- 1College of Geography and Tourism, Hengyang Normal University, Hengyang, China
- 2College of Tourism and Geographical Science, Leshan Normal University, Leshan, China
Accurately determining the retrograde metamorphic ages of pelitic granulites is crucial for reconstructing their metamorphic evolution and understanding associated post-collisional extensional and subsequent exhumation processes in orogenic belts. This study integrates zircon and monazite geochronology with thermodynamic modelling to determine the retrograde metamorphic history of medium-pressure (MP) pelitic granulites in the Qianlishan Complex of the Khondalite Belt, North China Craton. Our findings show that all MP pelitic granulites follow similar clockwise pressure-temperature (P-T) trajectories, with precisely defined post-peak P-T conditions of 775°C–825°C and 4.9–6.5 kbar. U-Pb zircon dating via LA-ICP-MS on three MP granulites produced metamorphic ages of 1943 ± 18 Ma, 1931 ± 10 Ma, and 1941 ± 10 Ma. Similarly, monazite U-Pb analyses of the same samples yielded ages of 1932 ± 3 Ma, 1935 ± 3 Ma, and 1939 ± 3 Ma. A well-matched set of dates indicates a metamorphic episode at ∼1.93 Ga, which established a metamorphic period during the granulite-facies retrograde cooling phase. The geochronological studies of pelitic granulites from the Qianlishan Complex indicate that a peak-pressure metamorphic event took place between 1.96 and 1.94 Ga, with subsequent decompression and retrograde cooling occurring around 1.93 Ga. These findings reinforce the hypothesis that a continent-continent collision occurred in the Khondalite Belt around 1.95 Ga, followed by a rapid slab-breakoff at shallow depths, which triggered the uplift of pelitic granulites in the western Khondalite Belt to mid-crustal levels and their subsequent cooling around 1.93 Ga.
1 Introduction
As key accessory minerals in metamorphic rocks, zircon and monazite play a vital role in geochronology, providing critical insights into the timing of metamorphism and the thermal and tectonic history of high-grade terrains (Parrish, 1990; Rubatto et al., 2001; Rubatto et al., 2013; Kohn and Malloy, 2004; Harley et al., 2007; Johnson et al., 2015; Jiao et al., 2020). Their effectiveness stems from the U-Pb system’s ability to preserve records of polyphase metamorphic evolution, even under relatively high-temperature conditions (Rubatto et al., 2001). Compared to zircon, monazite effectively excludes common Pb during its growth, minimizing initial Pb interference while also exhibiting strong resistance to Pb diffusion loss and high tolerance to radiation damage (Seydoux-Guillaume et al., 2002; Cherniak et al., 2004). Furthermore, with a lower closure temperature (∼725°C; Parrish, 1990) than zircon (>900°C; Cherniak and Watson, 2001), monazite is more prone to recrystallization and age resetting during high-grade metamorphism, allowing it to effectively record multiple metamorphic stages, and thus serving as a valuable tool for unraveling the complex histories of polyphase metamorphic terrains (Dewolf et al., 1993; Catlos et al., 2002; Gibson et al., 2004; Kohn and Malloy, 2004; Mahan et al., 2006; Zhang et al., 2012). Given these distinctions, using zircon and monazite in geochronological studies offers complementary insights (Rubatto et al., 2013).
The Qianlishan Complex is located in the western Khondalite Belt (Figure 1) and contains predominantly medium-pressure (MP) pelitic granulites interspersed with occasional high-pressure (HP) pelitic and felsic granulites (Yin et al., 2014; Wu et al., 2020). Extensive research has been conducted on the pressure-temperature (P-T) evolution and peak metamorphic timing of high-pressure (HP) granulites within the Qianlishan Complex (Yin et al., 2009; Yin et al., 2014; Wu et al., 2020). The observed clockwise P-T trajectories, along with a zircon metamorphic dating at approximately 1.95 Ga, offer compelling evidence to support a continental collision between the Yinshan and Ordos Blocks, which led to the formation of the Khondalite Belt at ∼1.95 Ga (Yin et al., 2009; Yin et al., 2014; Qiao et al., 2016; Wu et al., 2020). Despite their widespread occurrence, MP granulites have received relatively limited focus. As representative lithologies of the middle-lower crust, these rocks are of fundamental significance, preserving crucial evidence of multistage metamorphic evolution, especially retrograde processes. Deciphering their metamorphic history is key to reconstructing the timing and geodynamic mechanisms of post-collisional extensional and subsequent exhumation processes in the Khondalite Belt.
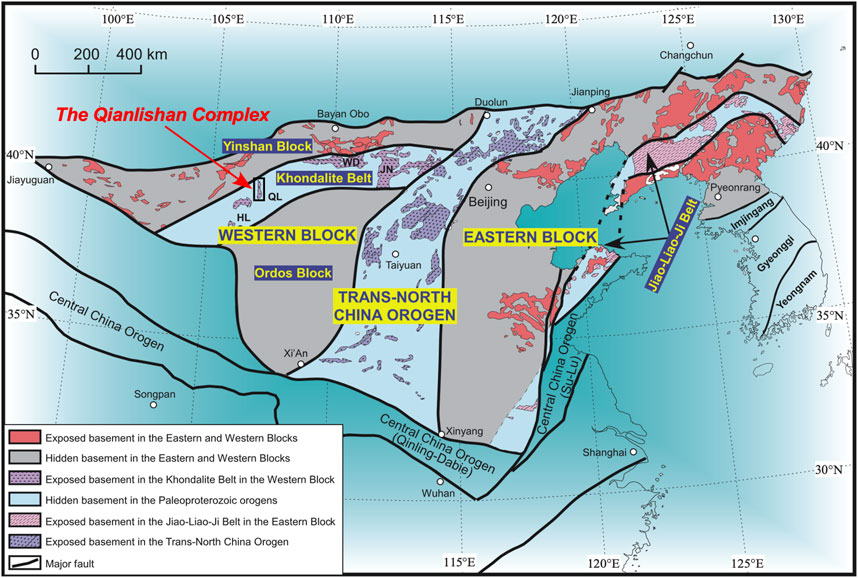
Figure 1. Simplified tectonic framework of the North China Craton (NCC, modified after Zhao et al., 2005). Abbreviations: HL, Helanshan Complex; QL, Qianlishan Complex; WD, Wulashan-Daqingshan Complex; JN, Jining Complex.
This study investigates three representative MP pelitic granulite samples from the Qianlishan Complex. Petrographic observations, together with phase equilibrium modelling, were employed to analyze the metamorphic history of these rocks by focusing on retrograde segments of the P-T path, including decompression and cooling. Furthermore, this study integrates the U-Pb dating of monazite and zircon to determine geochronological data for the first time regarding the MP pelitic granulites within the Qianlishan Complex, offering valuable insights into the timing of retrograde cooling metamorphism. These findings establish complete P-T-t trajectories of pelitic granulites and enhance deeper understanding of the tectonic evolution of the Khondalite Belt.
2 Geological background
The North China Craton (NCC) exists as an ancient craton that formed through Archean crustal development followed by reorganizational processes, with micro-blocks distributed along Paleoproterozoic linear structural belts (Zhao et al., 2012; Zhai, 2014). Located in the NCC, the Khondalite Belt represents a significant Paleoproterozoic orogenic belt with an east-west orientation, delineating the Yinshan Block from the Ordos Block in the Western Block (Figure 1). The belt consists of, from east to west, the Jining Complex, the Wulashan-Daqingshan Complex, and the Qianlishan-Helanshan Complex (Figure 1). Khondalites, defined by their assemblage of primarily Al-rich gneisses, calc-silicate rocks, marbles, and garnet-bearing quartzites, form the dominant lithologies within these complexes (Condie et al., 1992; Lu et al., 1996; Zhai, 2022). These Khondalites are accompanied by minor mafic granulites, charnockites, S-type granites, and tonalite-trondhjemite-granodiorite gneiss (Peng et al., 2010; Peng et al., 2012; Dan et al., 2012; Liu et al., 2014; Wang et al., 2018). The Khondalite Belt has undergone a complex tectonothermal history, involving successive stages of metamorphism, magmatism, and deformation (Yin et al., 2023). This evolution began with subduction-related processes and culminated in continental collision followed by post-orogenic extensional tectonics (Yin et al., 2023). Two distinct types of metamorphism involving HP metamorphism (Zhou et al., 2010; Yin et al., 2014; Yin et al., 2015; Wu et al., 2020; Liu et al., 2017) and ultra-high-temperature (UHT) metamorphism (Santosh et al., 2007; Santosh et al., 2012; Jiao et al., 2011; Jiao et al., 2017; Jiao et al., 2020; Jiao and Guo, 2011; Guo et al., 2012; Liu et al., 2012; Yang et al., 2014; Li and Wei, 2016; Li and Wei, 2018; Gou et al., 2018; Gou et al., 2019; Li et al., 2019; Wu et al., 2024) have been identified within the belt. In a recently developed geodynamic model, the complex thermal evolution of UHT metamorphism, characterized by alternating heating and cooling cycles, is explained as the result of two discrete episodes of lithospheric extension (Qi et al., 2024).
At the westernmost of the Khondalite Belt lies the Qianlishan Complex, a metamorphic complex exhibiting upper amphibolite-to granulite-facies conditions. Its primary constituents include Khondalites and Paleoproterozoic multistage granitic plutons (Lu et al., 1996; Li et al., 2022). In this region, the Khondalites are grouped under the Qianlishan Group, which comprises the Qianligou, Habuqigai, and Chaganguole Formations (Figure 2). Following their deposition at approximately 2.0–1.95 Ga, the protoliths of the Qianlishan Group experienced a granulite-facies peak metamorphic event around 1.95 Ga (Yin et al., 2009; Qiao et al., 2016; He et al., 2017; Wu et al., 2020). This metamorphism is primarily characterized by HP pelitic granulites that contain a peak mineral assemblage of garnet, kyanite, plagioclase, biotite, K-feldspar, and quartz (Yin et al., 2014; Wu et al., 2020). Metamorphic investigations indicate that these HP pelitic and felsic granulites in the Qianlishan Complex share a broadly similar clockwise P-T trajectory, peaking at conditions of 11–15 kbar and 800°C–850°C (Yin et al., 2014; Wu et al., 2020). The tectono-magmatic evolution of the complex also includes three main magmatic events at ∼2.06 Ga, ∼1.95 Ga, and ∼1.92 Ga (Li et al., 2022). Two significant deformation phases are also documented: The first (D1) occurred between 1.98 and 1.93 Ga, while the second (D2) took place between 1.93 and 1.90 Ga (Yin et al., 2020).
