- 1National Key Laboratory of Uranium Resource Exploration-Mining and Nuclear Remote Sensing, Beijing, China
- 2Beijing Research Institute of Uranium Geology, Beijing, China
- 3CNNC Key Laboratory of Uranium Resource Exploration and Evaluation Techniques, Beijing, China
The Xiangshan ore field, recognized as China’s premier volcanic-hosted uranium deposit cluster, has recently revealed significant exploration breakthroughs through the discovery of vertically zoned Pb-Zn-Ag-Cu polymetallic mineralization beneath shallow uranium systems, establishing a characteristic “upper uranium-lower polymetallic” vertical zoning configuration. This distinctive metallogenic architecture has propelled investigations into the genetic connections and superimposed mineralization mechanisms between deep polymetallic systems and surficial uranium enrichment, representing a pivotal frontier for advancing comprehensive evaluation strategies of uranium-polymetallic resources in volcanic terrains. Through systematic comparative analysis of H-O-S-Pb-Sr isotopic signatures across both mineralization types, this study elucidates: (1) Uranium mineralization exhibits significantly higher meteoric water contributions (δ18OH2O: 0.95‰–4.95‰) and crustal material inputs (87Sr/86Sr: 0.71447 to 0.72072; δ34S: 6.2‰–19.55‰) compared to polymetallic counterparts; (2) 206Pb/204Pb, 207Pb/204Pb, and 208Pb/204Pb ratios for sulfide minerals of polymetallic mineralization ranging from 17.599 to 18.262, 15.558 to 15.725, and 37.934 to 38.875 and sample points exhibit a linear distribution pattern imply unique sulfur enrichment processes potentially associated with uranium-rich hydrothermal systems; (3) Multivariate isotopic evidence confirms that uranium and polymetallic mineralization represent products of two discrete metallogenic events under the extensional tectonic regime of South China.
1 Introduction
The Xiangshan orefield, located in Fuzhou, Jiangxi province, is a typical representative of volcanic-type uranium deposit in China and has been investigated for about 60 years (Guo et al., 2020; Li and Zhang, 2016). Within a range of 300 square kilometers, over 30 uranium deposits/occurrences were discovered, and most of them were kept above the depth of 500 m. At long-term exploration and study, geologists have accumulated many sources about structural evolution (e.g., Li et al., 2014), magmatism (e.g., Guo et al., 2016), ore-forming fluid (e.g., Fan et al., 2003) and metallogenic age (e.g., Liu et al., 2015). In the recent decade, a lot of polymetallic mineralization under the uranium ore (700 m–2,000 m), discovered by deep drilling, has attached wide attention as a new focus of metallogenic research. So far, studies on polymetallic mineralization is just on the ascent, and several studies in Chinese refers to the mineralogy, metallogenic characteristics, fluid inclusion, and ore genesis (Guo et al., 2018; Wang et al., 2014a; Wu and Hu, 2014; Nie et al., 2015; Wang et al., 2015). Relatively systematic comparative research on uranium and polymetallic mineralization has not yet been extensively conducted, particularly lacking isotopic composition comparisons that are crucial for revealing the sources of ore-forming materials and metallogenic mechanisms. The recently implemented China Uranium Scientific Drilling (Abbrev. CUSD1) has revealed uranium and polymetallic deposits within the same borehole, exhibiting a vertical spatial distribution pattern of “uranium above and polymetals below.” This borehole provides an ideal section for systematically collecting samples and conducting in-depth comparative studies.
In this paper, we describe the metallogenic geological characteristics of the uranium-polymetallic mineralization and report H-O-S-Pb-Sr isotopic data and whole-rock Pb and Rb-Sr isotope of the wall rock. These new datasets, together with preciously published results, are utilized to constrain the sources of ore-forming fluids and metals and metallogenic mechanism. Based on previous research findings, this study investigated the magmatic and metallogenic epochs of the Xiangshan ore field, and established a uranium-polymetallic metallogenic model under regional extensional setting. Meanwhile, we take an in-depth comparison between uranium and polymetallic mineralization and establish a comprehensive ore-forming model for uranium-polymetallic mineralization.
2 Geological background
2.1 Regional geology and local geology
The Xiangshan uranium ore field is structurally situated within a large-scale volcanic collapse basin. This volcanic-intrusive basin, located in the Cretaceous extensional tectonic zone between the Yangtze and Cathaysia blocks (Li et al., 2014), occupies a critical metallogenic position in southeastern China. Specifically, it is positioned at the southwestern margin of the Gan-Hang tectonic belt and the northeastern periphery of the Qin-Hang metallogenic belt (Figure 1), where it developed through intense magmatic-tectonic interactions during the Late Mesozoic continental extension.
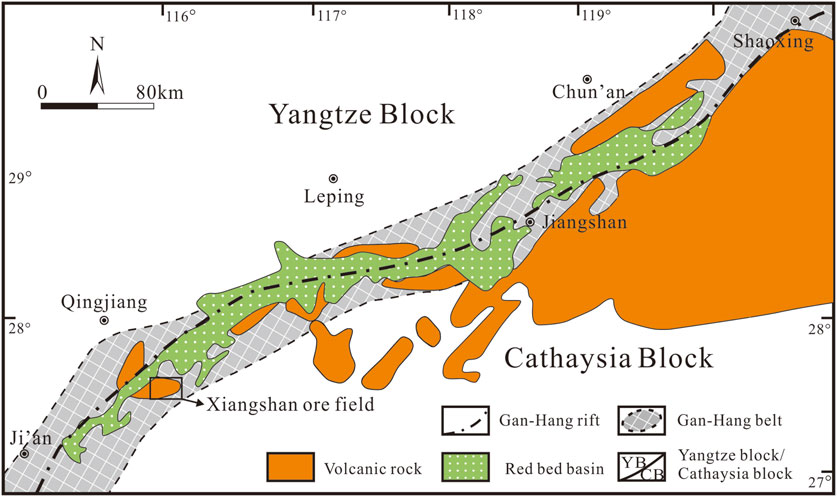
Figure 1. Geological sketch map showing the Gan-Hang Belt. Modified after Yu et al. (2006).
The basement of the volcanic basin consists mainly of low greenschist facies to low amphibolite facies Paleo-Mesoproterozoic metamorphic rocks (amphibolite and schist) and the less-metamorphosed Neoproterozoic (Sinian system) rocks, including phyllite, slate, and metasandstone (Yang et al., 2010). The volcanic rocks from the Xiangshan include rhyolitic crystal tuffs, welded tuffs, rhyodacites, porphyroclastic rhyolite, associated subvolcanic rocks such as monzogranite-porphyry and granite-porphyry, and late dykes such as quartz monzonitic porphyry and lamprophyre. Previous studies showed that the volcanic cover was formed by two cycles (Figure 2). The first volcanic cycle represents a fissure eruption and is composed mainly of rhyodacite that yielded a zircon U-Pb age of 135 Ma (Li et al., 2014; Yang et al., 2010) and rhyolitic welded tuff. The second volcanic cycle was a central vent eruption and was composed mainly of extrusive facies of felsic lava that formed the main part of the Xiangshan volcanic-intrusive complex, yeilding a zircon U-Pb age of 132 Ma (Li et al., 2014).
