water level. Even considering potential seasonal variations and measurement errors, the lake level of Qionghai has decreased considerably, indicating rapid shrinkage of the lake. Historical records suggest that Qionghai’s area was 31 km2 in 1952 and 27.4 km2 in 2003, with an average annual reduction of approximately 72,000 m2, representing an 11.6% decrease in area.
In recent years, severe soil erosion has occurred in the Qionghai watershed. Since 1850, the average annual erosion rate has been 1.88 mm/year (Wei et al., 2014). Debris flows from the main tributaries of the lake, particularly the Guanba River, frequently transport large amounts of sediment from the upstream source areas into the lake, advancing by 10–100 m each year. The Guanba River, which contributes the highest sediment load, accounts for more than half of the total soil erosion in the entire Qionghai watershed. As the sediment enters the lake from the northern part, it has caused significant shrinkage of the northern lake basin, which has a gentler slope. Furthermore, the high sediment content in the floods and debris flows from the Guanba River and Ezhang River leads to the formation of turbid flows, which continue to move along the lakebed. As a result, an underwater embankment has formed in the central part of the lake, extending 1 km from north to south, with a width of 100 m in the northern section, gradually expanding to 200 m in the southern section (Figure 1B).
4.2 Sedimentary structures and related indicators
4.2.1 Northern lake core
The positions of the QH-01 and QH-02 lake cores are located in the northern part of the lake (Figures 1B,C), with a water depth of approximately 2 m at the sampling sites, about 120 m from the north shoreline, and a horizontal distance of approximately 15 m between the two cores. These sampling locations are near the Guanba River, the largest inflow river of Qionghai Lake, where the sedimentation rate is the fastest. Due to the frequent occurrence of debris flows and floods in the Guanba River basin, the sediment in this area has a significantly higher silt content. Because the two cores are located close to each other and share similar sedimentary environments, they exhibit a high degree of correlation.
CT scanning images show that the sediments in the lake cores exhibit different depositional characteristics between normal background sediments and event-induced deposits (Figure 4). In the normal background sediments, the sediment displays a typical laminated structure, indicating stable sedimentation processes. The grain size of the normal sediments mainly ranges from 8 μm to 40 μm, with a small grain size deviation; however, the skewness of the grain size distribution is relatively high. The lake cores with normal laminated sediments also contain several layers of anomalous deposits with no laminated structure, exhibiting typical event deposit characteristics.
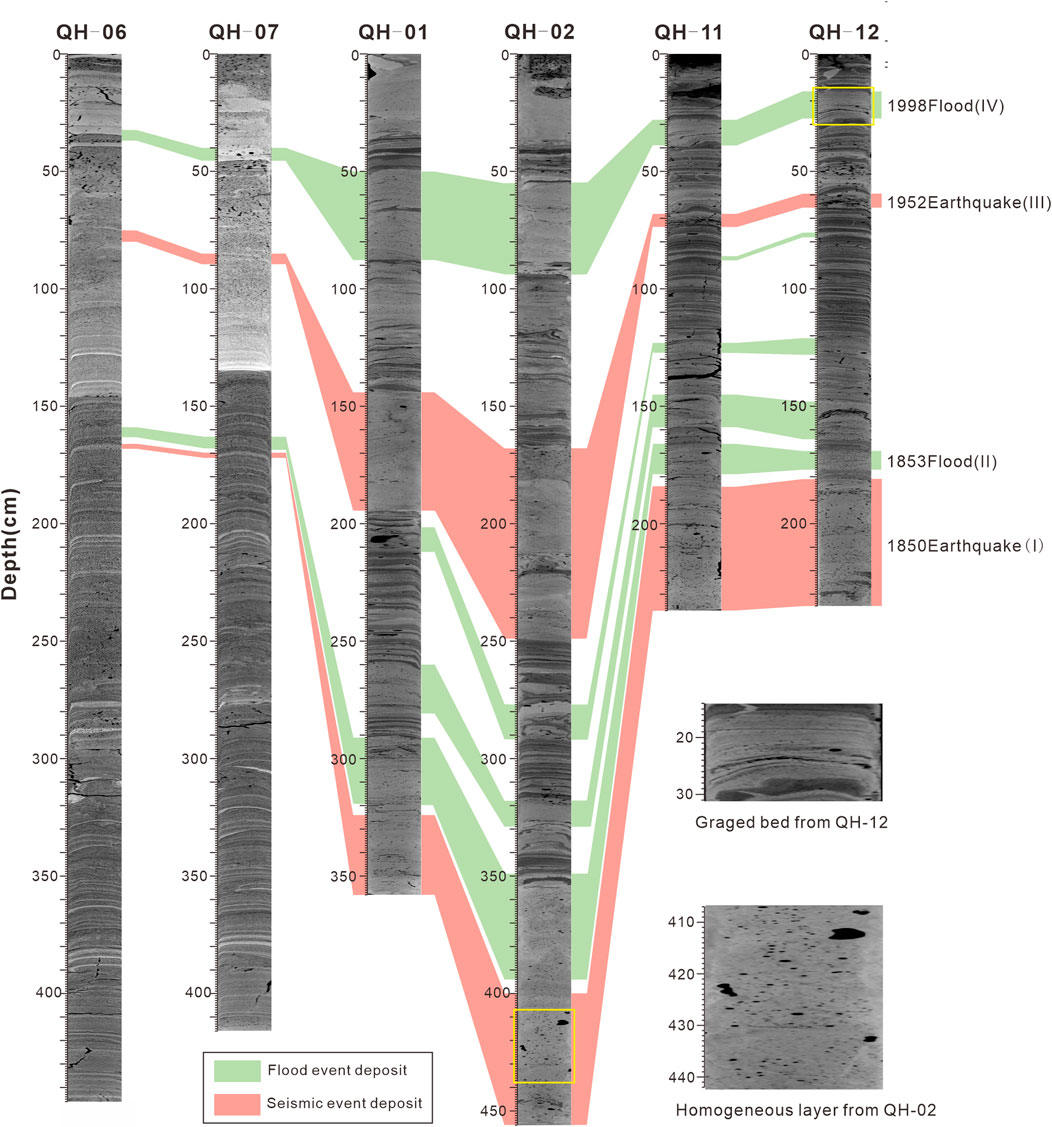
Figure 4. CT images of six lake cores from the northern shore, center, and southern shore, along with correlations among the cores, and enlarged views of graded bedding in flood event deposits and homogeneous layers in seismic event deposits. Flood event deposits are marked by green horizontal color bars, while seismic event deposits are indicated by red horizontal color bars.
In the QH-01 core, sediment intervals at depths of 50–88 cm, 144–194.5 cm, 201.5–212 cm, 260–281 cm, 291–319 cm, and the lower part of 324 cm exhibit deposition characteristics without obvious lamina. Compared with the normal background sediments, the mean grain size and mode of these layers are significantly larger, suggesting that these layers may have been influenced by different depositional environments or anomalous events. Among these, the 144–194.5 cm and lower 324 cm intervals show little variation in grain size parameters and exhibit homogeneous layer characteristics. Around 194.5 cm, there is a brief increase in the Mn content, reaching approximately 400 cps. Other anomalous sediment layers exhibit a trend of gradually finer grain sizes upwards, showing distinct normal grain sequence characteristics, with increased measurements of K, Zr, Fe, and other elements compared to the normal sediments. In some anomalous layers, the Mn measurements also increase at the bottom.
In the QH-02 core, the anomalous sediment layers are located at depths of 55–94 cm, 168–249 cm, 277–292 cm, 318–329 cm, 349–394 cm, and the lower part of 400 cm. These layers also exhibit no clear laminated structure and are characterized by significantly larger grain sizes and higher grain size mode, which correlate well with the anomalous layers in the QH-02 core. The anomalous sediment layers at depths of 168–249 cm and the lower part of 400 cm show little variation in grain size, with a significant increase in Mn content at 249 cm, while no obvious changes are observed in other related elements. Other anomalous sediment layers display normal grain sequences, with increased contents of elements such as Si, K, Fe, and Ti. Mn measurements also show an increase at depths around 94 cm and 394 cm.