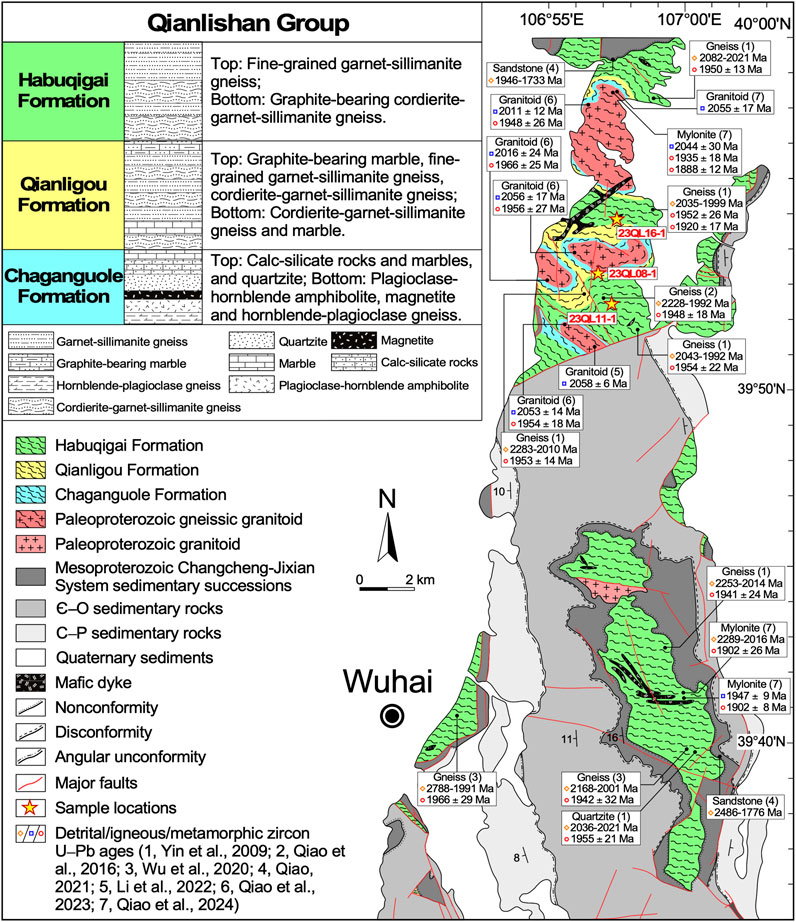
Figure 2. Geological overview of the Qianlishan Complex, modified from Yin et al. (2011) and Qiao et al. (2024), showing the sampling sites of MP pelitic granulite. U-Pb zircon ages for detrital, igneous, and metamorphic events are referenced from Yin et al. (2009), Qiao et al. (2016), Qiao et al. (2023), Qiao et al. (2024), Qiao (2021), Wu et al. (2020) and Li et al. (2022).
3 Field sampling
Three MP pelitic granulite samples, designated as 23QL08-1, 23QL11-1, and 23QL16-1, were gathered from the Qianlishan Complex. The sampling sites are shown in Figure 2. On an outcrop scale, these pelitic granulite samples exhibit well-developed gneissic foliations and a distinct interlayered texture with felsic granulites and felsic dykes (Figure 3). At the hand specimen scale, the dark gray pelitic granulites exhibit abundant garnet and display signs of significant partial melting, manifested by localized accumulations of melt that form veins and patches (Figure 3).
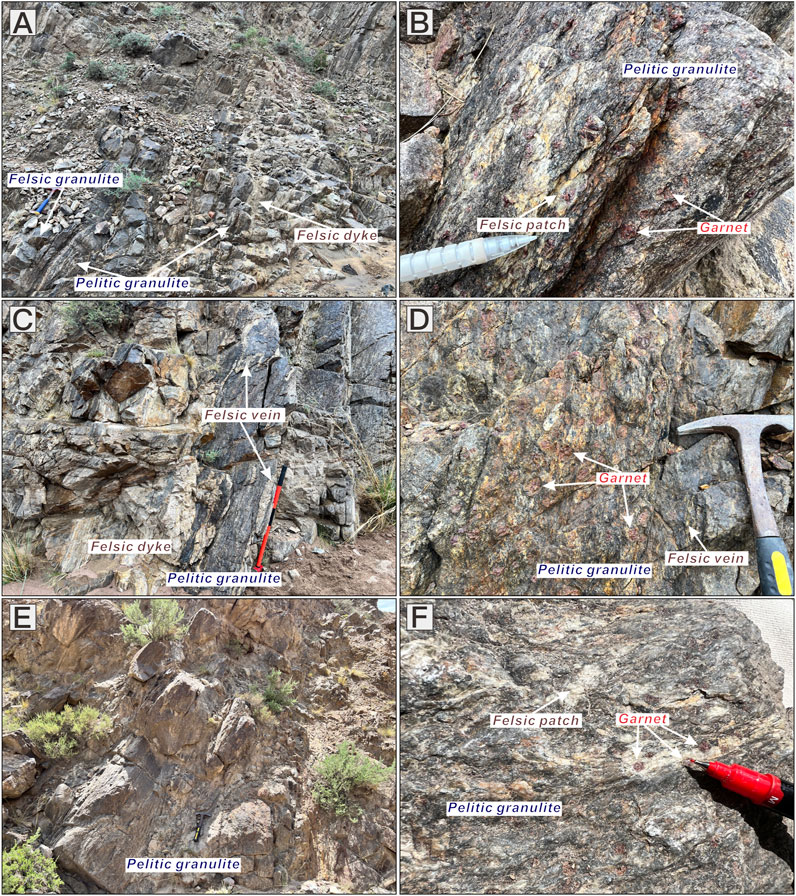
Figure 3. Representative outcrop images of MP pelitic granulites and their surrounding wall rocks in the Qianlishan Complex: (A, B) 23QL08-1, (C, D) 23QL11-1, and (E, F) 23QL16-1.
4 Methods
4.1 Bulk-rock composition
A Rigaku ZSX Primus II wavelength-dispersive X-ray fluorescence (XRF) spectrometer was used at Guangzhou Tuoyan Analytical Technology Co., Ltd., Guangzhou, China, to analyze the primary elemental compositions of three MP pelitic granulite samples. To ensure measurement accuracy, we employed GSR-3/4/6, OU-6, GSR/13, and NOD-P-1 for quality control. The analytical precision was maintained within a relative standard deviation not exceeding 5%.
4.2 U-Pb dating of zircon and monazite
We extracted zircon and monazite from three MP pelitic granulite samples by use of heavy liquid and magnetic techniques, with final grain selection conducted manually. Grains were embedded in epoxy, polished, and analyzed under reflected and transmitted light to view internal textures. Zircon grain internal features became visible through cathodoluminescence (CL) imaging, while the monazite structure became visible through backscattered electron (BSE) imaging.
Laser Ablation Inductively Coupled Plasma Mass Spectrometry (LA-ICP-MS) analyses for U–Pb isotopes and trace elements were undertaken at Guangzhou Tuoyan Analytical Technology Co., Ltd., Guangzhou, China. Then the NWR 193 nm ArF excimer laser system, coupled with Agilent 8900 quadrupole ICP-MS was used for the analysis through an ablation system set at 25 µm for zircon and 30 µm for monazite, at a laser repetition rate of 6 Hz.
Zircon U-Pb dating used the 91500 standard (Wiedenbeck et al., 1995), while trace elements were measured by use of the NIST SRM 610 glass (Reed, 1992) for calibration. Data reliability was achieved through analyses of two secondary reference materials: zircon Plešovice (Sláma et al., 2008) and Tan-Z (Hu et al., 2021). The analytical procedures adhered to the methodologies established by Yuan et al. (2004) and Chu et al. (2020). The dating of monazite samples relied on monazite 44069 (Aleinikoff et al., 2006) for correcting instrumental mass bias and elemental fractionation effects. The trace element calibration employed standard reference materials, NIST SRM 610 and BCR-2G (Jochum et al., 2011).
Data processing, including background signal subtraction, integration of analytical spectra, and time-drift corrections, was implemented by use of Iolite 4.0. The U-Pb age calculations with intercept age or weighted mean age determination came from Isoplot R (Vermeesch, 2018). Supplementary Tables S1,S2 provide the entire U-Pb age data set from zircon and monazite analyses in this study.
5 Results
5.1 Petrography
The three MP granulite samples exhibit distinct mineralogical compositions (Figure 4). In sample 23QL08-1 (Figures 4A,B), K-feldspar is the dominant phase (∼40 vol%), followed by quartz (∼20 vol%) and biotite (∼15 vol%). It also contains moderate amounts of sericitized cordierite (∼12 vol%) and plagioclase (∼8 vol%), with lesser amounts of garnet (∼3 vol%) and sillimanite (∼2 vol%). In contrast, Sample 23QL11-1 (Figures 4C,D) is dominated by sillimanite (∼30 vol%), followed by K-feldspar, quartz, and cordierite, each making up approximately 15 vol%. It also contains notable garnet (∼12 vol%) and biotite (∼10 vol%), with a small amount of plagioclase (∼2 vol%). Meanwhile, Sample 23QL16-1 (Figures 4E,F) features a high proportion of cordierite (∼30 vol%) and sillimanite (∼20 vol%). It has significant quartz and biotite, each comprising around 15 vol%, moderate K-feldspar (∼12 vol%), minor garnet (∼7 vol%), and a trace amount of plagioclase (∼1 vol%). All three samples contain monazite, zircon, and ilmenite as minor accessory phases. Despite their differing mineralogical compositions, the three samples share remarkably similar mineral assemblages. Garnet is found as subhedral crystals and has undergone replacement by cordierite in all three samples (Figures 4A,C,E). Sillimanite occurs in two distinct forms: one as fibrous inclusions enclosed within garnet and cordierite in all three samples (Figures 4C,D,F), and the other as euhedral crystals distributed within the matrix (Samples 23QL11-1 and 23QL16-1; Figures 4D,F). Biotite is present either within the matrix (Figures 4A–C) or as relict flakes embedded in sillimanite + cordierite symplectites (Figures 4C–E). K-feldspar appears as coarse subhedral grains within the matrix in all three samples (Figures 4B,D,F), whereas plagioclase is limited in occurrence and may have formed through melt crystallization (Sample 23QL08-1; Figure 4B).
