- Department of Earth, Environmental and Planetary Sciences, University of Tennessee, Knoxville, TN, United States
Early diagenetic chert serves as a critical archive of life on Earth, yet the mechanisms of chert formation and diagenesis remain uncertain. The present research deciphers chert formation and recrystallization through petrographic observations of Proterozoic microfossiliferous chert and explores its relationship to microfossil preservation. Petrographic analyses reveal that the primary chert fabric consists of a network of spherules that consist of chalcedony fibers that radiate outward from a central nucleation point. Original spherules then undergo neomorphic recrystallization that results in systematic grain coarsening and a range of distinctive textures. Subsequent recrystallization can largely erase evidence of primary spherules, but often maintains distinct internal domains within crystals that exhibit sweeping extinction consistent with initial spherulitic growth. We attribute the range of neomorphic features described here to a combination of 1) growth of initial chalcedony spherules within a silica gel that permeates the primary substrate, 2) the degree of alignment of the chalcedony fibers within and between adjacent spherules, 3) the behavior of the amorphous silica component within and external to chalcedony spherules during early neomorphic recrystallization, and 4) coalescence of adjacent grains with similar lattice orientation. Notably, in nearly all cases, remarkable fidelity is maintained in the preservation of microfossil morphology and primary sedimentary fabrics. These observations lead us to a refined model for microfossil silicification and emphasizes both the complex role of neomorphism in chert formation and the low levels of water-rock interaction required for the neomorphic process.
1 Introduction
Chert is a fine-grained chemically precipitated sedimentary rock composed of silicon dioxide (>95% SiO2). The silica that comprises chert can consist of a variety of polymorphs and classification of these polymorphs is complex because distinct crystallographic forms are often referred to by different names (Table 1). Chert is most commonly described by crystal size (e.g., cryptocrystalline or microcrystalline, with the macrocrystalline form is generally referred to as mesoquartz or megaquartz; Hesse, 1989; Hendry and Trewin, 1995), or by crystal habit (e.g., fibrous, bladed, or equant). In turn, crystal habit is commonly linked to additional terms, wherein fibrous crystals are generally termed chalcedony, which is then subdivided as either parabolic fiber bundles or radiating spherulites (Heaney, 1993; 1995; Graetsch et al., 1994; Cady et al., 1998; Cady and Farmer, 2007; Moxon et al., 2006), and equant crystals are more generically referred to simply as chert or quartz. More explicitly, terminology for chert can also recognize variation in lattice structure, with opal-A, opal-C, opal-CT, and quartz recording, respectively, greater lattice organization. (Flörke et al., 1991; Graetsch et al., 1994; Lynne and Campbell, 2004). Finally, additional names are given to chert that shows specific variation of lattice structure with respect to crystal morphology, wherein microcrystalline quartz is commonly subdivided into chalcedony (length-fast), and quartzine or moganite (length-slow) (Heaney, 1995).
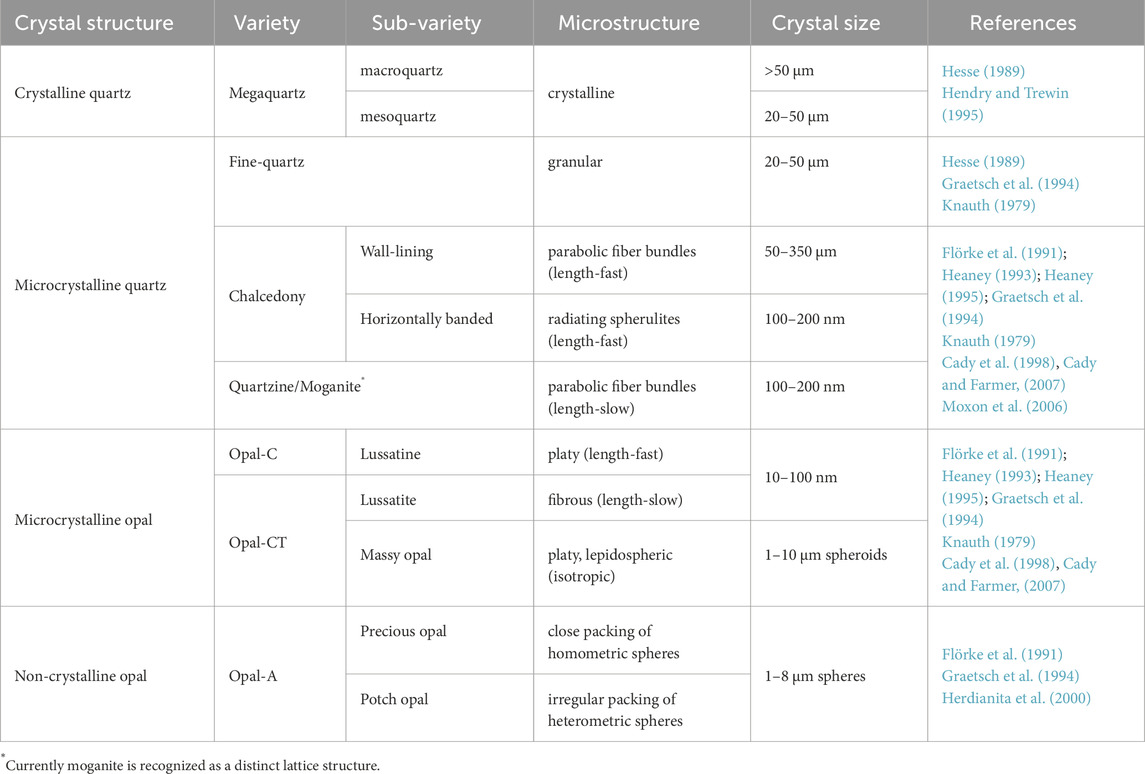
Table 1. Nomenclature and characteristics of selected silica phases (adapted from Flörke et al., 1991).
Precipitation of chert commonly occurs near the Earth’s surface as both a void-filling cement, and as a replacement of a precursor organic or mineral phases during early diagenesis. Primary precipitation of chert commonly consists of both metastable and stable silica phases, and diagenetic recrystallization of these phases typically results in higher quartz-to-opal ratio with increasing geologic age (Laschet, 1984).
Early diagenetic chert is perhaps most well-known for containing fossilized microorganisms that offer a unique glimpse into early life on Earth (Barghoorn and Tyler, 1965; Schopf, 1968; Schopf, 1993; Brasier et al., 2004; Brasier et al., 2005; Wacey et al., 2006; Schopf and Kudryavtsev, 2012). Microscopic remains of benthic microbial mats and their specific associations of coccoidal and filamentous bacteria provide tangible evidence of early biological communities and interactions with their environment (Hofmann, 1975; Knoll and Golubic, 1979; Kah and Knoll, 1996; Knoll et al., 2013). Perhaps more importantly, mimetic preservation of microbial mats and their associated geologic substrates also places valuable constraints on the synsedimentary processes responsible for their preservation. Thus far, however, a limited number of studies have addressed chert microfabrics associated with microorganisms. Rather, most studies focus primarily on determining the biogenicity of microfossil remains (Buick, 1990; Brasier et al., 2004; 2005; Wacey et al., 2006; De Gregorio et al., 2009; Schopf and Kudryavtsev, 2012; Dodd et al., 2017), the taxonomic variety of microfossils (Schopf, 1968; Knoll et al., 2013; Knoll, 1985; Hofmann and Jackson, 1991; Peng et al., 2016), or the quality of microbe preservation and taphonomic processes associated with the fossilization processes (Knoll and Sergeev, 1995; Guo et al., 2018; Manning-Berg et al., 2019; Luo and Zhu, 2025).
Despite substantial research on geologic chert fabrics (Maliva and Siever, 1989; 2005), experimental silicification (Westall, 1995; Lalonde et al., 2005; Moore et al., 2020; Osorio-Rodriquez et al., 2023), and the potential chemical environments of silicification (Manning-Berg and Kah, 2017; Moore et al., 2021), the primary mechanisms controlling silicification and the preservation of microbial communities remain poorly defined. What is certain, however, is that silicification likely occurred at or near the sediment-water interface, peneconteporaneously with mat growth (Manning-Berg and Kah, 2017; Moore et al., 2020; Osorio-Rodriquez et al., 2023). Exquisite preservation of Precambrian microbial mats, primary void space, and associated fabrics provide evidence that silicification was clearly rapid, as the taphonomic state of preserved microbial matter suggests a time frame of days to weeks (Bartley, 1996; Manning-Berg et al., 2019). The persistence of delicate microbial structures encapsulated within early diagenetic chert may therefore also place a critical constraint on the diagenetic history of chert.
Here, we provide a detailed petrographic analysis of microfossiliferous chert spanning both Precambrian space and time. Specifically, we aim (1) to determine whether a common silicification pathway occurs across microfossiliferous cherts, (2) to better understand the pathways of recrystallization that occurred from primary precipitation as hydrated silica phase to the current hydration-poor quartz phase, and (3) to explore the extent to which silica neomorphism affects microfossil preservation.
2 Geological setting of Proterozoic microfossiliferous chert
Here, we provide detailed petrography of early diagenetic, microfossiliferous from a range of Paleoproterozoic, Mesoproterozoic, and Neoproterozoic units (Figure 1; Table 2). Our investigation began with initial observations from the Mesoproterozoic Angmaat Formation (Dunham, 2018; Kah and Knoll, 1996; Manning-Berg and Kah, 2017). Dr. Andrew Knoll graciously provided materials from the Mesoproterozoic Billyakh Group and Neoproterozoic Bitter Springs and Draken Formations; and Dr. Keyron Hickman-Lewis provided material from the Paleoproterozoic Gunflint Formation. These units all exhibit remarkably well-preserved microbial remains, including intricate morphological details of filamentous and coccoidal microorganisms, thereby permitting exploration of how neomorphic processes associated with chert maturation affect microfossil preservation.
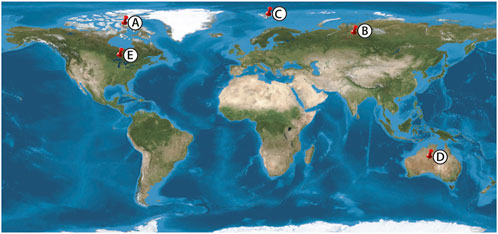
Figure 1. Proterozoic Sample localities. (A) Angmaat Formation, (B) Kotuikan Formation (C) Draken Formation (D) Bitter Springs Formation, (E) Gunflint Formation.
2.1 Angmaat Formation, Bylot Supergroup (arctic Canada)
The late Mesoproterozoic Angmaat Formation (formerly the upper Society Cliffs Formation; Turner, 2009) consists of ∼520 m of peritidal carbonate strata that is exposed in the Borden basins of northern Baffin Island, Nunavut. Re-Os dating of black shale from the underlying Arctic Bay and overlying Victor Bay formations constrain the depositional age of the Angmaat to between 1.048 ± 0.012 Ga and 1.046 ± 0.016 Ga (Gibson et al., 2018). This shallow, locally restricted carbonate platform consists of nested meter- and decameter-scale cycles that grade from subtidal irregularly laminated dolostone to well-laminated microbial dolostone. Microbial dolostone is regionally intermixed with ooids, seafloor precipitates capped by tepee cracks, and other desiccation features (Kah, 2000). Varicolored chert facies occurs throughout the Angmaat Formation (Hofmann and Jackson, 1991; Turner, 2009). Black chert that preserves a rich microfossil assemblage (Figure 2A) appears confined to intermittently restricted facies comprising stratiform microbial laminae, tufted microbial mats, and isopachously laminated seafloor precipitate facies (Figure 2B) (Kah and Knoll, 1996; Knoll et al., 2013; Manning-Berg and Kah, 2017).