4.2.2 Central lake core
The two lake cores located in the central part of Qionghai Lake (QH-06 and QH-07) were collected approximately 1.5 km from the northern shore and about 1.9 km from the southern coast, with a horizontal distance of approximately 34 m between the two cores (Figures 1B,C). The area around the cores is relatively flat, with a water depth of about 17 m. The sedimentary environment is stable, and the sedimentation rate is slow. The overall grain size of the sediments is fine, and the sediment primarily consists of red-brown silty clay. The grain size distribution of QH-06 and QH-07 is relatively uniform, with little variation in mean grain size, grain size deviation, and skewness.
CT scanning images reveal that the sediments in the QH-06 and QH-07 cores exhibit typical laminated structures (Figure 4). Most sediment layers show laminated structures, indicating seasonal alternations in sedimentary environments and representing normal background sediment characteristics. This suggests that the sedimentary environment in the lake’s central area has remained relatively stable over time. The mean grain size of the normal sediment layers is concentrated between 5 μm and 7 μm, with fine particles.
The sediment intervals at depths of 32–37 cm, 75–80 cm, 159–163 cm, and 166–168 cm in QH-06, and 40–45.5 cm, 85–89.5 cm, 163–168 cm, and 170–172 cm in QH-07, do not exhibit laminated structures in CT scanning images. The grain size analysis shows an increase in the mean grain size and mode, along with a smaller skewness, indicating that the sedimentary environment was disturbed by anomalous events. This suggests that, although the deep central parts of the lake are less affected by anomalous events, significant sedimentary responses to major disturbances are still observable in the lake center. Among these layers, the 75–80 cm and 166–168 cm layers of QH-06, as well as the 85–89.5 cm and 170–172 cm layers of QH-07, show homogeneous layer characteristics in both CT images and grain size distributions. Around the 168 cm depth in QH-06, Mn levels show a significant increase. Other anomalous sediment layers exhibit a trend of finer grain sizes upward, with notable increases in elements such as Si and Ti.
4.2.3 Southern lake core
Two lake cores, QH-11 and QH-12, were collected from the southern part of the lake. The distance between the two cores is 10 m, and the sampling site is approximately 580 m from the southern shore, with a water depth of about 16 m (Figures 1B,C). The sampling site is located on a relatively steep southern slope, but the sedimentary environment is overall stable, with a moderate sedimentation rate. The sediments are predominantly red-brown clay, interspersed with silt layers. The sedimentary characteristics of both cores show a good correlation, making them suitable for comparative analysis.
CT scan images of the lake cores reveal clear laminated structures in parts of the sediments, indicating normal background deposition (Figure 4), which reflects a stable sedimentary environment. The grain size of the normal background sediments is relatively fine, mainly ranging from 7 to 12 microns, with a uniform grain size distribution. In some sections of the QH-11 and QH-12 lake cores, sediments lacking clear laminated structures were observed. These layers show anomalous characteristics in terms of grain size, lamination structure, and geochemical element values. The sediment intervals at depths of 28–39 cm, 68–74 cm, 86–88 cm, 123–127 cm, 145–159 cm, 166–179 cm, and below 184 cm in the QH-11 core, as well as those at depths of 16–27.5 cm, 59.5–65.5 cm, 76–78 cm, 121–128 cm, 148–164 cm, 169–177 cm, and below 181 cm in the QH-12 core, correspond to anomalous deposits. These layers exhibit larger grain sizes and smaller skewness, with higher grain size deviation, showing significant differences compared to the normal background sediments. In particular, the sediment layers at depths of 68–74 cm in QH-11, and at depths of 59.5–65.5 cm and below 181 cm in QH-12, show minimal variation in grain size and are identified as homogeneous layers. At a depth of 74 cm in the QH-11 core, a sharp increase in Mn concentration is observed. The sediment characteristics at depths below 184 cm in the QH-11 core exhibit oscillatory variations in grain size. Other anomalous sediment layers show a trend of decreasing grain size with depth, along with an increase in the content of elements such as Si and Zr compared to the normal sediments.
4.3 Chronology
The results of the 210Pb and 137Cs measurements of the QH-06 lake core samples show that the specific activity of 137Cs increases and then decreases from top-down, with the maximum accumulation peak of 137Cs occurring at a depth of 64 cm, corresponding to the peak year of atmospheric nuclear tests in 1963. Based on the vertical variation in 210Pbex-specific activity, the Constant Rate of Supply (CRS) model was used to calculate the age sequence and calibrate the 210Pb ages using the 1963 137Cs peak as an independent age reference (DeMaster et al., 1985; Cai et al., 2015). This yielded a sedimentation rate of approximately 1 cm/year.
In 2023, Liu Xingqi’s team at Capital Normal University collected the QH-2019 lake core from Qionghai Lake and selected samples for 14C dating to construct an age-depth model (Sun et al., 2023) (Figure 3B). Both the QH-06 core and the QH-2019 core were collected from the flat central region of the lake (Figure 3A), with a water depth of around 16 m. According to the age-depth model developed by Liu’s team, the sedimentation rate for the top 6 m was approximately 0.7 cm/year, which is similar to the 1 cm/year rate obtained from the 137Cs/210Pb dating. Thus, the 137Cs/210Pb dating results are considered reliable Wei et al. (2014) used the three-parameter inverse Gamma probability distribution earthquake-triggered landslide model to inversely calculate the average annual sedimentation rate in Qionghai since 1850, which is 1.86 cm/year. Since Wei’s calculation is based on the average annual sedimentation rate for the entire lake, while this study obtained a sedimentation rate of 1 cm/year for the lake center through the lake center core, which is lower than 1.86 cm/year, the difference between the two is reasonable.
An age-depth model (Figure 3C) was constructed using the 137Cs/210Pb dating data. Common methods for constructing age-depth models include linear interpolation, polynomial regression, and spline interpolation (Bennett and Fuller, 2002). In this study, spline interpolation was chosen for constructing the age-depth model as it can effectively handle nonlinear variations between data points. Additionally, the interpolation results were smoothed to reduce the impact of potential fluctuations or outliers. The depth range of the predictive model was extended to 180 cm to cover deeper sediment layers that lacked direct age data.
4.4 Seismic intensity
In this study, we used the seismic intensity attenuation model for the southwest region, fitted by Lei et al. (2007) based on seismic data from Sichuan and the surrounding areas of Southwest China, to calculate the seismic intensity for historical earthquakes with a magnitude of Ms5.0 or above that occurred within 50 km of Qionghai since 1850. This model was derived from 96 earthquakes with magnitudes ranging from 4.0 to 7.8 in Southwest China between 1950 and 2006. The selection of the long and short axes in the intensity attenuation model depends on the positional relationship between Qionghai, the epicenter, and the fault orientation. If the line connecting Qionghai and the epicenter is parallel (or perpendicular) to the Anning River-Zemu River fault zone, the intensity values for the long (short) axis are chosen accordingly. The formulas are as follows:
Long axis:
Short axis:
Therein Ia and Ib represent the seismic intensity (MMI) along the long and short axes, respectively, M is the magnitude, R is the epicentral distance (km), and σ is the root mean square error (Lei et al., 2007).
Based on the calculation of seismic intensity for historical earthquakes since 1850, with epicentral distances within 50 km of Qionghai and magnitudes greater than Ms5.0 (Table 3), the following results were obtained: the 1850 CE earthquake (Ms7.5) had an intensity of 9.28 MMI at Qionghai; the 1952 CE earthquake (Ms6.75) had an intensity of 5.95 MMI at Qionghai; and the 1962 CE earthquake (Ms5.5) had an intensity of 4.44 MMI at Qionghai.

Table 3. Time, magnitude, and epicentral distance of historical earthquakes (Ms ≥ 5.0) within 50 km of Qionghai since 1850, and the corresponding seismic intensity observed in Qionghai.
5 Discussion
5.1 Correlation analysis between lake cores
Through the correlation between lake cores, the event-induced deposit layers in different cores can be further linked. These correlations help describe the response of disaster events in lake sediments on a basin-wide scale.