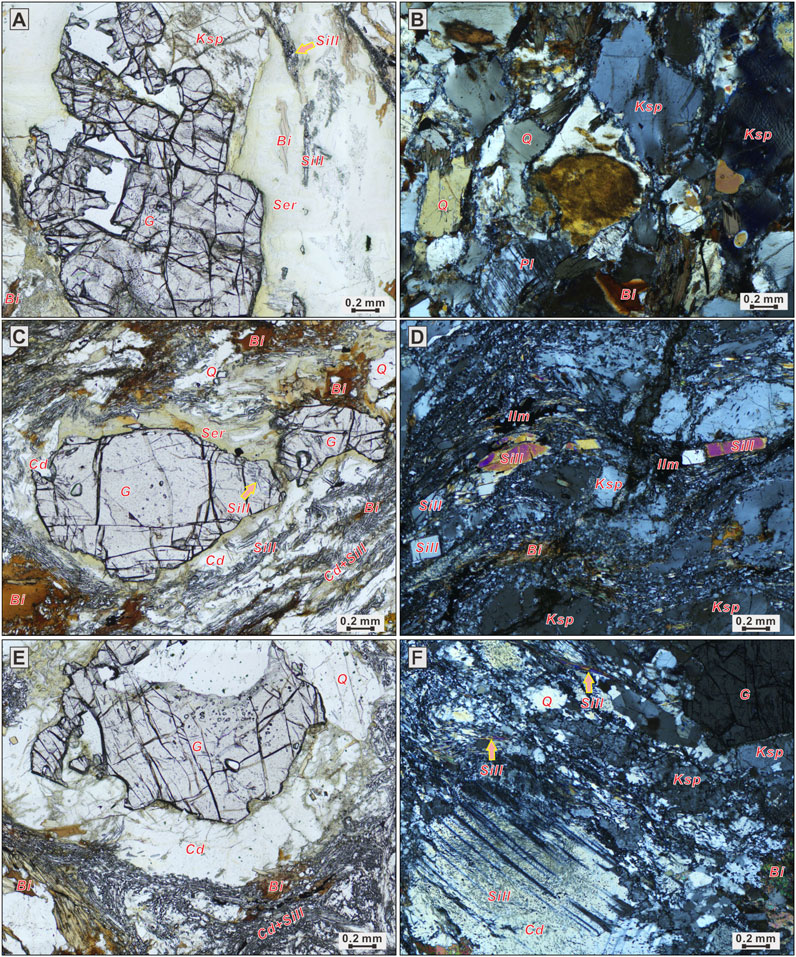
Figure 4. Photomicrographs showing key mineralogical assemblages and textural relationships in MP pelitic granulites: (A–B) 23QL08-1, (C–D) 23QL11-1, and (E–F) 23QL16-1. (A) Garnet porphyroblast enveloped by sericitized cordierite, enclosing sillimanite and biotite inclusions. (B) Matrix composed mainly of K-feldspar, biotite, and quartz, with minor plagioclase. (C) Garnet porphyroblast rimmed by symplectic intergrowths of cordierite and sillimanite. (D) Prismatic sillimanite occurs in the matrix. (E) Anhedral garnet porphyroblast encased by a cordierite corona and outer cordierite-sillimanite symplectites. (F) Fibrous sillimanite enclosed within cordierite.
As discussed above, a distinctive decompression metamorphic reaction texture is recognizable. This is evident from the emergence of a cordierite rim encircling garnet or the formation of cordierite + sillimanite symplectites (Figures 4A,C,E). These features define the metamorphic history, which can be categorized into peak, post-peak, and retrograde phases. The peak metamorphic phase exists without kyanite occurrence while including a g-bi-ksp-sill-liq-ilm-q mineral assemblage. During the post-peak phase, the rock displays g-pl-bi-ksp-sill-cd-liq-ilm-q assemblage. Consequently, the retrograde cooling phase is characterized by a sub-solidus assemblage, devoid of liquid.
5.2 Phase equilibria modelling
GeoPs v3.5.6.21170 software (Xiang and Connolly, 2022) was utilized for phase equilibria modelling, incorporating the ds62 thermodynamic dataset (Holland and Powell, 2011). Simulations were conducted within the NCKFMASHTO system, which includes Na2O, FeO, MgO, Al2O3, CaO, K2O, SiO2, TiO2, H2O, and O (Fe3+/Fetotal) as key components. Owing to its negligible concentration (<0.08 wt%; Supplementary Table S3), MnO was omitted from the modelling process. The total P2O5 content was assumed to be exclusively derived from apatite, necessitating a corresponding adjustment of the CaO content in the model. The appropriate H2O and O (Fe3+/Fetotal) contents were determined using the T–MH2O diagram (Korhonen et al., 2013) and the potassium dichromate titration method, respectively. Due to the O (Fe3+/Fetotal) value obtained for Sample 23QL11-1 using the titration method being excessively high, we adjusted our approach and adopted the T–MO diagram (Korhonen et al., 2013). The modelling incorporated activity-composition (a-x) models for plagioclase (Pl) and K-feldspar (Ksp) (Holland and Powell, 2003), together with garnet (G), silicate melt (Liq), muscovite (Mu), biotite (Bi), staurolite (St), cordierite (Cd), and orthopyroxene (Opx) (White et al., 2014). In addition, ilmenite (Ilm) (White et al., 2014), spinel (Sp), and magnetite (Mt) (White et al., 2002) were incorporated into the model. Additionally, the following minerals were treated as pure phases: andalusite (And), rutile (Ru), kyanite (Ky), sillimanite (Sill), and quartz (Q). The whole-rock geochemical data obtained via XRF, along with the corresponding normalized molar ratios, are presented in Supplementary Table S3.
5.2.1 Sample 23QL08-1
To establish the optimal H2O concentration for Sample 23QL08-1, T–XH2O pseudosection analysis was performed at 5.5 kbar (Figure 5A), aligning with the retrograde pressure range of 4–6 kbar documented in previous research (Yin et al., 2014; Wu et al., 2020). For subsequent thermodynamic modelling, a value of 1.69 mol% H2O was chosen based on the solidus midpoint of post-peak mineral assemblages (g-pl-bi-ksp-sill-cd-liq-ilm-q) (Figure 5A; Korhonen et al., 2013). Due to the restricted H2O content, phase equilibrium modelling was conducted across 700°C–900°C and 4–14 kbar, taking into account an excess of quartz (Figure 5B). The solidus exhibits a sharp slope over a limited temperature interval of approximately 770°C–805°C. Similarly, the biotite-out curve follows a comparable trend but appears at temperatures 20°C–40°C higher. The rutile-in and cordierite-in curves both exhibit a positive correlation with pressure, occurring at pressures above ∼8.2 kbar and below ∼7.0 kbar, respectively.
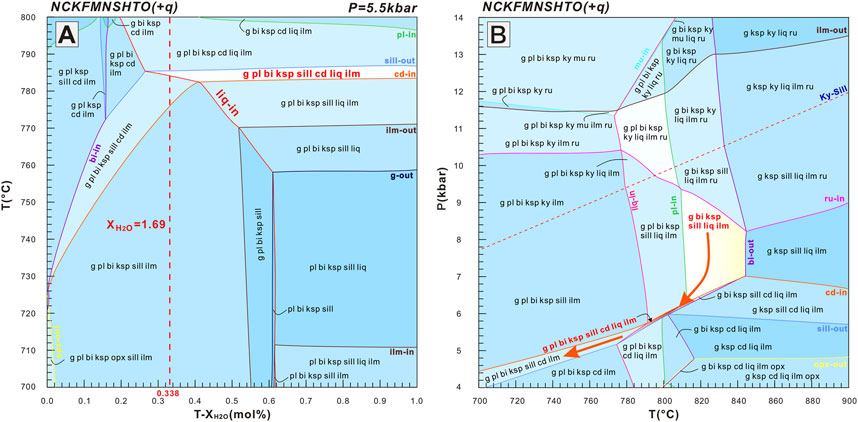
Figure 5. Phase equilibrium modelling results for 23QL08-1. (A) T-XH2O diagram computed at 5.5 kbar, with the red line indicating H2O content used for P-T pseudosection. (B) P-T pseudosection illustrating the stability of fields of mineral assemblage and the proposed P-T trajectory.
The peak mineral assemblage of g-bi-ksp-sill-liq-ilm-q exists in a broad P-T field of 6.3–9.4 kbar and 810°C–844°C (Figure 5B). In contrast, the post-peak mineral assemblage of g-pl-bi-ksp-sill-cd-liq-ilm-q occurs within the restricted P-T conditions of 775°C–812°C and 5.2–6.3 kbar (Figure 5B). The development of sericitized cordierite encasing the garnet primarily indicates a transition from peak to post-peak stage, while the crystallization of plagioclase from melt marks the onset of the retrograde cooling phase (Figure 5B).
5.2.2 Sample 23QL11-1
For Sample 23QL11-1, an H2O concentration of 1.42 mol%, representing the median position of the solidus within post-peak mineral assemblages, was adopted for subsequent thermodynamic simulations (Figure 6A). Based on the restricted H2O content, phase equilibrium modelling was conducted across a P-T space spanning 700°C–900°C and 4–14 kbar, considering an excess of quartz (Figure 6B). The solidus exhibits a sharp slope, confined to a temperature window of approximately 778°C–845°C. The biotite-out curve appears above ∼830°C. The rutile-in and cordierite-in curves both exhibit a positive correlation with pressure, with rutile-in occurring at pressures above ∼10.5 kbar and cordierite-in at pressures lower than ∼6.4 kbar.
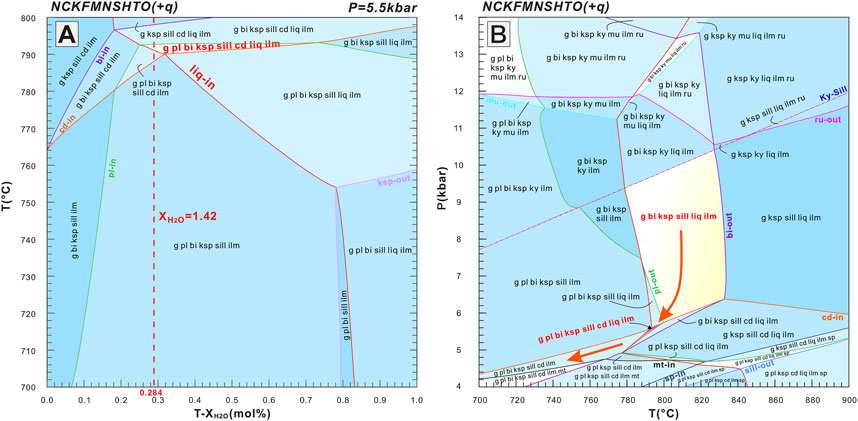
Figure 6. Phase equilibrium modelling results for 23QL11-1. (A) T-XH2O diagram modeled at 5.5 kbar, where the red line represents the chosen H2O content for subsequent P-T pseudosection. (B) P-T pseudosection showing mineral assemblage stability fields and the proposed P-T trajectory.
The peak metamorphic g-bi-ksp-sill-liq-ilm-q assemblage spans a broad P-T range of approximately 5.8–10.4 kbar and 780°C–832°C (Figure 6B). In contrast, the post-peak mineral assemblage of g-pl-bi-ksp-sill-cd-liq-ilm-q is well constrained within a much narrower P-T field, specifically 778°C–800°C and 4.9–5.8 kbar (Figure 6B). Similarly, the development of cordierite coronas or symplectitic assemblages comprising sillimanite and cordierite primarily signifies the shift from peak metamorphic conditions to the post-peak stage, with continued cooling transitioning into the retrograde cooling phase (Figure 6B).