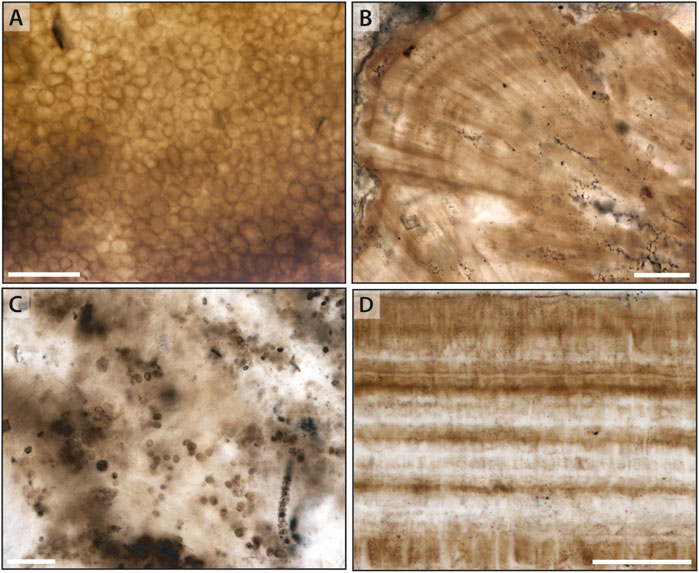
Figure 2. Common microfacies preserved in Mesoproterozoic chert. (A,C) Mat fabrics dominated by coccoidal microfossils in the Mesoproterozoic Angmaat and Kotuikan formations, respectively. (B,D) Aragonitic seafloor precipitates mimetically replaced by chert in the Angmaat and Kotuikan formations, respectively. Scale bars are 50 μm in each image.
2.2 Kotuikan Formation, Billyakh Group (Russia)
In Northern Siberia, the Anabar uplift exposes more than 1,000 m of marine sandstone, shale, and carbonate strata of the Mesoproterozoic Billyakh Group (Vorob’eva et al., 2015; Sergeev et al., 2017). The ∼500 m thick Kotuikan Formation is constrained in age to be older than 1,502 ± 6 Ma based on U-Pb dates on dolerite sills that intrude the lowermost part of the section (Ernst et al., 2016), which is consistent with Pb-Pb dates on dolomite of 1,513 ± 35 Ma for the Kotuikan and overlying Yusmastakh formations (Gorokhov et al., 2019). Within this formation, open marine shale of the lower Kotuikan Formation (Vorob’eva et al., 2015) gives way to dominantly restricted peritidal facies. Chert, and the best-preserved microfossils (Figure 2C), are predominantly confined to the Upper Kotuikan Formation in association with well-developed seafloor precipitates (Figure 2D) (Bartley et al., 2000; Sharma and Sergeev, 2004).
2.3 Draken Formation, Akademikerbreen Group (Spitsbergen)
In northeastern Spitsbergen, the Neoproterozoic Draken Formation represents an approximately 150–250 m-thick carbonate sequence deposited within an extensive intertidal to supratidal tidal flat and lagoon complex (Knoll and Swett, 1990; Fairchild et al., 1991). Although radiometric dates are absent from the Akademikerbreen Group, chemostratigraphic correlation to Re–Os dated carbon isotope anomalies of related Neoproterozoic strata help bracket the Draken Formation depositional age between approximately 737 and 800 Ma. Statistical chronological methods suggest deposition of the Draken Formation occurred over a 10-million year old period ending at 782 + 3.0/-3.2 Ma (Halverson et al., 2018). Both carbonate and shale facies host microfossils, with the best preservation occurring in early diagenetic chert (Fairchild et al., 1991; Knoll, 1982; Knoll and Swett, 1990; Knoll et al., 1991; Fadel et al., 2024). Chert commonly manifests as mm-to cm-scale clasts within intraformational breccias where both micritic and cherty clasts are embedded in a silty, microsparitic matrix. Chert clasts host a range of microbial populations consisting of complex assemblages that include both mat dwellers and allochthonous elements (Figure 3A) or uniform communities of tightly interwoven filaments (Figure 3B).
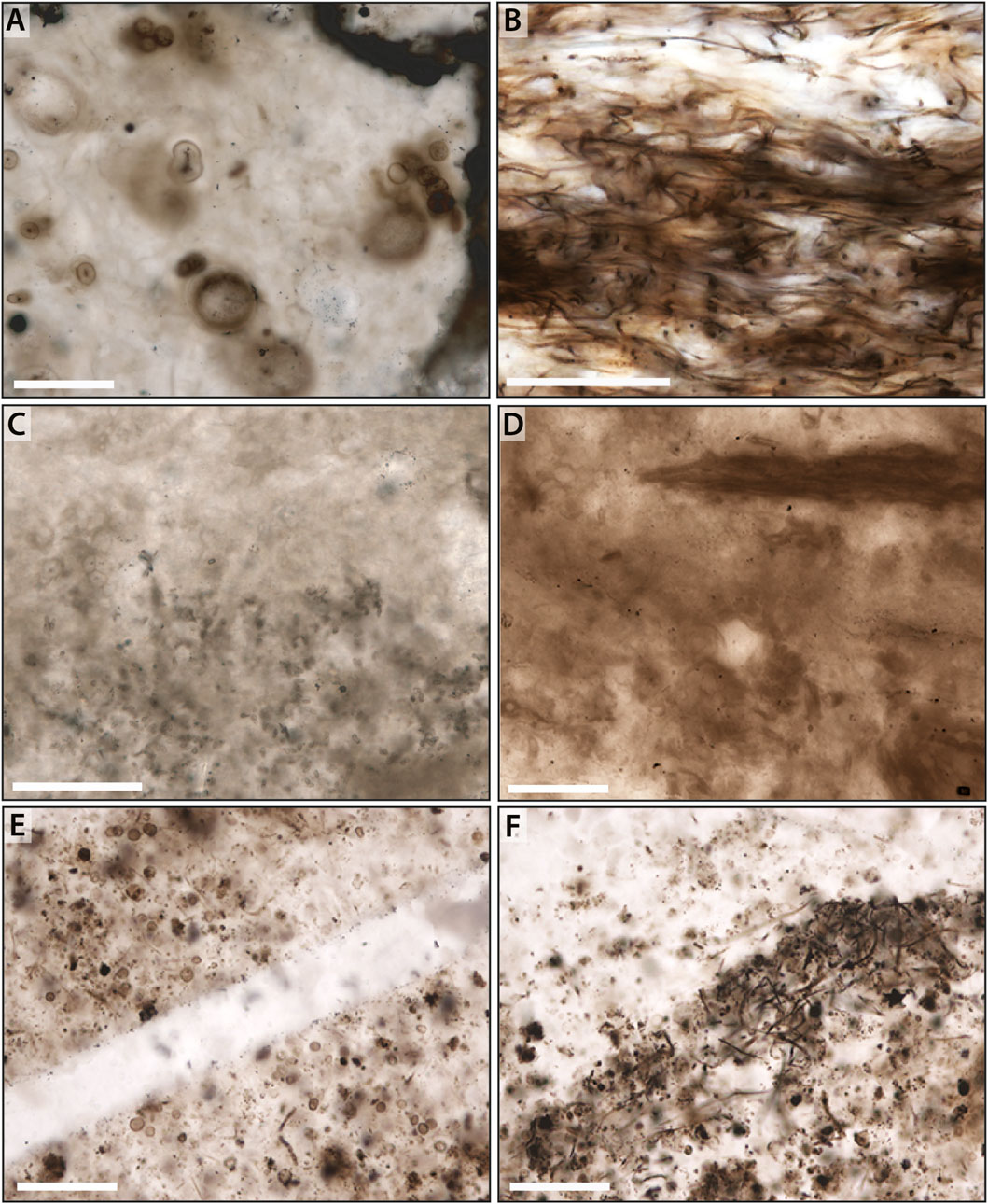
Figure 3. Common microbial facies preserved in Neoproterozoic and Paleoproterozoic chert. (A,B) Microbial constituents within a silicified clast of the Neoproterozoic Draken Formation. (C,D) More poorly preserved coccoidal and filamentous mat fabrics from the Neoproterozoic Bitter Springs Formation. (E,F) Variously degraded coccoids and filaments within the Paleoproterozoic Gunflint Formation. Scale bar is 50 μm in (A–C), and 100 μm in (D–F).
2.4 Bitter Springs Formation (Australia)
In central Australia, the Amadeus Basin preserves nearly 1,000 m of Neoproterozoic strata predominantly deposited in shallow water environments (Hill et al., 2000; Southgate, 1986). The Bitter Springs Formation is considered to be approximately 800 Ma based on Sm-Nd mineral isochrons (Zhao et al., 1994) and a U-Pb baddeleyite age of 824 ± 4 Ma (Glikson, 1996) from contemporary volcanic rocks. Within the Bitter Springs, stromatolite assemblages are similar to that in the adjacent Officer Basin, with a maximum age date of 802 ± 10 (Grey et al., 2005). The Bitter Springs Formation initiated with sandstone, siltstone and limestone deposition along with extensive interbedding of sulfate and halite evaporite facies, interpreted to have been deposited in a stagnant, hypersaline marine lagoon (Schmid et al., 2017) or supratidal salt flat (Hill et al., 2000). Overlying strata represent regional flooding and deposition of carbonate that preserves a diverse range of stromatolitic morphologies deposited in hypersaline lacustrine to marine peritidal environments (Schmid et al., 2017; Southgate, 1986). Black chert nodules commonly occur within stromatolitic facies, with microfossils restricted to finely laminated chert nodules (Figures 3C,D) (Southgate, 1986; 1989).
2.5 Gunflint Iron Formation, Animikie Group (USA-Canada)
The Paleoproterozoic Gunflint Formation was deposited in the Animikie Basin, which developed as a subsiding back-arc basin along the southern margin of the Superior Province (Schulz and Cannon, 2007). The depositional sequence reflects a transition from shallow marine conditions in the lower member, through a transgressive deepening phase, returning to shallower conditions in the upper member (Petrash et al., 2016). The age of the Gunflint Formation is constrained to 1,878 Ma
3 Materials and methods
Traditional light microscopy was conducted at the University of Tennessee on an Olympus BX60 compound optical microscope with a Q-imaging Micropublisher 5.0 color camera. Petrographic analyses were done on standard polished thin sections (30 μm thickness). To more easily identify microfossils and primary fabrics, observations were made under plane polarized light with the microscope condenser, which lessens the intensity of light refraction along grain boundaries. The condenser was then removed, and observations were made under both plane and cross polarized light to more easily identify individual grain boundaries within the chert fabrics. The gypsum (λ) plate was used to identify variations in crystal lattice orientation (cf. Miehe et al., 1984; Schmidt et al., 2013) and to readily highlight crystal orientations.
