- 1Department of Geography, Environmental Change Research Centre, University College London, London, United Kingdom
- 2Leibniz Institute for Baltic Sea Research, Rostock, Germany
While the ubiquity and rising abundance of microplastic contamination is becoming increasingly well-known, there is very little empirical data for the scale of their historical inputs to the environment. For many pollutants, where long-term monitoring is absent, paleoecological approaches (the use of naturally-accumulating archives to assess temporal trends) have been widely applied to determine such historical patterns, but to date this has been undertaken only very rarely for microplastics, despite the enormous potential to identify the scale and extent of inputs as well as rates of change. In this paper, we briefly assess the long-term monitoring and paleoecological microplastic literature before considering the advantages and disadvantages of various natural archives (including lake and marine sediments, ice cores and peat archives) as a means to determine historical microplastic records, as well as the range of challenges facing those attempting to extract microplastics from them. We also outline some of the major considerations in chemical, physical and biological taphonomic processes for microplastics as these are critical to the correct interpretation of microplastic paleoecological records but are currently rarely considered. Finally, we assess the usefulness of microplastic paleoecological records as a stratigraphic tool, both as a means to provide potential chronological information, as well as a possible marker for the proposed Anthropocene Epoch.
Research Highlights
- The concept of paleoecology is explored from a microplastic context
- Dated natural archives provide reliable microplastic temporal records
- Taphonomic processes influence microplastic transport and accumulation
- Microplastic/polymers have utility as stratigraphic markers in sediments
- Methodological standardization is required in microplastic paleoecology.
Introduction
Microplastics are now considered ubiquitous in the environment. They have been recorded in polar ice (Obbard et al., 2014), within amphipods in the deepest ocean trenches (Jamieson et al., 2017), and in the atmosphere and sediments of remote mountain lakes (Free et al., 2014; Allen et al., 2019). They have been recorded in high concentrations in fresh- and ocean surface waters, in a wide range of biota and terrestrial soils and therefore represent evidence of diverse anthropogenic contamination sources on a global scale.
Although there is currently no standardized definition, microplastics are generally understood to be solid, insoluble, polymeric or co-polymeric materials either created (primary microplastics) or fragmented (secondary) to a size of below 5 mm. There have been a number of proposals calling for standardization of terminology but even these have different classifications. Hartmann et al. (2019) suggested a size-range of 1–1,000 μm while Frias and Nash (2019) proposed 1 μ m to 5 mm, and the European Chemical Agency, 1 nm−5 mm (ECHA, European Chemicals Agency, 2019) for intentional (i.e., primary) microplastics. Within these size-bounds the term encompasses a wide range of polymers to which a further variety of additives including plasticizers, flame retardants and stabilizers may have been added, as well as a broad range of morphologies from fibers and fragments to beads, films and foams and all imaginable colors (Rochman et al., 2019). Furthermore, these particles may adsorb contaminants including persistent organic pollutants and trace metals and provide a transfer mechanism for attached microbiota. Such contaminant adsorption may be enhanced during environmental weathering as surface areas increase (Teuten et al., 2009). Clearly, microplastics cannot be considered a single contaminant but rather a “diverse contaminant suite” (Rochman et al., 2019) and this raises considerable challenges in their extraction and analysis from within environmental compartments. However, while many of the properties of microplastics are wide-ranging, physical and chemical durability are commonplace. These properties, plus the dramatic increase in plastic production in recent decades, reaching more than 359 million tons in 2018 (Plastics Europe, 2019) (Figure 1), have resulted in their global ubiquity and preservation.
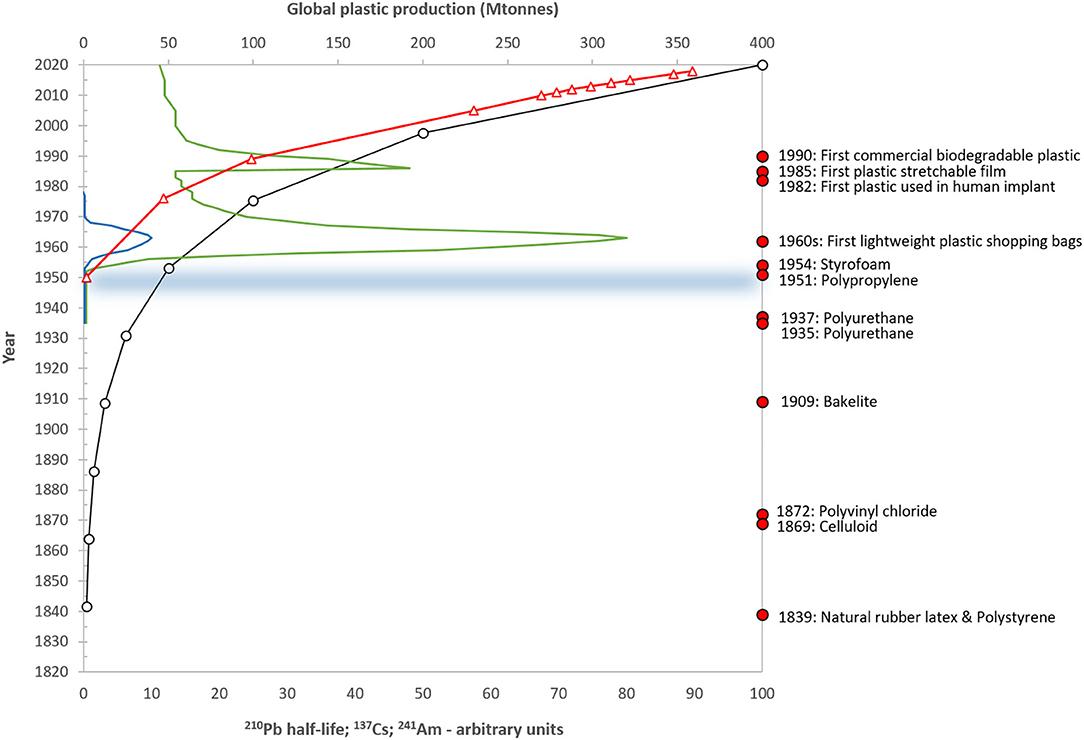
Figure 1. Schematic diagram showing the timescales of radiometric dating techniques for natural archives, including the decay of the natural isotope 210Pb (half-life 22.23 years; open circles); the 1963 nuclear weapons bomb-testing peaks of 137Cs (green line) and 241Am (blue line); and the Chernobyl reactor accident in 1986 (137Cs). The global production of plastics in millions of tons is also shown (red triangles) (data from Plastics Europe, 2012, 2016, 2019) along with selected moments in plastic production history (taken from Crawford and Quinn, 2016) and the start of the proposed Anthropocene Epoch in circa. 1950 (horizontal line).
The majority of microplastics studies have been undertaken in the oceans with far fewer in freshwater, terrestrial and atmospheric systems (Meng et al., 2020). However, although there is now considerable information on the distribution of macro- and microplastic abundance in ocean surface waters and shorelines, and rapidly increasing knowledge on chemical and morphological classes, there remains very little information on temporal changes. For example, the rates at which microplastic inputs to aquatic and terrestrial systems are increasing is very poorly understood even though this would provide valuable insights into the potential exposure to biota. The relative novelty of microplastics as an environmental contaminant has so far precluded any long-term monitoring of concentrations and even for macroplastics such data are sparse.
Where such long-term data have been absent, paleoecological approaches, the use of naturally accumulating archives to provide historical data of varying resolution and longevity, have been widely used to assess physical, biological and chemical change. However, this has only recently started to be applied to microplastics. As a result, the science of microplastic paleoecology is in its infancy and studies to date are generally limited to producing historical profiles from individual sites and comparing these against broad-scale, plastic production data. However, the development of paleoecology tells us that there are considerable challenges to the interpretation of the records stored in natural archives and that such comparisons may come to be viewed as rather simplistic. These challenges are not only those of using standardized and comparable techniques and units between studies, although these remain for microplastics, but also issues around taphonomy, i.e., the processes affecting how microplastics of varying provenance are transported to, and buried within, the selected archive location. Future paleoecological studies involving microplastics will certainly have to consider these issues. The aims of this paper, therefore, are 4-fold: (i) to assess the current status of microplastic paleoecology and highlight gaps in knowledge; (ii) to consider the advantages and disadvantages of common natural archives for determining microplastic records; (iii) to use paleoecological knowledge to highlight some of the issues and uncertainties that will need to be considered for future microplastic records to be interpreted in a more robust way; and (iv) in the light of these issues, consider how microplastic paleoecological records may be used chronologically as a stratigraphic marker, e.g., for the proposed Anthropocene Epoch (Zalasiewicz et al., 2016).
Long-Term Records of Macro- and Microplastics
With the rapid increase in global plastic production and the input of debris into the oceans it might be expected that increasing trends in macroplastics would be observed throughout the world, but data indicate that trends are far more ambiguous. At the HAUSGARTEN deep-sea observatory (located at 79°N 4°E; 2,500 m below the ocean surface), litter densities increased from 3,635 to 7,710 km−2 between 2004 and 2011, and especially since 2007, with plastics remaining the dominant litter-type throughout (Bergmann and Klages, 2012). However, on shores around Antarctic islands, abundances in plastic accumulation between 1990 and 2006 were similar and may even have declined (Barnes et al., 2009). In Hawaii, debris densities showed considerable inter-annual variability between 1990 and 2006 but no directional trend, while in the UK, debris increased steadily from 1994 (Barnes et al., 2009). In South Africa, trends in the number of plastic bottles increased between 1984 and 2005 on beaches with no cleaning programmes but stayed much the same where those programmes existed. By contrast, numbers of plastic bottle lids increased in both locations, thought to be due to their small size, i.e., that they might be overlooked by beach-cleaning teams (Ryan et al., 2009). The monitoring of plastic on the floor of the North Sea has been undertaken since 1992 and also shows considerable variation in spatial litter densities (for example, between 0 and 1835 km−2 in 2011). Here, no clear difference was observed between near-shore and off-shore areas (Maes et al., 2018) and while 63% of all sampling trawls over the 25 years contained plastic, there was no significant trend through the monitoring period. However, trends in specific litter categories such as plastic sheeting (including packaging) and “fishing-related” debris (including fishing line, cable ties, straps, and crates) did show statistically significant increases while plastic bags were the only category to show a negative trend, considered to be due to the implementation of a plastic bag charge in some regions around the North Sea (Maes et al., 2018).