5.2.3 Sample 23QL16-1
For Sample 23QL16-1, an H2O concentration of 2.47 mol% was determined and used in the subsequent thermodynamic modelling (Figure 7A). Building on the determined H2O content, phase equilibrium modelling was executed across 700°C–900°C and 4–14 kbar, assuming excess quartz (Figure 7B). The biotite-out curve appears above ∼878°C. The rutile-in and cordierite-in curves both exhibit a positive correlation with pressure, occurring at pressures above ∼9.8 kbar and below ∼7.1 kbar, respectively.
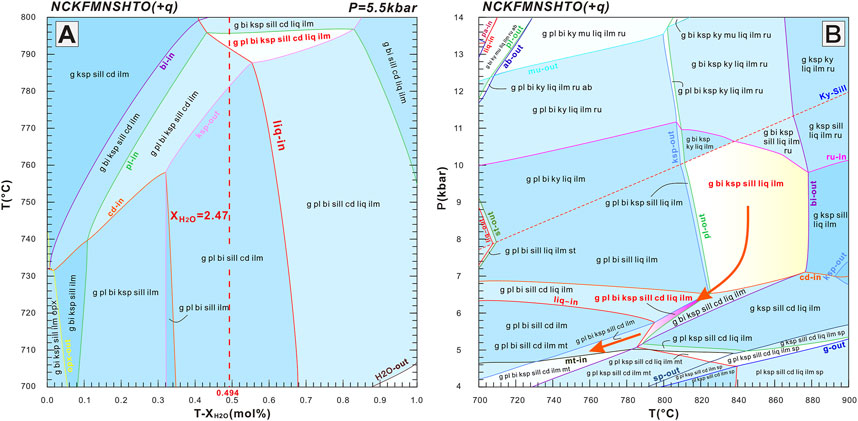
Figure 7. Phase equilibrium modelling results for 23QL16-1. (A) T-XH2O diagram computed at 5.5 kbar, with the red line illustrating the H2O content used for subsequent P-T pseudosection. (B) P-T pseudosection depicting mineral assemblage stability fields and the proposed P-T evolution path.
The g-bi-ksp-sill-liq-ilm assemblage at peak metamorphism remains stable over a broad pressure–temperature interval, ranging from 6.6 to 10.6 kbar and 812°C–878°C (Figure 7B). In contrast, the post-peak mineral assemblage of g-pl-bi-ksp-sill-cd-liq-ilm-q is well constrained within a much narrower P-T field, specifically 785°C–825°C and 5.1–6.5 kbar (Figure 7B). The emergence of textural features dominated by cordierite, or symplectitic intergrowths comprised of sillimanite and cordierite delineates the transition from peak pressure metamorphism to post-peak decompression, while sustained cooling initiates the retrograde regime (Figure 7B).
5.3 Geochronology
5.3.1 U-Pb geochronology of zircon
Zircon crystals obtained from Sample 23QL08-1 exhibit either sub-rounded or stubby morphologies, typically measuring between 50 and 150 μm in size. Most of the grains in CL imaging exhibit well-defined core-rim textures (Figure 8A). The cores, displaying distinct bright oscillatory zoning, are considered relics of detrital sources, while the structureless overgrowth rims and the individual grains with chaotic zoning are regarded as metamorphic in origin. The zircon core analysis yielded nine datasets and two spots (29 and 24), which delivered concordant results showing 207Pb/206Pb ages at 2011 ± 89 Ma and 2021 ± 45 Ma (Figure 9A). High Th/U ratios (0.53–0.54) observed in these analyses indicate an igneous origin for zircon (Supplementary Table S1; Figure 9A). These results indicate the crystallization age of MP pelitic granulite protoliths. Additionally, the upper intercept age analysis based on twenty-one analytical measurements targeting zircon rims and discrete metamorphic zircons yielded a result of 1943 ± 18 Ma (n = 21; MSWD = 2.6; Figure 9A), and Th/U ratios were 0.00–0.23 (Supplementary Table S1). The geochronological results are considered to represent the metamorphic age of the MP pelitic granulites.
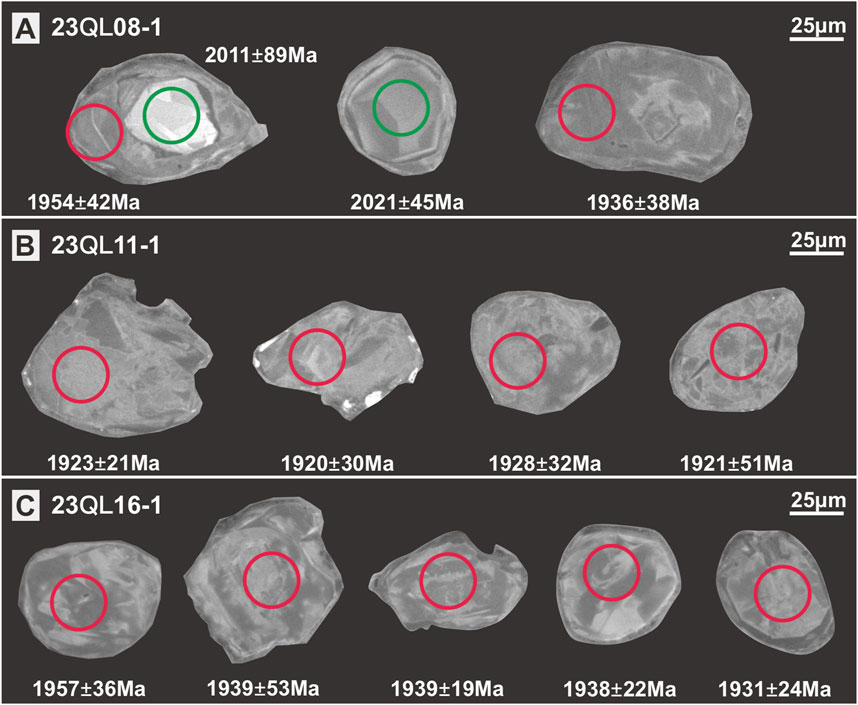
Figure 8. CL images of representative zircon grains from (A) 23QL08-1, (B) 23QL11-1, and (C) 23QL16-1. Green and red circles indicate analytical spots at core and rim positions, respectively, with their corresponding 207Pb/206Pb ages labeled.
Zircon crystals from Sample 23QL11-1 exhibit dimensions predominantly between 40 and 100 μm, with most grains showing irregular to moderately rounded shapes (Figure 8B). CL analysis reveals that a significant proportion of these grains contain weakly sector-zoned or chaotic internal textures (Figure 8B), consistent with characteristics of metamorphic zircons. Twenty-four available analyses were performed on these metamorphic zircons, with two excluded attributed to variable Pb loss (Supplementary Table S1; Figure 9B). The remaining data define an intercept age of 1931 ± 10 Ma (n = 22, MSWD = 1.1; Figure 9B), indicating the age of metamorphism.
Zircons isolated from Sample 23QL16-1 generally measure between 50 and 100 μm in maximum dimension and primarily exhibit equant to subrounded or angular grain shapes (Figure 8C). CL images of these zircons demonstrate that the majority display faintly sector-zoned or disordered internal textures (Figure 8C), aligning with features typically associated with metamorphic origin. Twenty analytical datasets were acquired from these metamorphic zircon grains, which exhibited characteristically low Th/U ratios of 0.00–0.11 (Supplementary Table S1). The analyzed data pointed to a metamorphic event that occurred at 1941 ± 10 Ma (n = 20, MSWD = 1.6; Figure 9C) based on an upper intercept age.
5.3.2 U-Pb geochronology of monazite
Monazite crystals extracted from Sample 23QL08-1 exhibit subrounded to equant morphologies, with diameters of 100–200 μm (Figure 10A). BSE imaging reveals their overall homogeneity, though some grains exhibit distinct core-rim structures (Figure 10A). The weighted mean 207Pb/206Pb age from nineteen out of twenty-four spot analyses targeting homogeneous domains and lower-luminescence rims came out to be 1931 ± 3 Ma (MSWD = 0.46, n = 19; Figure 11A). Two analyses of core domains produced concordant ages of 1960 ± 17 Ma and 1942 ± 10 Ma (Figure 11A), whereas three remaining core-focused analyses generated a weighted mean 207Pb/206Pb age of 1935 ± 6 Ma (MSWD = 0.32; n = 3; Figure 11A). Since the cores and rim ages fall within analytical uncertainty, the combined data yielded a final weighted mean 207Pb/206Pb age of 1932 ± 3 Ma (MSWD = 0.66; n = 23; Figure 11B), which is taken to represent the monazite formation age and the corresponding metamorphic event. Chondrite-normalized REE patterns of monazite are characterized by strong LREE enrichment, a pronounced negative Eu anomaly (Eu/Eu* = 0.01–0.39), and depleted HREE contents, resulting in a right-inclined pattern (LaN/YbN = 327–10995; Figure 12A).
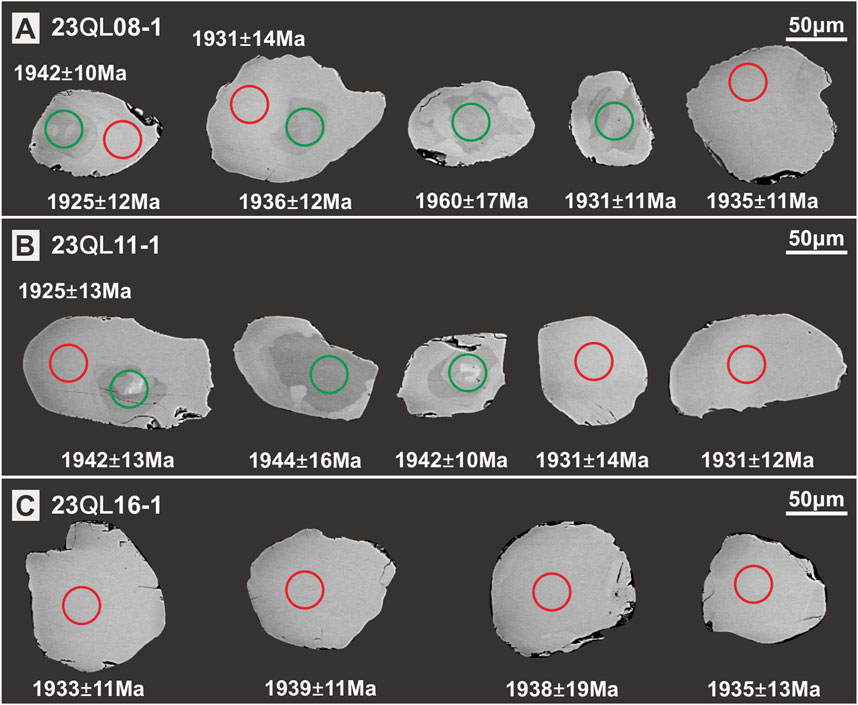
Figure 10. BSE images of representative monazite grains from (A) 23QL08-1, (B) 23QL11-1, and (C) 23QL16-1. Analytical spots at core and rim positions are marked by green and red circles, respectively, with associated 207Pb/206Pb ages labeled.