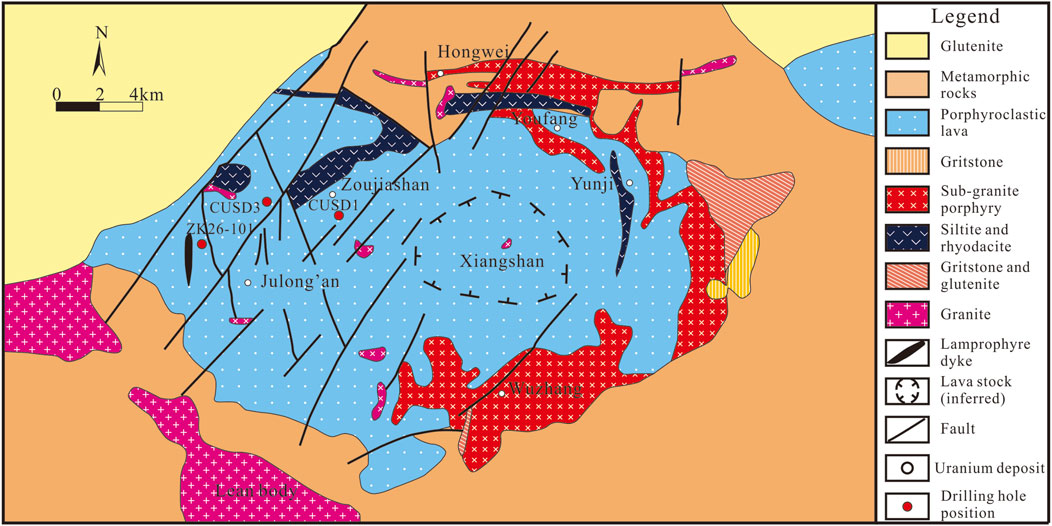
Figure 2. Geological map of Xiangshan volcanic-intrusive complex. Modified after Li and Zhang (2016) and Guo et al. (2020).
So far, almost 30 uranium deposits have been discovered, mainly distributed in the western and northern Xiangshan. Uranium mineralization occurs in fractures attached to NE-trending faults, which cut the volcanic-intrusive complex without dependence on wall rock. Polymetallic mineralization has been discovered at depths of 500–1,500 m below uranium ore bodies, such as in the Niutoushan area (boreholes ZK26-9, ZK26-11, ZK26-101), the Heyuanbei area (CUSD3), and the Niuonaoshang area (CUSD1). The mineralization occurs in both overlying volcanic rocks and basement metamorphic rocks, showing no selectivity toward host rocks. Spatially, the two mineralization types (uranium and polymetallic) exhibit a vertical zoning characterized by “uranium above and polymetallic below” (Figure 3).
2.2 Geology of uranium and polymetallic mineralization
The CUSD1 has been carried out in the Xiangshan volcanic basin, 2 km southeast of the Zoujiashan uranium deposit, with a final depth of 2818 m for the main hole. Three uranium mineralizations and five polymetallic mineralizations, with hydrothermal alteration developing, have been recovered by CUSD1.
Uranium mineralization occurs in cataclastic rhyolite at shallow depths of 200–900 m in borehole CUSD1, with strongly developed hydrothermal alteration such as albitization, hematitization (Figure 4a), fluoritization, carbonatization (Figure 4b), and pyritization. The ore exhibits vein-type and stockwork-type mineralization. The main ore minerals are pitchblende (Figure 4c), brannerite, conffinite, thorite, pyrite, minor molybdenite, chalcopyrite, galena, sphalerite, and native bismuth. The main gangue minerals are calcite, fluorite, quartz, apatite, minor rutile and monazite.
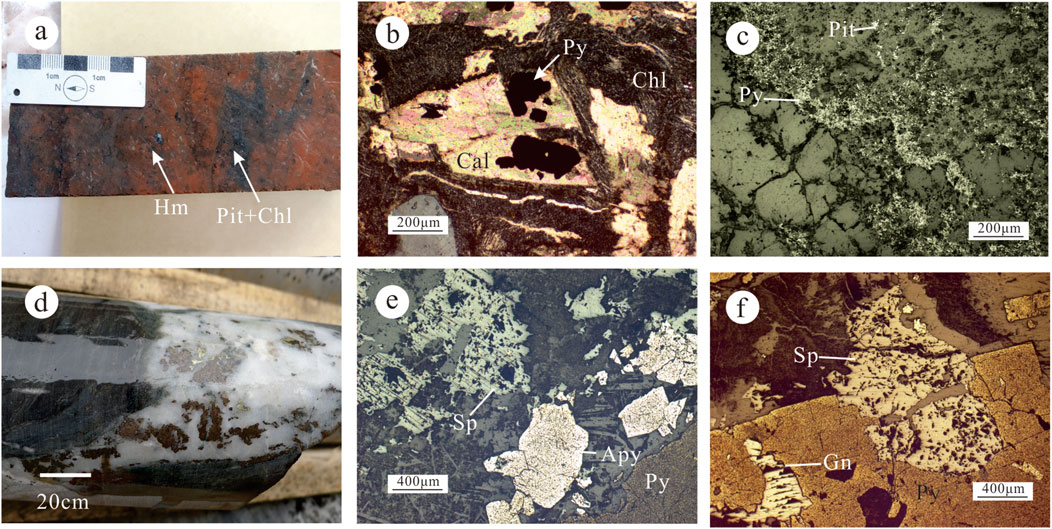
Figure 4. Microscopic characteristics of uranium and polymetallic mineralization in the Xiangshan Uranium Ore Field. (a) Strongly hematitized uranium ore; (b) Intense carbonatization and chloritization; (c) Disseminated pyrite-pitchblende assemblage; (d) Banded polymetallic ore; (e) Paragenesis of sphalerite, arsenopyrite, and pyrite; (f) Sphalerite and galena replacing pyrite, displaying replacement relict texture. Abbreviations: Hm- Hematite, Cal - Calcit e, Chl - Chlorite, Pit - Pitchblende, Py - Pyrite, Sp - Sphalerite, Gn - Galena, Apy - Arsenopyrite.
Polymetallic mineralization occurs within the deep-seated basement metamorphic rocks at vertical depth of 1,400–2,800 m in borehole CUSD1, primarily characterized by hydrothermal alterations such as silicification (Figure 4d), carbonatization, sericitization, and chloritization. The main ore minerals are galena, sphalerite (Figure 4f), arsenopyrite (Figure 4e), pyrite, pyrrhotite, minor chalcopyrite, and argentite. The main gangue minerals are quartz, calcite, siderite, minor sericite, chlorite, and cassiterite.
3 Samples and analytical methods
3.1 Sampling
All samples used in this study were collected from CUSD1, including monomineralic samples for multi-isotope analysis and fresh rock samples. Mineral separates were extracted from crushed and washed sample fragments (40–60 mesh) and were handpicked under a binocular microscope to achieve a purity of >99%. Each grain was carefully examined in order to avoid inclusions.