While shore-line monitoring data appear to show no consistent temporal trends in macroplastic accumulation after the 1990s (Barnes and Milner, 2005; Barnes et al., 2009), the occurrence of plastics associated with wildlife does. For example, the percentage of kittiwake (Rissa tridactyla) nests in Denmark containing plastic debris increased from 39 to 57% between 1992 and 2005 (Hartwig et al., 2007), while the number of seals in California entangled in plastic debris and the percentage of prions (Pachyptila spp.) reported with plastics in their stomachs have largely shown steady growth since these records began (Ryan et al., 2009). More recently, a 60-year time-series (1957–2016) of marine plastics in the North Atlantic based on records of entanglement by trawls of the Continuous Plankton Recorder has shown a marked increase in macroplastic abundance especially since the 1990s (Ostle et al., 2019). Unfortunately, for microplastics, no similar long-term datasets exist and, in the absence of monitoring, paleoecological approaches, using the accumulation of natural archives such as lake and marine sediments, ice cores and peat sequences, are one of the only ways to assess temporal trends in the environment.
The Value of Contaminant Paleoecology
The paleoecological approach has been used to observe temporal trends for a wide-range of contaminants in many areas around the world including trace metals (Yang et al., 2010), fly-ash particles (Rose, 2015) and a large number of different organic chemicals such as organochlorine pesticides (Muir et al., 1995; Lin et al., 2012), brominated flame retardants (Yang et al., 2016), and pharmaceuticals (Kerrigan et al., 2018).
Paleoecology uses the properties of undisturbed natural archives and the law of superposition to observe and record environmental change over a broad range of historical scales from annual (Gajewski et al., 1997; Kinder et al., 2019) to millennial (Meyers and Lallier-Vergès, 1999). For lake systems, where the majority of this work has been undertaken, benthic sediments provide a means to determine the changes occurring both within the lake and its catchment as well as atmospheric deposition from local, regional and long-range sources. Lake sediments include not just records of contaminants and other stressors, but also the preservation of a broad range of biological remains from single-celled algae, such as diatoms, to invertebrates (e.g., chironomid head capsules; mollusc shells), plant pollen and macro-fossils (seeds, spores) to fish-scales. As a result, these natural archives contain a record of both stressors and biological response and so are powerful tools in exploring environmental change. However, while they can clearly show directions of change, i.e., increases or decreases in contaminant concentrations or changes in the abundance of different species, it is the use of dating techniques to provide robust chronologies that allows rates of change to be determined. For the microplastic time period (i.e., since c. 1950) (Figure 1) such chronological approaches are mainly radiometric (e.g., 210Pb, 137Cs, 241Am) and these can provide accurate dates to a sub-decadal resolution or better. Other approaches to sediment dating such as the counting of annual varves are clearly better than this but are only rarely present.
Microplastic concentrations have been reported in a number of environmental archives including lake (Imhof et al., 2013; Fischer et al., 2016; Vaughan et al., 2017), river (Klein et al., 2015; Hurley et al., 2018a; Peng et al., 2018), deep-sea and coastal sediments (Claessens et al., 2011; Van Cauwenberghe et al., 2013; Nor and Obbard, 2014; Woodall et al., 2014), as well as soils (Scheurer and Bigalke, 2018) and ice (Obbard et al., 2014; Peeken et al., 2018) and so there is clearly considerable potential. However, very few studies have considered using these records to observe changes in microplastic abundance and type through time and even fewer have also employed chronological techniques.
In 2011, Claessens et al. presented microplastic concentrations in beach sediments from four different depths from Groenendijk-Bad, Belgium showing an increase from 54.7 ± 8.7 to 156.2 ± 6.3 particles kg−1 dry sediment between 1993–96 and 2005–08. However, these dates were not derived from direct chronological measurements but rather from annual local deposition rates produced from coast-line maps. Similarly, Matsuguma et al. (2017) reported changing concentrations of microplastics in sediments from a range of locations in Asia and Africa including Tokyo Bay, the moat of the Imperial Palace in Tokyo, the Gulf of Thailand, the Straits of Johor in Malaysia and Durban Bay, South Africa. For most of these sites, microplastic concentrations were compared within just two or three sediment depths and, although they were not dated, the particles were allocated to a polymer-type by Fourier-Transform Infra-Red spectrometry (FT-IR) thereby providing information on changing sources at different, albeit unspecified, times. A more detailed profile (six undated sediment levels) were analyzed from a canal in Tokyo Bay. In Lake Ontario, Corcoran et al. (2015) reported increases in microplastic concentrations in surficial sediments (0–8 cm depth, no microplastics found below 8 cm). Although these sediment cores were also not directly dated, comparison with sediment accumulation rates from cores taken elsewhere indicated that the first presence of microplastics probably occurred between 1977 and 1997.
Very few studies have so far undertaken the analysis of microplastics on directly dated sediment cores. In 2019, Turner et al. reported on microplastic concentrations from a 210Pb-dated sediment core taken from an urban lake in north London, UK. Here, an increase in microplastic concentrations (number kg−1 dry sediment) and accumulation rates (number m−2 yr−1) was evident after the late-1950s and these were analyzed by Raman spectroscopy to reveal that the main polymer-type was polystyrene, while polyacrylonitrile and polyvinyl chloride fibers were also prevalent. Then, just a few months later, Brandon et al. (2019) presented microplastic accumulation rates for a varved marine sediment core collected from 580 m water depth in the Santa Barbara Basin, off the coast of California. Although polymer data through time were not reported, the microplastic accumulation rates showed an excellent agreement with trends in global plastic production, and increased rates, especially from the 1970s onwards, were driven mainly by microplastic fibers and fragments. Most recently, two further studies have been produced, both from China. First, Dong et al. (2020) produced a microplastics profile from Donghu in Wuhan, Hubei Province. Here, fibers were the only microplastic-type found to be present but their increasing concentrations, through the lake sediment record since 1960, showed a very good agreement with global synthetic fiber production. Second, Xue et al. (2020) produced a microplastic concentration profile from a sediment core taken from the Beibu Gulf in south China and found that peak concentrations occurred in the 1930s, although a presence of microplastics was recorded throughout the core, including basal sediments dated to c.1897. The presence of microplastics in these early sediments was attributed to bioturbation by burrowing invertebrates such as “peanut” worms (Sipunculus nudus) and lugworms (Arenicola marina). Clearly, such disturbances need to be taken into consideration when selecting both coring locations as well as appropriate cores for analysis, otherwise interpretation of temporal trends is exceptionally difficult or impossible. As these few studies show, there is enormous untapped potential for exploring natural archives to provide temporal trends and accumulation rates of microplastics in a range of environments. These can provide information on increasing risk to biotic, including human, health as well as providing their own chronological information. However, the derivation of robust microplastic data from these records also presents considerable analytical and interpretative challenges.
Microplastic Paleoecology in Different Natural Archives
A wide range of natural archives have been used to provide temporal records of environmental change including freshwater and marine sediments, ice cores, peat sequences, speleothems, tree-rings, corals, whale ear wax plugs and faunal (e.g., bird, bat) middens. More intermittent records have also been compiled from teeth, antlers and bird eggs as well as from herbaria and museum specimens. Not all of these archives have been used for microplastic records and a number would not be appropriate. Here, we consider only the more commonly used paleoarchives (Table 1).
Marine Sediments
As concentrations of microplastic contamination in deep-sea sediments from the Atlantic Ocean, Mediterranean Sea and Indian Ocean have been found to be up to four orders of magnitude higher than in surface waters, these profundal areas (more than 300 million km2) are likely to be a global sink for microplastic debris (Woodall et al., 2014). For example, Tekman et al. (2020) found that Arctic Ocean sediments contained microplastic concentrations 16,000 times higher than in the water column. Less disturbed than shallow waters and less exposed to storm water discharge, waves, tides, currents, landslides and dredging (Mulder et al., 2011), deep-sea sediments have a great potential for reconstructing the deposition histories of microplastic contamination. Marine sediment records have shown a close correlation between increasing worldwide plastic production and microplastic concentrations (Brandon et al., 2019) while comparisons between different locations may be applied to transport models in order to extrapolate microplastic distributions and to predict potential “hot-spots” of plastic deposition, as has already been successfully undertaken for surface waters (Law et al., 2010; Pagter et al., 2018).
The distribution and preservation of contaminants in marine sediment cores is determined by site-specific factors such as sedimentation rate and bioturbation (Johannessen and Macdonald, 2012; Outridge and Wang, 2015) and the mechanisms by which microplastics reach and deposit on the sea floor are still poorly understood (Gregory, 2009). If floating microplastic particles become denser due to heavy biofouling they may sink and be deposited as “marine snow” (Zhao et al., 2017; Porter et al., 2018). This comprises microaggregates of phytoplankton, organic matter and clay particles held together by extracellular polymeric material and sinking rates in marine systems range from 1 to 368 m d−1 (Alldredge and Silver, 1988). Therefore, deposition to some deep-sea locations may only occur several years after the microplastic particles originally entered the marine environment (Van Cauwenberghe et al., 2013). As a consequence, some deep-sea depositional systems characterized by very slow sedimentation rates are not likely to offer a high-resolution stratigraphy over short timescales. Very low deposition rates might compromise the possibility of estimating deposition and microplastic concentrations, even in undisturbed sediments, except on longer timescales. A potential solution to this issue might be to collect benthic samples from areas where overlying surface waters are highly productive, facilitating the sinking of microplastics to the seafloor via biofouling, ingestion, and formation of fecal pellets and enhancing sedimentation rates (Brandon et al., 2019).