The six sediment cores demonstrate significant correlation, allowing for the association and correlation of anomalous event sediment layers across different cores using similar sedimentary facies, geochemical elements, and grain size characteristics, coupled with the thickness and properties of normal background sediment layers (Figure 4). These associations are then temporally aligned using age data, and some event layers are correlated with historical disaster records to facilitate further analysis and study. For example, cores QH-01 (50–88 cm), QH-02 (55–94 cm), QH-06 (32–37 cm), QH-07 (40–45.5 cm), QH-11 (28–39 cm), and QH-12 (16–27.5 cm) exhibit similar granulometric characteristics, characterized by a sudden increase in grain size and a gradational fining from bottom to top, with poor sorting. Additionally, the levels of elements such as Ti, Zr, and Si are found to be increasing. The depths of the anomalous sediment layers are similar across cores located at the same positions, with faster sedimentation rates observed in the northern and southern cores resulting in thicker upper normal sediment layers, while central cores exhibit slower sedimentation rates and thinner upper normal layers. Consequently, these anomalous layers can be associated as the sedimentary record of a single event. Based on the analysis of chronological data, this event is inferred to be the flood of 1998.
5.2 Normal background sediments
Normal background sediments exhibit clear laminated structures, indicating seasonal alternations in sedimentary environments and reflecting the stability of the sedimentary conditions (Figures 4–7). In the central region of the lake, where water flow is slower, fine-grained materials dominate, and the mean grain size typically ranges from 5 to 7 μm. In contrast, nearshore areas, such as the two lake cores in the northern part of the lake, contain coarser sediments due to the faster water flow near the river inlet, with the average grain size ranging from approximately 8–40 μm. The standard deviation is an important statistical measure for evaluating the dispersion of grain size distribution, quantifying the degree of deviation of sediment particle sizes from the arithmetic mean. Therefore, the standard deviation serves as a key indicator of sorting, reflecting the uniformity of grain sizes (Lu and Shi, 2010). Sediments under normal background conditions exhibit smaller standard deviations, indicating better sorting and further supporting the relative stability of the lake environment.
Previous studies on lake sediments have shown that principal component analysis of the elemental composition yields three end members. The first end-member is characterized by Ca and Sr, the second by Ti, Fe, Rb, K, Zr, and Si, and the third consists of only one element, Mn (Sun et al., 2023). The metalliferous sediments from watershed inputs are primarily composed of Ti, Fe, Rb, K, and Si, indicating terrestrial input. While primary sediments are dominated by Ca and Sr, reflecting endogenic processes within the lake, Calcium serves as a proxy for authigenic carbonates. While Mn may indicate redox or early diagenetic processes (Kylander et al., 2011; Wils et al., 2021a; Bertrand et al., 2024).
5.3 Event-induced deposits
Xichang Qionghai Lake is located at the intersection of the Anninghe Fault and Zemuhe Fault, within the Sichuan-Yunnan Seismic Belt, one of the most tectonically active regions in mainland China. Due to the high tectonic activity, the region experiences frequent earthquakes, with several moderate to strong seismic events recorded throughout history. For example, the Ms 7.5 Anninghe Fault earthquake in 1,536 and the Ms 7.5 Zemuhe Fault earthquake in 1850.
In addition, Xichang has a subtropical monsoon climate, with heavy and frequent rainfall, especially during the summer flood season, which makes the area highly susceptible to flooding and debris flow disasters. The mountainous terrain of the Qionghai watershed is steep, with loose soil structures, further exacerbated by vegetation degradation caused by human activities. Under conditions of heavy or continuous rainfall, debris flow disasters are easily triggered. For instance, on 6 July 1998, a century-level mountain flood disaster occurred in Guanbahe, where the flood carried large amounts of sediment and debris, resulting in severe destructive impacts. In 1853, a large debris flow also occurred in the Ezhanghe River.
As natural event recorders, lake sediments can respond to disaster events. By combining event-induced deposit layers in the Qionghai Lake sediments with age-dating data and historical disaster records, specific disaster event layers can be identified and analyzed (Figures 4–7).
5.3.1 The earthquake-induced sediments of 1850 CE and 1952 CE
Event layers I and III, found in all six sediment cores, exhibit similar characteristics. CT scan results indicate that these layers lack distinct lamination and show a significant increase in grain size, with the internal grain size variation being relatively uniform, presenting the characteristics of a homogenous layer. Additionally, a notable increase in Mn content is observed at the bottom of some event layers, such as at a depth of approximately 194.5 cm in core QH-01 and around 168 cm in core QH-06.
The historical seismic records indicate that a strong earthquake occurred in Xichang on 12 September 1850 CE. This was the largest earthquake in the Qionghai region in nearly three centuries (Ren and Li, 1993) and the most powerful earthquake ever recorded on the Zemu River fault, with a magnitude of M ≥ 7.5. The intensity in the Qionghai region reached level X. The earthquake caused significant surface damage and deformation, including the formation of large fault scarps. The seismic surface deformation zone extends from Qionghai in the north, passing through Daqingliangzi and gradually disappearing southeast of Ningnanbazi, with a total length of about 90 km (Yu et al., 2001). Typically, earthquakes with a magnitude of 6 or higher can cause noticeable surface ruptures. However, lake sediments are more sensitive to seismic activity, with a response threshold as low as magnitude 4 (McCalpin and Nelson, 2009). Therefore, the sediments in Qionghai should exhibit clear sedimentary disturbances in response to this earthquake. Based on age data, Event I closely corresponds to the 1850 CE Ms 7.5 earthquake, therefore it formed around that time and exhibits anomalous sediment layers in all four lake cores from Qionghai.
On 30 September 1952, a Ms 6.75 earthquake occurred in Shilong, Mianning County. This was the largest seismic event in the northern section of the Anning River fault zone since the 1536 CE Ms 7.5 magnitude earthquake in northern Xichang. The focal depth was between 7 and 9 km, and the intensity at the epicenter reached level IX. The epicenter was located 40.6 km from Qionghai, and the seismic waves affected the Xichang area, causing house collapses. By comparing the event layer depth and age dating results, Event III is closely dated to around 1952 CE, and therefore, it is associated with the 1952 Ms 6.75 earthquake in Mianning.
Geochemical data from the Event I and Event III sediment layers show that the contents of terrestrial elements such as Ti, Si, Fe, Rb, and K remained relatively stable (Figures 5–7). This suggests that the clastic particles from these events are primarily recycled materials from the lake. The mean grain size of the Event I and Event III layers shows a sharp increase, and the sediment structure lacks clear laminations, with very poor sorting. The grain size-related parameters within these layers show minimal variation, and they are mostly homogeneous (Figures 4–7), which is considered a seismic-induced homogeneous layer (Chapron et al., 1999). The formation of homogeneous layers in lake sediments due to seismic activity is primarily associated with the disturbance of lake-bottom sediments and the influence of seiche during the earthquake. Seismic waves trigger the formation of seiche within the lake, and these seiches, accompanied by strong water currents, can cause erosion and transport sediments from the shore areas to the lake’s sedimentary center, redistributing lake-bottom sediments and forming homogeneous layers (Ichinose et al., 2000; Avalos Cueva et al., 2019) (Figure 8A). Furthermore, underwater landslides triggered by seismic waves are also common causes of homogeneous layers (Bertrand et al., 2008; Praet et al., 2017) (Figure 8A). In his study of several lakes in the northern Alps, Beck (2009) proposed that seismic shaking triggered the remobilization of lacustrine sediments, generating turbidites in the central lake basins. These event layers exhibited minimal variations in grain size and other sedimentary parameters, demonstrating homogeneous characteristics. These slopes usually remain stable under static conditions but become unstable under seismic motion, leading to slope failure. The bottom of the landslide sediments is coarser and more poorly sorted. The Event I and Event III sediment layers in the lake cores QH-1, QH-2, QH-11, and QH-12 near the lake shore exhibit coarse grain sizes and poor sorting, which are consistent with landslide-induced sediments. Therefore, these layers are likely formed from slope failures in the lake caused by seismic vibrations. Additionally, the Event I layer in the QH-11 core shows a clear oscillatory pattern, with oscillations in the mean grain size and standard deviation (Figure 7). This characteristic is typically considered to be the result of seiche oscillations triggered by seismic activity (Petersen et al., 2014).