4 Petrographic results and interpretation
4.1 Primary chert fabric
Chert samples examined in this study consist of a mosaic of variably-sized quartz crystals (typically 20–100 μm) that mimetically preserve primary depositional constituents. Primary constituents vary between samples, and include a complex array of silicified microbial elements (i.e., irregularly distributed microbal elements such as coccoids and filament sheaths, as well as extrapolymeric substances; EPS) that occur in a range of taphonomic states (cf. Figures 2, 3); silicified carbonate precipitates that occurs as isopachous layers and as individual crystal fans (Figure 2, Figure 4A); and silicified carbonate microspar that occurs both as draping elements within layered microbial mats and as microbreccia horizons containing submillimeter clasts of silicified microspar and microbial fragments (Figure 4C). Some samples also contain mm-scale irregular nodules that displace primary lamination within the mat—potentially representing a pocket of EPS or synsedimentary precipitation of a precursor mineral phase—and mm-scale primary void spaces within the mat (Figure 4E).
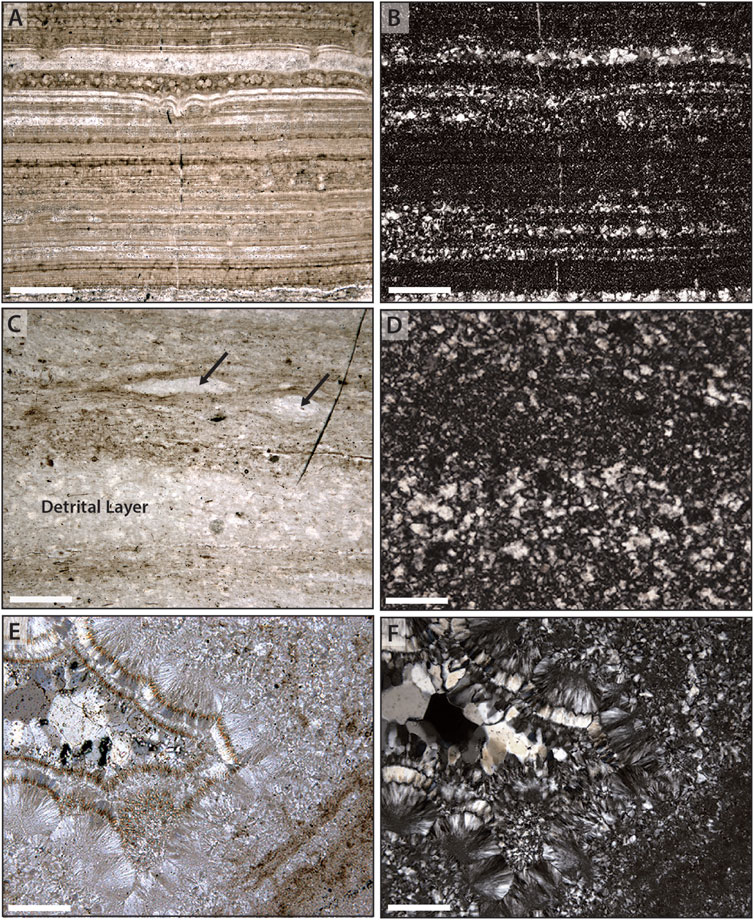
Figure 4. Relationships between host rock and chert fabrics. (A) Finely laminated seafloor precipitates from the Mesoproterozoic Kotuikan Formation; brighter areas reflect a combination of larger primary crystal size and an absence of organic staining and are reflected in larger crystal sizes in replacement chert (B) when observed under crossed-polars. (C) Compacted filamentous mat from the Neoproterozoic Bitter Springs Formation, interlaminated with detrital carbonate; brighter areas (arrows) reflect an absence of organic staining and are reflected in larger crystal sizes in replacement chert (D) when observed under crossed polars. (E, F) Primary void within a host microbial substrate that records multiple stages of fibrous to bladed to blocky chert. Scale bar is 200 μm in all images.
Under crossed polars, quartz crystals show distinct differences of size and orientation. Within most of the host materials, the size of quartz crystals appears to covary with the density of organic staining, wherein finer crystals occur in regions of more abundant organic staining and coarser crystals occur within regions that lack organic staining (Figures 4B,D,F). When mimetically replacing a primary carbonate fabric, the observed difference in quartz crystal size may also represent a difference in crystal size of the primary carbonate. Unlike precursor host materials, primary voids within the mat typically preserve a succession of chert fabrics from wall-lining chalcedony (± bladed quartz) to equant megaquartz crystals in the void interior (Figures 4E,F).
A more detailed examination of the chert shows a strong degree of alignment of the optic axes of quartz crystals within most of the primary host phases. Although late-stage quartz crystals within primary void spaces lack a clear relationship between adjacent crystals (cf. Figure 4F), the vast majority of chert displays a prominent rectilinear or gridwork pattern (Figure 5). This rectilinear pattern is characterized by wedge-shaped regions that display extinction that alternates between mutually perpendicular directions. Use of the gypsum (550 nm) plate enhances regions of alternating extinction and aids in the recognition of this rectilinear pattern. Such rectilinear texture occurs independently of the cut of the thin section through any given sample, suggesting that the texture is largely homogeneous in three dimensions. Variation in the rectilinear pattern correlates to differences in overall crystal size, suggesting patches of distinct crystal size distributed through the samples, A similar rectilinear pattern is observed both when the chert is the primary mineral phase (i.e., replacing primary microbial elements and EPS; Figure 5), or when it is replacing a primary carbonate phase (Figure 6).
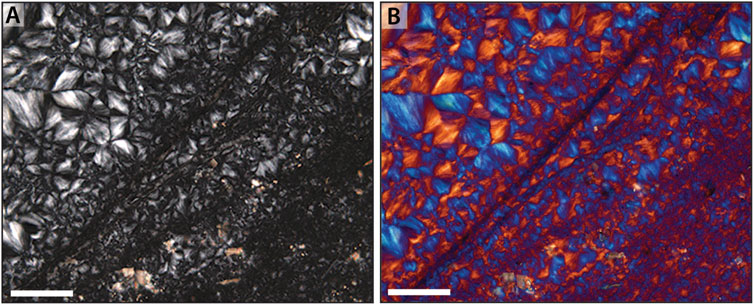
Figure 5. Rectilinear fabric in microfossiliferous early diagenetic chert. (A) Under crossed-polars, a rectilinear fabric—here from in the Mesoproterozoic Angmaat Formation—is observed as the dominant chert fabric within all samples investigated in this study. This fabric arises from the juxtaposition of wedge-shaped regions of similarly aligned fibrous crystals and occurs at a variety of different scales. (B) The rectilinear nature of this chert is often highlighted by insertion of the gypsum (λ) plate, which permits ready identification of the fabric at a variety of grain scales. Images are of a filamentous, tufted mat wherein smaller crystals represent distinct filamentous laminae (cf. Figure 9B in Knoll et al., 2013). Scale bar is 100 μm in both images.
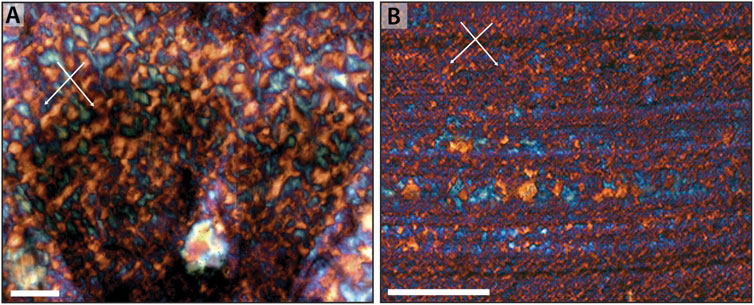
Figure 6. Rectilinear fabric in carbonate-replacing early diagenetic chert. (A) Rectilinear fabric of chert that replaces seafloor aragonitic seafloor precipitate of the Angmaat Formation (cf. Figure 1B), highlighted by insertion of the gypsum plate. (B) Rectilinear fabric of chert that replaces synsedimentary seafloor precipitate of the Kotuikan Formation (cf. Figure 1D), highlighted by insertion of the gypsum plate. Arrows show directionality of rectilinear fabric. Scale bar is 100 μm in both images.
Within this rectilinear fabric, a variety of distinct growth terminations are observed. In rare cases where radiating fibrous growth terminates without interference, it is apparent that growth proceeded as the crystallization of individual spheroids, with rounded edges readily identifiable (Figures 7A,B). More commonly, radiating growth continues until all space between adjacent nucleation points is filled, resulting in competitive growth between adjacent nucleation centers, forming a more discrete rectilinear fabric (Figure 7C). Secondary silica growth is also observed and can result in either increase in size of original radiating spherules, or filling of interstices between primary spherules (Figure 7B). Secondary growth is typically syntaxial and in the same lattice structure as precursor growth, and only rarely shows alternation in lattice orientation. Changes in orientation of lattice structure, however, is most common in fibrous chert that lines primary void spaces. More typically, however, identification of discrete boundaries between radiating components is difficult, and the rectilinear fabric appears primarily as intersecting wedges of opposing orientation (Figure 7D).
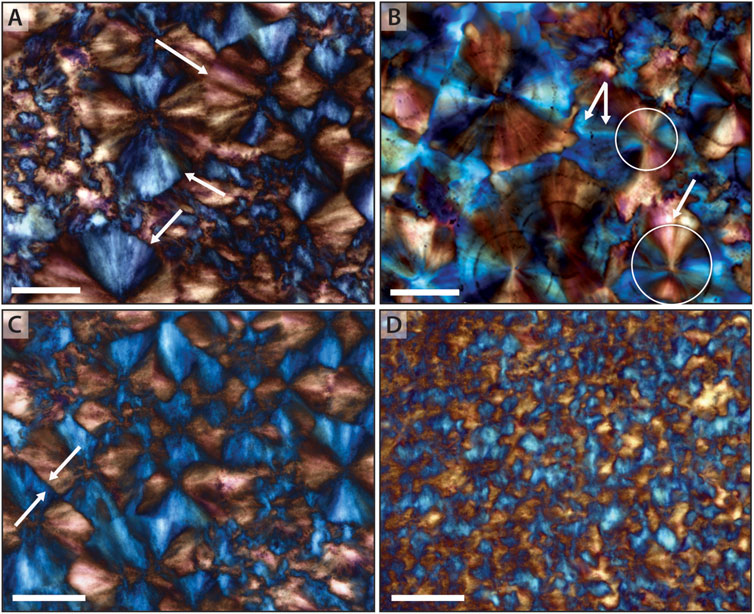
Figure 7. Patterns in radiating crystallites. (A) Spheroidal rounded edges, (arrows) showing termination of large spherules. (B) A rare example of radiating crystallites that show clear spheroidal growth (arrows); additional growth occurs as expansion of spherules and, ultimately, as syntaxial growth (arrows) filling interstices between individual elements. (C) Radiating crystallites showing rectilinear compromise boundaries (arrows) with growth from adjacent nucleation sites. (C) Rarely, growth of crystallites shows a change in lattice orientation, here identified by a switch from orange-red to blue color (arrow) when observed under the gypsum plate. (D) Most commonly, termination of radiating bundles is difficult to discern, resulting in the standard rectilinear fabric composed of discrete wedges in opposing orientation. Scale bar is 50 μm in all images.