Another factor affecting distribution of microplastics within marine sediments is bioturbation. This process of sediment reworking by living organisms (e.g., by lug-worms, Arenicola spp., which can live up to 70 cm below the sediment surface) (Claessens et al., 2011) alters sediment stratigraphies and can occur during or after deposition (Mulder et al., 2011). As a result, upper sediment layers potentially containing microplastics, could be partially or totally homogenized compromising the true temporal record (Claessens et al., 2011). To avoid such disturbance, areas of anerobic bottom water or low-oxygen marine basins should be chosen for sample collection. This minimizes the possibility of bioturbation and increases the likelihood of collecting undisturbed sediments containing continuous temporal microplastic records (Brandon et al., 2019).
Finally, while undisturbed deep-sea marine sediment cores may represent an excellent archive for analyzing long-term historical trends of microplastic accumulation, the provenance of microplastics depositing there is difficult to reliably predict as they may cover a very large source area. Almost all microplastics ending up in deep-sea sediments will have originated from sites located on the continental margin (Van Cauwenberghe et al., 2013) and ocean circulation dynamics will control both their vertical and horizontal transfer from these coastal regions to deeper areas where they ultimately sink (Galgani et al., 1995, 1996; Van Cauwenberghe et al., 2013; Woodall et al., 2014). The heterogeneity of microplastic abundances in sediments is a result of different densities, buoyancies and residence times in the water which are further affected by factors including wave-mixing, fragmentation and biofouling (Galloway et al., 2017; Erni-Cassola et al., 2019) as well as a wide range of potential sources (Woodall et al., 2014; Tekman et al., 2020). Consequently, while marine archives, collected from appropriate locations, may provide a useful tool for understanding long-term historical trends in microplastic contamination they are unlikely to be able to provide information on the precise origin of the microplastic debris accumulated there (Woodall et al., 2014; Tekman et al., 2020).
Lake Sediments
As with marine environments, lake sediments are likely to be a final sink for both low-density positively-buoyant and high-density negatively-buoyant microplastics (Barnes et al., 2009). Many synthetic polymers with densities less than that of water, such as polyethylene and polypropylene, will be buoyant as they enter a lake and will only deposit to benthic habitats once their density has increased due to biofouling (Andrady, 2011; Woodall et al., 2014), the “fecal express” (Cole et al., 2013; Setälä et al., 2014; Zalasiewicz et al., 2016) or via the adsorption of particulate matter to plastic surfaces (Frias et al., 2016). Although data are more sparse for freshwaters than for marine systems, these processes are likely to result in the substantial accumulation of microplastics in lake sediments (Woodall et al., 2014; Tekman et al., 2020) and research shows that microplastic concentrations in freshwaters and their sediments are comparable to those in marine environments (Corcoran et al., 2015; Ballent et al., 2016; Klein et al., 2018). Furthermore, as observed by Dong et al. (2020) and Turner et al. (2019), down-core variations in microplastic abundance and polymer-type can be observed in lake sediments and may reflect changes in plastic production and usage, providing a temporal perspective to our understanding of microplastic inputs to lakes which has, to date, been underexplored.
Although contaminant deposition in lake sediments is controlled by factors including lake hydrology and bathymetry, the small size and restricted, well-defined catchments when compared to oceans are likely to enable a better differentiation of local and regional hydrological pathways, and regional to global inputs from atmospheric sources (Fischer et al., 2016). Hence, catchment-scale microplastic assessments are possible for freshwater systems which are not possible for ocean sediments where microplastics from long-range transport from multiple catchments, sink and are deposited (Hidalgo-Ruz et al., 2012; Hardesty et al., 2017). As only an estimated 5% of the more than 350 million tons of plastic waste generated each year are directly discharged into the oceans (Xiong et al., 2018), lake sediments are not only generally more accessible for sampling than their marine equivalents, but are also closer to vast terrestrial sources. Furthermore, given that overall lacustrine sedimentation rates are typically an order of magnitude (or more) higher than in marine systems, where sedimentation rates of 1–10 cm Ka−1 are common, lake sediments are also likely to offer a good microplastic stratigraphy with high temporal resolution over relatively short timescales (Scholz, 2001).
As in marine environments, where organic aggregates influence the fate and sinking of microplastics, biofilm coverings and combinations of microplastics with organic matter also increase settling rates and accelerate the transport of microplastics to freshwater sediments (Möhlenkamp et al., 2018; Porter et al., 2018). Nutrient enrichment, particularly common in shallow lakes, can result in high primary productivity, increasing biofouling processes and accelerating microplastic deposition and burial (Kaiser et al., 2017). Furthermore, increasing microplastic stress has the potential to lower the resilience of shallow lake food-webs, increasing the probability of abrupt changes (Kong and Koelmans, 2019) and as a result shallow lakes are likely to be priority systems when assessing temporal trends in microplastic contamination.
Shallow waters tend to be better mixed by wind and water currents resulting in a more homogenous distribution of microplastics. By contrast, microplastic deposition in deeper lakes may be affected by lake stratification, resulting in the generation of a thermocline and waters of differing densities. Sinking microplastic particles which have densities similar to that of the epilimnion are likely to remain within this layer and accumulate at the thermocline (Fischer et al., 2016). As a result, the presence of a thermocline might play a key role in microplastic transport and retention within the water column (Zobkov et al., 2019) while seasonal stratification and mixing may affect microplastic sedimentation rates and distribution in sediments. The same may also be true for other aquatic systems where stratification occurs.
Bioturbation by benthic fauna also influences lake sediment microplastic stratigraphies. Tubificid worm bioturbation, for example, is associated with sediment reworking and the production of burrows (Brinkhurst et al., 1972; McCall and Fisher, 1980). In lake sediments, biological mixing of sediments is generally less significant than in marine habitats because of anoxic bottom waters or less active benthic communities (Robbins, 1978; Appleby, 2001), and careful site selection can pre-empt many potential issues. However, human disturbance of the sediment record is likely a greater issue for many freshwater records than for marine sites, especially for urban lakes. Here, even where sampling points are selected far from the shore there remains a higher potential for disturbance from a range of anthropogenic impacts, including dredging, construction and a wide range of catchment activities. These may result in an altered distribution of contaminants within sediments as well as compromised accumulation and chronologies (Dong et al., 2020). Although urban, shallow water systems can be difficult ones from which to recover undisturbed sediment cores, when they are obtained, they can provide valuable records of microplastic pollution as well as a wide range of other anthropogenic contaminants over the recent historical period (Turner et al., 2019; Dong et al., 2020).
Ice Cores
Ice cores extracted from Antarctic and Greenland ice sheets and from high altitude glaciers can provide important historical records of human activities (Gabrielli and Vallelonga, 2015). Aerially transported debris and contaminants deposited onto the surface are retained and accumulate as ice layers form (Lovett and Kinsman, 1990; Lei and Wania, 2004). Although, to our knowledge, no published research has yet produced microplastic temporal trends and accumulation rates in dated ice cores, due to their high accumulation rates they have the potential to provide very detailed paleo-environmental information on microplastic deposition especially for regions where other archives may be less available (Gabrielli and Vallelonga, 2015). While polar ice core records might be sufficiently remote from anthropogenic sources to provide reconstructions of hemispheric and global atmospheric contamination (McConnell and Edwards, 2008; McConnell et al., 2014), lower-latitude/high-altitude ice cores (i.e., alpine cores) are likely to be more indicative of regional pollution (Eichler et al., 2012; Gabrieli and Barbante, 2014; Uglietti et al., 2015; Beaudon et al., 2017).
Ice cores have been drilled worldwide since the 1950s in order to determine the history of atmospheric pollutants such as lead and mercury as well as other trace metals, organic compounds, radioactive species and black carbon (Gabrielli and Vallelonga, 2015; Gabrielli et al., 2020). Indeed, the major changes occurring in the chemical composition of the atmosphere since the beginning of the Anthropocene are well-recorded in ice cores extracted from polar regions and high-altitude glaciers (Gabrielli and Vallelonga, 2015). As most of these contaminants have been found everywhere from the Northern Hemisphere to Antarctica, it is likely that there are no glaciers or ice sheets where atmospherically-transported anthropogenic contaminants cannot be detected (Gabrielli and Vallelonga, 2015). Therefore, as microplastics are now largely considered to be both ubiquitous and atmospherically transported, ice core studies are also likely to provide archive information on microplastic fallout over the past 80 years.
The efficiency of snow at scavenging contaminants from the atmosphere combined with the high rates of snow accumulation at high-latitudes and altitudes provide the possibility of recovering long records of microplastics, characterized by a high temporal resolution (Hong et al., 2009; Gabrielli et al., 2020), especially where ice stratigraphy is continuous, and reworking processes at the surface such as wind erosion, re-deposition and summer melting are limited (Schotterer et al., 2004). Ice core chronologies may be produced by counting annual ice-layers, using the seasonal variability of stable isotopes and soluble ions, and/or the concentration profiles of seasonal species. Lead-210 may also be used for dating the more recent period (e.g., post-1900) (Döscher et al., 1996) and all these methods may be supplemented by the use of independent stratigraphic markers. These are typically chemical or particulate signals in the ice which identify major events, such as volcanic eruptions, aeolian dust deposits or, previously, atmospheric nuclear tests (Barbante et al., 2004; Gabrielli and Vallelonga, 2015).
Assessing the abundance of microplastics trapped in ice would not only provide an understanding of accumulation and dynamics of atmospheric microplastics but also the potential consequences associated with these contaminants being released back into the environment due to global warming and progressive ice melting (Obbard et al., 2014). The preservation and continuity of ice stratigraphy is critical to the use of ice cores as paleo-environmental archives (Schotterer et al., 2004). Increasing climate warming leads to summer melting, percolation and refreezing which alter depositional sequences and cause the loss of valuable historical information (Gabrielli and Vallelonga, 2015). However, even where ice sequences remain extant, the volume of meltwater available from each layer may limit their use in generating high resolution microplastics records and it is likely that to ensure reliable historical information, ice core records will need to be replicated with inevitable increases in fieldwork and analytical procedure costs (Jouzel et al., 1989).