3.2 Analytical methods
H-O-S-Pb-Sr isotope analyses were performed in the Analytical Laboratory of the Beijing Research Institute of Uranium Geology.
The H-O isotope analysis of calcite and quartz single minerals was conducted using a MAT253 isotope mass spectrometer. The δ18O measurement was obtained by generating O2 using the BrF5 method, which was then converted to CO2 for analysis on the mass spectrometer to obtain the hydrogen and oxygen isotope composition. Through standard calculations, the ΔDH2O of the inclusions was obtained, with an analytical precision of ±2%.
The sulfur isotope compositions of sulfide samples were analyzed on a MAT251E gas mass spectrometer by using Cu2O to oxidize the sulfides. The analytical procedure usually yielded an in-run precision of 0.2‰. The calibration were performed with regular analyses of internal δ34S standard samples.
The lead isotope compositions of sulfide samples were analyzed on the GV IsoProbe-T Thermal Ionization Mass Spectrometer. The analytical procedure involved dissolution of samples using HF and HClO4 in crucibles, followed by basic anion exchange resin to purify Pb. Analytical results for the standard NBS 981 are 208Pb/204Pb = 36.611 ± 0.004 (2σ), 207Pb/204Pb = 15.457 ± 0.002 (2σ), and 206Pb/204Pb = 16.937 ± 0.002 (2σ), in agreement with the reference value (Belshaw et al., 1998).
The strontium isotope composition was determined on a multicollector IsoProbe-T Thermal Ionization Mass Spectrometer. Details of the chemical separation and mass spectrometric procedures are described by Wang et al. (2014b). 87Sr/86Sr is normalized to 88Sr/86Sr = 8.375219 to correct for instrumental fraction. During the period of this study, measurement for the American Standard Reference Material NBS 987 Sr standard gave 87Sr/86Sr = 0.710250 ± 0.000007 (2σ). The total procedural blanks for Rb and Sr were 0.2 ng and 0.2 ng, respectively.
4 Analytical results
4.1 H-O isotope
The analytical results of hydrogen and oxygen isotopes for uranium-polymetallic mineralization are presented in Table 1. The δ18OH2O values were obtained based on the modal temperature measurements of fluid inclusions and the oxygen isotope equilibrium fractionation formulas for the SiO2-H2O and CaCO3-H2O systems. During the uranium mineralization period, the δ18O values of four calcite samples ranged from 8.6‰ to 12.6‰, with an average of 10.63‰. The calculated δ18OH2O values ranged from 0.95‰ to 4.95‰, averaging 2.98‰, while the δ18D values ranged from −87.3‰ to −78.1‰. During the polymetallic mineralization period, the δ18O values of ten quartz samples ranged from 10.4‰ to 14.7‰, with an average of 13.7‰. The calculated δ18OH2O values ranged from 3.51‰ to 7.81‰, averaging 6.80‰, while the δ18D values ranged from −91.3‰ to −71.4‰.
4.2 Sulfur isotope
S isotope compositions of pyrite, pyrrhotite, sphalerite, and arsenopyrite separated from the polymetallic mineralization of CUSD1, together with previously published data (Nie et al., 2015), are listed in Table 2 and showed in histogram (Figure 5). Fourty-five δ34S values of sulfides range from 2 to 6.9‰, with an average value of 4.16‰. The sulfur isotope compositions of pyrite, pyrrhotite, sphalerite, and arsenopyrite range from 2.2‰ to 6.9‰ (n = 21), from 2 to 5.8‰ (n = 12), from 3.3‰ to 3.9‰ (n = 4), and from 3.1 to 4.8 (n = 6), respectively. The obtained single sulfur isotope values of galena and chalcopyrite from polymetallic mineralization are 2.5‰ and 4.6‰. The S isotope compositions of pyrite in uranium mineralization differ significantly from those of galena and chalcopyrite. The S isotope values of 24 pyrites show strong δ34S enrichment, with a wide distribution range and values of δ34S between 6.2‰ and 19.55‰. The average δ34S value of pyrite in uranium ore is 13.15‰.
4.3 Lead isotope
Pb isotope compositions of polymetallic mineralization, uranium ore, porphyroclastic rhyolite, rhyodacite, and metamorphic basement are listed in Table 3, respectively. We estimate the Pb isotope compositions of metamorphic basement with U, Th, and Pb values, denoting an age of 130 Ma, which is the sulfide Rb-Sr dating age (Yang et al., 2015; Guo et al., 2018), to exclude the influence of radioactive lead. We use the Pb isotope compositions of volcanic rock, porphyroclastic rhyolite, and rhyodacite without estimating because of the same age between polymetallic mineralization and the formation of volcanic rock.
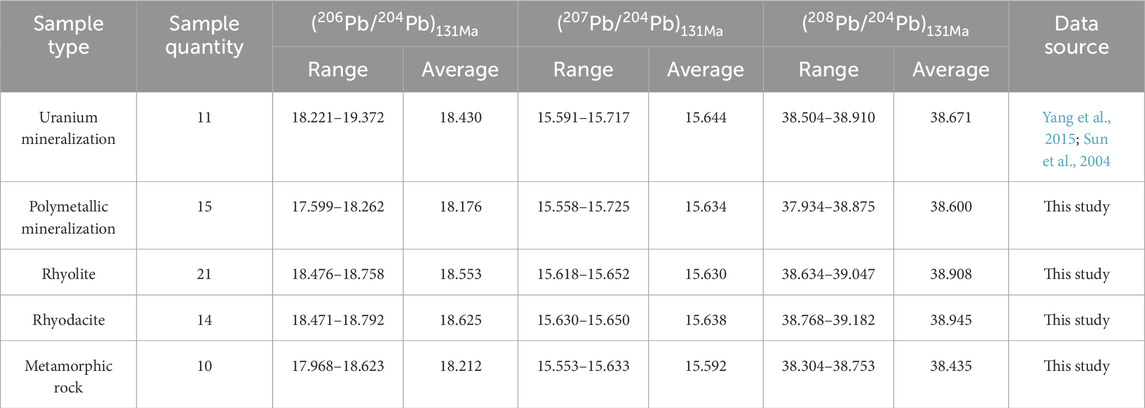
Table 3. Statistical results of the Pb isotope compositions of the uranium mineralization, polymetallic mineralization, volcanic rocks and metamorphic basement.
206Pb/204Pb, 207Pb/204Pb, and 208Pb/204Pb ratios for sulfide minerals of polymetallic mineralization ranging from 17.599 to 18.262, 15.558 to 15.725, and 37.934 to 38.875; for pyrite from uranium ore ranging from 18.221 to 19.372, 15.591 to 15.717, and 38.504 to 38.910; for volcanic rock ranging from 18.471 to 18.792, 15.618 to 15.652, and 38.634 to 39.182. The estimated ratios of metamorphic basement range from 17.968 to 18.623, 15.553 to 15.633, and 38.304 to 38.753.
Sulfide samples have relatively homogeneous and characteristically low radiogenic lead; those from uranium ore is more radiogenic than sulfide from polymetallic mineralization.