4.1.1 Interpretation of primary chert fabric
Sweeping extinction observed within individual spherules and wedge-shaped quadrants demonstrates that the initial fabric is composed of aggregates of fibers, or crystallites, that each radiate outward from a central point. Radiating fibers show that crystallization proceeded primarily as growth of three-dimensional spherules, emanating from individual nucleation points. Furthermore, when the gypsum (
The widespread observation of a rectilinear fabric is consistent an apparent alignment of crystalline fibers across adjacent spherules. As noted above, heterogeneity of spherule size appears to correlate with density of preserved organic matter, suggesting that the presence of organic matter may have affected initial nucleation of chalcedony spherules. Whereas the continuity of spherules between regions of different crystal sizes suggests that silicification of the substrate occurred simultaneously, the similarity of spherule size within distinct regions of the host substrate suggests that compositional differences in the host (e.g., the presence and density of diffuse organic staining) may have played a critical role in the nucleation of chalcedony spherules, for instance by providing metallic ions from degrading organic material (cf. Dupraz et al., 2009; Moore et al., 2021). Unlike experimental work (cf., Moore et al., 2020), however, spherules do not appear to nucleate directly on organisms or their sheaths, instead permeating through individual fossils.
4.2 Variation in spherulitic fabric
Despite observation of a pervasive rectilinear fabric in early diagenetic chert, here interpreted as originating from a three-dimensional network of length-fast chalcedony spherules, we recognize that there is substantial variation in this fabric, even within single thin sections. Fine-scale variation in degree of extinction (or color, when viewed with the gypsum plate inserted) is interpreted to reflect slight variation in the orientation of the individual chalcedony fibers that comprise the spherule (Figure 8A). Such fine-scale variation, however, is not the most common expression of this fabric. More commonly, distinct grain boundaries—as identified by increased light refraction and, sometimes, an increased concentration of microimpurities—divide spherules into a cross section containing four wedge-shaped crystals with indistinct intercrystalline domains (Figure 8B). In the most common case (cf. Figure 7D), spherule perimeters are poorly preserved and such fabrics are observed as individual wedges or linear crystal regions oriented in two mutually perpendicular orientations.
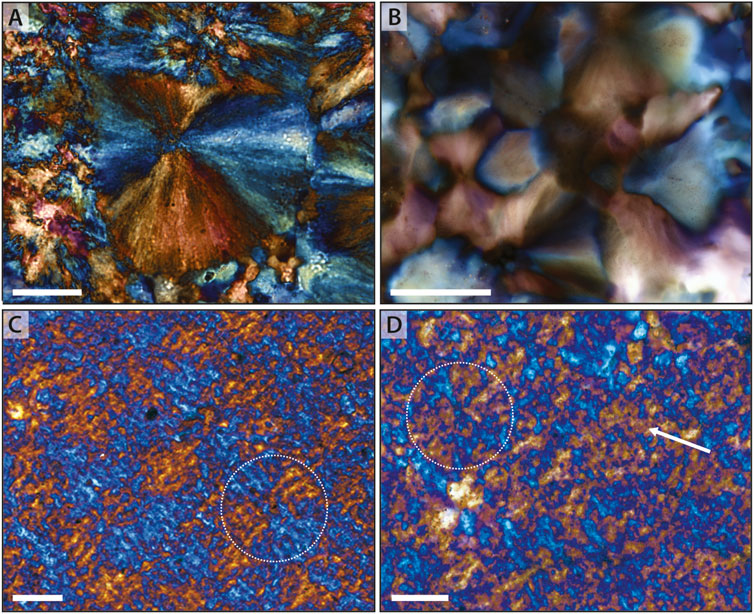
Figure 8. Neomorphism of chalcedony spherules. (A) Angmaat Formation spherule that retains fine-scale preservation of individual crystallites, suggesting little neomorphic recrystallization. (B) Angmaat Formation spherules in that show grain boundaries between quadrants of distinct crystallographic orientation, suggesting neomorphic coarsening of crystallites. (C) Cross-hatched fabric within Bitter Spring Formation chert that mimics form of primary spherules (circle). (D) Bitter Springs Formation chert in which spherules (circle) and broader-scale regions (arrow) are largely represented by a single crystallographic domain that contains micro-domains of different orientation (cf. poikilitic texture). Scale bar is 50 μm in each image.
Several other scenarios occur in which a rectilinear fabric is apparent even without clear identification of individual, discrete spherules. This fabric is expressed by a cross-hatch pattern that reflect discrete regions—consistent with spherule quadrants—that are composed of sub-parallel, similarly oriented blades separated by microscale regions of the opposite orientation (Figure 8C). In a final scenario, rectilinear fabric can consist of diffuse regions that are composed primarily of chert with a single crystallographic orientation (i.e., a region that is primarily orange, or primarily blue, under the gypsum plate) but which contains microdomains of the opposite orientation (Figure 8D), suggesting a poikilitic fabric. Interestingly, each of the latter scenarios have only been observed when the primary host materials consist primarily of micritic carbonate.
A final endmember is commonly observed in the Draken Formation, which lacks the abundance of rectilinear fabric observed in chert from other localities. Here, the most common fabric is a more traditional neomorphic chert fabric consisting of amoeboid-shaped interlocking crystals (Figures 9A,C). Several observations suggest that this more traditional fabric represents an end-member of rectilinear fabric. First, many of the larger amoeboid-shaped crystals show intercrystalline domains of sweeping extinction when viewed under crossed polars or with insertion of the gypsum plate. Additionally, larger amoeboid-shaped crystals commonly contain micro-domains of the opposite orientation, similar to that observed in poikilitic rectilinear fabric (cf. Figure 8D). Finally, discrete regions within this more traditional neomorphic fabric often records distinct, wedge-shaped regions (Figures 9B,D), which are a hallmark of rectilinear fabric.
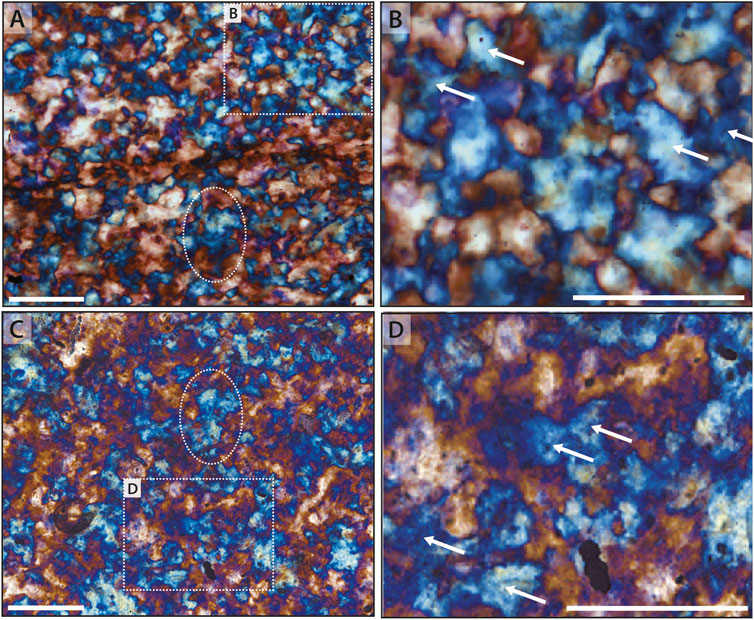
Figure 9. End-member neomorphism within microfossiliferous chert. (A,C) Draken Formation microfossiliferous chert showing amoeboid-shaped interlocking crystals. Larger crystals, such as highlighted by the ovals, commonly host microdomains of differing orientation. Faint evidence of the primary filamentous microbial fabric is visible in both (A,C). (B,D) Magnified images of regions noted by dotted rectangles within (A,C). Individual neomorphic crystals appear to be constructed of wedge-shaped regions (arrows) that mimic spherulitic quadrants. Scale bar is 100 μm in all images.
4.2.1 Interpretation of fabric variation
A rectilinear fabric comprised of precipitation of a three-dimensional network of chalcedony spherules comprises the primary fabric of microfossiliferous chert investigated for this study. This fabric, however, contains substantial variation. Observed petrographic variation is consistent, for the most part, with a primary origin as chalcedony spherules followed by neomorphic modification. Best-preserved spherulitic elements show minimal evidence for neomorphic modification preserve fine scale sweeping extinction and show clear grain boundaries (i.e., discontinuities of lattice structure) only at the interface between adjacent spherules (cf. Figure 7A, Figure 10A). By contrast, polycrystalline spherules—the most commonly observed fabric—record clear grain boundaries that divide spherules into distinct wedge-shaped quadrants with distinct lattice orientations. Although small variation in lattice orientation is recorded in these wedge-shaped quadrants by slight variation in extinction (or color, when viewed with the gypsum plate), the vast majority show orthogonal orientations, suggesting only slight modification of crystal lattice structure during neomorphism (cf. Figure 7D, Figure 10B).
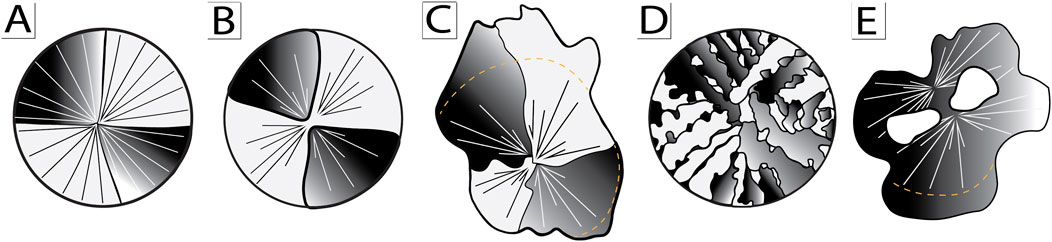
Figure 10. Cartoon illustration model of spherule neomorphism. (A) Primary radial-fibrous chalcedony spherule. (B) Chalcedony spherule neomorphosed into distinct wedge-shaped quadrants. (C) Chalcedony spherule and adjacent syntaxial overgrowth neomorphosed into irregular wedge-shaped quadrants. (D) Cross-hatched chalcedony spherule. (E) Amoeboid-shaped neomorphic crystal consisting of multiple coalesced spherules.
More extreme neomorphism is represented by traditional microsparitic chert fabrics that consist of amoeboid-shaped interlocking crystals (cf. Figures 9A–D, Figures 10D,E). Such fabrics, however, still contain domains of sweeping extinction or discrete wedge-shaped elements that suggest a primary spherulitic origin. Highly irregular crystal boundaries and incorporation of isolated patches of orthogonal orientation (“dissection microstructure” of Urai et al., 1986) suggest grain coarsening via migration of grain boundaries that result in coalescence of regions of similar lattice orientation.