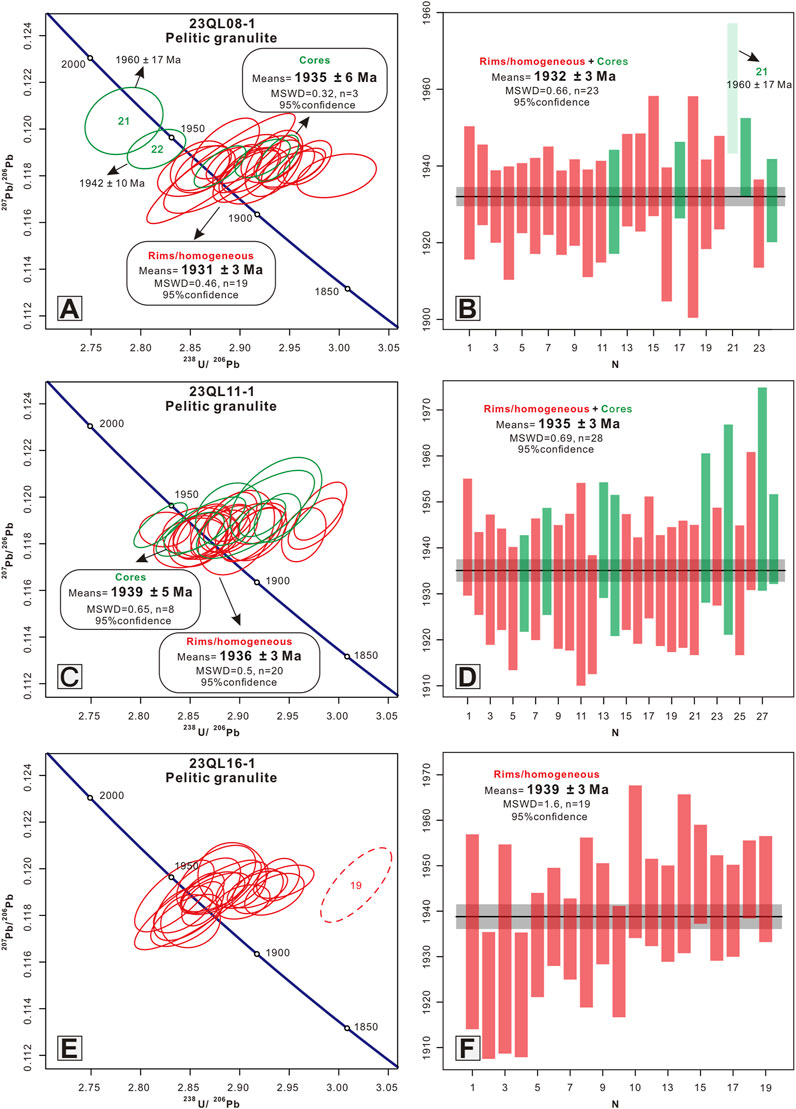
Figure 11. Monazite U-Pb Tera-Wasserburg diagrams and bar charts for (A, B) 23QL08-1, (C, D) 23QL11-1, and (E, F) 23QL16-1.
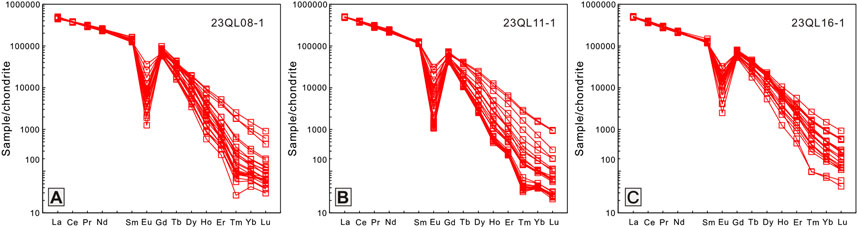
Figure 12. REE patterns of monazite from (A) 23QL08-1, (B) 23QL11-1, and (C) 23QL16-1, normalized to chondrite values. Chondrite reference values are taken from Sun and McDonough (1989).
Monazite crystals from Sample 23QL11-1 are subrounded and 100–200 μm in diameter (Figure 10B). BSE imaging reveals predominantly homogeneous textures, though some grains display concentric zonation indicative of core-rim structures (Figure 10B). The weighted mean 207Pb/206Pb age from twenty out of twenty-eight spot analyses of targeting homogeneous domains and low-luminescence rims came out to be 1936 ± 3 Ma (MSWD = 0.50, n = 20; Figure 11C). Eight core-focused analyses yielded a weighted mean 207Pb/206Pb age of 1939 ± 5 Ma (MSWD = 0.65; n = 8; Figure 11C). However, given the overlap within analytical uncertainty, no statistically significant distinction exists between core and rim ages. Consequently, a composite weighted mean 207Pb/206Pb age of 1935 ± 3 Ma (MSWD = 0.69; n = 28; Figure 11D) is considered representative of monazite crystallization during metamorphic events. Monazite from sample 23QL11-1 exhibits a right-inclined REE pattern (LaN/YbN = 316–12520), characterized by strong enrichment in LREE and significant depletion in HREE (Figure 12B), along with a pronounced negative Eu anomaly (Eu/Eu* = 0.02–0.35).
Monazite crystals from Sample 23QL16-1 are subrounded to equant, with diameters spanning 100–150 μm. Moreover, BSE images indicate that most grains exhibit a predominantly uniform texture (Figure 10C). A total of 20 monazite analyses were conducted, with one analysis (spot 19) displaying discordance, probably owing to Pb loss. Nineteen additional analyses yielded a weighted mean 207Pb/206Pb age of 1939 ± 3 Ma (MSWD = 1.6; n = 19; Figures 11E,F), which is considered to reflect the metamorphic event. Monazite from sample 23QL16-1 also shows a right-inclined REE pattern (LaN/YbN = 335–7,232) with strong LREE enrichment, HREE depletion, and a pronounced negative Eu anomaly (Eu/Eu* = 0.03–0.36; Figure 12C).
6 Discussion
6.1 Metamorphic P-T path
Through the integration of petrography, geochronology and phase equilibrium modelling, the MP pelitic granulites of the Qianlishan Complex underwent three different metamorphic stages. Without mineral chemistry data, the peak mineral assemblage of g-bi-ksp-sill-liq-ilm-q remains broadly defined, with its constraints primarily controlled by the pl-out line, ky-sill transformation line, ru-in line, and bi-out line. Following peak metamorphism, cordierite develops at the expense of garnet, while plagioclase crystallizes from the melt. The post-peak mineralogical assemblage is located in the very narrow field (g-pl-bi-ksp-sill-cd-liq-ilm-q), which defines well-constrained P-T conditions (775°C–825°C/4.9–6.5 kbar) of the-post-peak stage. All MP granulites experience metamorphic evolution that follows a single clockwise P-T trajectory, transitioning from peak pressure conditions through post-peak evolution to sub-solidus cooling. An initial phase of nearly isothermal decompression, succeeded by near-isobaric cooling, characterizes the P-T paths (Figures 5–7). Subsequently, we analyzed these P-T paths in relation to previously reported HP, MP, UHT pelitic/felsic granulites within the Qianlishan-Helanshan Complexes of the western Khondalite Belt (Figure 13), which share similar clockwise P-T trajectories but differ in their peak pressure conditions (Zhou et al., 2010; Yin et al., 2014; Yin et al., 2015; Xu et al., 2018; Gou et al., 2018; Wu et al., 2020; Wu et al., 2024). Furthermore, our results are comparable to those documented for MP pelitic granulites in the central segment (Daqingshan-Wulashan Complex; Cai et al., 2014; Cai et al., 2017) and the eastern segment (Jining Complex; Jiao et al., 2013) of the Khondalite Belt. This suggests that they may have undergone the same coherent orogenic event (Spear, 1993), despite being buried at different depths.
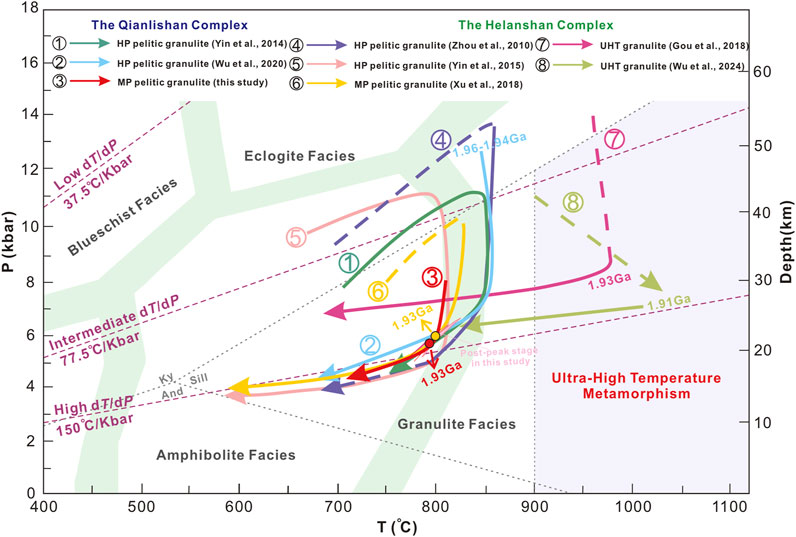
Figure 13. Comparative P-T trajectories for HP, MP, and UHT pelitic granulites within the Qianlishan-Helanshan Complex, located in the western Khondalite Belt. Metamorphic facies boundaries follow the classification by Brown and Johnson (2018).
6.2 Age interpretation and tectonic significance
U-Pb dating via LA-ICP-MS on three MP pelitic granulites produced zircon upper intercept ages of 1943 ± 18 Ma, 1931 ± 10 Ma, and 1941 ± 10 Ma (Figure 9), while monazite from the same samples yielded weighted mean U-Pb ages of 1932 ± 3 Ma, 1935 ± 3 Ma, and 1939 ± 3 Ma (Figure 11). It seems that zircon ages appear to be slightly older than monazite ages within the same rock (Figures 9, 11), likely due to differences in the temperatures at which zircon and monazite grow (Kelsey et al., 2008). However, both age populations converge on a metamorphic timing of ∼1.93 Ga within the uncertainty. The obtained age is slightly younger than the metamorphic zircon ages (1.96–1.94 Ga) identified in HP pelitic and felsic granulites (Yin et al., 2009; Qiao et al., 2016; Wu et al., 2020), which were linked to the HP metamorphic phase. The ∼1.93 Ga age also corresponds with a ∼1.92 Ga metamorphic population in g-sill-bi-pl gneiss from the Qianlishan Complex (Yin et al., 2009), as well as similar ages in MP granulites from the Helanshan Complex (Xu et al., 2018).