Peat Sequences
Peatlands represent 3% of the continental area, covering ~5 million km2 of the Earth's surface (Gore, 1983). They are characterized by water at, or near the surface, anoxic conditions and specific vegetation, the decay of which leads to the formation and accumulation of the peat as well as to characteristic acidic conditions (Charman, 2002; Hansson et al., 2015). As a consequence, peats have low density, a high organic matter content (Lennartz and Liu, 2019) and a high porosity which facilitates water and solute movement (Quinton et al., 2009; Rezanezhad et al., 2016). Peatlands are defined as ombrotrophic only when their surface layers are supplied with nutrients by atmospheric sources, such as aerosols, rain, snow, fog and dust and are completely hydraulically isolated from groundwater (Damman, 1986; Shotyk, 1996).
Ombrotrophic peats record atmospheric inputs more directly than other continental archives such as lake sediments (Hansson et al., 2015) and are therefore important stores of historical information of both natural changes and human activities (Martínez-Cortizas and Weiss, 2002). Together with ice cores, ombrotrophic peat cores are the only archives which exclusively record atmospheric deposition at high resolution (De Vleeschouwer et al., 2010), but peats have the additional advantages of having a wider global distribution, being generally more accessible and easier to sample (Hansson et al., 2015). As a result, peats have been widely used to analyze historical changes in the atmospheric deposition of many trace metals including Pb, Ni, Cu, Zn, Hg, Co, and Cd, to produce high-resolution multi-metal chronologies (Martínez-Cortizas et al., 1999; Nieminen et al., 2002; Rausch et al., 2005; Allan et al., 2013). These have been cross-validated with other natural archives, including lake sediments, ice, and herbaria samples (Renberg et al., 2001; Hansson et al., 2015). However, as with ice cores, to our knowledge, no microplastic peat records have yet been published.
Peat cores are likely to be valuable archives of microplastic atmospheric fallout. As they tend to have higher accumulation rates than those of marine and many lake sediments (Gałuszka et al., 2017), peat cores are likely to provide a high-resolution history of microplastic atmospheric contamination and accumulation in the environment, covering a wide range of spatial scales from local to global (Martínez-Cortizas and Weiss, 2002). However, due to local variations in topography and vegetation, which might affect the retention efficiency of microplastic deposition, multiple cores at different sites are likely to be required (Bindler et al., 2004; Allan et al., 2013; Souter and Watmough, 2016). Furthermore, in order to directly relate microplastic concentrations in peat cores to atmospheric deposition, coring locations should be selected to minimize post-depositional remobilization (Martínez-Cortizas and Weiss, 2002). While major disturbance from human activities, such as peatland drainage for agricultural practices may be avoided in this way (Holden et al., 2006) post-depositional remobilization of microplastics as a result of historical and contemporary root growth may be more difficult to avoid (Laiho et al., 2014). Although no records of microplastics in dated peat cores have yet been published, peatlands are likely to have a great potential as a sink for microplastic atmospheric fallout as their high porosity should enhance retention and accumulation of microplastics deposited to surface layers. Furthermore, as peatlands are often located in transition zones connecting soils with aquatic systems (e.g., coastal wetlands), they may also act as a source of microplastics to adjacent systems (Lennartz and Liu, 2019). Therefore, assessing microplastic contamination in peatlands might not only help determine high-resolution spatial and temporal patterns of deposition, but also lead to a better understanding of the potential for microplastic exchange between ecosystem compartments.
Analytical Challenges for Microplastic Paleoecology
While there is still no fully agreed definition for microplastics, a further concern is the difficulty in making comparisons between studies due to the lack of standardization in analytical techniques. Full comparability will only be possible when units of abundance, methodologies for extraction and identification are standardized and harmonized. While such problems have been reported elsewhere, these issues will also be key to comparisons between paleoecological studies. Therefore, although a detailed analysis is beyond the scope of this current paper, we briefly highlight the main issues here.
Standardization of Extraction Methodology
Microplastic extraction from solid matrices, such as sediments, is usually performed by density separation, agitating the sample in saturated salt solutions (Crawford and Quinn, 2016). The higher the density of these solutions, the more polymers may be separated. However, the medium for density separation varies widely across studies from 1.0 to 1.2 g cm−3 for sodium chloride (NaCl) up to 2.1 g cm−3 for aqueous solutions of sodium polytungstate (Käppler et al., 2016; Turner et al., 2019). Other solutions, including sodium iodide (NaI), zinc chloride (ZnCl2) (Van Cauwenberghe et al., 2015) and zinc bromide (ZnBr2) (Quinn et al., 2017), have intermediate densities normally ranging between 1.6 and 1.8 gcm−3. This wide range of solutions and densities, coupled with differences in methodologies between laboratories, results in the reporting of both different total concentrations and polymer assemblages. This makes comparisons between studies challenging and a move toward standardization of extraction techniques is required.
A further challenge for the extraction of microplastics from many lake sediment cores, and certainly for future studies involving peats, is the removal of organic material and this may be by chemical or enzymatic means (Li et al., 2018). Many studies have used hydrogen peroxide (H2O2) at different strengths (10–35%), temperatures and time intervals (Nuelle et al., 2014; Erni-Cassola et al., 2017; Li et al., 2018; Mai et al., 2018; Prata et al., 2019) while alternative approaches use acid (Avio et al., 2015; Dehaut et al., 2016) or alkaline digestions (Dehaut et al., 2016; Hurley et al., 2018b). While degradation of some polymer types has been observed following these chemical extractions (Nuelle et al., 2014; Dehaut et al., 2016), Fenton's reagent, an oxidant involving H2O2 in the presence of a ferrous catalyst (Fe2+) at room temperature, does not appear to affect plastic polymers (Hurley et al., 2018b). Enzymatic approaches appear to be an effective technique when applied to small samples (i.e., biological tissues), but are expensive for large samples with high organic matter content and potentially require a range of enzymes to digest different organic compounds (Hurley et al., 2018b).
Microplastic Identification
While many studies, especially earlier ones, have used visual sorting only, this may lead to a great under- or over-estimation of microplastic contamination and the use of spectroscopic approaches such as infra-red (IR) or Raman analysis greatly increases reliability (Hidalgo-Ruz et al., 2012; Eriksen et al., 2013; Mendoza and Balcer, 2019). However, a major challenge for microplastic paleoecology when using spectroscopic methods is that reference databases (Zarfl, 2019) typically only include “virgin” plastics and these do not easily match those from environmentally exposed, degraded and aged materials. Spectra from polymers at different stages of degradation, or containing additives which are not recognized by commercial plastic libraries, need to be included in reference databases for environmental analyses (Ribeiro-Claro et al., 2017; Silva et al., 2018; Zarfl, 2019). Primpke et al. (2020) have begun this by developing free semi-automated software that allows the matching of FT-IR spectra with a library collected from degraded microplastics.
Validation
Validation of microplastic extraction methods is a fundamental step that is currently often neglected (Zhao et al., 2018). Recovery tests involving spiked microplastics in environmental samples, preferably including different colors, sizes, composition and densities, should be included with extractions in order to estimate recovery efficiencies and confidence intervals for the methodology employed. Furthermore, as spectroscopic techniques are time consuming, it is common practice to analyze either a proportion (10–20%) of the total particles visually identified as potential microplastics (Mendoza and Balcer, 2019) or a fractional area (25%) of a final filter when visual sorting has not been performed (Mintenig et al., 2017; Redondo-Hasselerharm et al., 2018). However, as total numbers of identified plastic polymers vary considerably between studies, the proportions of particle numbers or filter areas analyzed should be reported in order to aid comparison while a sufficiently high proportion needs to be analyzed to provide statistical significance.
Units of Measurement
Studies on microplastic contamination use a wide range of different units for microplastic quantification and show very heterogeneous concentrations, which may differ by several orders of magnitude (Klein et al., 2018). Some units may over-represent sample size, leading to an exponential increase in values when upscaling calculated microplastic densities to larger volumes or areas (Mendoza and Balcer, 2019). For paleoecological studies, this may be less of an issue but standardization is still required. For natural archives such as sediments and peats the numbers of particles per unit dry weight (dw), expressed either in g or kg is proposed (Ng and Obbard, 2006). Furthermore, the use of reliably dated archives allows the calculation of microplastic accumulation rates or “fluxes,” as well as concentrations, and the two should both be reported together whenever possible. The use of fluxes takes into account variation in archive accumulation rates within a sequence that may increase or decrease concentrations and, for microplastics, should be reported in units of numbers of particles per area and time e.g., n m−2 yr−1 (e.g., Brandon et al., 2019; Turner et al., 2019). This results in more comparable data, both in input rates through time within a single archive, but also between sites.
For ice cores, microplastic concentrations could be reported as number of particles/L of filtered, melted ice as often used for smaller volume water samples (Mendoza and Balcer, 2019). Additional information on concentrations of size-classes and polymer-types should be given where possible (Koelmans et al., 2019; Mendoza and Balcer, 2019). These are important as differing approaches to methodology and identification affect lower-size detection limits as well as the polymer assemblage extracted.
Contamination
The control of contamination is fundamental to the accurate analysis of microplastics in environmental samples particularly where concentrations are expected to be very low, either due to isolation from emission sources or where rapid accumulation rates dilute inputs. For example, a combination of these factors might be expected to occur for ice cores in polar regions. For paleoecological studies, the potential contamination of older samples is especially important not only because microplastic concentrations are at their lowest, but also because a first presence may be used to provide stratigraphic information (see below).
To prevent contamination from plastic core tubes, the outer 1 cm of each sediment layer can be removed during extrusion (Matsuguma et al., 2017). The use of aluminum tubes may avoid this core-trimming, although their lack of transparency can be problematic with regard to assessing the quality and quantity of the retained material. Similarly, the outer layers of ice cores, which could be contaminated during drilling, may be removed mechanically in order to obtain a “clean inner core” (Candelone et al., 1994).