4.4 Strontium isotope
The Sr isotope compositions of uranium mineralization, polymetallic mineralization, and major geological units in the Xiangshan ore field were obtained (Table 4). The analyzed results indicate that the Sr isotope composition of cataclastic rhyolite ranges from 0.710511 to 0.713861, with an average of 0.712225. The Sr isotope composition of rhyodacite ranges from 0.710773 to 0.711841, averaging at 0.711147. The Sr isotope composition of the basement metamorphic rocks ranges from 0.718085 to 0.724013, averaging at 0.720212, which is significantly higher than that of the volcanic rocks. The Sr isotope composition of individual fluorite minerals in uranium mineralization ranges from 0.71447 to 0.72072, averaging at 0.71752 (Jiang et al., 2006). The Sr isotope composition of individual pyrite minerals in polymetallic mineralization ranges from 0.719656 to 0.720133, averaging at 0.719833, which is higher than that of individual fluorite minerals in uranium mineralization and similar to the whole-rock Sr isotope composition of the basement metamorphic rocks.
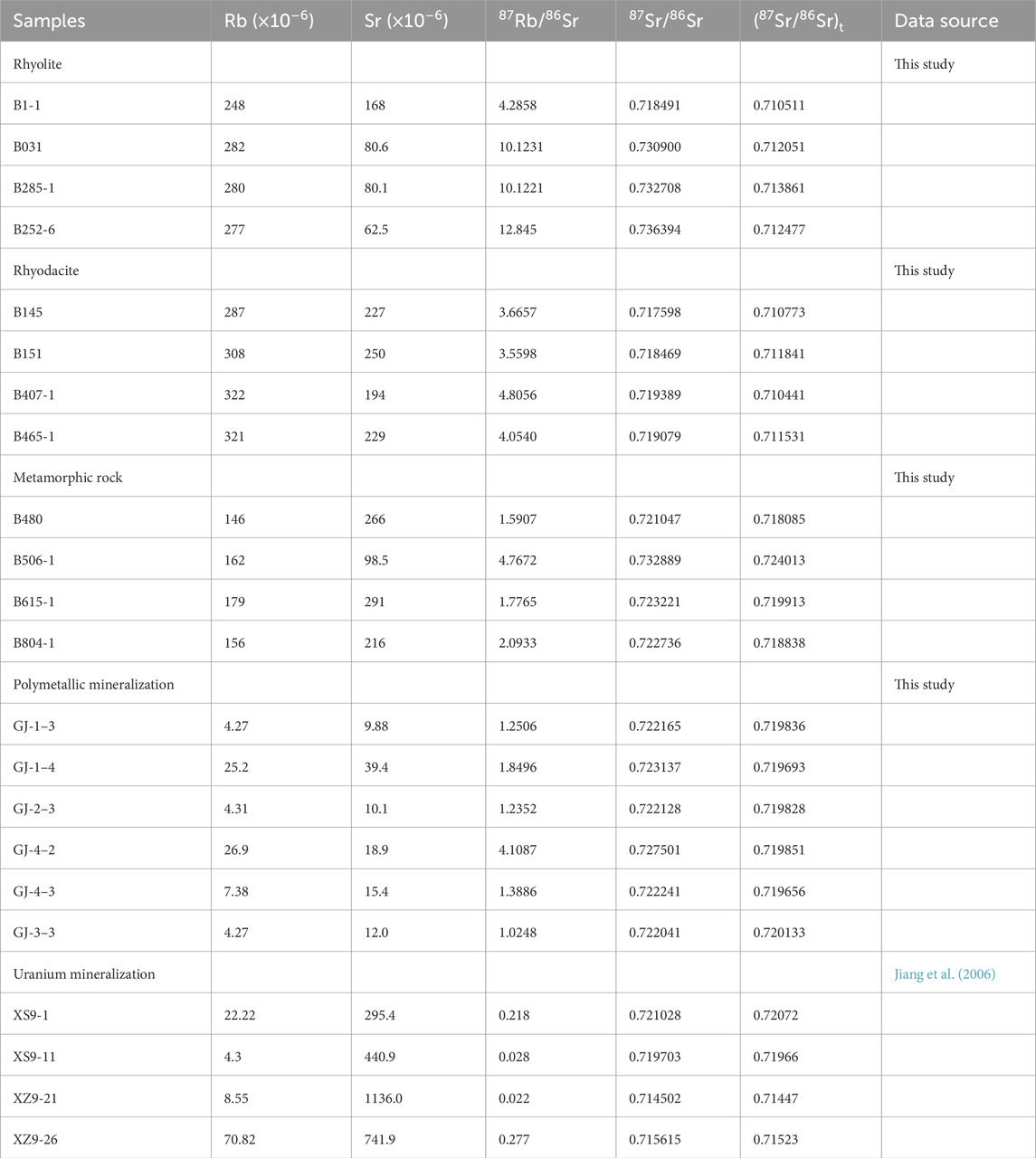
Table 4. Sr isotope composition of uranium mineralization, polymetallic mineralization, and various geological units in the Xiangshan area.
5 Discussion
5.1 Multivariate isotope composition and comparison
5.1.1 H-O isotope
The H-O isotope composition results of the ore-forming fluids in the polymetallic mineralization mainly fall within the range of magmatic water (Figure 6). The hydrogen and oxygen isotope composition results of uranium mineralization are relatively close to those of magmatic water but show a slight deviation toward the meteoric water line. This evidence suggests that the isotopic composition of uranium ore-forming fluids is similar to that of polymetallic mineralization, with both primarily derived from magmatic water. However, the uranium ore-forming fluids exhibit a higher contribution of meteoric water compared to the polymetallic mineralization.
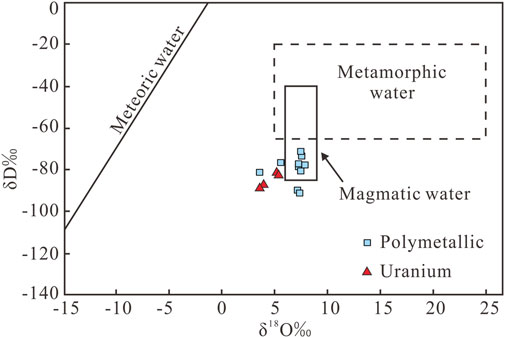
Figure 6. H-O isotopes composition of uranium and polymetallic mineralization (modified after Taylor, 1979).
5.1.2 Sulfur isotope composition
Primary ores from the polymetallic mineralization are composed chiefly of pyrite, pyrrhotite, galena, sphalerite, and arsenopyrite. The lack of sulfate minerals in ores suggests that δ34S value of the hydrothermal fluid can be represented by that of sulfide minerals. All ore minerals have δ34S values that are relatively high, with a range of 2–6.9‰ (average 4.16‰). These values are close to the meteorite and meomantle-derived magmatic sulfur (0‰ ± 3‰, Rollinsion, 1993), suggesting a deep crust and upper mantle source (Wang et al., 2014b).