The ∼1.93 Ga age identified in MP granulites from the Helanshan Complex has been interpreted as marking the onset of nearly isobaric cooling (Xu et al., 2018). Experimental petrology and phase modelling support this interpretation, which shows that zircon dissolves during heating but crystallizes during melt solidification in the cooling phase (Kelsey and Powell, 2011; Zhang et al., 2013; Lu et al., 2022). Monazite exhibits a similar behavior (Yakymchuk and Brown, 2014). Monazite grains from three MP pelitic granulites all exhibit significant negative Eu anomalies (Figure 12), likely reflecting enhanced feldspar crystallization at the retrograde cooling phase (Wang et al., 2017; Lu et al., 2022). Pseudosection analysis indicates that as metamorphism progresses from peak pressure conditions toward the post-peak and cooling phases, melt crystallization becomes dominant (Figure 14), accompanied by the initiation of plagioclase formation (Figures 5–7). Based on this interpretation, the older monazite ages of 1960 ± 17 Ma and 1942 ± 10 Ma (Figures 11A,B) may have been preserved as inclusions within large, intact minerals, preventing it from dissolution (Baldwin et al., 2006; Jiao et al., 2020) and potentially representing the peak metamorphic age. However, the majority of monazite grains, with ages clustered around 1.93 Ga, may correspond to the initiation of the cooling phase or retrograde metamorphic processes following granulite-facies conditions in the Qianlishan Complex.
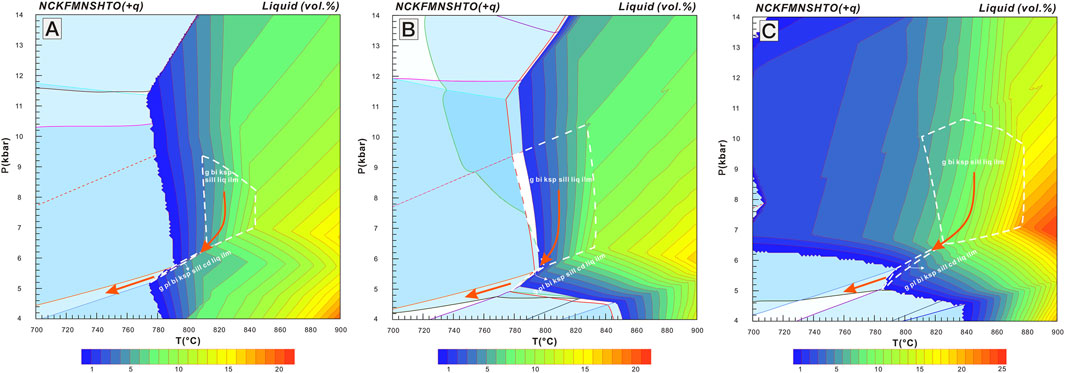
Figure 14. Melt mole (%) isopleths computed using GeoPs, overlaid on the constructed P–T pseudosection for (A) 23QL08-1, (B) 23QL11-1, and (C) 23QL16-1.
Integrating prior geochronological data and P-T trajectory results (Figure 13), we can deduce that the peak-pressure metamorphism of pelitic granulites in the Qianlishan Complex happened between 1.96 and 1.94 Ga before experiencing decompression and retrograde cooling around 1.93 Ga. This further supports that the Khondalite Belt underwent a continent-continent collision around ca. 1.95 Ga (Yin et al., 2009; Yin et al., 2011), which was shortly followed by a shallow slab-breakoff event (e.g., Gou et al., 2019; Yin et al., 2023). This process triggered the uplift of pelitic granulites in the western Khondalite belt to mid-crustal levels, culminating in their subsequent cooling around 1.93 Ga (Xu et al., 2018).
7 Conclusion
(1) The Qianlishan MP pelitic granulites experienced metamorphic processes along a clockwise P-T trajectory, starting from near-isothermal decompression and transitioning into near-isobaric cooling.
(2) U-Pb geochronology applied to zircon and monazite yielded a metamorphic age of ∼1.93 Ga, suggesting the initiation of the retrograde cooling phase.
(3) The peak-pressure metamorphism of pelitic granulites in the Qianlishan Complex ouccured at ∼1.95 Ga, followed by decompression and retrograde cooling around 1.93 Ga.
Data availability statement
The original contributions presented in the study are included in the article/Supplementary Material, further inquiries can be directed to the corresponding authors.
Author contributions
SW: Writing – review and editing, Investigation, Funding acquisition, Conceptualization, Supervision, Writing – original draft, Validation, Data curation, Visualization, Methodology. YS: Methodology, Writing – review and editing, Validation, Data curation, Formal Analysis. QW: Formal Analysis, Writing – review and editing, Data curation, Validation, Methodology. HQ: Funding acquisition, Writing – review and editing, Investigation, Validation, Supervision, Data curation, Methodology, Visualization.
Funding
The author(s) declare that financial support was received for the research and/or publication of this article. The National Natural Science Foundation of China (Grant No. 42302222), the Hunan Provincial Natural Science Foundation (Grant No. 2024JJ6102), the Scientific Research Fund of Hunan Provincial Education Department (Grant No. 22A0499), Science & Technology Department of Sichuan Province (Grant No. 2024ZYD0085), and the research support program of Hengyang Normal University (Grants No. 2022QD13, 2022QD10) granted financial support for conducting this research.
Acknowledgments
We thank the Editor and the two reviewers for their careful reviews and valuable comments.
Conflict of interest
The authors declare that the research was conducted in the absence of any commercial or financial relationships that could be construed as a potential conflict of interest.
Generative AI statement
The authors declare that no Generative AI was used in the creation of this manuscript.
Publisher’s note
All claims expressed in this article are solely those of the authors and do not necessarily represent those of their affiliated organizations, or those of the publisher, the editors and the reviewers. Any product that may be evaluated in this article, or claim that may be made by its manufacturer, is not guaranteed or endorsed by the publisher.
Supplementary material
The Supplementary Material for this article can be found online at: https://www.frontiersin.org/articles/10.3389/feart.2025.1602565/full#supplementary-material
References
Aleinikoff, J. N., Schenck, W. S., Plank, M. O., Srogi, L., Fanning, C. M., Kamo, S. L., et al. (2006). Deciphering igneous and metamorphic events in high-grade rocks of the Wilmington complex, Delaware: morphology, cathodoluminescence and backscattered electron zoning, and SHRIMP U-Pb geochronology of zircon and monazite. Geol. Soc. Am. Bull. 118 (1–2), 39–64. doi:10.1130/b25659.1
Baldwin, J. A., Bowring, S. A., Williams, M. L., and Mahan, K. H. (2006). Geochronological constraints on the evolution of high-pressure felsic granulites from an integrated electron microprobe and ID-TIMS geochemical study. Lithos 88, 173–200. doi:10.1016/j.lithos.2005.08.009
Brown, M., and Johnson, T. (2018). Secular change in metamorphism and the onset of global plate tectonics. Am. Mineralogist 103, 181–196. doi:10.2138/am-2018-6166
Cai, J., Liu, F. L., Liu, P. H., Liu, C. H., Wang, F., and Shi, J. R. (2014). Metamorphic P–T path and tectonic implications of pelitic granulites from the Daqingshan complex of the Khondalite belt, North China craton. Precambrian Res. 241, 161–184. doi:10.1016/j.precamres.2013.11.012
Cai, J., Liu, F. L., Liu, P. H., Wang, F., Liu, C. H., and Shi, J. R. (2017). Anatectic record and P–T path evolution of metapelites from the Wulashan complex, Khondalite belt, North China craton. Precambrian Res. 303, 10–29. doi:10.1016/j.precamres.2016.09.007
Catlos, E. J., Gilley, L. D., and Harrison, T. M. (2002). Interpretation of monazite ages obtained via in situ analysis. Chem. Geol. 188, 193–215. doi:10.1016/s0009-2541(02)00099-2
Cherniak, D. J., and Watson, E. B. (2001). Pb diffusion in zircon. Chem. Geol. 172, 5–24. doi:10.1016/s0009-2541(00)00233-3
Cherniak, D. J., Watson, E. B., Grove, M., and Harrison, T. M. (2004). Pb diffusion in monazite: a combined RBS/SIMS study. Geochimica Cosmochimica Acta 68, 829–840. doi:10.1016/j.gca.2003.07.012
Chu, G. B., Chen, H. Y., Falloon, T. J., Han, J. S., Zhang, S. T., Cheng, J. M., et al. (2020). Early Cretaceous mantle upwelling and melting of juvenile lower crust in the Middle-Lower Yangtze River Metallogenic Belt: example from Tongshankou Cu-(Mo-W) ore deposit. Gondwana Res. 83, 183–200. doi:10.1016/j.gr.2020.02.004
Condie, K. C., Boryta, M. D., Liu, J. Z., and Qian, X. L. (1992). The origin of khondalites: geochemical evidence from the Archean to Early Proterozoic granulite belt in the North China craton. Precambrian Res. 59, 207–223. doi:10.1016/0301-9268(92)90057-u
Dan, W., Li, X. H., Guo, J. H., Liu, Y., and Wang, X. C. (2012). Integrated in situ zircon U-Pb age and Hf–O isotopes for the Helanshan khondalites in North China Craton: juvenile crustal materials deposited in active or passive continental margin? Precambrian Res. 222–223, 143–158. doi:10.1016/j.precamres.2011.07.016
DeWolf, C. P., Belshaw, N., and O’Nions, R. K. (1993). A metamorphic history from micron-scale 207Pb/206Pb chronometry of Archean monazite. Earth Planet. Sci. Lett. 120, 207–220. doi:10.1016/0012-821x(93)90240-a
Gibson, H. D., Carr, S. D., Brown, R. L., and Hamilton, M. A. (2004). Correlations between chemical and age domains in monazite, and metamorphic reactions involving major pelitic phases: an integration of ID-TIMS and SHRIMP geochronology with Y–Th–U X-ray mapping. Chem. Geol. 211, 237–260. doi:10.1016/j.chemgeo.2004.06.028
Gou, L. L., Li, Z. H., Liu, X. M., Dong, Y. P., Zhao, J., Zhang, C. L., et al. (2018). Ultrahigh-temperature metamorphism in the Helanshan complex of the Khondalite Belt, North China Craton: petrology and phase equilibria of spinel-bearing pelitic granulites. J. Metamorph. Geol. 36, 1199–1220. doi:10.1111/jmg.12442
Gou, L. L., Zi, J.-W., Dong, Y. P., Liu, X. M., Li, Z. H., Xu, X. F., et al. (2019). Timing of two separate granulite-facies metamorphic events in the Helanshan complex, North China Craton: constraints from monazite and zircon U-Pb dating of pelitic granulites. Lithos 350–351, 105216. doi:10.1016/j.lithos.2019.105216