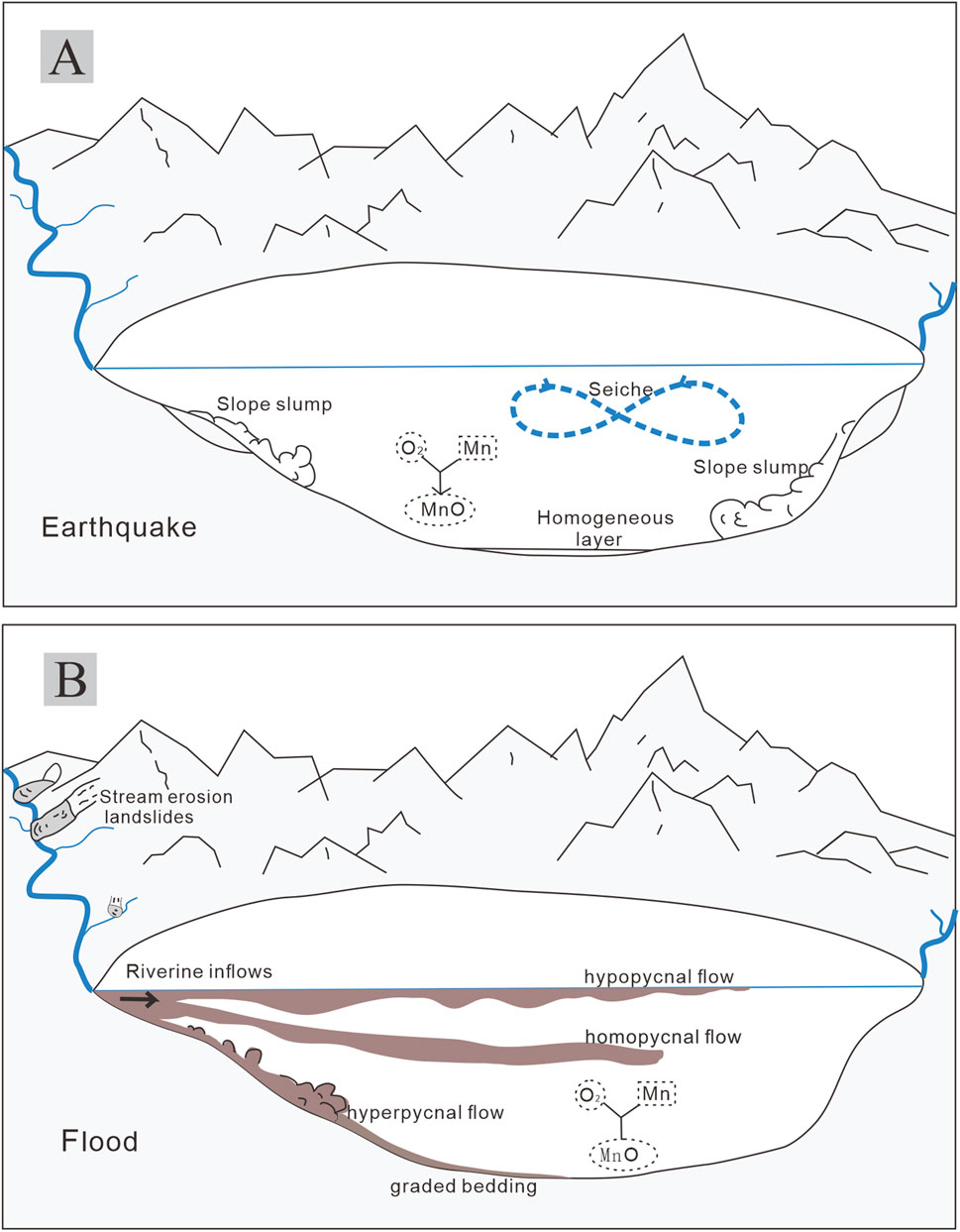
Figure 8. (A) Conceptual model diagram of sedimentary and geochemical response within the Qionghai Sea for seismic events. (B) Conceptual model diagram of sedimentary and geochemical process response within the Qionghai Sea for flood events.
Manganese (Mn) in the sediments serves as an important indicator of the redox state of bottom waters. Mn precipitation typically occurs under oxidizing conditions, forming either Mn oxides or Mn or Ca carbonates (Calvert and Pedersen, 1993; Wils et al., 2021b). Geochemical data show anomalously high Mn concentrations at the bottom of some earthquake event layers (Figures 5–7), such as the 1952 earthquake layer (Event III) at the base of cores QH-01 and QH-02. This phenomenon indicates that the bottom water in the lake was oxidizing at the time these layers formed. The high Mn concentration may be directly related to the lake water mixing processes triggered by the earthquake. During seismic events, seismic vibrations cause intense turbulence and mixing in the lake water, redistributing oxygen from the surface to the bottom layers and significantly increasing the dissolved oxygen levels at the lake bottom. In this process, manganese (Mn2+) is oxidized and precipitated, forming Mn oxides or Mn carbonates (Figure 8A). These chemical deposition processes further record the impact of the earthquake on the redox environment of the lake.
5.3.2 The flood-induced sediments of 1853 CE and 1998 CE
Event layers II and Ⅳ are also found in all six cores. CT scans reveal that these sediment layers lack lamination and display a significant increase in average grain size, which gradually fines upwards, creating a distinct normal grading characteristic. In these event layers, there is a marked increase in elements such as Ti, Fe, Rb, K, and Si, which represent minerals deposited from catchment inputs. Additionally, at the base of some event layers, a notable increase in manganese (Mn) content is observed, for example, at approximately 94 cm depth in core QH-01.
The Qionghai Basin has historically experienced frequent mountain floods and debris flow disasters. The large-scale flood in 1853 occurred following continuous heavy rainfall, which led to river surges and triggered landslides in the Ezhang River Basin. These landslides carried massive amounts of sediment, forming debris flows that rushed downstream. This flood and debris flow event is considered the largest in the Ezhang River Basin in a century. Based on age data, Event II is likely associated with the 1853 CE flood. In 1998, a once-in-a-century large-scale debris flow disaster occurred in the Guanba River, lasting nearly 2 h and flooding thousands of acres of farmland, with large amounts of sediment accumulating in Qionghai (Wei and Chen, 2018). Based on 137Cs dating results, the sediment layer of Event IV dates to around 1998, and this anomalous sediment layer is likely the sedimentary response to the 1998 flood event. Both of these large flood-debris flow events have been considered massive disasters in recent centuries and show clear sedimentary responses in the Qionghai sediments.
Geochemical data from Events II and IV indicate a significant increase in the concentrations of indicator elements such as Si, Ti, Fe, and Rb, which are associated with terrestrial clastic sediments (Figures 5–7). Excluding volcanic eruptions, floods are the most likely source of external sediment supply to Qionghai. The flood turbidites originate from river floods, which carry large amounts of terrestrial sediments, forming high-density water flows that enter Qionghai (Figure 8B). Flood turbidites typically show a trend of gradually finer grain size upwards, exhibiting distinct normal grading. Vandekerkhove et al. (2020) distinguished earthquake-triggered turbidites from flood-triggered turbidites in Eklutna Lake based on this characteristic. The sediment layers of Events II and IV show an increase in average grain size and exhibit a fining-upward gradational bedding, with poor sorting (Figures 5–7), indicating that these are flood deposition layers. Additionally, some event layers exhibit a sharp increase in Mn content at the bottom (Figures 5–7), which may be related to changes in the redox conditions at the sediment-water interface when the flood enters the lake. These redox changes lead to the precipitation of redox-sensitive chemicals, causing Mn to accumulate at the interface between oxygen-rich water and sediment (Calvert and Pedersen, 1993), resulting in elevated Mn concentrations at the bottom of certain event layers.