In the field, all available measures must be taken to minimize contamination, for example by sampling upwind of other activities, the use of nitrile gloves while handling cores, the avoidance of plastic equipment as much as possible and the use of exposed filters during coring activities to determine airborne contamination during sample collection (Kanhai et al., 2020). As contamination can also occur during sample processing, it is extremely important to avoid the use of plastic tools whenever possible during subsampling. For example, aluminum extrusion heads may be used together with metal implements to slice the core into layers. For ice cores, all analytical procedures must take place in ultraclean laboratories, where work areas and equipment must be washed with filtered Milli-Q water between the processing of different core subsections (Barbante et al., 2004; Kanhai et al., 2020). Methodological blanks should be included regularly to detect potential contamination during the processing of sediment or melted ice samples, and clean filters left on work areas to check airborne contamination (Kanhai et al., 2020). Cotton, instead of synthetic, laboratory coats should be worn during sample processing, but attention should also be paid to potential self-contamination from synthetic clothes during sample collection. Scopetani et al. (2020) found that 23% of fibers detected in environmental samples produced FT-IR spectra matching the cotton worn by personnel during sampling. Higher numbers of fibers were found in samples where collection was associated with higher physical effort and movement, and longer exposure to air. To help eliminate these contaminants from further consideration, it may be useful to create a library of FT-IR spectra for fibers collected from laboratory coats and clothing worn during fieldwork, as well as fragments of any plastic tools used during field and laboratory activities. Comparison of the spectra obtained from plastic particles in samples against those in the spectra library could then help identify whether contamination has occurred.
The Taphonomy of Microplastics
Microplastics have now joined the ranks of the numerous stratigraphic indicators of human activity stored in natural archives. Like other anthropogenic paleoecological signatures, their final occurrence in depositional settings are a result of a myriad of human and natural processes, related to their production, utility, composition and transport to burial. However, unlike more classical paleoecological indicators that are used to interpret human activity (e.g., pollen, diatoms, invertebrate micro-remains, charcoal) our understanding of how ecological and environmental processes influence the final record of microplastics in natural archives is still to be determined. Though the potential historical occurrence and environmental abundance of plastics has been assessed by collation of development, production and usage data (Zalasiewicz et al., 2016) the release of plastic waste into the environment has not been systematically monitored. If we are to expand the use of sedimentary microplastics as a reliable archive of plastic use and waste emission, as well as apportion sources, we need to consider environmental transport and depositional factors that determine or skew the accumulating record.
The study of plant, animal and human paleoecology deals with the issues of representation, bias and differential preservation through the study of taphonomy. With a few exceptions, the majority of fossil assemblages are understood to have been intensely modified by taphonomic processes (Benton and Harper, 1997). Taphonomic processes affecting microplastics are therefore in conflict with the uniformitarian assumption that a microplastic record in an environmental archive faithfully represents its historical record of production and disposal. Without a greater understanding of taphonomic processes, microplastic sequences extracted from sediments, peats and ice cores will provide a distorted, even biased, historical narrative of the changing abundance and composition of plastic waste in the environment. Conversely, along with other fossils, the presence of microplastics and their taphonomic data add to our understanding of sedimentary processes operating in depositional environments.
The principal taphonomic considerations for microplastics is the interplay of (i) their high resistance to environmental influences, leading to extremely low degradation and long residence times (Klein et al., 2018) and (ii) the compositional and structural mixture of polymers released into the environment. A difference in the age of the sediment sample and the age of “death” (or release of fossil material to be preserved) is usually to be expected. For benthic or planktonic lifeforms and atmospherically transported materials, this time difference may be relatively small following transport through the atmosphere and /or water-column. With greater distances, or time taken to reach a depositional setting, materials have a greater potential for temporary storage and being re-worked en route, resulting in their eventual burial with sediments inconsistent with the age of that “fossil.” Producing a robust interpretation of past environments from a fossil record is therefore complex. Even for a traditional paleoecological discipline such as pollen analysis, that has been used globally and intensively for many decades, it is only comparatively recently that vegetation reconstruction from fossil records has become more quantifiable and objective (Davis, 2000).
Working in parallel to processes of death/release and time/distance to burial is the resistance of fossils to degradation. Less resistant forms will not survive being transported, stored and reworked, often leading to a bias in more resistant, transportable or locally dominant forms in sediment sequences e.g., Pinus pollen grains (Wiltshire, 2006). Paleolithic stone tools provide a good example of durable man-made materials that can upset the normal rules of stratigraphic succession. Lithic remains create more complex scenarios due to their durability and survive being eroded and transported from primary to secondary contexts (Barham et al., 2015; Archer et al., 2020). Similarly, although information can be obtained from analysis of durable stone tools and their contexts, what we know about prehistoric human life is greatly enhanced when exceptional preservation allows remains such as wood and other organic remains to survive, e.g., Neanderthal string (Knight et al., 2019; Hardy et al., 2020). For stone tools and microplastics alike, the same consequences of distance from source, durability, reworking and movement from primary to secondary contexts apply to correctly interpreting their depositional assemblage.
Although we have a well-documented history of plastic invention and usage, it will be many decades before early-mid twentieth century plastics can be ruled out from occurring in contemporary basins due to reworking of “natural” (soils, floodplain sediments) or anthropogenic archives, e.g., eroding coastal landfills (Brand et al., 2018). This lag not only provides the potential for older microplastics to recur, but also blurs the first occurrence in sequences due to the rapidity of polymer inventions. This is further exacerbated by the time taken to generate microplastics from macroplastic debris ether in situ or en route. This is discussed more fully below. Hence, in poorly dated, slowly accumulating stratigraphic sequences downstream of urban areas, a potential technological chronostratigraphy of plastics may well be lost.
Taphonomic Processes Affecting Microplastic Particles
Although self-selective due to the density and form of particles capable of becoming airborne, the atmosphere allows minimal delay between the production, usage and transport of microplastics to their deposition in archives. Microplastic fibers and dust-sized particles may be transported over at least regional, and possibly global, scales (Dris et al., 2016; Bergmann et al., 2019) while larger micro- and macroplastics may be dragged, saltated or become airborne only if wind and landscape conditions allow (Zylstra, 2013; Dris et al., 2016; Šilc et al., 2018; Rezaei et al., 2019). With less catchment influence, remote, atmospherically-dominated sites have long provided essential global and regional paleoecological data (Birks, 2019), however, for microplastics even here more proximal contamination has been found to be significant (Free et al., 2014; Zhang et al., 2016; Miller et al., 2017). Quantifying long-range atmospherically-transported vs. local hydrological plastic inputs is a challenge but detailed statistical analysis of morphometry and composition may have the potential to differentiate between sources at remote sites or those isolated from wastewater inputs.
Microplastics moving toward depositional archives via rivers, glaciers and air currents are an agglomeration from multiple spatial and temporal sources, mirroring natural sedimentary particles. Like sediments, microplastics enter from both point and diffuse sources, or are created by the breakdown of larger plastic particles also being transported, and therefore occur as bedload, in suspension and at the near-surface (buoyant) depending on their density and shape (Morritt et al., 2014; Horton et al., 2017; Kooi et al., 2018). Microplastic particles within bedload have a higher potential for temporary in-channel sediment storage when flow velocities decrease, whereas buoyant particles during high-flow events have a greater potential of being transported into low-flow, vegetated areas (Yao et al., 2019). Buoyant, lower density plastics are also affected by wind-wave conditions; sometimes oblique to bedload flow paths, e.g., in estuaries (Browne et al., 2010) and therefore may be circulated for longer, while other materials sink (Lebreton et al., 2019). Microplastics are also added during flow by repeated chemical and structural degradation of macroplastics during transport, e.g., UV and physical degradation of river plastics trapped by obstructions (Williams and Simmons, 1996), although quantification of this process contributing to the total pool of microplastic remains limited (Castro-Jiménez et al., 2019). During flow downstream, microplastics move between bedload and suspension (Hurley et al., 2018a) depending on flow rates and, when conditions allow, may be temporarily stored (Tibbetts et al., 2018).
Even within depositional settings microplastic assemblages continue to be spatially and temporally complex, due to the interaction of physical, chemical and biological factors affecting their burial. The route to burial is not straightforward for any particulate entering a depositional environment; they are rarely homogenous sinks, with internal flows connecting areas of higher and lower rates of deposition. The controlling factors of density and durability that control the distribution of plastics in environmental flows continue, but upon entering lentic, low energy settings, physical degradation is reduced, and chemical and biological processes can take precedence.
The boundaries between high and low energy conditions do not often occur abruptly, and are usually connected by transitional environments, such as floodplains, deep water channels (Kane et al., 2020), estuaries, shorelines and coasts. Both micro- and macroplastics in these settings continue to be re-worked, temporarily stored and released, contributing to the overall amount of microplastics in the environment. The common occurrence of considerably aged plastics in coastal systems; “Plastic bottle washes up looking 'almost new' after nearly 50 years at sea” (Lyons, 2018); “Crisp packet from the 60s found washed up on beach” (Byrne, 2019) and “Plastic bag found in Sunshine Coast waterway could be up to 40 years old and it's just the tip of the iceberg” (Mapstone, 2019); highlight the ability of plastics to remain in these transitional environments for considerable periods of time (years to multiple decades). Plastics identified by production dates as many years/decades old in scientific surveys of buried, surface and buoyant plastics (Hoffmann and Reicherter, 2014; Sander, 2016; Lebreton et al., 2018) support the idea of a long-term build-up of anachronistic microplastics now found in depositional settings. Modeling of transport and removal of buoyant plastic from the surface ocean predicts that most of the plastic mass that has entered the marine environment since the 1950s has not disappeared by degradation, but is stranded or settled on its way to offshore waters, possibly slowly circulating between coastal environments with repeated episodes of beaching, fouling, defouling and resurfacing (Lebreton et al., 2019). This “accumulation and slow release” loop will likely have occurred at different scales, since the mid-twentieth century at the margins of depositional basins globally. The implication of this is that without independent dating of individual particles, a paleoecological assemblage of microplastics in a sediment sequence is best considered as time cumulative. Aside from the stratigraphic “first occurrence” of invented plastics (see below), microplastic types and volumes occurring in stratigraphic intervals should be considered anachronistic. It is now perhaps too simplistic to continue comparing global production of plastic data (Plastics Europe, 2016; Geyer et al., 2017) against the abundance of fragments found in monitoring and sediment studies (Thompson et al., 2004; Claessens et al., 2011; Willis et al., 2017; Brandon et al., 2019) without considering that microplastic totals within defined time slices also contain historical releases.