Guo, J. H., Peng, P., Chen, Y., Jiao, S. J., and Windley, B. F. (2012). UHT sapphirine granulite metamorphism at 1.93–1.92Ga caused by gabbronorite intrusions: implications for tectonic evolution of the northern margin of the North China Craton. Precambrian Res. 222–223, 124–142. doi:10.1016/j.precamres.2011.07.020
Harley, S. L., Kelly, N. M., and Moller, A. (2007). Zircon behaviour and the thermal histories of mountain chains. Elements 3, 25–30. doi:10.2113/gselements.3.1.25
He, X. L., Yin, C. Q., Long, X. P., Qian, J. H., Wang, L. J., and Qiao, H. Z. (2017). Archean to Paleoproterozoic continental crust growth in the Western Block of North China: constraints from zircon Hf isotopic and whole-rock Nd isotopic data. Precambrian Res. 303, 105–116. doi:10.1016/j.precamres.2017.02.018
Holland, T., and Powell, R. (2003). Activity–composition relations for phases in petrological calculations: an asymmetric multicomponent formulation. Contributions Mineralogy Petrology 145, 492–501. doi:10.1007/s00410-003-0464-z
Holland, T. J. B., and Powell, R. (2011). An improved and extended internally consistent thermodynamic dataset for phases of petrological interest, involving a new equation of state for solids. J. Metamorph. Geol. 29 (3), 333–383. doi:10.1111/j.1525-1314.2010.00923.x
Hu, Z. C., Li, X. H., Luo, T., Zhang, W., Crowley, J., Li, Q. L., et al. (2021). Tanz zircon megacrysts: a new zircon reference material for the microbeam determination of U–Pb ages and Zr–O isotopes. J. Anal. Atomic Spectrom. 36 (12), 2715–2734. doi:10.1039/d1ja00311a
Jiao, S. J., Fitzsimons, I. C. W., and Guo, J. H. (2017). Paleoproterozoic UHT metamorphism in the Daqingshan Terrane, North China Craton: new constraints from phase equilibria modeling and SIMS U-Pb zircon dating. Precambrian Res. 303, 208–227. doi:10.1016/j.precamres.2017.03.024
Jiao, S. J., Fitzsimons, I. C. W., Zi, J. W., Evans, N. J., Mcdonald, B. J., and Guo, J. H. (2020). Texturally controlled U–Th–Pb monazite geochronology reveals paleoproterozoic UHT metamorphic evolution in the Khondalite belt, north China craton. J. Petrology 61, egaa023. doi:10.1093/petrology/egaa023
Jiao, S. J., and Guo, J. H. (2011). Application of the two-feldspar geothermometer to ultrahigh-temperature (UHT) rocks in the Khondalite belt, North China craton and its implications. Am. Mineralogist 96, 250–260. doi:10.2138/am.2011.3500
Jiao, S. J., Guo, J. H., Harley, S. L., and Windley, B. F. (2013). New constraints from garnetite on the P-T path of the Khondalite belt: implications for the tectonic evolution of the North China craton. J. Petrology 54, 1725–1758. doi:10.1093/petrology/egt029
Jiao, S. J., Guo, J. H., Mao, Q., and Zhao, R. F. (2011). Application of Zr-in-rutile thermometry: a case study from ultrahigh-temperature granulites of the Khondalite belt, North China Craton. Contributions Mineralogy Petrology 162, 379–393. doi:10.1007/s00410-010-0602-3
Jochum, K. P., Weis, U., Stoll, B., Kuzmin, D., Yang, Q., Raczek, I., et al. (2011). Determination of reference values for NIST SRM 610–617 glasses following ISO guidelines. Geostand. Geoanalytical Res. 35 (4), 397–429. doi:10.1111/j.1751-908x.2011.00120.x
Johnson, T. E., Clark, C., Taylor, R. J. M., Santosh, M., and Collins, A. S. (2015). Prograde and retrograde growth of monazite in migmatites: an example from the Nagercoil Block, southern India. Geosci. Front. 6, 373–387. doi:10.1016/j.gsf.2014.12.003
Kelsey, D. E., Clark, C., and Hand, M. (2008). Thermobarometric modelling of zircon and monazite growth in melt-bearing systems: examples using model metapelitic and metapsammitic granulites. J. Metamorph. Geol. 26, 199–212. doi:10.1111/j.1525-1314.2007.00757.x
Kelsey, D. E., and Powell, R. (2011). Progress in linking accessory mineral growth and breakdown to major mineral evolution in metamorphic rocks: a thermodynamic approach in the Na2 O-CaO-K2O-FeO-MgO-Al2O3-SiO2-H2O-TiO2-ZrO2 system. J. Metamorph. Geol. 29, 151–166. doi:10.1111/j.1525-1314.2010.00910.x
Kohn, M. J., and Malloy, M. A. (2004). Formation of monazite via prograde metamorphic reactions among common silicates: implications for age determinations. Geochimica Cosmochimica Acta 68, 101–113. doi:10.1016/s0016-7037(03)00258-8
Korhonen, F. J., Brown, M., Clark, C., and Bhattacharya, S. (2013). Osumilite–melt interactions in ultrahigh temperature granulites: phase equilibria modelling and implications for the P-T–t evolution of the Eastern Ghats Province, India. J. Metamorpic Geol. 31 (8), 881–907. doi:10.1111/jmg.12049
Li, W. X., Yin, C. Q., Lin, S. F., Li, W. J., Gao, P., Zhang, J., et al. (2022). Paleoproterozoic tectonic evolution from subduction to collision of the Khondalite belt in north China: evidence from multiple magmatism in the Qianlishan complex. Precambrian Res. 368, 106471. doi:10.1016/j.precamres.2021.106471
Li, X. W., and Wei, C. J. (2016). Phase equilibria modelling and zircon age dating of pelitic granulites in Zhaojiayao, from the Jining Group of the Khondalite belt, north China craton. J. Metamorph. Geol. 34, 595–615. doi:10.1111/jmg.12195
Li, X. W., and Wei, C. J. (2018). Ultrahigh-temperature metamorphism in the Tuguiwula area, Khondalite belt, north China craton. J. Metamorph. Geol. 36, 489–509. doi:10.1111/jmg.12301
Li, X. W., White, R. W., and Wei, C. J. (2019). Can we extract ultrahigh-temperature conditions from Fe-rich metapelites? An example from the Khondalite Belt, North China Craton. Lithos 328–329, 228–243. doi:10.1016/j.lithos.2019.01.032
Liu, P. H., Liu, F. L., Cai, J., Liu, C. H., Liu, J. H., Wang, F., et al. (2017). Spatial distribution, P-T-t paths, and tectonic significance of high-pressure mafic granulites from the Daqingshan-Wulashan Complex in the Khondalite Belt, North China Craton. Precambrian Res. 303, 687–708. doi:10.1016/j.precamres.2017.09.004
Liu, P. H., Liu, F. L., Liu, C. H., Liu, J., Wang, F., Xiao, L. L., et al. (2014). Multiple mafic magmatic and high-grade metamorphic events revealed by zircons from meta-mafic rocks in the Daqingshan–Wulashan Complex of the Khondalite Belt, North China Craton. Precambrian Res. 246, 334–357. doi:10.1016/j.precamres.2014.02.015
Liu, S. J., Tsunogae, T., Li, W. S., Shimizu, H., Santosh, M., Wan, Y. S., et al. (2012). Paleoproterozoic granulites from Heling’er: implications for regional ultrahigh-temperature metamorphism in the North China Craton. Lithos 148, 54–70. doi:10.1016/j.lithos.2012.05.024
Lu, C. S., Qian, J. H., Yin, C. Q., Gao, P., Guo, M. J., and Zhang, W. F. (2022). Ultrahigh temperature metamorphism recorded in the Lüliang Complex, Trans-North China Orogen: P–T–t evolution and heating mechanism. Precambrian Res. 383, 106900. doi:10.1016/j.precamres.2022.106900
Lu, L., Jin, S., Xu, X., and Liu, F. L. (1996). Early Precambrian Khondalites in North China. Changchun: Changchun Publishing House, 1–277.
Mahan, K. H., Goncalves, P., Williams, M. L., and Jercinovic, M. J. (2006). Dating metamorphic reactions and fluid flow: application to exhumation of high- P granulites in a crustal-scale shear zone, western Canadian Shield. J. Metamorph. Geol. 24, 193–217. doi:10.1111/j.1525-1314.2006.00633.x
Parrish, R. R. (1990). U-Pb dating of monazite and its application to geological problems. Can. J. Earth Sci. 27, 1431–1450. doi:10.1139/e90-152
Peng, P., Guo, J. H., Windley, B. F., Liu, F., Chu, Z., and Zhai, M. G. (2012). Petrogenesis of late paleoproterozoic Liangcheng charnockites and S-type granites in the central-northern margin of the North China craton: implications for ridge subduction. Precambrian Res. 222–223, 107–123. doi:10.1016/j.precamres.2011.06.002
Peng, P., Guo, J. H., Zhai, M. G., and Bleeker, W. (2010). Paleoproterozoic gabbronoritic and granitic magmatism in the northern margin of the North China craton: evidence of crust–mantle interaction. Precambrian Res. 183, 635–659. doi:10.1016/j.precamres.2010.08.015
Qi, Y., Jiao, S. J., Chen, L., Liu, J. H., Liu, Y., and Guo, J. H. (2024). Heating–cooling–heating cycles within ca. 70 Myr recorded in UHT granulites in the Khondalite Belt, North China Craton. Precambrian Res. 411, 107508. doi:10.1016/j.precamres.2024.107508
Qiao, H. Z. (2021). Detrital zircon U–Pb ages of the Huangqikou formation of the Changcheng system in the Qianlishan area, western north China craton and their geological implications. Acta Geol. Sichuan 41, 33–39.
Qiao, H. Z., Deng, P. P., and Li, J. W. (2023). Geochronological constraints on the origin of the paleoproterozoic Qianlishan gneiss domes in the Khondalite belt of the North China craton and their tectonic implications. Minerals 13 (11), 1361. doi:10.3390/min13111361
Qiao, H. Z., Liu, M., and Dai, C. (2024). Timing and tectonic implications of the development of the orosirian Qianlishan ductile shear zones in the Khondalite belt, north China craton. Minerals 14, 561. doi:10.3390/min14060561
Qiao, H. Z., Yin, C. Q., Li, Q. L., He, X. L., Qian, J. H., and Li, W. J. (2016). Application of the revised Ti-in-zircon thermometer and SIMS zircon U-Pb dating of high-pressure pelitic granulites from the Qianlishan-Helanshan Complex of the Khondalite Belt, North China Craton. Precambrian Res. 276, 1–13. doi:10.1016/j.precamres.2016.01.020
Reed, W. P. (1992). Certificate of analysis: standard reference materials 610 and 611. Gaithersburg, MD: National Institute of Standards and Technology.