The sediments from these two large flood events can be found in all six lake cores (Figure 4), demonstrating the continuous sedimentary process in the lake related to these events. In terms of spatial distribution, the sediment layer thickness gradually decreases and grain size becomes finer from the lake shore to the lake center (Figure 4). Specifically, the sediment thickness for Event II on the northern shore at QH-01 and QH-02 is about 20 cm and 40 cm, respectively, while at the lake center, QH-06 and QH-07 have sediment layers of only about 5 cm, and closer to the southern shore, QH-11 and QH-12 have sediment layers about 10 cm thick. For Event IV, the sediment thickness on the northern shore at QH-01 and QH-02 is about 40 cm, while at the lake center (QH-06 and QH-07), the thinnest layer is 5 cm, and near the southern shore at QH-11 and QH-12, the sediment layers are about 10 cm thick. The grain size of the flood event sediment layers in the lake cores from the lake center is finer than that in the nearshore lake cores, indicating that the hydrodynamics of the flood turbidity current gradually weakened from the inlet area toward the lake center (Wilhelm et al., 2022). High-sediment-content floods and debris flows can penetrate deep into the bottom of Qionghai, forming turbidity currents that continue to move along the lakebed. When the flood density is greater than 1.001 g/cm3 and the lake water density is 1.0 g/cm3, turbidity currents can form. When the flood density exceeds 1.013 g/cm3, the turbidity current can remain stable and travel long distances; higher-density floods or debris flows can travel hundreds or even thousands of kilometers (Qian and Wan, 1999). Previous studies have shown that the sediment volume content of the debris flow before it entered Qionghai in 1998 was about 10%–20% in the fan area (Yu et al., 2005). Similarly, the sediment volume content of the 1853 debris flow was also high enough to enable the turbidity current to transport sediment across long distances on the lakebed to the lake center.
5.3.3 Other event-induced deposits
The Qionghai area is located at the intersection of the Anning River fault and the Zemu River fault, where geological activity is frequent. The tectonic movements cause surface fracturing and loose soil, providing ample sources of loose material for floods and debris flows. Additionally, the Qionghai area has a subtropical monsoon climate, with rainfall concentrated during the summer flood season. Short, intense rainfall or continuous rain often triggers mountain floods and debris flows, particularly during the summer flood season each year. Since the late 20th century, small floods and debris flows have occurred almost every year during the flood season, especially during years with extreme rainfall, when the frequency and scale are more significant. The occurrence frequency of small and medium-sized floods and debris flows is typically every 1–5 years, making them high-frequency events.
Other event-induced deposit layers exhibit graded bedding characteristics, accompanied by a trend of decreasing grain size (Figures 5–7), which is consistent with typical flood event layers. These event-induced deposit layers mainly appear in lake cores near the shore, further confirming their association with small and medium-sized flood events.
5.4 The seismic intensity that triggers seismically-induced deposits in lakes
The six lake cores from Qionghai record the 1850 CE Ms7.5 earthquake and the 1952 CE Ms6.75 earthquake in Events I and III, respectively. The 1850 CE Ms7.5 earthquake, recorded in Event I, produced a seismic intensity of 9.28 MMI at Qionghai, while the 1952 CE Ms6.75 earthquake, recorded in Event III, produced a seismic intensity of 5.95 MMI at Qionghai. Due to differences in slope, sediment sources, and sedimentation rates, different lakes exhibit varying seismic responses to ground shaking (Daxer et al., 2022). The Ms5.5 earthquake in 1962, 46.9 km from Qionghai, produced a seismic intensity of approximately 4.44 MMI at Qionghai. However, no corresponding sedimentary event response was found in the Qionghai cores. Based on this, it is inferred that the threshold for seismic response in Qionghai sediments lies within the range of greater than 4.44 MMI and less than or equal to 5.95 MMI.
6 Conclusion
Lake sediments preserve abundant information with high sensitivity, high resolution, long-term records, and wide geographic distribution, making them valuable for studying and reconstructing anomalous disaster events such as earthquakes and floods through multi-dimensional data. However, the origins of event-induced deposits are diverse, and accurately identifying their source is a key challenge.
High-resolution analysis of age dating, CT images, grain size, and geochemical elements from six short sediment cores collected from the northern, central, and southern parts of Qionghai Lake shows that the common characteristics of flood and earthquake-induced lake event deposits include a sharp increase in mean grain size, poor sorting, and abnormally high Mn content at the bottom of some event layers. The distinguishing characteristics of earthquake-induced deposits include relatively stable levels of terrestrial elements, such as Si and Rb, a lack of clear bedding structure by homogeneous layers, with some event layers displaying oscillatory grain size variations. The distinguishing characteristics of flood-induced sediments include a significant increase in the concentrations of terrestrial clastic elements, along with a gradual fining-upward trend in grain size, exhibiting distinct normal grading features. These characteristics provide a basis for studying ancient disaster events in lake sediments.
Furthermore, seismic intensity calculations for earthquakes surrounding Qionghai indicate that the threshold for earthquake-induced deposit responses in Qionghai Lake lies between 4.44 and 5.95 MMI.
Data availability statement
The raw data supporting the conclusions of this article will be made available by the authors, without undue reservation.
Author contributions
YL: Data curation, Methodology, Visualization, Writing – original draft, Writing – review and editing. LL: Writing – review and editing, Conceptualization, Funding acquisition, Investigation, Resources, Supervision. QL: Writing – review and editing. DL: Writing – review and editing. YJ: Writing – review and editing, Data curation.
Funding
The author(s) declare that financial support was received for the research and/or publication of this article. This study was financially supported by the National Institute of Natural Hazards, Ministry of Emergency Management of China (Grant No. ZDJ2019-14), the National Key Research and Development Program of China (Grant No. 2022YFF0801503), and the National Science Foundation of China (Grant No. 41471002).
Conflict of interest
The authors declare that the research was conducted in the absence of any commercial or financial relationships that could be construed as a potential conflict of interest.
Generative AI statement
The author(s) declare that no Generative AI was used in the creation of this manuscript.
Publisher’s note
All claims expressed in this article are solely those of the authors and do not necessarily represent those of their affiliated organizations, or those of the publisher, the editors and the reviewers. Any product that may be evaluated in this article, or claim that may be made by its manufacturer, is not guaranteed or endorsed by the publisher.
References
Araya, K., Dezileau, L., Munoz, P., Maldonado, A., Condomines, M., Khalfaoui, O., et al. (2024). Reconstruction of extreme floods and tsunamis from coastal sedimentary archives in Los Choros, Coquimbo region, 28°S, Chile. Nat. Hazards 120, 11323–11347. doi:10.1007/s11069-024-06644-8
Avalos Cueva, D., Monzon, C. O., Filonov, A., Tereshchenko, I., Limon Covarrubias, P., and Galaviz Gonzalez, J. R. (2019). Natural frequencies of seiches in Lake chapala. Sci. Rep. 9 (1), 11863. doi:10.1038/s41598-019-48319-6
Azennoud, K., Baali, A., El Asmi, H., Brahim, Y. A., Hakam, O., Hayati, A., et al. (2022). Soft-sediment deformation structures recognised in a reverse-drag associated with normal faulting (Lake Ifrah, Northwest Africa): palaeoseismic assessment and neotectonic implications. Sediment. Geol. 441, 106264. doi:10.1016/j.sedgeo.2022.106264
Banjan, M., Crouzet, C., Sabatier, P., Jomard, H., Bajard, M., Demory, F., et al. (2023). Did the Younger Dryas to Holocene climate transition favour high seismicity rates in the north-western Alps? Sedimentology 70 (2), 538–568. doi:10.1111/sed.13050
Beck, C. (2009). Late Quaternary lacustrine paleo-seismic archives in north-western Alps: examples of earthquake-origin assessment of sedimentary disturbances. Earth-Science Rev. 96 (4), 327–344. doi:10.1016/j.earscirev.2009.07.005
Bennett, K. D., and Fuller, J. L. (2002). Determining the age of the mid-Holocene Tsuga canadensis (hemlock) decline, eastern North America. Holocene 12 (4), 421–429. doi:10.1191/0959683602hl556rp