The sulfur isotope composition of pyrite during the uranium mineralization period ranges from 9.6‰ to 19.55‰, with an average of 14.65‰ and a range (difference between the maximum and minimum values) of 9.95‰. This composition is significantly higher than that of the main stratigraphic units and polymetallic mineralization in the Xiangshan ore field (Figure 7), and the range of isotope composition is relatively large. Previous studies have shown that the uranium ore-forming materials in the Xiangshan ore field mainly originated from deep-sourced magma (Li et al., 2014; Guo, 2014). The isotope composition ranging from 9.6‰ to 19.55‰ and the large range indicate that, compared to polymetallic mineralization, the uranium mineralization period involved a greater contribution of crustal sulfur.
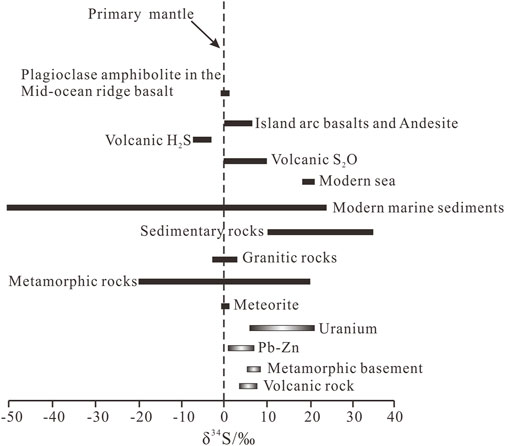
Figure 7. Uranium polymetallic mineralization and sulfur isotope composition of major geological units in the Xiangshan ore field. The sulfur isotope composition of main geological unit come from Kelly and Rye, 1979, Chaussidon and Lorand, 1990, and Rollinson 2014.
5.1.3 Lead isotope composition
Generally, if lead from different geological units is derived from the same source, the Pb isotope compositions and variation trends should be similar (Cannon et al., 1961). The isotopic values from the volcanic rock and metamorphic basement were compared to the Pb isotopic compositions of sulfides from the polymetallic mineralization. The Pb isotopic composition of the ores mostly falls in the metamorphic basement-forming region, which is very far from the volcanic rock-forming region (Figure 8). The volcanic rock is dated at ca. 132 to 135 Ma (Guo, 2014; Li et al., 2014), which closely matches the age of the polymetallic mineralization (131 Ma, Liu et al., 2019). However, the Pb isotope composition of the volcanic rock is inconsistent with that of the polymetallic ore, which also suggests that it could not have been a significant source of lead in the ores. Considering that the ore sufides were the result of fluid-rock interaction, the metamorphic basement is possibly a major source for lead and other metals in the Pb-Zn mineralization (Guo et al., 2018).
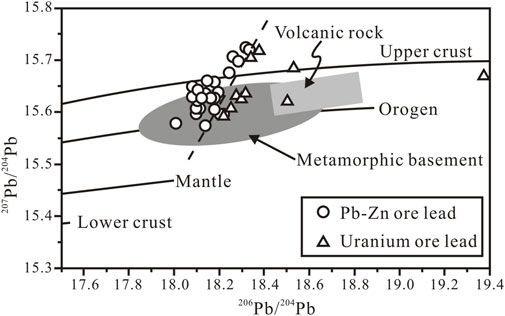
Figure 8. Uranium-polymetallic mineralization and lead isotope composition of major geological units in the Xiangshan ore field. Trends for the upper crust, orogen, mantle, and lower crust are taken from Zartman and Doe (1981).
Compared to polymetallic sulfides, the lead isotope composition of pyrite in uranium ores is relatively closer to the distribution range of lead isotopes in metamorphic rock bases and orogenic belts (Figure 8). This suggests that more components from surrounding rocks such as the basement and volcanic rocks were incorporated during uranium mineralization. The sample points in the polymetallic mineralized sulfides also exhibit a linear distribution pattern. Compared to most samples, there is an addition of radiogenic lead in the sulfides, and the distribution pattern of lead isotope composition is similar to that of pyrite samples from uranium mineralization. This indicates that during the process of polymetallic mineralization, there may have been a relative enrichment of uranium due to the polymetallic mineralization process.
5.1.4 Strontium isotope composition
Based on the age of polymetallic mineralization in Xiangshan area (about 131 Ma), we calculated the initial Sr isotope compositions of the sulfide and whole-rock. Sulfide separates from the polymetallic mineralization has initial 87Sr/86Sr ratios ranging from 0.719656 to 0.720133, which is higher than that of the continental crust mean value of 0.719 (Zeng et al., 2020). The metamorphic rock has (87Sr/86Sr) t (t = 131 Ma) ratios ranging from 0.718085 to 0.724013 (Table 3), including the 87Sr/86Sr ratios range of sulfides. The volcanic rocks, including rhyolite and rhyodacite, have (87Sr/86Sr) t (t = 131 Ma) ratios ranging from 0.7103 to 0.7139 (Table 3), lower than those of sulfides and lower metamorphic basement. Therefore, the source materials for the polymetallic mineralization may have been derived mainly from the crust repository, most likely come from the metamorphic basement.
Compared to polymetallic mineralization, uranium mineralization exhibits a broader range of strontium isotope compositions, displaying a bimodal characteristic (Figure 9). One portion of these compositions is relatively close to those of polymetallic mineralization and metamorphic rock basement, while another portion is closer to those of volcanic rocks. This indicates that, in comparison to polymetallic mineralization, the sources of uranium ore-forming materials are more complex or that the mineralization process was more significantly influenced by contamination from exogenous materials.
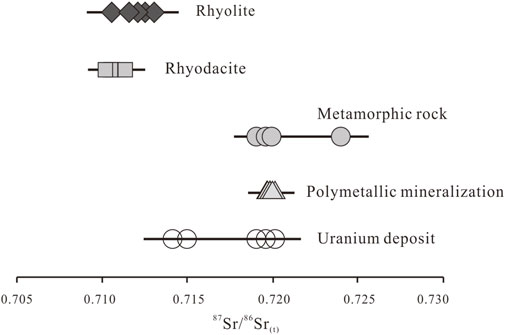
Figure 9. Comparison of initial Sr isotope values between uranium mineralization, polymetallic mineralization, and related strata in Xiangshan area (Modified after Guo et al., 2018)
5.2 The mineralization model of uranium and polymetallic mineralization
5.2.1 Temporal and spatial relationships of magmatism and mineralization in the Xiangshan area
The age of magmatic and hydrothermal events is important for the understanding of ore deposits from both academic and exploration viewpoints (Stein et al., 1998). Previous studies have concluded that the volcanic activity derived two eruptive circles, forming the main Xiangshan volcanic complex at 135 Ma to 132 Ma (Li et al., 2014; Guo, 2014). Following the extensive volcanic activity, the polymetallic mineralization occurred at 131 Ma (Guo et al., 2018). The early Cretaceous was newly discovered and yet to be discovered at the depth of Uranium mineralization. Meng (2012) and Wang et al. (2023) have summarized the ore-forming age of uranium mineralization ranges from 143 to 98 Ma, with a primary focus around 100 Ma. On this basis, we have systematically constructed the geochronological framework of petrogenesis and mineralization in the Xiangshan ore field, as illustrated in Figure 10.