Physical models using microplastic size, composition and density have made significant improvements to our understanding of microplastics in the environment; revealing non-steady state transport and long-term cycling between storage and release and mechanisms to explain the preponderance of types of plastic waste in certain locations. The highly efficient, spatially restricted sorting of macroplastic waste by size and composition is evident at channel margins, strandlines and beaches globally. Subtle changes in shape (e.g., handedness of sneakers as flotsam), may direct their orientation to wind/currents and their shoreline accumulation (Ebbesmeyer and Scigliano, 2009). There is a paucity of data describing how subtle differences in form (e.g., spherical vs. film) and polymer composition affect the spatial variability of microplastics found in depositional settings, but sorting by wind and water currents clearly occurs (Corcoran et al., 2015; Fischer et al., 2016; Su et al., 2016; Vaughan et al., 2017; Yao et al., 2019) as well as entrainment with sediment matrices of similar density and size (Pietrelli et al., 2017; Haave et al., 2019).
Biological Taphonomy
The interaction of plastic waste with organisms in the environment has long been recognized, with studies of plastic ingestion and entanglement of seabirds and cetaceans, elevating global plastic waste to the forefront of conservation concerns. Understanding the impact of plastics on organisms has therefore been a driving cause for microplastic research, due to detrimental effects of ingestion and potential ontogenic accumulation of plastic-associated chemicals. Due to their durability, microplastic particles have a high potential for circulating through trophic levels. However, how much of an effect biological processes have on the final stratigraphic record of microplastics is little understood, but from studies of the interaction of organisms and plastics in the environment, we can identify likely taphonomic factors.
As soon as plastic waste is emitted, biological activity is intrinsic to its alteration and accumulation. In low energy environments, biological processes can become central, determining capture, sinking, burial and re-working (see sections above on aquatic archives) (Figure 2). Plastic surfaces are quickly (hours to days) colonized in aquatic environments by a diverse microbial community dependent on location, season and substrate (i.e., type of plastic) (Amaral-Zettler et al., 2020). Biofilm formation and algal colonization change surface chemistry characteristics, influencing UV and chemical degradation, while biofilm-induced particle clumping leads to enhanced sinking rates (Michels et al., 2018). The sorting effects of preferential biofilm development on some plastics are conveyed to higher trophic levels by consumption of biofilms and microplastics by invertebrates, e.g., rasping and grazing by gastropods (Weinstein et al., 2016; Vosshage et al., 2018). Physical and chemical predilection of biofilm formation on some plastics compared with those durable to microbial degradation drives a sorting gradient to separate polymers (Amaral-Zettler et al., 2020); sustaining continued transport and distribution in some while enhancing clustering and sinking in others.
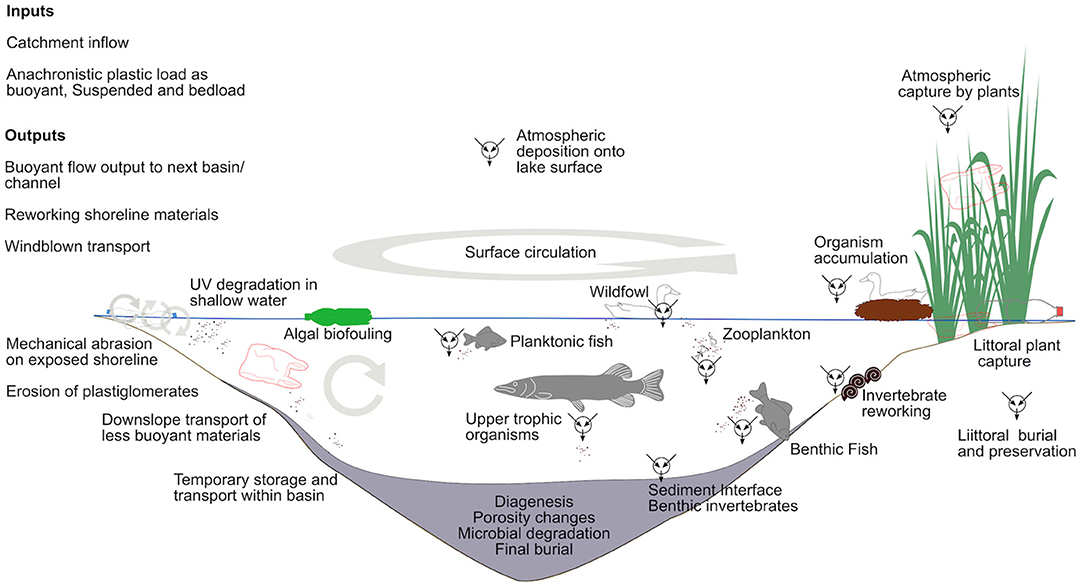
Figure 2. Indirect transport of microplastics to a simple aquatic basin and sediment sequence. Plastic waste is transported to and broken down by interacting physical, chemical, and biological processes.
Primary production in the form of vegetation growth is effective at capturing plastics at the periphery of depositional basins. Films and fibers may be tangled in stems and branches intercepting flow (i.e., the “Christmas tree effect”; Williams and Simmons, 1996) and sorting plastics across the range of capture and energy conditions found in freshwater and coastal wetlands (Ivar do Sul et al., 2014; Li et al., 2019; Yao et al., 2019; Helcoski et al., 2020). Variability in growth rate, stand-density, water-level changes from flooding or tidal regime affect microplastic capture and hence abundance, leading to spatial and temporal variability of microplastics accumulated over time, irrespective or additional to emission inputs.
Microplastic accumulation by primary trophic levels is followed by secondary consumption by invertebrates in the water column (plankton) as well as by detritivores and filter-feeders in benthic habitats. Due to the basin-scale volumes of water and suspended material able to be processed over time by plankton and benthic invertebrates, any selection of microplastics due to feeding strategy, will have a taphonomic effect on what reaches the sediment surface by sinking or benthic incorporation of fecal matter. How much of an effect particular feeding strategies or abundance of filter feeders have had on historical microplastic accumulation is poorly understood and ingestion studies have typically used concentrations far higher than realistic environmental levels (e.g., Katija et al., 2017; Scherer et al., 2017). Measurements of microplastic concentrations in zooplankton indicate that concentrations of ingested plastic is a positive function of available plastic and inversely related to particle size (Desforges et al., 2015) but more experimental work (Aljaibachi et al., 2020; Redondo-Hasselerharm et al., 2020), comparative monitoring, and sediment studies from areas with contrasting zooplankton and benthic ecosystems are clearly required (Su et al., 2016; Naidu et al., 2018).
Feeding strategies, trophic level and existing environmental concentrations continue to determine microplastic ingestion in higher organisms. Selective feeding, based on size and color (Martí et al., 2020), by planktonic fish will have a measurable effect as will non-selective feeding e.g., benthic fish at the sediment water-interface (Sanchez et al., 2014; Baldwin et al., 2020). Increased longevity (multiple years to decades) and trophic position of wildfowl increases their potential to incorporate microplastics from multiple sources and vectoring to the sediment via feces (Reynolds and Ryan, 2018).
Finally, as observed for other contaminants including a range of trace metals (Brimble et al., 2009), biovector transport may be an additional transport mechanism by which microplastics are transferred between environments. Microplastics accumulated by anadramous fish such as salmon, feeding in the oceans over periods of years, may be transferred to terrestrial headwaters as the fish return to spawn and then die. Similarly, seabirds feeding at sea, will accumulate microplastics themselves or transfer them to chicks, in which they are accumulated or released via feces to the coastal terrestrial environment. Furthermore, seabirds transfer macroplastic to terrestrial environments by collecting marine plastic debris and using it as nesting material and the same has been observed in freshwaters (e.g., Vaughan et al., 2017). A Northern gannet (Morus bassanus) colony of 40,000 birds on Grassholm, in Wales, UK, included a mean of 470 g of plastic debris in each nest resulting in an estimated colony total of more than 18 tons (Votier et al., 2011). Although biological activity is ubiquitous in depositional settings (Figure 2) and the interaction with plastics and microplastics is easily conceived, our lack of basic knowledge regarding biological processes and their interaction with chemical and physical factors on microplastic deposition, currently limits our understanding of organism bias on the paleoecology of plastics.
Microplastics as Stratigraphic Markers
Given the issues surrounding anachronistic microplastics in the environment as a result of differing taphonomies, as well as the various strengths and weaknesses of natural archives from which they might be extracted, there is a need to consider the role of microplastics as stratigraphic markers. In particular, it has been suggested that they may play a role in defining the start of the proposed Anthropocene Epoch, even though chronologically constrained historical records of microplastics are currently remarkably sparse.
The current internationally agreed method for defining chronostratigraphic boundaries is via selection of a Global Boundary Stratotype Section and Point (GSSP) as a physical reference level for a geological time boundary. The process of deciding on a lower boundary of the Anthropocene is complex and requires an initial selection of a primary marker and, ideally, auxiliary markers that support a global correlation (Waters et al., 2018). Different from any geological unit previously determined, the Anthropocene hinges more on effects than on cause (Zalasiewicz et al., 2019). This is particularly relevant for microplastics since these materials may be considered not only as environmental pollutants, but also as contributors to the character of recent (post mid-twentieth century) strata (i.e., plastic-rich sediments) (Zalasiewicz et al., 2016). Furthermore, in contrast to some other organic and inorganic pollutants that are also considered as potential markers of the Anthropocene (i.e., PAHs, metals), microplastics (or their constituent plastic polymers) have the advantage of being exclusively anthropogenic in nature, which means there are no naturally occurring background levels in the environment.