Bertrand, S., Charlet, F., Chapron, E., Fagel, N., and De Batist, M. (2008). Reconstruction of the Holocene seismotectonic activity of the southern andes from seismites recorded in lago icalma, Chile, 39°S. Palaeogeogr. Palaeoclimatol. Palaeoecol. 259 (2-3), 301–322. doi:10.1016/j.palaeo.2007.10.013
Bertrand, S., Tjallingii, R., Kylander, M. E., Wilhelm, B., Roberts, S. J., Arnaud, F., et al. (2024). Inorganic geochemistry of lake sediments: a review of analytical techniques and guidelines for data interpretation. Earth-Science Rev. 249, 104639. doi:10.1016/j.earscirev.2023.104639
Biguenet, M., Sabatier, P., Chaumillon, E., Chague, C., Arnaud, F., Jorissen, F., et al. (2021). A 1600 year-long sedimentary record of tsunamis and hurricanes in the Lesser Antilles (Scrub Island, Anguilla). Sediment. Geol. 412, 105806. doi:10.1016/j.sedgeo.2020.105806
Cai, Q., Jia, P., and Shao, C. (2015). The application of 210Pb and 137Cs dating in the study of the ancient environment evolution of China coastal zone. Jilin Geol. 34 (02), 107–111. doi:10.3969/j.issn.1001-2427.2015.02.024
Calvert, S. E., and Pedersen, T. F. (1993). Geochemistry of recent oxic and anoxic marine sediments: implications for the geological record. Mar. Geol. 113 (1-2), 67–88. doi:10.1016/0025-3227(93)90150-t
Chapron, E., Beck, C., Pourchet, M., and Deconinck, J. F. (1999). 1822 earthquake-triggered homogenite in Lake le bourget (NW Alps). Terra nova. 11 (2-3), 86–92. doi:10.1046/j.1365-3121.1999.00230.x
Chen, S., Wang, S., Chen, Y., Zhang, E., Chen, Y., and Zhu, Y. (2009). Vertical distribution and chronological implication of 210Pb and 137Cs in sediments of dongping lake, shandong Province. Quat. Sci. 29 (5), 981–987. doi:10.3969/j.issn.1001-7410.2009.05.16
Colbo, K., Ross, T., Brown, C., and Weber, T. (2014). A review of oceanographic applications of water column data from multibeam echosounders. Estuar. Coast. Shelf Sci. 145, 41–56. doi:10.1016/j.ecss.2014.04.002
Constantinescu, A. M., Tyler, A. N., Stanica, A., Spyrakos, E., Hunter, P. D., Catianis, I., et al. (2023). A century of human interventions on sediment flux variations in the Danube-Black Sea transition zone. Front. Mar. Sci. 10, 1068065. doi:10.3389/fmars.2023.1068065
Dai, K., Tie, Y., Xu, Q., Feng, Y., Zhuo, G., and Shi, X. (2020). Early identification of potential landslide geohazards in alpine-canyon terrain based on SAR interferometry-a case study of the middle section of Yalong River. J. Radars 9 (3), 554–568. doi:10.12000/JR20012
Daxer, C., Ortler, M., Fabbri, S. C., Hilbe, M., Hajdas, I., Dubois, N., et al. (2022). High-resolution calibration of seismically-induced lacustrine deposits with historical earthquake data in the Eastern Alps (Carinthia, Austria). Quat. Sci. Rev. 284, 107497. doi:10.1016/j.quascirev.2022.107497
DeMaster, D. J., McKee, B. A., Nittrouer, C. A., Jiangchu, Q., and Guodong, C. (1985). Rates of sediment accumulation and particle reworking based on radiochemical measurements from continental shelf deposits in the East China Sea. Cont. Shelf Res. 4 (1), 143–158. doi:10.1016/0278-4343(85)90026-3
Diaz, C., Moreno, P. I., Villacis, L. A., Sepulveda-Zuniga, E. A., and Maidana, N. I. (2023). Freshwater diatom evidence for Southern Westerly Wind evolution since-18 ka in northwestern Patagonia. Quat. Sci. Rev. 316, 108231. doi:10.1016/j.quascirev.2023.108231
Dong, Y., Zhang, P., Li, X., and Zheng, B. (2024). Spatio-temporal distribution of aquatic macrophytes and their causes of degradation in Lake Qionghai. J. Lake Sci. 36 (1), 236–246. doi:10.18307/2024.0135
Du, P. (2000). Slip displacement and its rate about Zemuhe faults. Earthq. Res. Sichuan 1-2, 49–64. doi:10.3969/j.issn.1001-8115.2000.02.004
Eltom, H. A. (2020). Limitation of laboratory measurements in evaluating rock properties of bioturbated strata: a case study of the Upper Jubaila Member in central Saudi Arabia. Sediment. Geol. 398, 105573. doi:10.1016/j.sedgeo.2019.105573
Fan, X., Gong, X., Cai, F., and Huang, D. (2023). Dynamic changes of locking and slip distribution in the anninghe-zemuhe Fault Zone. J. Geodesy Geodyn. 43 (9), 914–918. doi:10.14075/j.jgg.2023.09.007
Gastineau, R., Sabatier, P., Fabbri, S. C., Anselmetti, F. S., Roeser, P., Findling, N., et al. (2023). Lateral variations in the signature of earthquake-generated deposits in Lake Iznik, NW Turkey. Depositional Rec. 10 (5), 470–495. doi:10.1002/dep2.232
Ghazoui, Z., Bertrand, S., Vanneste, K., Yokoyama, Y., Nomade, J., Gajurel, A. P., et al. (2019). Potentially large post-1505 AD earthquakes in western Nepal revealed by a lake sediment record. Nat. Commun. 10 (1), 2258. doi:10.1038/s41467-019-10093-4
Horvei, B., and Nilsen, K. E. (2010). A new high resolution wideband multibeam echo sounder for inspection work and hydrographic mapping. Ocean. 2010 MTS, 1–7. doi:10.1109/OCEANS.2010.5664080
Ichinose, G. A., Anderson, J. G., Satake, K., Schweickert, R. A., and Lahren, M. L. (2000). The potential hazard from tsunami and Seiche waves generated by large earthquakes within Lake Tahoe, California-Nevada. Geophys. Res. Lett. 27 (8), 1203–1206. doi:10.1029/1999gl011119
Ierodiaconou, D., Schimel, A. C. G., Kennedy, D., Monk, J., Gaylard, G., Young, M., et al. (2018). Combining pixel and object based image analysis of ultra-high resolution multibeam bathymetry and backscatter for habitat mapping in shallow marine waters. Mar. Geophys. Res. 39 (1-2), 271–288. doi:10.1007/s11001-017-9338-z
Knapp, S., Gilli, A., Anselmetti, F. S., Krautblatter, M., and Hajdas, I. (2018). Multistage rock-slope failures revealed in lake sediments in a seismically active Alpine region (Lake Oeschinen, Switzerland). J. Geophys. Research-Earth Surf. 123 (4), 658–677. doi:10.1029/2017jf004455
Kusnierz, M., and Lomotowski, J. (2015). Using Avrami equation in the studies on changes in granulometric composition of algal suspension. Hydrobiologia 758 (1), 243–255. doi:10.1007/s10750-015-2294-1
Kylander, M. E., Ampel, L., Wohlfarth, B., and Veres, D. (2011). High-resolution X-ray fluorescence core scanning analysis of Les Echets (France) sedimentary sequence: new insights from chemical proxies. J. Quat. Sci. 26 (1), 109–117. doi:10.1002/jqs.1438
Lan, J., Xu, H., Lang, Y., Yu, K., Zhou, P., Kang, S., et al. (2020). Dramatic weakening of the east asian summer monsoon in northern China during the transition from the medieval warm period to the little ice age. Geology 48 (4), 307–312. doi:10.1130/g46811.1
Lei, J., Gao, M., and Yu, Y. (2007). Seismic motion attenuation relations in Sichuan and adjacent areas. Acta Seismol. Sin. 29 (5), 500–511. doi:10.3321/j.issn:0253-3782.2007.05.007
Li, D., Li, L., Ma, B., and Zhang, J. (2021). Characteristics of lake sediment response to earthquakes and the reconstruction of paleoseismic sequences. Earth Sci. Front. 28 (2), 232–245. doi:10.13745/j.esf.sf.2020.9.15
Li, G., Wang, X., Yan, M., Chen, W., Zhang, Q., Zhang, E., et al. (2023). Spatial-temporal variations and pollution assessment of heavy metals in sediments of Qionghai, Lake in Xichang City. Huanjing Kexue 44 (10), 5536–5545. doi:10.13227/j.hjkx.202211058
Li, H., Wang, Z., Fu, X., Hou, L., Si, J., Qiu, Z., et al. (2008). The surface rupture zone distribution of the Wenchuan earthquake (Ms8.0) happened on May 12th, 2008. Geol. China 35 (5), 803–813. doi:10.3969/j.issn.1000-3657.2008.05.002
Li, J., Zhou, B., Li, T., Yang, Y., Li, Z., and Long, F. (2020). Seismogenic depths of the Anninghe-Zemuhe and Daliangshan fault zones and their seismic hazards. Chin. J. Geophysics-Chinese Ed. 63 (10), 3669–3682. doi:10.6038/cjg2020N0201
Liao, S., Qin, Y., Liu, Z., Yang, C., Shi, Y., Ma, Y., et al. (2022). Characteristics of soil nutrients and groundwater pollution of greenhouse vineyards in Qionghai Lake Basin. J. Environ. Eng. Technol. 12 (2), 597–606. doi:10.12153/j.issn.1674-991X.20210497
Liu, B., Sheng, E., Yu, K., Zhou, K. e., and Lan, J. (2022). Variations in monsoon precipitation over southwest China during the last 1500 years and possible driving forces. Sci. China-Earth Sci. 65 (5), 949–965. doi:10.1007/s11430-021-9888-y
Liu, G., Wu, J., Zhou, C., Ni, T., Wei, M., and Wang, S. (2023). Anisotropy of middle-upper crust in the Anninghe-Zemuhe fault zone and its adjacent area and its implications. Chin. J. Geophysics-Chinese Ed. 66 (11), 4533–4551. doi:10.6038/cjg2023Q0585
Lu, L., and Shi, Z. (2010). Analysis for sediment grain size parameters of connotations and calculation method. Environ. Sci. Manag. 35 (06), 54–60. doi:10.3969/j.issn.1673-1212.2010.06.013
Magno, M. C., Venti, F., Bergamin, L., Gaglianone, G., Pierfranceschi, G., and Romano, E. (2018). A comparison between Laser Granulometer and Sedigraph in grain size analysis of marine sediments. Measurement 128, 231–236. doi:10.1016/j.measurement.2018.06.055
Maleika, W. (2020). Inverse distance weighting method optimization in the process of digital terrain model creation based on data collected from a multibeam echosounder. Appl. Geomatics 12 (4), 397–407. doi:10.1007/s12518-020-00307-6
McCalpin, J. P., and Nelson, A. R. (2009). Introduction to paleoseismology. California: Academic Press.