The most unusual modifications (cf. Figure 8C,D, Figure 10C) occur with silicification of a microcrystalline carbonate precursor phase. Here, broad-scale fabric shows division into distinct quadrants that mimic spherulitic fabric. Quadrants, however, are defined by a dominant orientation of linear parallel elements interspersed with (recessive, less dominant) linear elements of orthogonal lattice orientations. Such fabrics may represent an interaction between silica nucleation templated by precursor phase and spheroidal growth intergrain regions.
5 Discussion
5.1 Model for spherulitic growth and neomorphism
Detailed petrographic analysis defines precipitation of chalcedony spherules as the primary fabric of microfossiliferous chert from across Proterozoic time and space. Similar spherulitic chalcedony has been recognized, as well, from other examples of Proterozoic (Simonson, 1987; Maliva et al., 2005) and Archean-aged (Corpolongo, 2024; Sugitani et al., 1998) chert. The resulting rectilinear fabric is similar (although of a much larger scale) to that observed in a variety of lacustrine environments which has been interpreted as the pseudomorphic replacement of magadiite by chert (Schubel and Simonson, 1990; Hattori et al., 1996; cf. Moraishi, 1989).
Our study follows earlier arguments (Moore et al., 2021; Manning-Berg and Kah, 2017), that microfossil preservation by silicification is an early diagenetic phenomenon that occurred at or near the sedimentary substrate, penecontemporaneous with mat growth and decay. Fundamental to the model presented here are the observations that chalcedony spherules 1) comprise the vast majority of the chert matrix, 2) mimetically preserve precursor microbial and associated carbonate fabrics, and 3) crosscut microbial elements (primarily filamentous sheaths) without disruption. These observations demand that the precursor constituents to spherule precipitation (e.g., sufficient silica) were in abundance at or near the sedimentary substrate. Nucleation points of spherules are also distributed through the substrate, and not specifically on microbial substrates (cf. Moore et al., 2021) or within microbial sheaths (cf. Foucher and Westall, 2013; Guo et al., 2018). This observation, combined with the observation that the density of nucleation points co-varies with the density of organic staining (cf. Figure 5), suggests a precursor phase that permeated the microbial substrate. Prior measurement of the crystal size distribution of spherules within regions of visually distinct size, found similar lognormal distributions (Dunham, 2018). Such distributions suggest a decay-rate nucleation within a closed, nutrient-limited system (Kile et al., 2000; Kile and Eberl, 2003). Conbined, these lined of evidence suggest that the precursor phase was a silica gel that permeated the microbial substrate. Such silica gels have been previously linked to microfossil preservation (Oehler, 1976a; Benning et al., 2004; Kremer et al., 2012).
Silica gels consist of discrete colloidal particles linked together into a three-dimensional network of branched chains (Iler, 1979). Silica-rich fluids consist of largely monomers and dimers of silicic acid. Colloidal silica, on the other hand, consists of discrete particles, <1,000 nm in size, that remain in suspension within the fluid. These particles consist of polymerized cores, wherein oxygen bridging of silicic acid polymerizes to form siloxane (
In nature, polymerization of a silica gel may be enhanced by metallic bonding (Dove and Craven, 2005) associated with EPS degredation (Moore et al., 2021) or geochemical reactions involving Na (Dove et al., 2019) or a variety of silica minerals (Behr, 2002). Because polymerization equilibria, however, is strongly affected by solution concentration, pH, silica to metal cation ratio (e.g., K, Na, Ca, Mg, Al), resulting in heterogeneity even at small length scales (Matinfar and Nychka, 2023). For instance, most early diagenetic chert contains micrometer-scale or larger voids that are identified by the occurrence of void-lining chalcedony followed by megaquartz (cf. Figure 4E; cf. Simonson, 1987; Schubel and Simonson, 1990; Sugitani et al., 1998; Maliva et al., 2005; Gabriel et al., 2021; Jing et al., 2022). The presence of voids, whether formed by shrinkage during conversion from magadiite to chert (Behr, 2002; Schubel and Simonson, 1990) or via gas release during microbial metabolism (Bosak et al., 2010; Knoll et al., 2013; Manning-Berg and Kah, 2017), may reflect heterogeneity of the underlying gel phase. Different distributions of spherule nucleation in regions of weaker and stronger organic staining (cf. Figure 5) may also reflect heterogeneity in gel behavior.
A key element in the behavior of silica gels is synaeresis; once a branched network is formed, polymerization of surface silanol continues, resulting in the condensation of the network, reduction of gel volume, and expulsion of water (Matinfar and Nychka, 2023). The presence of metallic cations increases both the rate and extent of synaeresis due to metallic bridging (Hamouda and Amiri, 2014), with divalent cations abundant within microbial EPS (Dupraz et al., 2009; Moore et al., 2021). Extent of synaeresis is also dependent on temperature, with ∼25% reduction of total volume at 20°C occurring in <100 h (Wilhelm and Kind, 2015), which is consistent with timescales of microbial degradation (Bartley, 1996; Manning-Berg et al., 2022). We suggest that crystallization of chalcedony spherules proceeded from this condensed gel phase, and condensation may be reflected in differential compaction of thin- and thick-walled filamentous sheaths (Manning-Berg et al., 2019).
Precipitation from a precursor gel phase likely reflects pH changes during degradation of microbial matter (Eugster and Jones, 1968; Leet et al., 2021). In siliceous deposits, the diversity of crystal form is attributed to the pH conditions present during mineral formation (Folk and Pittman, 1971; Iler, 1979; Heaney, 1993). In alkaline conditions (pH > 7), silica forms discrete silica tetrahedra [SiO4] that attach directly to the growing surface, producing fibers with c-axes aligned parallel to the direction of elongation (i.e., length-slow; Folk and Pittman, 1971; Hesse, 1989). Conversely, in acidic environments (pH < 7), silica occurs primarily as silanol groups. Silanol groups attach tangentially to the growth surface, resulting in c-axes oriented parallel to the surface but perpendicular to the direction of fiber growth (i.e., length-fast; Folk and Pittman, 1971; Iler, 1979). Acidic conditions that are inferred from the dominantly length-fast growth observed here can arise from influx of fresh waters (Hesse, 1989; Knauth et al., 1994; Manning-Berg and Kah, 2017) or the presence of organic acids within microbial mats (Konhauser et al., 2004; Lalonde et al., 2005).
At present, the driver of spherule nucleation remains uncertain, but may have been regulated by the distribution of divalent cations in the gel. The presence of cation impurities may promote nucleation and growth by influencing charge balance and growth kinetics and by modifying surface energies during nucleation (Wang and Merino, 1990; Merino et al., 1994). Fiber growth then appears to have proceeded by syntaxial addition. Fibers commonly penetrate microfossil sheaths without apparent disruption suggesting that sheath-forming EPS was permeated by the silica gel network and syntaxial crystal growth simply incorporated EPS as inclusions. In cases where there was a precursor carbonate phase, such as seafloor precipitates of Angmaat and Kotuikan formations (e.g., Figure 6), silica gel likely permeated pore space around acicular carbonate. Mimetic preservation of primary precipitates suggest that dissolution of the primary phase proceeded via force of crystallization of chalcedony (Maliva et al., 2005). Unusual behavior of chalcedony spherulites during silicification of precursor micrite (cf. Figures 8C,D) suggests a potentially complex interaction between primary and secondary mineral phases.
Silica gel may have also temporarily stabilized the EPS (cf. Moore et al., 2020). In rare cases in the Draken formation (i.e., Figures 9A,C), distinct orientations of chalcedony are observed inside and outside of thick-walled filmentous sheaths, suggesting that sheaths can occasionally serve as barriers to syntaxial growth. This observation is consistent with Raman analysis of rare Proterozoic microfossils that record signals of opaline silica within some sheath and cell wall material (Foucher and Westall, 2013; Guo et al., 2018).
5.2 Implications for microfossil preservation
The model for silicification presented here is distinct from models derived from either siliceous sinters or experimental silicification. Siliceous sinters form when hydrothermal waters enriched in silica cool and rapidly become oversaturated with respect to silica (Lynne et al., 2007). Under these conditions, silica rapidly precipitates as nanospheres of opal-A that coat all pre-existing surfaces, including microbial sheaths and their associated extracellular polymeric substances (Cady and Farmer, 2007; Konhauser et al., 2001). These nanospheres initially form a porous network that progressively densifies through continued precipitation, dehydration, and diagenetic maturation, eventually transforming to microcrystalline quartz (Lynne et al., 2007; Jones and Renault, 2007; Lynne, 2015; Campbell et al., 2015). Despite preserving general morphological features, siliceous sinters typically do not exhibit the mimetic preservation of microorganisms observed in the Precambrian microfossiliferous cherts described here. By contrast, experiments on microbial silicification commonly explore organic-mineral interactions with cell walls and associated EPS (Westall, 1995; Konhauser et al., 2004; Benning et al., 2004; Lalonde et al., 2005), or the metabolic role of these microbes (Moore et al., 2020; Osario-Rodriquez, 2023). The latter of these experiments have suggested multiple pathways may favor silica precipitation even in undersaturated solutions. Osario-Rodriguez et al. (2023), however, shows silica spherules interstitial to microbes. By contrast, experiments of Moore et al. (2020) show accumulation of colloidal silica directly on microbe surfaces, wherein cation bridging aids in adhering silica to the microbe surface.
Whereas we agree cation bridging is a critical element in microbial silicification, current models for silicification also require extensive recrystallization to transition from colloidal silica
If crystallization proceeded directly from a condensed gel, as is proposed here, we also suggest that the initial mineralogical phase may have been a metastable silica phase with greater crystallinity that would have released less water during subsequent phase transformations. Although opal-A may have been present in limited amounts (Fadel et al., 2024; Foucher and Westall, 2013; Guo et al., 2018), we suggest that the primary phase was a more ordered, less hydrated phase, such as a mixture of opal-CT and quartz. Exquisite preservation of microbial elements (cf. Knoll et al., 2013), regardless of their taphonomic state (Manning-Berg et al., 2019) suggests minimal water-rock interaction. Similarly, although we find widespread evidence for neomorphism, most changes appear to reflect modification of grain boundaries. Initial modification may result from syntaxial mineral growth from amorphous silica residing between either adjacent fibers (e.g., Figure 10B) or between adjacent spherules (e.g., Figure 10B). Even recrystallization into amoeboid-like crystals (e.g., Figure 10E) retains slightly misaligned intercrystalline domains, suggesting coalescence of grains and grain boundary migration (Li, 1962; Stipp et al., 2002). Such grain boundary modification requires little water-rock interaction.