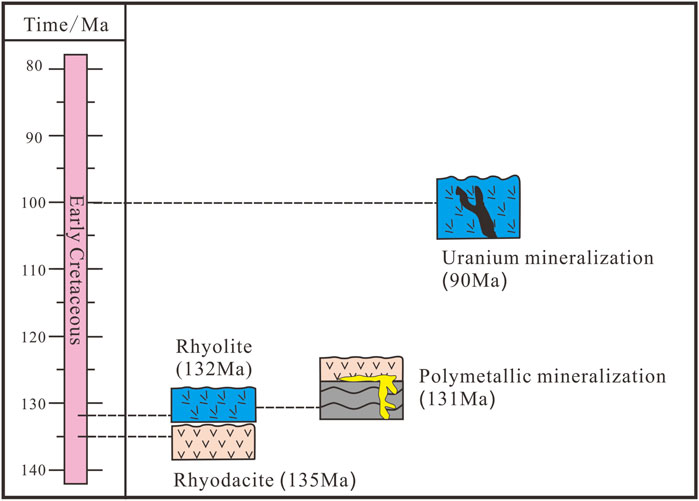
Figure 10. Geochronological framework of petrogenesis and mineralization in Xiangshan area. The ages come from Guo et al., 2018; Li et al., 2014, Meng, 2012 and Wang et al. (2023).
5.2.2 Possible geodynamic setting and genetic model for polymetallic and U mineralization
The geodynamic setting responsible for the Cretaceous magmatism and mineralization has been a controversy. The widespread A-type granites (Chen et al., 2013; Jiang et al., 2011; Peng et al., 2021) and the development of pull-apart basins filled with bimodal volcanic rocks (Li, 2000), such as the Xiangshan volcanic intrusive complex (He et al., 2009; Yang et al., 2010; Yang et al., 2011) strongly suggest that SE China was in an extensional setting during the Cretaceous, mainly caused by the subduction of the Paleo-pacific slab.
Previous studies support a transitional regime from compressive extension at approximately 135 Ma (Mao et al., 2004; Mao et al., 2013), just as it is the time to begin large-scale tectonic-magmatic activities in the Xiangshan area (Figure 11A). During 135–90 Ma, thermal activity related to subduction of the Paleo-pacific slab resulted in the ore fluids and accompanying metals from the enriched lithospheric mantle upwelling (Jiang et al., 2011). The polymetallic ore-forming progress can be divided to two endmembers. One provided the reduced sulfur, and the other provided the important ore-forming metal. The sulfur is mainly derived from mantle reservoirs, gathering and transferring by magmatism. The polymetallic enriched metamorphic basement may have provided the Pb-Sr metals for hydrothermal fluids. Extensive fluid migration and circulation of hydrothermal fluids homogenized isotopically. At about 131 Ma, driven by tectonic and thermal dynamics, the resulting hydrothermal fluids were enriched in metals when migrated upward along regional and local faults and incorporated reduced sulfur-bearing solutions, leading to the precipitation of the polymetallic ores along fracture zones and inter-formational boundaries (Figure 11B). During 100–90 Ma, the polymetallic mineralization process led to continuous enrichment of uranium in deep source regions and changes in physicochemical conditions. The rapid and sustained extension and thinning of the Xiangshan area drove the occurrence of uranium mineralization. Intense crust-mantle material exchange was manifested as an increasingly open uranium mineralization environment. During the uranium mineralization process, abundant crust-derived materials such as country rocks and meteoric water continuously mixed and contaminated the system, resulting in a wide range of sulfur isotopic compositions and lead isotopic distribution patterns similar to those of major geological units in the Xiangshan area (Figure 11C).
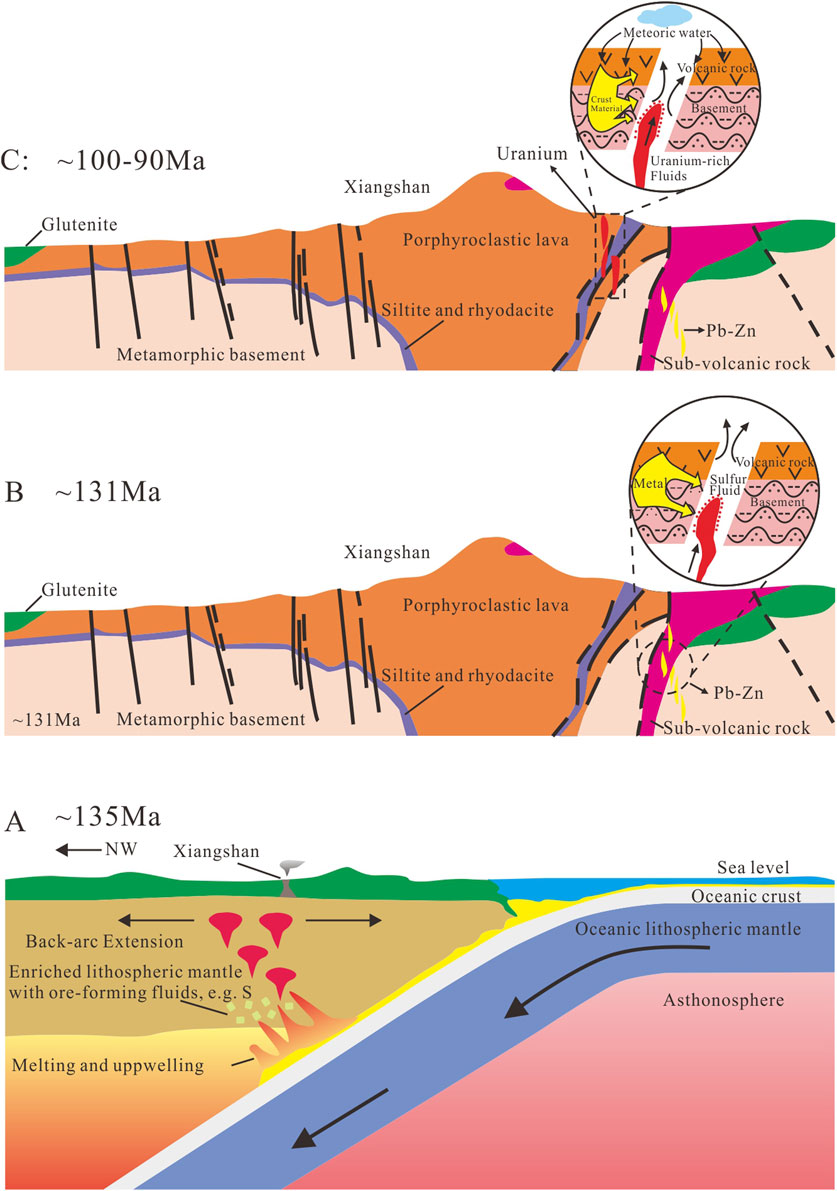
Figure 11. (A) Formation stage of Xiangshan volcanic intrusion complex; (B) The uranium mineralization stage of Xiangshan ore field; (C) The polymetallic mineralization stage of Xiangshan ore field.
6 Conclusion
(1) Comparative analysis of H-O isotopic compositions between uranium and polymetallic mineralization fluids reveals that polymetallic mineralization fluids are primarily derived from magmatic water, whereas uranium mineralization fluids exhibit significant incorporation of meteoric water compared to polymetallic systems.