Molenaar, A., Van Daele, M., Vandorpe, T., Degenhart, G., De Batist, M., Urrutia, R., et al. (2021). What controls the remobilization and deformation of surficial sediment by seismic shaking? Linking lacustrine slope stratigraphy to great earthquakes in South-Central Chile. Sedimentology 68 (6), 2365–2396. doi:10.1111/sed.12856
Monecke, K., Anselmetti, F. S., Becker, A., Sturm, M., and Giardini, D. (2004). The record of historic earthquakes in lake sediments of Central Switzerland. Tectonophysics 394 (1-2), 21–40. doi:10.1016/j.tecto.2004.07.053
Montereale-Gavazzi, G., Roche, M., Lurton, X., Degrendele, K., Terseleer, N., and Van Lancker, V. (2018). Seafloor change detection using multibeam echosounder backscatter: case study on the Belgian part of the North Sea. Mar. Geophys. Res. 39 (1-2), 229–247. doi:10.1007/s11001-017-9323-6
Oswald, P., Strasser, M., Hammerl, C., and Moernaut, J. (2021). Seismic control of large prehistoric rockslides in the Eastern Alps. Nat. Commun. 12 (1), 1059. doi:10.1038/s41467-021-21327-9
Owen, G., Moretti, M., and Alfaro, P. (2011). Recognising triggers for soft-sediment deformation: current understanding and future directions. Sediment. Geol. 235 (3-4), 133–140. doi:10.1016/j.sedgeo.2010.12.010
Petersen, J., Wilhelm, B., Revel, M., Rolland, Y., Crouzet, C., Arnaud, F., et al. (2014). Sediments of Lake Vens (SW European Alps, France) record large-magnitude earthquake events. J. Paleolimnol. 51 (3), 343–355. doi:10.1007/s10933-013-9759-x
Praet, N., Moernaut, J., Van Daele, M., Boes, E., Haeussler, P. J., Strupler, M., et al. (2017). Paleoseismic potential of sublacustrine landslide records in a high-seismicity setting (south-central Alaska). Mar. Geol. 384, 103–119. doi:10.1016/j.margeo.2016.05.004
Praet, N., Van Daele, M., Collart, T., Moernaut, J., Vandekerkhove, E., Kempf, P., et al. (2020). Turbidite stratigraphy in proglacial lakes: deciphering trigger mechanisms using a statistical approach. Sedimentology 67 (5), 2332–2359. doi:10.1111/sed.12703
Qian, N., and Wan, Z. (1999). Mechanics of sediment transport. Reston: American Society of Civil Engineers Press.
Ran, Y., Cheng, J., Gong, H., and Chen, L. (2008). Late Quaternary geomorphic deformation and displacement rates of the Anninghe fault around Zimakua. Seismol. Geol. 30 (1), 86–98. doi:10.3969/j.issn.0253-4967.2008.01.006
Rapuc, W., Sabatier, P., Arnaud, F., Palumbo, A., Develle, A.-L., Reyss, J.-L., et al. (2019). Holocene-long record of flood frequency in the Southern Alps (Lake Iseo, Italy) under human and climate forcing. Glob. Planet. Change 175, 160–172. doi:10.1016/j.gloplacha.2019.02.010
Ren, C., and Zheng, B. (2024). Trend recognition and driving factors of water quality change in plateau lakes: a case study of Lake Qionghai, Sichuan Province. J. Lake Sci. 36 (3), 756–769. doi:10.18307/2024.0323
Ren, J., and Li, P. (1993). The characteristics of surface faulting of 1850 earthquake in Xichang, Sichuan. Seismol. Geol. 15 (2), 97–108.
Ren, Z., and Lin, A. (2010). Deformation characteristics of co-seismic surface ruptures produced by the 1850 M 7.5 Xichang earthquake on the eastern margin of the Tibetan Plateau. J. Asian Earth Sci. 38 (1-2), 1–13. doi:10.1016/j.jseaes.2009.12.008
Ross, P. S., Bourke, A., and Fresia, B. (2013). A multi-sensor logger for rock cores: Methodology and preliminary results from the Matagami mining camp, Canada. Ore Geol. Rev. 53, 93–111. doi:10.1016/j.oregeorev.2013.01.002
Shah, R. A., Achyuthan, H., Lone, A. M., Kumar, S., Kumar, P., Sharma, R., et al. (2020). Holocene palaeoenvironmental records from the high-altitude wular lake, western himalayas. Holocene 30 (5), 733–743. doi:10.1177/0959683619895592
Shi, Y., Gao, Y., Zhang, H., Zhang, Z., and Li, G. (2023a). Crustal azimuthal anisotropy in the lateral collision zone of the SE margin of the Tibetan Plateau and its tectonic implications. Geophys. J. Int. 234 (1), 1–11. doi:10.1093/gji/ggad059
Shi, Y., Zhang, L., Qin, Y., Ma, Y., Yang, C., Liu, Z., et al. (2023b). Temporal and spatial distribution characteristics of nitrogen and phosphorous in the water of Qionghai Lake, Sichuan Province and their response to ecological environment. Earth Sci. Front. 30 (2), 495–505. doi:10.13745/j.esf.sf.2022.2.86
Strasser, M., Anselmetti, F. S., Faeh, D., Giardini, D., and Schnellmann, M. (2006). Magnitudes and source areas of large prehistoric northern Alpine earthquakes revealed by slope failures in lakes. Geology 34 (12), 1005–1008. doi:10.1130/g22784a.1
Strasser, M., Monecke, K., Schnellmann, M., and Anselmetti, F. S. (2013). Lake sediments as natural seismographs: a compiled record of Late Quaternary earthquakes in Central Switzerland and its implication for Alpine deformation. Sedimentology 60 (1), 319–341. doi:10.1111/sed.12003
Sun, H., Liu, X., Mao, X., Jia, W., and Herzschuh, U. (2023). Centennial-scale variability of the Indian Summer Monsoon during the middle to late Holocene and its links with ENSO activity. Palaeogeogr. Palaeoclimatol. Palaeoecol. 612, 111380. doi:10.1016/j.palaeo.2022.111380
Van Daele, M., Moernaut, J., Doom, L., Boes, E., Fontijn, K., Heirman, K., et al. (2015). A comparison of the sedimentary records of the 1960 and 2010 great Chilean earthquakes in 17 lakes: implications for quantitative lacustrine palaeoseismology. Sedimentology 62 (5), 1466–1496. doi:10.1111/sed.12193
Vandekerkhove, E., Van Daele, M., Praet, N., Cnudde, V., Haeussler, P. J., and De Batist, M. (2020). Flood-triggered versus earthquake-triggered turbidites: a sedimentological study in clastic lake sediments (Eklutna Lake, Alaska). Sedimentology 67 (1), 364–389. doi:10.1111/sed.12646
Wang, L., Guo, H., Liu, Y., Dai, Y., and Wang, J. (2005). Ecological fragility of Qionghai Lake basin and its asssessment. Chin. J. Ecol. 24 (10), 1192–1196. doi:10.13292/j.1000-4890.2005.0126
Wei, X., and Chen, N. (2018). Development of debris flows in Guanba River and its effect on sediment deposition in Qionghai Lake of sichuan. Acta Geogr. Sin. 73 (1), 81–91. doi:10.11821/dlxb201801007