Direct recrystallization of a more ordered phase is also consistent with experimental crystallization of silica gels which resulted in the formation of chalcedony spherules but lacked evidence for intermediate phases (Oehler, 1976b). Previous experiments that ran at lower temperature and pressures resulted in a mixture of opal-CT and chalcedony spherules (Oehler, 1976b). We suggest that synaeresis of silica gel is a critical step in the crystallization process as it drives off water while forming a more condensed silica gel network. Under natural conditions, the presence of abundant cations from degrading microbial EPS (Dupraz et al., 2009; Moore et al., 2020) could act as a driver for the synaeresis process (and elevated temperature in experiments of Oehler, 1976b) resulting in minimal hydration of the primary mineral phase to support crystallization. Opal-CT has, in fact, been observed via TEM (cf. Moreau and Sharp, 2004) and Raman (Foucher and Westall, 2013) in Proterozoic chert, but more investigation is required to identify this as a primary, rather than recrystallized silica phase. If primary, initial precipitation of a more crystalline phase will ultimately reduce the potential for water loss during mineralogical stabilization, resulting in greater fidelity of microfossil preservation.
6 Conclusion
Our investigation of Proterozoic microfossil-bearing chert shows that early diagenetic chert consist of length-fast, chalcedony spherules. We identify a range of fabrics that are inferred to represent neomorphic recrystallization of the primary spherules. Observed fabrics suggest neomorphism via syntaxial cementation within fiber bundles, or between constituents that are similar in optical orientation. Notably, all samples examined for this study show exceptional ability to preserve intricate details of microfossils and other primary fabrics. We use these petrographic constraints to refine models for microfossil silicification. We suggest that silicification proceeded by formation and condensation of a silica gel followed by direct precipitation of a more ordered, less hydrated phase, such as a mixture of opal-CT and quartz. Additional lattice-scale investigation is required to confirm direct precipitation of an ordered crystalline phase. Precipitation of an initial crystallization phase limits water loss during neomorphic recrystallization, ultimately resulting in greater fidelity of microfossil preservation.
Data availability statement
The original contributions presented in the study are included in the article/supplementary material, further inquiries can be directed to the corresponding author.
Author contributions
KG: Writing – review and editing, Conceptualization, Investigation, Formal Analysis, Writing – original draft. LK: Project administration, Funding acquisition, Resources, Conceptualization, Writing – review and editing, Supervision.
Funding
The author(s) declare that financial support was received for the research and/or publication of this article. Funding for this work was supplied by Kenneth R. Walker and Robert Rex funds, Department of Earth, Environmental & Planetary Sciences, the University of Tennessee.
Acknowledgments
This contribution could not have happened without the generous donation of sample material by A.H. Knoll and K. Hickman-Lewis. We also thank former lab members, A. Manning-Berg and J. Dunham for foundational work. The authors would like to thank two reviewers whose comments helped to improve this manuscript.
Conflict of interest
The authors declare that the research was conducted in the absence of any commercial or financial relationships that could be construed as a potential conflict of interest.
Generative AI statement
The author(s) declare that no Generative AI was used in the creation of this manuscript.
Publisher’s note
All claims expressed in this article are solely those of the authors and do not necessarily represent those of their affiliated organizations, or those of the publisher, the editors and the reviewers. Any product that may be evaluated in this article, or claim that may be made by its manufacturer, is not guaranteed or endorsed by the publisher.
References
Alleon, J., Bernard, S., Le Guillou, C., Marin-Carbonne, J., Pont, S., Beyssac, O., et al. (2016). Molecular preservation of 1.88 Ga Gunflint organic microfossils as a function of temperature and mineralogy. Nat. Comm. 7, 11977. doi:10.1038/ncomms11977
Barghoorn, E. S., and Tyler, S. A. (1965). Microorganisms fromthe Gunflint chert. Science 147, 563–575. doi:10.1126/science.147.3658.563
Bartley, J. K. (1996). Actualistic taphonomy of cyanobacteria: implications for the Precambrian fossil record. Palaois 11, 571–586. doi:10.2307/3515192
Bartley, J. K., Knoll, A. H., Grotzinger, J. P., and Sergeev, V. N. (2000). Lithification and fabric genesis in precipitated stromatolites and associated peritidal carbonates, Mesoproterozoic Billyakh Group, Siberia. SEPM Spec. Pub. 67, 60–73.
Behr, H.-J. (2002). Magadiite and Magadi chert: a critical analysis of the silica sediments in the Lake Magadi Basin, Kenya. SEPM Spec. Pub. 73, 257–273. doi:10.2110/pec.02.73.0257
Benning, L. G., Phoenix, V. R., Yee, N., and Tobin, M. J. (2004). Molecular characterization of cyanobacterial silicification using synchrotron infrared micro-spectroscopy. Geochim. Cosmochim. Acta 68, 729–741. doi:10.1016/S0016-7037(03)00489-7
Bosak, T., Bush, J. W. M., Flynn, M. R., Liang, B., Ono, S., Petroff, A. P., et al. (2010). Formation and stability of oxygen-rich bubbles that shape photosynthetic mats. Geobiology 8, 45–55. doi:10.1111/j.1472-4669.2009.00227.x
Brasier, M. D., Green, O. R., Lindsay, J. F., McLoughlin, N., Steele, A., and Stoakes, C. (2005). Critical testing of Earth’s oldest putative fossil assemblage from the ∼3.5 Ga apex chert, chinaman creek, western Australia. Precam. Res. 140, 55–102. doi:10.1016/j.precamres.2005.06.008
Brasier, M. D., Green, O. R., and McLoughlin, N. (2004). Characterization and critical testing of potential microfossils from the early Earth: the Apex ‘microfossil debate’ and its lessons for Mars Sample Return. Int. J. Astrobiol. 3, 139–150. doi:10.1017/S1473550404002058
Buick, R. (1990). Microfossil recognition in Archean rocks: an appraisal of spheroids and filaments from a 3500 M.Y. old chert-barite unit at North Pole, Western Australia. Palaios 5, 441–459. doi:10.2307/3514837
Cady, S. L., and Farmer, J. D. (2007). “Fossilization processes in siliceous thermal springs: trends in preservation along thermal gradients,”. Novartis foundation symposia. Editors G. R. Bock, and J. A. Goode (John Wiley and Sons, Ltd), 202, 150–173. doi:10.1002/97804-70514-986.ch9
Cady, S. L., Wenk, H. R., and Sintubin, M. (1998). Microfibrous quartz varieties: characterization by quantitative x-ray texture analysis and transmission electron microscopy. Contrib. Mineral. Petrol. 130, 320–335. doi:10.1007/s004100050368
Campbell, K. A., Lynne, B. Y., Handley, K. M., Jordan, S., Farmer, J. D., Guido, D. M., et al. (2015). Tracing biosignature preservation of geothermally silicified microbial textures into the geological record. Astrobiology 15, 858–882. doi:10.1089/ast.2015.1307
Corpolongo, A. (2024). Neoarchean microfossils and microbialites inform the search for extraterrestrial life in the solar system. (PhD Thesis). University of Cincinnati, Cincinnati, OH. Available online at: http://rave.ohiolink.edu/etdc/view?acc_num=ucin1721145153623216
De Gregorio, B. T., Sharp, T. G., Flynn, G. J., Wirick, S., and Hervig, R. L. (2009). Biogenic origin for Earth’s oldest putative microfossils. Geology 37, 631–634. doi:10.1130/G25683A.1
Dodd, M. S., Papineau, D., Grenne, T., Slack, J. F., Rittner, M., Pirajno, F., et al. (2017). Evidence for early life in Earth’s oldest hydrothermal vent precipitates. Nature 543, 60–64. doi:10.1038/nature21377
Dove, P. M., and Craven, C. M. (2005). Surface charge density on silica in alkali and alkaline earth chloride electrolyte solutions. Geochim. Cosmochim. Acta 69, 4963–4970. doi:10.1016/j.gca.2005.05.006
Dove, P. M., Han, N., and Wallace, A. F. (2019). Systematic sependence of kinetic and thermodynamic barriers to homogeneous silica nucleation on NaCl and amino acids. J. Mat. Res. 34, 442–555. doi:10.1557/jmr.2018.474
Dunham, J. I. (2018). Understanding early diagenetic silicification: petrographic Ffabrics within Proterozoic microfossiliferous chert. (master's thesis). University of Tennessee, Knoxville, TN. Available online at: https://trace.tennessee.edu/utk_gradthes/5350/
Dupraz, C., Reid, R. P., Braissant, O., Decho, A. W., Norman, R. S., and Visscher, P. T. (2009). Processes of carbonate precipitation in modern microbial mats. Earth-Sci. Rev. 96, 141–162. doi:10.1016/j.earscirev.2008.10.005
Ernst, R. E., Okrugin, A. V., Veselovskiy, R. V., Kamo, S. L., Hamilton, M. A., Pavlov, V., et al. (2016). The 1501 Ma Kuonamka Large Igneous Province of Northern Siberia: U-Pb geochronology, geochemistry, and links with coeval magmatism on other crustal blocks. Russ. Geol. Geophys. 57, 653–671. doi:10.1016/j.rgg.2016.01.015
Eugster, H. P., and Jones, B. F. (1968). Gels composed of sodium-aluminum silicate, Lake Magadi, Kenya. Science 161, 160–163. doi:10.1126/science.161.3837.160
Fadel, A., Lepot, K., Bernard, S., Addad, A., Riboulleau, A., and Knoll, A. H. (2024). Ultrastructural perspectives on the biology and taphonomy of tonian microfossils from the Draken Formation, spitsbergen. Geobiology 22, e70000. doi:10.1111/gbi.70000
Fairchild, I. J., Knoll, A. H., and Swett, K. (1991). Coastal lithofacies and biofacies associated with syndepositional dolomitization and silicification (Draken Formation, Upper Riphean, Svalbard). Precam. Res. 53, 165–197. doi:10.1016/0301-9268(91)90071-h
Flörke, O. W., Graetsch, H., Martin, B., Röller, K., and Wirth, R. (1991). Nomenclature of micro-and non-crystalline silica minerals, based on structure and microstructure. Neues Jahrb. Mineral. 163, 19–42. Available online at: https://pascal-francis.inist.fr/vibad/index.php?action=getRecordDetail&idt=6481832
Folk, R. L., and Pittman, J. S. (1971). Length-slow chalcedony; a new testament for vanished evaporites. J. Sed. Res. 41, 1045–1058. doi:10.1306/74D723F1-2B21-11D7-8648000102C1865D
Foucher, F., and Westall, F. (2013). Raman imaging of metastable opal in carbonaceous microfossils of the 700-800 Ma old Draken Formation. Astrobiology 13, 57–67. doi:10.1089/ast.2012.0889
Fralick, P., Davis, D. W., and Kissin, S. A. (2002). The age of the Gunflint Formation, Ontario, Canada: single zircon U Pb age determinations from reworked volcanic ash. Can. J. Earth Sci. 39, 1085–1091. doi:10.1139/e02-028
Gabriel, N. W., Papineau, D., She, Z., Leider, A., and Fogel, M. L. (2021). Organic diagenesis in stromatolitic dolomite and chert from the late Palaeoproterozoic McLeary Formation. Precam. Res. 354, 106052. doi:10.1016/j.precamres.2020.106052
Gibson, T. M., Shih, P. M., Cumming, V. M., Fischer, W. W., Crockford, P. W., Hodgskiss, M. S. W., et al. (2018). Precise age of Bangiomorpha pubescens dates the origin of eukaryotic photosynthesis. Geology 46, 135–138. doi:10.1130/G39829.1
Glikson, A. Y. (1996). Geology of the western musgrave block, Central Australia, with particular reference to the mafic-ultramafic giles complex. Canberra: Australian Government Publishing Service, 239.