Rubatto, D., Chakraborty, S., and Dasgupta, S. (2013). Timescales of crustal melting in the Higher Himalayan Crystallines (Sikkim, Eastern Himalaya) inferred from trace element-constrained monazite and zircon chronology. Contributions Mineralogy Petrology 165, 349–372. doi:10.1007/s00410-012-0812-y
Rubatto, D., Williams, I. S., and Buick, I. S. (2001). Zircon and monazite response to prograde metamorphism in the Reynolds Range, central Australia. Contributions Mineralogy Petrology 140, 458–468. doi:10.1007/pl00007673
Santosh, M., Liu, S. J., Tsunogae, T., and Li, J. H. (2012). Paleoproterozoic ultrahigh-temperature granulites in the North China Craton: implications for tectonic models on extreme crustal metamorphism. Precambrian Res. 222–223, 77–106. doi:10.1016/j.precamres.2011.05.003
Santosh, M., Wilde, S. A., and Li, J. H. (2007). Timing of Paleoproterozoic ultrahigh-temperature metamorphism in the North China craton: evidence from SHRIMP U-Pb zircon geochronology. Precambrian Res. 159, 178–196. doi:10.1016/j.precamres.2007.06.006
Seydoux-Guillaume, A.-M., Paquette, J.-L., Wiedenbeck, M., Montel, J.-M., and Heinrich, W. (2002). Experimental resetting of the U–Th–Pb systems in monazite. Chem. Geol. 191, 165–181. doi:10.1016/s0009-2541(02)00155-9
Sláma, J., Košler, J., Condon, D. J., Crowley, J. L., Gerdes, A., Hanchar, J. M., et al. (2008). Plešovice zircon—a new natural reference material for U–Pb and Hf isotopic microanalysis. Chem. Geol. 249 (1-2), 1–35. doi:10.1016/j.chemgeo.2007.11.005
Spear, F. S. (1993). Metamorphic phase equilibria and pressure–temperature–time paths. Washington, DC: Mineralogical Society of America, 799.
Sun, S. S., and McDonough, W. F. (1989). Chemical and isotopic systematics of oceanic basalts: implications for mantle composition and processes. Geol. Soc. Lond. Spec. Publ. 42 (1), 313–345. doi:10.1144/gsl.sp.1989.042.01.19
Vermeesch, P. (2018). IsoplotR: a free and open toolbox for geochronology. Geosci. Front. 9, 1479–1493. doi:10.1016/j.gsf.2018.04.001
Wang, J. M., Wu, F. Y., Rubatto, D., Liu, S. R., Zhang, J. J., Liu, X. C., et al. (2017). Monazite behaviour during isothermal decompression in pelitic granulites: a case study from Dinggye, Tibetan Himalaya. Contributions Mineralogy Petrology 172 (10), 81–111. doi:10.1007/s00410-017-1400-y
Wang, L. J., Guo, J. H., Yin, C. Q., Peng, P., Zhang, J., Spencer, C. J., et al. (2018). High-temperature S-type granitoids (charnockites) in the Jining complex, North China Craton: restite entrainment and hybridization with mafic magma. Lithos 320–321, 435–453. doi:10.1016/j.lithos.2018.09.035
White, R. W., Powell, R., and Clarke, G. L. (2002). The interpretation of reaction textures in Fe-rich metapelitic granulites of the Musgrave Block, Central Australia: constraints from mineral equilibria calculations in the system K2O-FeO-MgO-Al2O3-SiO2-H2O-TiO2-Fe2O3: Reaction textures, Musgrave block granulites. J. Metamorph. 20, 41–55. doi:10.1046/j.0263-4929.2001.00349.x
White, R. W., Powell, R., Holland, T. J. B., Johnson, T. E., and Green, E. C. R. (2014). New mineral activity–composition relations for thermodynamic calculations in metapelitic systems. J. Metamorph. Geol. 32 (3), 261–286. doi:10.1111/jmg.12071
Wiedenbeck, M., Alle, P., Corfu, F. Y., Griffin, W., Meier, M., Oberli, F., et al. (1995). Three natural zircon standards for U-Th-Pb, Lu-Hf, trace element and REE analyses. Geostand. Newsl. 19 (1), 1–23. doi:10.1111/j.1751-908x.1995.tb00147.x
Wu, S. J., Yin, C. Q., Davis, D. W., Zhang, J., Qian, J. H., Qiao, H. Z., et al. (2020). Metamorphic evolution of high-pressure felsic and pelitic granulites from the Qianlishan complex and tectonic implications for the Khondalite Belt, North China Craton. Geol. Soc. Am. Bull. 132, 2253–2266. doi:10.1130/b35502.1
Wu, S. J., Yin, C. Q., Qian, J. H., Qiao, H. Z., Wang, X. M., Xia, Y. F., et al. (2024). Paleoproterozoic ultrahigh-temperature metamorphism in the Helanshan Complex, North China Craton: new constraints from chloritized sapphirine-bearing pelitic granulites. Lithos 488–489, 107814. doi:10.1016/j.lithos.2024.107814
Xiang, H., and Connolly, J. A. D. (2022). GeoPS: an interactive visual computing tool for thermodynamic modelling of phase equilibria. J. Metamorph. Geol. 40, 243–255. doi:10.1111/jmg.12626
Xu, X. F., Gou, L. L., Long, X. P., Dong, Y. P., Liu, X. M., Zi, J.-W., et al. (2018). Phase equilibrium modelling and SHRIMP zircon U-Pb dating of medium-pressure pelitic granulites in the Helanshan complex of the Khondalite Belt, North China Craton, and their tectonic implications. Precambrian Res. 314, 62–75. doi:10.1016/j.precamres.2018.05.030
Yakymchuk, C., and Brown, M. (2014). Behaviour of zircon and monazite during crustal melting. J. Geol. Soc. 171, 465–479. doi:10.1144/jgs2013-115
Yang, Q.-Y., Santosh, M., and Tsunogae, T. (2014). Ultrahigh-temperature metamorphism under isobaric heating: new evidence from the North China Craton. J. Asian Earth Sci. 95, 2–16. doi:10.1016/j.jseaes.2014.01.018
Yin, C. Q., Qiao, H. Z., Lin, S. F., Li, C. C., Zhang, J., Qian, J. H., et al. (2020). Deformation history of the Qianlishan complex, Khondalite belt, north China: structures, ages and tectonic implications. J. Struct. Geol. 141, 104176. doi:10.1016/j.jsg.2020.104176
Yin, C. Q., Zhao, G. C., Guo, J. H., Sun, M., Xia, X. P., Zhou, X. W., et al. (2011). U-Pb and Hf isotopic study of zircons of the Helanshan complex: constrains on the evolution of the Khondalite belt in the western block of the North China craton. Lithos 122, 25–38. doi:10.1016/j.lithos.2010.11.010
Yin, C. Q., Zhao, G. C., and Sun, M. (2015). High-pressure pelitic granulites from the Helanshan complex in the Khondalite belt, North China craton: metamorphic P-t path and tectonic implications. Am. J. Sci. 315, 846–879. doi:10.2475/09.2015.03
Yin, C. Q., Zhao, G. C., Sun, M., Xia, X. P., Wei, C. J., Zhou, X. W., et al. (2009). LA-ICP-MS U-Pb zircon ages of the Qianlishan complex: constrains on the evolution of the Khondalite belt in the western block of the North China craton. Precambrian Res. 174, 78–94. doi:10.1016/j.precamres.2009.06.008
Yin, C. Q., Zhao, G. C., Wei, C. J., Sun, M., Guo, J. H., and Zhou, X. W. (2014). Metamorphism and partial melting of high-pressure pelitic granulites from the Qianlishan complex: constraints on the tectonic evolution of the Khondalite belt in the North China craton. Precambrian Res. 242, 172–186. doi:10.1016/j.precamres.2013.12.025
Yin, C. Q., Zhao, G. C., Xiao, W. J., Lin, S. F., Gao, R., Zhang, J., et al. (2023). Paleoproterozoic accretion and assembly of the Western Block of North China: a new model. Earth-Science Rev. 241, 104448. doi:10.1016/j.earscirev.2023.104448
Yuan, H. L., Gao, S., Liu, X. M., Li, H. M., Gunther, D., and Wu, F. Y. (2004). Accurate U-Pb age and trace element determinations of zircon by laser ablation-inductively coupled plasma-mass spectrometry. Geostand. Geoanalytical Res. 28, 353–370. doi:10.1111/j.1751-908x.2004.tb00755.x
Zhai, M. G. (2014). Multi-stage crustal growth and cratonization of the North China Craton. Geosci. Front. 5, 457–469. doi:10.1016/j.gsf.2014.01.003
Zhai, M. G. (2022). Khondalite revisited-record of special geological processes on Earth. Acta Petrol. Sin. 96, 2967–2997.
Zhang, C., Van Roermund, H., Zhang, L. F., and Spiers, C. (2012). A polyphase metamorphic evolution for the Xitieshan paragneiss of the north Qaidam UHP metamorphic belt, western China: in-situ EMP monazite- and U–Pb zircon SHRIMP dating. Lithos 136–139, 27–45. doi:10.1016/j.lithos.2011.07.024
Zhang, Y. H., Wei, C. J., Tian, W., and Zhou, X. W. (2013). Reinterpretation of metamorphic age of the Hengshan complex, North China craton. Chin. Sci. Bull. 58, 4300–4307. doi:10.1007/s11434-013-5993-x
Zhao, G. C., Cawood, P. A., Li, S. Z., Wilde, S. A., Sun, M., Zhang, J., et al. (2012). Amalgamation of the North China craton: key issues and discussion. Precambrian Res. 222–223, 55–76. doi:10.1016/j.precamres.2012.09.016
Zhao, G. C., Sun, M., Wilde, S. A., and Sanzhong, L. (2005). Late Archean to Paleoproterozoic evolution of the North China craton: key issues revisited. Precambrian Res. 136, 177–202. doi:10.1016/j.precamres.2004.10.002
Keywords: monazite-zircon U-Pb dating, phase equilibria modelling, pelitic granulite, Khondalite Belt, North China Craton
Citation: Wu S, Su Y, Wang Q and Qiao H (2025) Timing of retrograde metamorphism in medium-pressure pelitic granulites of the Qianlishan Complex: insights from monazite-zircon U-Pb geochronology and phase equilibria modelling. Front. Earth Sci. 13:1602565. doi: 10.3389/feart.2025.1602565
Received: 29 March 2025; Accepted: 22 April 2025;
Published: 06 May 2025.
Edited by:
Chen Zhao, Shenyang Center of China Geological Survey, ChinaCopyright © 2025 Wu, Su, Wang and Qiao. This is an open-access article distributed under the terms of the Creative Commons Attribution License (CC BY). The use, distribution or reproduction in other forums is permitted, provided the original author(s) and the copyright owner(s) are credited and that the original publication in this journal is cited, in accordance with accepted academic practice. No use, distribution or reproduction is permitted which does not comply with these terms.
*Correspondence: Shangjing Wu, d3VzaGoyNUBoeW51LmVkdS5jbg==; Hengzhong Qiao, cWlhb2h6aEBsc251LmVkdS5jbg==