When compared to macroplastics, microplastics have a greater potential to spread and be distributed over wider areas, which makes them potentially globally correlatable within sedimentary layers. Therefore, they have a greater potential to become auxiliary markers for the Anthropocene boundary. However, microplastics have yet to be identified within some natural archives and, as described above, this may not be straightforward. In general, independent of the environmental matrix (water, sediment, biota), identifying microplastic in the small size ranges (particularly <100 μm) that will be transported over longer distances, is particularly challenging and requires care to extract particles and avoid external contamination (Turner et al., 2019; Enders et al., 2020). In addition, the characteristics of the archives themselves will add a layer of complexity and challenge to their stratigraphic interpretation.
In the Anthropocene context, archives need to be varved, or accurately dated and undisturbed, to allow reliable correlations between microplastic (or polymer) concentrations or fluxes and create a reliable microplastic deposition profile. Specific polymers (or occasionally entire plastic objects; Zalasiewicz et al., 2016) are potentially correlatable since they were invented, produced and discarded at different times. For example, when considering the most prevalent polymers (i.e., PE, PP, PVC, PET, and PS; Geyer et al., 2017) there are sometimes decadal gaps between their first creation and their subsequent production at large scales (Andrady and Neal, 2009), when they may be expected to be found in deep marine sediment layers for the first time. On the other hand, relatively modern polymer types are expected to be found only in more recent sediment layers, which will accumulate all polymer types currently in use (Figure 3) as well as older microplastics delayed en route to the same depositional environment. Therefore, while the increasing abundance of microplastic particles in natural archives over the last <70 years may indicate Anthropocene-related strata (see Abundance zone 2 in Figure 3), it is the first presence of polymer-types in stratigraphic layers (see Abundance zone 1 in Figure 3) which may potentially provide a physical reference marker for the onset of the Anthropocene Epoch.
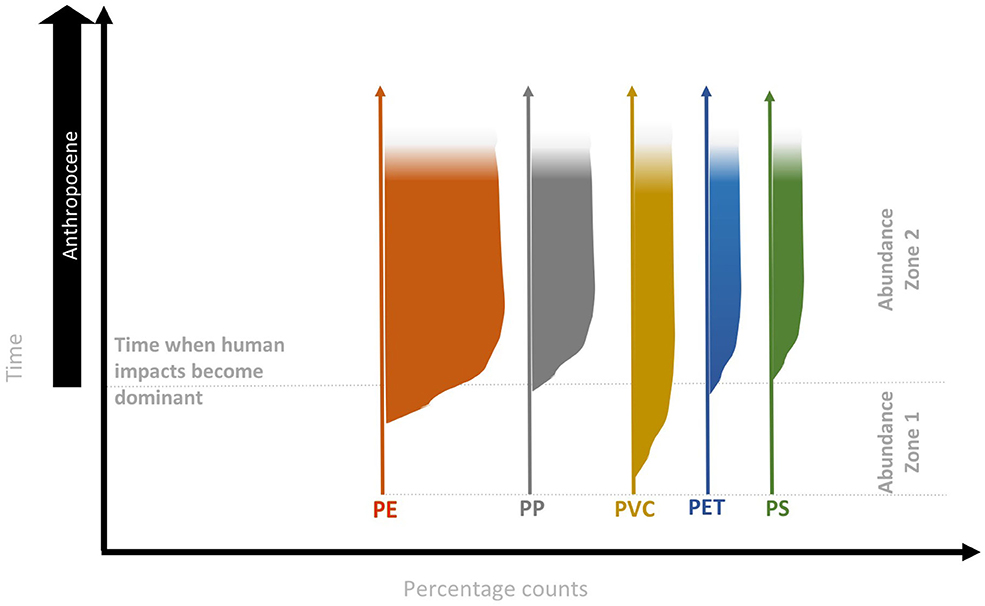
Figure 3. Microplastic potential abundance zones and their utility for defining the Anthropocene. Abundant zones are based on the relative percentages of individual polymer species (herein represented by production rates of PE, PP, PVC, PE, and PS) and can potentially be used to characterize the Anthropocene strata. Original figures and complete discussion on the biostratigraphy of the Anthropocene are published in Barnosky (2014).
Microplastics in the environment occur with a wide range of shapes (Frias and Nash, 2019). Microbeads, originating mainly from cosmetic and personal products such as exfoliants and toothpastes, are expected to occur in differing abundances and accumulate in sediments at significantly different times in the developed northern hemisphere when compared to the less developed and less populated southern hemisphere. Therefore, microbeads may be irregularly distributed, which makes this specific particle-type less suitable as a globally synchronous stratigraphic marker. By contrast, fibers appear to be ubiquitous over a range of habitats (Dris et al., 2016; Bergmann et al., 2019) and are also expected to occur in sediments in a more synchronous way on a global scale, independent of sources. Fibers are incredibly mobile, often being the only microplastic particle identified in lake sediments and especially where atmospheric deposition is the main route of microplastic accumulation. Hence, abundances of microplastic forms, rather than total concentrations may have greater stratigraphic utility.
The Preservation of the Microplastic Record
Microplastics comprise hundreds of different polymer-types (Andrady, 2011) but they all have long polymeric chains that are composed mostly of carbon (e.g., polypropylene (PP) and polyethylene (PE) are >90% carbon-based) (Rillig, 2018). These high-molecular-weight organic chains resemble the long polymeric chains in persistent organic fossils such as wood, spores, pollen and graptolites (Zalasiewicz et al., 2016). Therefore, even if microplastic particles themselves do not endure, these polymers are expected to be preserved in sediments as trace technofossils. Although many studies imply that plastic longevity in the environment is at the scale of “centuries to millennia” under specific environmental conditions (Gregory and Andrady, 2003), these are often based on short-term laboratory experiments and should be interpreted with caution. What is clearer is that solar ultraviolet (UV) light is by far the main driver of plastic fragmentation, while the absence of UV light combined with low temperatures and a lack of oxygen may facilitate microplastic preservation in the deposited sediments. Deep ocean sediments may therefore offer the best conditions for long-term preservation and this is another key criterion in the selection of an appropriate stratigraphic marker for the Anthropocene.
Microplastic polymer types or “species” such as PE or PP in natural archives, may be able to fulfill a role similar to that played by fossils in specific biostratigraphic units. Within these units, fossils help to establish the relative age of specific strata at different locations (Barnosky, 2014). As stratigraphic markers, microplastics or polymers could be used as, not bio-, but chemostratigraphic units and therefore as a means to correlate between strata, be this indicative of the Anthropocene or other time periods (Ivar do Sul and Labrenz, 2020). The long polymeric chain N-acetylglucosamine, a derivative of glucose considered to be a component of chitin, is known to be preserved in graptolite fossils for 500 million years. However, while plastic polymers are clearly long-lived on human time-scales, knowledge of their potential fossilization and final preservation remains lacking. While natural examples suggest that such chemical preservation may seem likely, it is less clear whether microplastic particles themselves could be preserved as permanent casts and molds in lithified rocks (Leinfelder and Ivar do Sul, 2019) over such vast time-scales as occurs for biological micro-fossils.
Concluding Remarks
Rapidly increasing knowledge on the distribution of microplastics across a broad range of environments suggests that, to all practical extents, they are likely to be ubiquitous. In particular, microplastic fibers are easily transported through the atmosphere and as a result, it may be expected that a range of natural archives from lake and marine sediments to ice and peat cores would contain historical records of their deposition. Therefore, although no microplastic records have yet been published for peats and ice cores, it seems probable that microplastic fibers will offer the best opportunity as a global stratigraphic marker.
As with other environmental contaminants, the paleoecological records of microplastics will be invaluable in determining the scale and extent of contamination at a range of geographical and temporal scales. They will allow directions of change (increasing or decreasing inputs) to be assessed as well as the rates at which that change is occurring. However, while there is considerable potential, data remain sparse and much remains to be done to explore these records and their possible role as stratigraphic markers.
What is clear is that the science of microplastic paleoecology is currently still in its infancy. Microplastics were not mentioned within the “50 priority research questions in paleoecology” produced only a few years ago (Seddon et al., 2014) and while such data are now being generated, little attention is currently being paid to the complexities of their interpretation. In particular the taphonomy of microplastics, i.e., the processes affecting their transport to, and deposition within, natural archives needs to be understood. This will allow a better understanding of microplastic records and their use, while conversely allowing microplastic records to contribute to our knowledge of depositional processes. Paleoecology has a rich history of interpreting temporal data and many lessons for the interpretation of microplastics in natural archives may well be learned from these more established techniques.
Data Availability Statement
The original contributions generated for the study are included in the article/supplementary material, further inquiries can be directed to the corresponding author/s.
Author Contributions
All authors conceived, wrote, edited, and reviewed this manuscript.
Conflict of Interest
The authors declare that the research was conducted in the absence of any commercial or financial relationships that could be construed as a potential conflict of interest.
Acknowledgments
CB acknowledged the support of the Natural Environment Research Council as part of the London NERC DTP (Grant no. NE/L002485/1). This paper contributes to the research of the Anthropocene Working Group (AWG), which is a working group of the Sub-commission on Quaternary Stratigraphy of the International Commission on Stratigraphy.