(2) Comparative study of sulfur isotopic compositions indicates that polymetallic mineralization materials share consistent sulfur isotopic characteristics with regional typical polymetallic deposits, both originating from magmatic sources. In contrast, sulfur isotopes in uranium mineralization materials suggest substantial contributions from crust-derived materials such as volcanic rocks and basement metamorphic rocks during mineralization.
(3) Lead and strontium isotopic comparisons between uranium and polymetallic mineralization materials demonstrate that uranium mineralization incorporates more crust-derived components (e.g., volcanic rocks and basement metamorphic rocks) compared to polymetallic mineralization. Furthermore, Pb isotopic compositions of polymetallic mineralization imply potential U-enriched mineralization processes during polymetallic ore formation.
Data availability statement
The datasets presented in this study can be found in online repositories. The names of the repository/repositories and accession number(s) can be found in the article/supplementary material.
Author contributions
JG: Writing – original draft, Writing – review and editing. ZL: Investigation, Writing – review and editing. JN: Investigation, Writing – review and editing. SH: Data curation, Writing – review and editing. JW: Formal Analysis, Writing – review and editing. WK: Methodology, Writing – review and editing.
Funding
The author(s) declare that financial support was received for the research and/or publication of this article. This article is supported by the China Uranium Industry Youth Innovation Fund (YQN2401).
Conflict of interest
The authors declare that the research was conducted in the absence of any commercial or financial relationships that could be construed as a potential conflict of interest.
Generative AI statement
The author(s) declare that no Generative AI was used in the creation of this manuscript.
Publisher’s note
All claims expressed in this article are solely those of the authors and do not necessarily represent those of their affiliated organizations, or those of the publisher, the editors and the reviewers. Any product that may be evaluated in this article, or claim that may be made by its manufacturer, is not guaranteed or endorsed by the publisher.
References
Belshaw, N. S., Freedman, P. A., O’nions, R. K., Frank, M., and Guo, Y. (1998). A new variable dispersion double-focusing plasma mass spectrometer with performance illustrated for Pb isotopes. Int. J. Mass Spectrom. 181 (1-3), 51–58. doi:10.1016/s1387-3806(98)14150-7
Cannon, R. S., Pierce, A. P., Antweiler, J. C., and Buck, K. L. (1961). The data of lead isotope geology related to problems of ore genesis. Econ. Geol. 56 (1), 1–38. doi:10.2113/gsecongeo.56.1.1
Chaussidon, M., and Lorand, J.-P. (1990). Sulphur isotope composition of orogenic spinel lherzolite massifs from Ariege (North-Eastern Pyrenees, France): an ion microprobe study. Geochimica Cosmochimica Acta 54 (10), 2835–2846. doi:10.1016/0016-7037(90)90018-g
Chen, K., Gao, S., Wu, Y., Guo, J., Hu, Z., Liu, Y., et al. (2013). 2.6–2.7 Ga crustal growth in Yangtze craton, South China. Precambrian Res. 224, 472–490. doi:10.1016/j.precamres.2012.10.017
Fan, H. H., Ling, H. F., Wang, D. Z., Liu, C. S., Shen, W. Z., and Jiang, Y. H. (2003). Study on metallogenic mechanism of Xiangshan uranium ore-field. Uranium Geol. 4 (4), 208–213.
Guo, F. S., Yang, Q. K., Meng, X. J., Xie, C. F., Shi, G., Chen, L. Q., et al. (2016). Geochemical characteristics and petrogenesis of the acidic volcanic-intrusive complexes,Xiangshan,Jiangxi. Acta Geol. Sin. 90 (4), 769–784.
Guo, J., Li, Z., Nie, J., Huang, Z., Wang, J., and Lai, C. K. (2018). Genesis of Pb–Zn mineralization beneath the Xiangshan uranium orefield, South China: constraints from H–O–S–Pb isotopes and Rb–Sr dating. Resour. Geol. 68 (3), 275–286. doi:10.1111/rge.12170
Guo, J. (2014). The petrology and uranium mineralization alteration study of deep drilling in Xiangshan uranium orefield. Beijing: Beijing Research Institute of Uranium Geology.
Guo, J., Li, Z. Y., Nie, J. T., Huang, Z. Z., Li, X. Z., Wang, J., et al. (2020). Trace-element geochemistry characteristics of pyrite in polymetallic mineralization in the depth of Xiangshan uranium orefield,Jiangxi Province. Acta Petrologica Mineralogica 39 (03), 257–266.
He, G. S., Dai, M. Z., Li, J. F., Cao, S. S., Xia, B., Xu, D. R., et al. (2009). SHRIMP zircon U-Pb dating and its geological implication for the Xiangshan porphyric dacite-rhyolitic. Geotect. Metallogenia 33 (2), 299–303. (in Chinese with English abstract). doi:10.16539/j.ddgzyckx.2009.02.007
Jiang, Y. H., Ling, H. F., Jiang, S. Y., Shen, W. Z., Fan, H. H., and Ni, P. (2006). Trace element and Sr-Nd isotope geochemistry of fluorite from the Xiangshan uranium deposit, southeast China. Econ. Geol. 101 (8), 1613–1622. doi:10.2113/gsecongeo.101.8.1613
Jiang, Y. H., Zhao, P., Zhou, Q., Liao, S. Y., and Jin, G. D. (2011). Petrogenesis and tectonic implications of Early Cretaceous S-and A-type granites in the northwest of the Gan-Hang rift, SE China. Lithos 121 (1-4), 55–73. doi:10.1016/j.lithos.2010.10.001
Kelly, W. C., and Rye, R. O. (1979). Geologic, fluid inclusion, and stable isotope studies of the tin-tungsten deposits of Panasqueira, Portugal. Econ. Geol. 74 (8), 1721–1822. doi:10.2113/gsecongeo.74.8.1721
Li, X. H. (2000). Cretaceous magmatism and lithospheric extension in Southeast China. J. Asian Earth Sci. 18 (3), 293–305. doi:10.1016/s1367-9120(99)00060-7
Li, Z. Y., Huang, Z. Z., Li, X. Z., Zhang, J. D., Lin, Z. Y., and Zhang, Y. Y. (2014). Xiangshan igneous rocks and uranium mineralization. Beijing: Geological Publishing House.
Li, Z. Y., and Zhang, W. L. (2016). Main uranium mineralization types and their comparison of geochemical characteristics in Xiangshan orefield,Jiangxi. Geoscience 30 (1), 1–16.
Liu, H. B., Jin, G. S., Han, J., Li, J. J., Zhang, J., and Zhang, J. F. (2015). Metallogenic chronology of hydrothermal uranium deposits in southeastern China. Acta Geol. Sin. 89 (S1), 157–159.