Wei, X., Chen, N., Cheng, Q., He, N., Deng, M., and Tanoli, J. I. (2014). Long-term activity of earthquake-induced landslides: a case study from Qionghai Lake Basin, Southwest of China. J. Mt. Sci. 11 (3), 607–624. doi:10.1007/s11629-013-2970-4
Wen, X., Fan, J., Yi, G., Deng, Y., and Long, F. (2008). A seismic gap on the Anninghe fault in western Sichuan, China. Sci. China Earth Sci. 38 (07), 797–807. doi:10.3321/j.issn:1006-9267.2008.07.002
Wen, X., Ma, S., Lei, X., Yasuto, N., Tsutomu, K., and Chen, Q. (2007). Newly found surface rupture remains of large historical earthquakes on and near the transition segment of the Anninghe and Zemuhe Fault zones, western Sichuan, China. Seismol. Geol. 29 (4), 826–833. doi:10.3969/j.issn.0253-4967.2007.04.013
Wilhelm, B., Amann, B., Corella, J. P., Rapuc, W., Giguet-Covex, C., Merz, B., et al. (2022). Reconstructing paleoflood occurrence and magnitude from lake sediments. Quaternary 5 (1), 9. doi:10.3390/quat5010009
Wilhelm, B., Arnaud, F., Sabatier, P., Magand, O., Chapron, E., Courp, T., et al. (2013). Palaeoflood activity and climate change over the last 1400 years recorded by lake sediments in the north-west European Alps. J. Quat. Sci. 28 (2), 189–199. doi:10.1002/jqs.2609
Wils, K., Daryono, M. R., Praet, N., Santoso, A. B., Dianto, A., Schmidt, S., et al. (2021a). The sediments of Lake Singkarak and Lake Maninjau in West Sumatra reveal their earthquake, volcanic and rainfall history. Sediment. Geol. 416, 105863. doi:10.1016/j.sedgeo.2021.105863
Wils, K., Deprez, M., Kissel, C., Vervoort, M., Van Daele, M., Daryono, M. R., et al. (2021b). Earthquake doublet revealed by multiple pulses in lacustrine seismo-turbidites. Geology 49 (11), 1301–1306. doi:10.1130/g48940.1
Wu, W. (2020). Stress distribution characteristics of faults intersection in Anninghe-Zemuhe Fault Zone and its adjacent area. J. Seismol. Res. 43 (4), 601–609. doi:10.3969/j.issn.1000-0666.2020.04.001
Wu, W., Wu, P., Wei, Y., and Sun, W. (2017). Regional characteristics of stress state of main seismic active faults in mid-northern part of Sichuan-Yunnan block. Chin. J. Geophys. 60 (5), 1735–1745. doi:10.6038/cjg20170511
Wu, Z., Zhao, G., Long, Z., Zhou, C., and Fan, T. (2014). The seismic hazard assessment around south-east area of Qinghai-Xizang plateau:A preliminary results from active tectonics system analysis. Acta Geol. Sin. 88 (08), 1401–1416. doi:10.19762/j.cnki.dizhixuebao.2014.08.004
Xiao, X., Yao, A., Hillman, A., Shen, J., and Haberle, S. G. (2020). Vegetation, climate and human impact since 20 ka in central Yunnan Province based on high-resolution pollen and charcoal records from Dianchi, southwestern China. Quat. Sci. Rev. 236, 106297. doi:10.1016/j.quascirev.2020.106297
Xu, X., Zhang, P., Wen, X., Qin, Z., Chen, G., and Zhu, A. (2005). Features of active tectonics and recurrence behaviors of strong earthquakes in the western Sichuan Province and its adjacent regions. Seismol. Geol. 27 (3), 446–461. doi:10.3969/j.issn.0253-4967.2005.03.010
Yi, J., Wang, F., Cheng, Y., and Zhang, Y. (2022). Study on the risk assessment of geological disasters in alpine valley area:A case study in Aba County,Sichuan Province. Chin. J. Geol. Hazard Control 33 (3), 134–142. doi:10.16031/j.cnki.issn.1003-8035.2022.03-15
Yu, B., Zhang, S., and Wang, S. (2005). A preliminary study on the deposition of turbidity currents in Qionghai Lake, Sichuan, China. Acta Sedimentol. Sin. 23 (4), 559–565. doi:10.3969/j.issn.1000-0550.2005.04.001
Yu, H., Zhang, Z., Hu, F., Xu, D., and Chen, X. (2023a). Estimation of the nucleation location and rupture extent of the 1850 Xichang, Sichuan, China, Earthquake by dynamic rupture simulations on a multi-segment stepover structure. Earth Space Sci. 10 (6), e2022EA002775. doi:10.1029/2022ea002775
Yu, W., Song, F., Wen, X., and Li, C. (2001). Study of surface rupture zone of Xichang earthquake in 1850. J. Seismol. Res. 24 (4), 346–350. doi:10.3969/j.issn.1000-0666.2001.04.011
Yu, X., Wang, G., Zhang, T., Ma, X., Zhang, X., Li, L., et al. (2023b). Chemical weathering intensity as a reliable indicator for southwest summer monsoon reconstruction: evidence from clay minerals of Qionghai Lake sediments since the Last Glacial Maximum. Geochem. Geophys. Geosystems 24 (7), e2023GC010900. doi:10.1029/2023gc010900
Zhang, L., Zhou, Y., Zhang, X., Zhu, A., Li, B., Wang, S., et al. (2023). 2022 Mw6.6 luding, China, earthquake: a strong continental event illuminating the moxi seismic gap. Seismol. Res. Lett. 94 (5), 2129–2142. doi:10.1785/0220220383
Zhong, N., Jiang, H., Li, H., Su, D., Xu, H., Liang, L., et al. (2022). The potential of using soft-sediment deformation structures for quantitatively reconstructing paleo-seismic shaking intensity: progress and prospect. Environ. Earth Sci. 81 (16), 408. doi:10.1007/s12665-022-10504-8
Keywords: Qionghai lacustrine sediments, event-induced deposits(EID), earthquake, flood, abnormal deposit recognition mark
Citation: Li Y, Li L, Li Q, Li D and Jiang Y (2025) Recognition of the flood- and earthquake-induced deposits in Qionghai Lake: implications for the quantitative analysis of abnormal lacustrine sediments. Front. Earth Sci. 13:1574115. doi: 10.3389/feart.2025.1574115
Received: 10 February 2025; Accepted: 11 April 2025;
Published: 29 April 2025.
Edited by:
Xingqi Liu, Capital Normal University, ChinaReviewed by:
Jiawei Fan, Institute of Geology, China Earthquake Administration, ChinaNorihiro Nakamura, Tohoku University, Japan
Copyright © 2025 Li, Li, Li, Li and Jiang. This is an open-access article distributed under the terms of the Creative Commons Attribution License (CC BY). The use, distribution or reproduction in other forums is permitted, provided the original author(s) and the copyright owner(s) are credited and that the original publication in this journal is cited, in accordance with accepted academic practice. No use, distribution or reproduction is permitted which does not comply with these terms.
*Correspondence: Linlin Li, bGVlaW5Ac2luYS5jb20=