Gorokhov, I. M., Kuznetsov, A. B., Semikhatov, M. A., Vasil’eva, I. M., Rizvanova, N. G., Lipenkov, G. V., et al. (2019). Early riphean Billyakh group of the anabar uplift, north Siberia: C–O isotopic geochemistry and Pb–Pb age of dolomites. Strat. Geol. Correl. 27, 514–528. doi:10.1134/s0869593819050022
Graetsch, H., Gies, H., and Topalović, I. (1994). NMR, XRD and IR Study on microcrystalline opals. Phys. Chem. Miner. 21, 166–175. doi:10.1007/bf00203147
Grey, K., Hocking, R. M., Stevens, M. K., Bagas, L., Carlsen, G. M., Irimies, F., et al. (2005). Lithostratigraphic nomenclature of the Officer Basin and correlative parts of the paterson orogen, 93. Western Australia: Western Australia Geological Survey, 89.
Guo, Z., Peng, X., Czaja, A. D., Chen, S., and Ta, K. (2018). Cellular taphonomy of well-preserved Gaoyuzhuang microfossils: a window into the preservation of ancient cyanobacteria. Precam. Res. 304, 88–98. doi:10.1016/j.precamres.2017.11.007
Halverson, G. P., Kunzmann, M., Strauss, J. V., and Maloof, A. C. (2018). The Tonian-Cryogenian transition in northeastern Svalbard. Precam. Res. 319, 79–95. doi:10.1016/j.precamres.2017.12.010
Hamouda, A. A., and Amiri, H. A. A. (2014). Factors affecting alkaline sodium silicate gelation for in-depth reservoir profile modification. Energies 7, 568–590. doi:10.3390/en7020568
Hattori, I., Umeda, M., Nakagawea, T., and Yamamoto, H. (1996). From chalcedonic chert to quartz chert; diagenesis of chert hosted in a Miocene volcanic-sedimentary succession, Central Japan. J. Sed. Res. 66, 163–174. doi:10.1306/D42682E8-2B26-11D7-8648000102C1865D
Heaney, P. J. (1993). A proposed mechanism for the growth of chalcedony. Contrib. Mineral. Petrol. 115, 66–74. doi:10.1007/BF00712979
Heaney, P. J. (1995). Moganite as an indicator for vanished evaporites; a testament reborn? J. Sed. Res. 65, 633–638. doi:10.1306/D4268180-2B26-11D7-8648000102C1865D
Hendry, J. P., and Trewin, N. H. (1995). Authigenic quartz microfabrics in Cretaceous turbidites; Evidence for silica transformation processes in Ssandstones. J. Sed. Res. 65, 380–392. doi:10.1306/D42680CC-2B26-11D7-8648000102C1865D
Herdianita, N. R., Browne, P. R. L., Rodgers, K. A., and Campbell, K. A. (2000). Mineralogical and textural changes accompanying ageing of silica sinter. Miner. Deposita 35, 48–62. doi:10.1007/s001260050005
Hesse, R. (1989). Silica diagenesis: origin of inorganic and replacement cherts. Earth-Sci. Rev. 26, 253–284. doi:10.1016/0012-8252(89)90024-X
Hill, A. C., Arouri, K., Gorjan, P., and Walter, M. R. (2000). Geochemistry of marine and nonmarine environments of a Neoproterozoic cratonic carbonate/evaporite: the Bitter Springs Formation, Central Australia. SEPM Spec. Pub. 67, 327–344. doi:10.2110/pec.00.67.0327
Hofmann, H. J. (1975). Stratiform precambrian stromatolites, belcher islands, Canada; relations between silicified microfossils and microstructure. Am. J. Sci. 275 (10), 1121–1132. doi:10.2475/ajs.275.10.1121
Hofmann, H. J., and Jackson, G. D. (1991). Shelf-facies microfossils from the uluksan group (proterozoic bylot supergroup), Baffin Island, Canada. J. Paleo. 65, 361–382. doi:10.1017/s0022336000030353
Iler, R. K. (1979). “The chemistry of silica: solubility, polymerization,” in Colloid and surface properties, and biochemistry. New York: Wiley.
Jing, Y., Chen, Z.-Q., and Tu, C. (2022). A late Paleoproterozoic microfossil community from siliceous granules, Dahongyu Formation, North China. Precam. Res. 377, 106723. doi:10.1016/j.precamres.2022.106723
Jones, B., and Renault, R. W. (2007). Microstructural changes accompanying the opal-A to opal CT transition: new evidence from the silicious sinters of Geysir, Haukadalur, Iceland. Sedimentology 54, 921–948. doi:10.1111/j.1365-3091.2007.00866.x
Kah, L. C. (2000). Preservation of depositional δ18O signatures in Proterozoic dolostones: geochemical constraints on seawater chemistry and early diagenesis. SEPM Spec.Pub. 67, 345–360.
Kah, L. C., and Knoll, A. H. (1996). Microbenthic distribution of Proterozoic tidal flats: environmental and taphonomic considerations. Geology 24, 79–82. doi:10.1130/0091-7613(1996)024<0079:mdoptf>2.3.co;2
Kile, D. E., and Eberl, D. D. (2003). On the origin of size-dependent and size-independent crystal growth: influence of advection and diffusion. Am. Minerol. 88, 1514–1521. doi:10.2138/am-2003-1014
Kile, D. E., Eberl, D. D., Hoch, A. R., and Reddy, M. M. (2000). An assessment of calcite crystal growth mechanisms based on crystal size distributions. Geochim. Cosmochim. Acta 64, 2937–2950. doi:10.1016/s0016-7037(00)00394-x
Knauth, L. P. (1979). A model for the origin of chert in limestone. Geology 7, 274–277. doi:10.1130/0091-7613(1979)7<274:amftoo>2.0.co;2
Knauth, L. P., Heaney, P. J., Prewitt, C. T., and Gibbs, G. V. (1994). “Petrogenesis of chert,” in Silica: physical behavior, geochemistry, and materials applications (AZ: Tempe), 233–258. doi:10.1515/9781501509698-012
Knoll, A. H. (1982). Microfossils from the late precambrian draken conglomerate, ny friesland, svalbard. J. Paleo, 755–790. Available online at: https://www.jstor.org/stable/1304406
Knoll, A. H. (1985). The distribution and evolution of microbial life in the late Proterozoic Era. Ann. Rev. Microbi. 39, 391–417. doi:10.1146/annurev.mi.39.100185.002135
Knoll, A. H., and Golubic, S. (1979). Anatomy and taphonomy of a Precambrian algal stromatolite. Precam. Res. 10, 115–151. doi:10.1016/0301-9268(79)90022-6
Knoll, A. H., and Sergeev, V. N. (1995). Taphonomic and evolutionary changes across the Mesoproterozoic-Neoproterozoic transition. Neues Jahrb. Geol. Palaontologie 195, 289–302. doi:10.1127/njgpa/195/1995/289
Knoll, A. H., and Swett, K. (1990). Carbonate deposition during the late proterozoic era: an example from spitsbergen. Am. J. Sci. 290, 104–132. Available online at: https://pubmed.ncbi.nlm.nih.gov/11538689/
Knoll, A. H., Swett, K., and Mark, J. (1991). Paleobiology of a neoproterozoic tidal flat/lagoonal complex: the draken conglomerate formation, spitsbergen. J. Paleo. 65, 531–570. doi:10.1017/S0022336000030663
Knoll, A. H., Wörndle, S., and Kah, L. C. (2013). Covariance of microfossil assemblages and microbialite textures across an upper Mesoproterozoic carbonate platform. Palaios 28, 453–470. doi:10.2110/palo.2013.p13-005r
Konhauser, K. O., Jones, B., Phoenix, V. R., Ferris, G., and Renaut, R. W. (2004). The microbial role in hot spring silicification. AMBIO 33, 552–558. doi:10.1579/0044-7447-33.8.552
Konhauser, K. O., Phoenix, V. R., Bottrell, S. H., Adams, D. G., and Head, I. M. (2001). Microbial–silica interactions in Icelandic hot spring sinter: possible analogues for some Precambrian siliceous stromatolites. Sedimentology 48, 415–433. doi:10.1046/j.1365-3091.2001.00372.x
Kremer, B., Kazmierczak, J., Łukomska-Kowalczyk, M., and Kempe, S. (2012). Calcification and silicification: fossilization potential of cyanobacteria from stromatolites of Niuafo ‘ou's Caldera Lakes (Tonga) and implications for the early fossil record. Astrobiology 12, 535–548. doi:10.1089/ast.2011.0742
Lalonde, S. V., Konhauser, K. O., Reysenbach, A.-L., and Ferris, F. G. (2005). The experimental silicification of Aquificales and their role in hot spring sinter formation. Geobiology 3, 41–52. doi:10.1111/j.1472-4669.2005.00042.x
Leet, K., Lowenstein, T. K., Renaut, R. W., Bernhart Owen, R., and Cohen, A. (2021). Labyrinth patterns in Magadi (Kenya) cherts: evidence for early formation from siliceous gels. Geology 49, 1137–1142. doi:10.1130/G48771.1
Lepot, K., Addad, A., Knoll, A. H., Wang, J., Troadec, D., Béché, A., et al. (2017). Iron minerals within specific microfossil morphospecies of the 1.88 Ga Gunflint Formation. Nat. Comm. 8, 14890. doi:10.1038/ncomms14890
Li, J. C. M. (1962). Possibility of subgrain rotation during recrystallization. J. App. Phys. 33, 2958–2965. doi:10.1063/1.1728543
Luo, C., and Zhu, M. (2025). Chert Lagerstätten as a new window to the biological revolution across the Ediacaran−Cambrian boundary. Geology. doi:10.1130/G52956.1
Lynne, B. Y. (2015). Impact of three common post-depositional environmental settings on siliceous sinter diagenesis: an eight year experiment. J. Volcan. Geotherm. Res. 292, 84–101. doi:10.1016/j.jvolgeores.2015.01.007
Lynne, B. Y., and Campbell, K. A. (2004). Morphologic and mineralogic transitions from opal-A to opal-CT in low-temperature siliceous sinter diagenesis, Taupo Volcanic Zone, New Zealand. J. Sed. Res. 74, 561–579. doi:10.1306/011704740561
Lynne, B. Y., Campbell, K. A., James, B., Browne, P. R. L., and Moore, J. N. (2007). Tracking crystallinity in siliceous hot-spring deposits. Am. J. Sci. 307, 612–641. doi:10.2475/03.2007.03
Maliva, R. G., Knoll, A. H., and Simonson, B. M. (2005). Secular change in the Precambrian silica cycle: insights from chert petrology. Geol. Soc. Am. Bull. 117, 835–845. doi:10.1130/B25555.1
Maliva, R. G., and Siever, R. (1989). Nodular chert formation in carbonate rocks. J. Geol. 97, 421–433. doi:10.1086/629320
Manning-Berg, A., Selly, T., and Bartley, J. K. (2022). Actualistic approaches to interpreting the role of biological decomposition in microbial preservation. Geobiology 20, 216–232. doi:10.1111/gbi.12475