Liu, J. L., Li, Z. Y., Nie, J. T., Zhang, W. L., Wang, Y. J., and Tian, M. M. (2019). The timing and ore-forming fluid evolution of deep polymetalic mineralization in western Xiangshan uranium ore field, South China: constraints from Rb-Sr isotope systematics. Acta Petrol. Sin. 35 (09), 2787–2800. doi:10.18654/1000-0569/2019.09.11
Mao, J. W., Cheng, Y. B., Chen, M. H., and Franco, P. (2013). Major types and time–space distribution of Mesozoic ore deposits in South China and their geodynamic settings. Miner. Deposita 48 (3), 267–294. doi:10.1007/s00126-012-0446-z
Mao, J. W., Xie, G. Q., Li, X. F., Zhang, C. Q., and Mei, Y. X. (2004). Mesozoic large-scale mineralization and multi-stage lithospheric extension in South China. Earth Sci. Front. (01), 45–55.
Meng, Y. N. (2012). Study on the metallogenic mechanism of uranium-thorium ore deposits in the western xiangshan ore field. Beijing: Beijing Research Institute of Uranium Geology.
Nie, J. T., Li, Z. Y., Wang, J., and Guo, J. (2015). Characteristics of polymetallic ore-forming fluid and metallogenesis of the Xiangshan ore-field in Jiangxi. Geol. Bull. China 34 (S1), 535–547.
Peng, H. W., Fan, H. R., Jiang, P., Hu, H. L., and Lan, T. G. (2021). Two-stage rollbacks of the paleo-Pacific plate beneath the Cathaysia block during Cretaceous: insights from A-type granites and volcanic rocks. Gondwana Res. 97, 158–175. doi:10.1016/j.gr.2021.05.020
Rollinson, H. R. (1993). A terrane interpretation of the archaean limpopo belt. Geol. Mag. 130 (6), 755–765. doi:10.1017/s001675680002313x
Rollinson, H. R. (2014). Using geochemical data: evaluation, presentation, interpretation. London: Routledge.
Si, Z. (2018). “Comparative study on uranium and polymetallic mineralization in Xiangshan ore field,Jiangxi Province,”. Master’s thesis (Beijing: Beijing Research Institute of Uranium Geology).
Stein, H. J., Sundblad, K., Markey, R. J., Morgan, J. W., and Motuza, G. (1998). Re-Os ages for Archean molybdenite and pyrite, Kuittila-Kivisuo, Finland and Proterozoic molybdenite, Kabeliai, Lithuania: testing the chronometer in a metamorphic and metasomatic setting. Miner. Deposita 33, 329–345. doi:10.1007/s001260050153
Sun, Z. X. (2004). Geochemical evidence for uranium sources in Xiangshan uranium ore field. Acta Mineral. Sin. 24 (1), 19–24.
Taylor, H. P. (1979). Oxygen and hydrogen isotope relationships in hydrothermal mineral deposits. Geochem. hydrothermal ore deposits, 236–277.
Wang, J. G., Chen, R. Q., Xie, G. F., Ji, G. P., and Ma, Y. Y. (2014a). Geological features and prospecting potential of multimetal deposit in Niutoushan area of Xiangshan ore field. World Nucl. Geosci. 31 (1), 23–26.
Wang, J., Nie, J. T., and Guo, J. (2015). Characteristic of copper mineralization and fluid evolution in Xiangshan ore field,Jiangxi Province. J. Mineralogy Petrology 35 (4), 74–84. doi:10.19719/j.cnki.1001-6872.2015.04.012
Wang, X., Li, S. R., Santosh, M., Gan, H. N., and Sun, W. Y. (2014b). Source characteristics and fluid evolution of the Beiyingxigou Pb–Zn–Ag deposit, central North China Craton: an integrated stable isotope investigation. Ore Geol. Rev. 56, 528–540. doi:10.1016/j.oregeorev.2013.02.003
Wang, Y., Fan, H., Qin, K., Evans, N. J., Zhang, C., Zou, X., et al. (2023). Mineralization age of the Xiangshan uranium ore field, South China redefined by hydrothermal apatite U-Pb geochronology. Ore Geol. Rev. 160, 105586. doi:10.1016/j.oregeorev.2023.105586
Wu, Z. J., and Hu, Z. H. (2014). Uranium-polymetallic metallogenic characteristics and prospecting direction of Niutoushan uranium deposit in Xiangshan orefield. World Nucl. Geosci. 31 (2), 89–94.
Yan, B., Yan, H., Zhou, L., Wang, T., Xu, G. M., and Cao, Y. (2013). Isotopic characteristics of C,O,H,and S in xiangshan uranium orefield,Jiangxi province. J. Mineralogy Petrology 33 (3), 47–53. doi:10.19719/j.cnki.1001-6872.2013.03.008
Yang, Q. K., Huang, Q. T., and Sun, Q. Z. (2015). Geological characteristics of sulfer and lead isotopes in the Xiangshan ore field. Bull. Mineralogy,Petrology Geochem. 34 (4), 755–762.
Yang, S. Y., Jiang, S. Y., Jiang, Y. H., Zhao, K. D., and Fan, H. H. (2011). Geochemical, zircon U–Pb dating and Sr–Nd–Hf isotopic constraints on the age and petrogenesis of an Early Cretaceous volcanic-intrusive complex at Xiangshan, Southeast China. Mineralogy Petrology 101, 21–48. doi:10.1007/s00710-010-0136-4
Yang, S., Jiang, S., Jiang, Y., Zhao, K., and Fan, H. (2010). Zircon U-Pb geochronology, Hf isotopic composition and geological implications of the rhyodacite and rhyodacitic porphyry in the Xiangshan uranium ore field, Jiangxi Province, China. Sci. China Earth Sci. 53, 1411–1426. doi:10.1007/s11430-010-4058-0
Yu, X. Q., Wu, G. G., Shu, L. S., Yan, T. Z., Zhang, D., and Di, Y. J. (2006). The cretaceous tectonism if the Gan-Hang tectonic belt, southeastern China. Earth Sci. Front. 13 (03), 31–43.
Keywords: Xiangshan ore field, uranium mineralization, polymetallic mineralization, multi-isotope data, metallogenic model
Citation: Guo J, Li Z, Nie J, He S, Wang J and Khan W (2025) Uranium-polymetallic mineralization relationship and comprehensive mineralization model in the Xiangshan ore field, South China: constraints from H-O-S-Pb-Sr multi-isotope data. Front. Earth Sci. 13:1604873. doi: 10.3389/feart.2025.1604873
Received: 02 April 2025; Accepted: 28 April 2025;
Published: 14 May 2025.
Edited by:
Mao-Wen Yuan, China University of Geosciences, ChinaReviewed by:
Jie Li, Hebei GEO University, ChinaZhen Yan, Chinese Academy of Geological Sciences (CAGS), China
Chuan Xu, Chengdu University of Technology, China
Copyright © 2025 Guo, Li, Nie, He, Wang and Khan. This is an open-access article distributed under the terms of the Creative Commons Attribution License (CC BY). The use, distribution or reproduction in other forums is permitted, provided the original author(s) and the copyright owner(s) are credited and that the original publication in this journal is cited, in accordance with accepted academic practice. No use, distribution or reproduction is permitted which does not comply with these terms.
*Correspondence: Jian Guo, Z3Vvamlhbjk4MThAMTYzLmNvbQ==