Manning-Berg, A. R., and Kah, L. C. (2017). Proterozoic microbial mats and their constraints on environments of silicification. Geobiology 15, 469–483. doi:10.1111/gbi.12238
Manning-Berg, A. R., Wood, R. S., Williford, K. H., Czaja, A. D., and Kah, L. C. (2019). The taphonomy of Proterozoic microbial mats and implications for early diagenetic silicification. Geosciences 9, 40. doi:10.3390/geosciences9010040
Matinfar, M., and Nychka, J. A. (2023). A review of sodium silicate solutions: structure, gelation, and syneresis. Adv. Colloid Interface Sci. 322, 103036. doi:10.1016/j.cis.2023.103036
Merino, E., Wang, Y., and Deloule, E. (1994). Genesis of banded, fibrous, and twisted quartz by catalysis, unstable crystallization fronts, and substitution. Mineral. Mag. 58, 597–598. doi:10.1180/minmag.1994.58A.2.47
Miehe, G., Graetsch, H., and Flörke, O. W. (1984). Crystal structure and growth fabric of length-fast chalcedony. Phys. Chem. Miner. 10, 197–199. doi:10.1007/BF00309311
Moore, K. R., Gong, J., Pajusalu, M., Skoog, E. J., Xu, M., Soto, T. F., et al. (2021). A new model for silicification of cyanobacteria in Proterozoic tidal flats. Geobiology 19, 438–449. doi:10.1111/gbi.12447
Moore, K. R., Pajusalu, M., Gong, J., Sojo, V., Matreux, T., Braun, D., et al. (2020). Biologically mediated silicification of marine cyanobacteria and implications for the Proterozoic fossil record. Geology 48, 862–866. doi:10.1130/g47394.1
Moraishi, H. (1989). Experimental study of amorphous silica crystallization in Na2CO3–NaHCO3 solutions as a source of chert formation in Lake Magadi. Bill. Chem. Soc. Jap. 68, 3027–3033. doi:10.1246/bcsj.68.3027
Moreau, J. W., and Sharp, T. G. (2004). A transmission electron microscopy study of silica and kerogen biosignatures in ˜1.9 Ga Gunflint microfossils. Astrobiology 4, 196–210. doi:10.1089/153110704323175142
Moxon, T., Nelson, D. R., and Zhang, M. (2006). Agate recrystallisation: evidence from samples found in Archaean and Proterozoic host rocks, Western Australia. Aust. J. Earth Sci. 53, 235–248. doi:10.1080/08120090500499255
Oehler, J. H. (1976a). Experimental studies in Precambrian paleontology: structural and chemical changes in blue-green algae during simulated fossilization in synthetic chert. Geol. Soc. Am. Bull. 87, 117–129. doi:10.1130/0016-7606(1976)87<117:esipps>2.0.co;2
Oehler, J. H. (1976b). Hydrothermal crystallization of silica gel. Geol. Soc. Am. Bull. 87, 1143–1152. doi:10.1130/0016-7606(1976)87<1143:hcosg>2.0.co;2
Osorio-Rodriquez, D., Metcalfe, K. S., McGlynn, W. E., Yu, H., Dekas, A. E., Ellisman, M., et al. (2023). Microbially induced precipitation of silica by anaerobic methane-oxidizing consortia and implications for microbial fossil preservation. Proc. Nat. Acad. Sci. 120, e2302156120. doi:10.1073/pnas.2302156120
Peng, X., Guo, Z., House, C. H., Chen, S., and Ta, K. (2016). SIMS and NanoSIMS analyses of well-preserved microfossils imply oxygen-producing photosynthesis in the Mesoproterozoic anoxic ocean. Chem. Geo. 441, 24–34. doi:10.1016/j.chemgeo.2016.08.011
Petrash, D. A., Robbins, L. J., Shapiro, R. S., Mojzsis, S. J., and Konhauser, K. O. (2016). Chemical and textural overprinting of ancient stromatolites: timing, processes, and implications for their use as paleoenvironmental proxies. Precam. Res. 278, 145–160. doi:10.1016/j.precamres.2016.03.010
Schmid, S., Kunzmann, M., and Pagès, A. (2017). Inorganic geochemical evaluation of hydrocarbon source rock potential of Neoproterozoic strata in the Amadeus Basin, Australia. Mar. Petrol. Geol. 86, 1092–1105. doi:10.1016/j.marpetgeo.2017.07.015
Schmidt, P., Slodczyk, A., Léa, V., Davidson, A., Puaud, S., and Sciau, P. (2013). A comparative study of the thermal behaviour of length-fast chalcedony, length-slow chalcedony (quartzine) and moganite. Phys. Chem. Miner. 40, 331–340. doi:10.1007/s00269-013-0574-8
Schopf, J. W. (1968). Microflora of the bitter Springs Formation, late precambrian, Central Australia. J. Paleontol. 42, 651–688. Available online at: https://www.jstor.org/stable/1302368
Schopf, J. W. (1993). Microfossils of the early archean apex chert: new evidence of the antiquity of life. Science 260, 640–646. doi:10.1126/science.260.5108.640
Schopf, J. W., and Kudryavtsev, A. B. (2012). Biogenicity of Earth’s earliest fossils: a resolution of the controversy. Gondwana Res. 22, 761–771. doi:10.1016/j.gr.2012.07.003
Schubel, K. A., and Simonson, B. M. (1990). Petrography and diagenesis of cherts from lake magadi, Kenya. J. Sed. Res. 60, 761–776. doi:10.1306/212F9269-2B24-11D7-8648000102C1865D
Schulz, K. J., and Cannon, W. F. (2007). The penokean orogeny in the lake superior region. Precam. Res. 157, 4–25. doi:10.1016/j.precamres.2007.02.022
Sergeev, V. N., Knoll, A. H., and Grotzinger, J. P. (1995). Paleobiology of the mesoproterozoic Billyakh group, anabar uplift, northern Siberia. J. Paleo 69, 1–37. doi:10.1017/S0022336000062375
Sergeev, V. N., Vorob’eva, N. G., and Petrov, P. Y. (2017). The biostratigraphic conundrum of Siberia: do true Tonian–Cryogenian microfossils occur in Mesoproterozoic rocks? Precam Res. 299, 282–302. doi:10.1016/j.precamres.2017.07.024
Shapiro, R. S., and Konhauser, K. O. (2015). Hematite-coated microfossils: primary ecological fingerprint or taphonomic oddity of the Paleoproterozoic? Geobiology 13, 209–224. doi:10.1111/gbi.12127
Sharma, M., and Sergeev, V. N. (2004). Genesis of carbonate precipitate patterns and associated microfossils in Mesoproterozoic Formations of India and Russia—a comparative study. Precam. Res. 134, 317–347. doi:10.1016/j.precamres.2004.07.001
Simonson, B. M. (1987). Early silica cementation and subsequent diagenesis in arenites from four early Proterozoic iron formations of North America. J. Sed. Pet. 57, 494–511. doi:10.1306/212F8B75-2B24-11D7-8648000102C1865D
Southgate, P. N. (1986). Depositional environment and mechanism of preservation of microfossils, upper proterozoic bitter Springs Formation, Australia. Geology 14, 683–686. doi:10.1130/0091-7613(1986)14<683:deamop>2.0.co;2
Southgate, P. N. (1989). Relationships between cyclicity and stromatolite form in the late proterozoic bitter Springs Formation, Australia. Sedimentology 36, 323–339. doi:10.1111/j.1365-3091.1989.tb00610.x
Stipp, M., Stünitz, H., Heilbronner, R., and Schmid, S. M. (2002). Dynamic recrystallization of quartz: correlation between natural and experimental conditions. Geol. Soc. Lond. Spec. Pub. 200, 171–190. doi:10.1016/S0191-8141(02)00035-4
Sugitani, K., Yamamoto, K., Adachi, M., Kawabe, I., and Sugisaki, R. (1998). Archean cherts derived from chemical, biogenic and clastic sedimentation in a shallow restricted basin: examples from the Gorge Creek Group in the Pilbara Block. Sedimentology 45, 1045–1062. doi:10.1046/j.1365-3091.1998.00198.x
Turner, E. C. (2009). Mesoproterozoic carbonate systems in the borden basin, Nunavut. Can. J. Earth Sci. 46, 915–938. doi:10.1139/E09-062
Urai, J. L., Means, W. D., and Lister, G. S. (1986). Dynamic recrystallization of minerals. Min. Rock Deform. 36, 161–199. doi:10.1029/gm036p0161
Vorob’eva, N. G., Sergeev, V. N., and Petrov, P. Y. (2015). Kotuikan Formation assemblage: a diverse organic-walled microbiota in the mesoproterozoic anabar auccession, northern Siberia. Precam. Res. 256, 201–222. doi:10.1016/j.precamres.2014.11.011
Wacey, D., McLoughlin, N., Green, O. R., Parnell, J., Stoakes, C. A., and Brasier, M. D. (2006). The ∼3.4 billion-year-old Strelley Pool sandstone: a new window into early life on Earth. Int. J. Astrobio. 5, 333–342. doi:10.1017/S1473550406003466
Wang, Y., and Merino, E. (1990). Self-organizational origin of agates: banding, fiber twisting, composition, and dynamic crystallization model. Geochim. Cosmochim. Acta 54, 1627–1638. doi:10.1016/0016-7037(90)90396-3
Westall, F. (1995). The experimental silicification of microorganisms. Palaeontology 38, 495–528. Available online at: https://www.palass.org/publications/palaeontology-journal/archive/38/3/article_pp495-528
Wilhelm, S., and Kind, M. (2015). Influence of pH, temperature and sample size on natural and enforced syneresis of precipitated silica. Polymers 7, 2504–2521. doi:10.3390/polym7121528
Keywords: Precambrian, microfossil, chert, chalcedony, neomorphism
Citation: Gauvey K and Kah LC (2025) Unravelling neomorphism: recrystallization pathways in Proterozoic microfossiliferous chert. Front. Earth Sci. 13:1598200. doi: 10.3389/feart.2025.1598200
Received: 22 March 2025; Accepted: 21 April 2025;
Published: 06 May 2025.
Edited by:
Shahab Varkouhi, University College London, United KingdomReviewed by:
Zixiao Guo, Hebei Normal University, ChinaIsraa S. Abu-Mahfouz, King Fahd University of Petroleum and Minerals, Saudi Arabia
Copyright © 2025 Gauvey and Kah. This is an open-access article distributed under the terms of the Creative Commons Attribution License (CC BY). The use, distribution or reproduction in other forums is permitted, provided the original author(s) and the copyright owner(s) are credited and that the original publication in this journal is cited, in accordance with accepted academic practice. No use, distribution or reproduction is permitted which does not comply with these terms.
*Correspondence: Kaitlyn Gauvey, a2dhdXZleUB2b2xzLnV0ay5lZHU=