References
Aljaibachi, R., Laird, W. B., Stevens, F., and Callaghan, A. (2020). Impacts of polystyrene microplastics on Daphnia magna: a laboratory and a mesocosm study. Sci. Total Environ. 705:135800. doi: 10.1016/j.scitotenv.2019.135800
Allan, M., Le Roux, G., De Vleeschouwer, F., Bindler, R., Blaauw, M., Piotrowska, N., et al. (2013). High-resolution reconstruction of atmospheric deposition of trace metals and metalloids since AD 1400 recorded by ombrotrophic peat cores in Hautes-Fagnes, Belgium. Environ. Pollut. 178, 381–394. doi: 10.1016/j.envpol.2013.03.018
Alldredge, A. L., and Silver, M. W. (1988). Characteristics, dynamics and significance of marine snow. Progr. Oceanogr. 20, 41–82. doi: 10.1016/0079-6611(88)90053-5
Allen, S., Allen, D., Phoenix, V. R., Le Roux, G., Jiménez, P. D., Simonneau, A., et al. (2019). Atmospheric transport and deposition of microplastics in a remote mountain catchment. Nat. Geogr. 12, 339–344. doi: 10.1038/s41561-019-0335-5
Amaral-Zettler, L. A., Zettler, E. R., and Mincer, T. J. (2020). Ecology of the plastisphere. Nat. Rev. Microbiol. 18, 139–151. doi: 10.1038/s41579-019-0308-0
Andrady, A. L. (2011). Microplastics in the marine environment. Mar. Pollut. Bull. 62, 1596–1605. doi: 10.1016/j.marpolbul.2011.05.030
Andrady, A. L., and Neal, M. A. (2009). Applications and societal benefits of plastics. Philos. Trans. R. Soc. Lond. B Biol. Sci. 364, 1977–1984. doi: 10.1098/rstb.2008.0304
Appleby, P. (2001). “Chronostratrigraphic techniques in Recent Sediments” in Tracking Environmental Change Using Lake Sediments - Volume 1: Basin Analysis, Coring, and Chronological Techniques, eds W. Last and J. P. Smol (Dordrecht: Kluwer Academic Publishers), 171–203.
Archer, W., Aldeias, V., and McPherron, S. P. (2020). What is ‘in situ'? A reply to Harmand et al. 2015. J. Hum. Evol. 142:102740. doi: 10.1016/j.jhevol.2020.102740
Avio, C. G., Gorbi, S., and Regoli, F. (2015). Experimental development of a new protocol for extraction and characterization of microplastics in fish tissues: first observations in commercial species from Adriatic Sea. Mar. Environ. Res. 111, 18–26. doi: 10.1016/j.marenvres.2015.06.014
Baldwin, A. K., Spanjer, A. R., Rosen, M. R., and Thom, T. (2020). Microplastics in Lake Mead national recreation area, USA: occurrence and biological uptake. PLoS ONE 15:e0228896. doi: 10.1371/journal.pone.0228896
Ballent, A., Corcoran, P. L., Madden, O., Helm, P. A., and Longstaffe, F. J. (2016). Sources and sinks of microplastics in Canadian Lake Ontario nearshore, tributary and beach sediments. Mar. Pollut. Bull. 110, 383–395. doi: 10.1016/j.marpolbul.2016.06.037
Barbante, C., Schwikowski, M., Döring, T., Gäggeler, H. W., Schotterer, U., Tobler, L., et al. (2004). Historical record of European emissions of heavy metals to the atmosphere since the 1650s from Alpine snow/ice cores drilled near Monte Rosa. Environ. Sci. Technol. 38, 4085–4090. doi: 10.1021/es049759r
Barham, L., Tooth, S., Duller, G. A. T., Plater, A. J., and Turner, S. (2015). Excavations at Site C North, Kalambo Falls, Zambia: new insights into the mode 2/3 transition in South-Central Africa. J. African Archaeol. 13, 187–214. doi: 10.3213/2191-5784-10270
Barnes, D. K., Galgani, F., Thompson, R. C., and Barlaz, M. (2009). Accumulation and fragmentation of plastic debris in global environments. Philos. Trans. R. Soc. B Biol. Sci. 364, 1985–1998. doi: 10.1098/rstb.2008.0205
Barnes, D. K. A., and Milner, P. (2005). Drifting plastic and its consequences for sessile organism dispersal in the Atlantic Ocean. Mar. Biol. 146, 815–825. doi: 10.1007/s00227-004-1474-8
Barnosky, A. (2014). “Palaeontological evidence for defining the Anthropocene,” in A Stratigraphical Basis for the Anthropocene, eds C. N Waters, J. A. Zalasiewicz, M. Williams, M. A. Ellis, and A. M. Snelling (London: Geological Society, Special Publications), 149–165.
Beaudon, E., Gabrielli, P., Sierra-Hernández, M. R., Wegner, A., and Thompson, L. G. (2017). Central Tibetan Plateau atmospheric trace metals contamination: a 500-year record from the Puruogangri ice core. Sci. Total Environ. 601–602, 1349–1363. doi: 10.1016/j.scitotenv.2017.05.195
Bergmann, M., and Klages, M. (2012). Increase of litter at the Arctic deep-sea observatory HAUSGARTEN. Mar. Pollut. Bull. 64, 2734–2741. doi: 10.1016/j.marpolbul.2012.09.018
Bergmann, M., Mützel, S., Primpke, S., Tekman, M. B., Trachsel, J., and Gerdts, G. (2019). White and wonderful? Microplastics prevail in snow from the Alps to the Arctic. Sci. Adv. 5:eaax1157. doi: 10.1126/sciadv.aax1157
Bindler, R., Klarqvist, M., Klaminder, J., and Förster, J. (2004). Does within-bog spatial variability of mercury and lead constrain reconstructions of absolute deposition rates from single peat records? The example of Store Moss, Sweden. Glob. Biogeochem. Cycles 18:GB3020. doi: 10.1029/2004GB002270
Birks, H. J. B. (2019). Contributions of quaternary botany to modern ecology and biogeography. Plant Ecol. Divers. 12, 189–385. doi: 10.1080/17550874.2019.1646831
Brand, J. H., Spencer, K. L., O'shea, F. T., and Lindsay, J. E. (2018). Potential pollution risks of historic landfills on low-lying coasts and estuaries. Wiley Interdisc. Rev. Water 5:e1264. doi: 10.1002/wat2.1264
Brandon, J. A., Jones, W., and Ohman, M. D. (2019). Multidecadal increase in plastic particles in coastal ocean sediments. Sci. Adv. 5:eaax0587. doi: 10.1126/sciadv.aax0587
Brimble, S. K., Foster, K. L., Mallory, M. L., Macdonald, R. W., Smol, J. P., and Blais, J. M. (2009). High arctic ponds receiving biotransported nutrients from a nearby seabird colony are also subject to potentially toxic loadings of arsenic, cadmium and zinc. Environ. Toxicol. Chem. 28, 2426–2433. doi: 10.1897/09-235.1
Brinkhurst, R. O., Chua, K. E., and Kaushik, N. K. (1972). Interspecific interactions and selective feeding by tubificid oligocheates. Limnology 17, 122–133. doi: 10.4319/lo.1972.17.1.0122
Browne, M. A., Galloway, T. S., and Thompson, R. C. (2010). Spatial patterns of plastic debris along estuarine shorelines. Environ. Sci. Technol. 44, 3404–3409. doi: 10.1021/es903784e
Byrne, P. (2019). ‘Crisp Packet From the 60s Found Washed Up on Beach Highlights Plastic Risk to Sea' The Mirror. Available online at: https://www.mirror.co.uk/news/uk-news/crisp-packet-60s-found-washed-18935596 (accessed June 16, 2020).
Candelone, J. P., Hong, S., and Boutron, C. F. (1994). An improved method for decontaminating polar snow and ice cores for heavy metal analysis. Anal. Chim. Acta 299, 9–16. doi: 10.1016/0003-2670(94)00327-0
Castro-Jiménez, J., González-Fernández, D., Fornier, M., Schmidt, N., and Sempere, R. (2019). Macro-litter in surface waters from the Rhone River: plastic pollution and loading to the NW Mediterranean Sea. Mar. Pollut. Bull. 146, 60–66. doi: 10.1016/j.marpolbul.2019.05.067
Claessens, M., De Meester, S., Van Landuyt, L., De Clerck, K., and Janssen, C. R. (2011). Occurrence and distribution of microplastics in marine sediments along the Belgian coast. Mar. Pollut. Bull. 62, 2199–2204. doi: 10.1016/j.marpolbul.2011.06.030
Cole, M., Lindeque, P., Fileman, E., Halsband, C., Goodhead, R., Moger, J., et al. (2013). Microplastic ingestion by zooplankton. Environ. Sci. Technol. 47, 6646–6655. doi: 10.1021/es400663f
Corcoran, P. L., Norris, T., Ceccanese, T., Walzak, M. J., Helm, P. A., and Marvin, C. H. (2015). Hidden plastics of Lake Ontario, Canada and their potential preservation in the sediment record. Environ. Pollut. 204, 17–25. doi: 10.1016/j.envpol.2015.04.009
Damman, A. W. (1986). Hydrology, development, and biogeochemistry of ombrogenous peat bogs with special reference to nutrient relocation in a western Newfoundland bog. Can. J. Bot. 64, 384–394. doi: 10.1139/b86-055
Davis, M. B. (2000). Palynology after Y2K—understanding the source area of pollen in sediments. Annu. Rev. Earth Planet. Sci. 28, 1–18. doi: 10.1146/annurev.earth.28.1.1
De Vleeschouwer, F., Le Roux, G., and Shotyk, W. (2010). Peat as an archive of atmospheric pollution and environmental change: a case study of lead in Europe. PAGES Mag. 18, 20–22. doi: 10.22498/pages.18.1.20
Dehaut, A., Cassone, A. L., Frère, L., Hermabessiere, L., Himber, C., Rinnert, E., et al. (2016). Microplastics in seafood: Benchmark protocol for their extraction and characterization. Environ. Pollut. 215, 223–233. doi: 10.1016/j.envpol.2016.05.018
Desforges, J. P. W., Galbraith, M., and Ross, P. S. (2015). Ingestion of microplastics by zooplankton in the northeast pacific ocean. Arch. Environ. Contam. Toxicol. 69, 320–330. doi: 10.1007/s00244-015-0172-5
Dong, M., Luo, Z., Jiang, Q., Xing, X., Zhang, Q., and Sun, Y. (2020). The rapid increases in microplastics in urban lake sediments. Sci. Rep. 10:848. doi: 10.1038/s41598-020-57933-8
Döscher, A., Gäggeler, H. W., Schotterer, U., and Schwikowski, M. (1996). A historical record of ammonium concentrations from a glacier in the Alps. Geophys. Res. Lett. 23, 2741–2744. doi: 10.1029/96GL02615
Downing, J. A., Prairie, Y. T., Cole, J. J., Duarte, C. M., Tranvik, L. J., Striegl, R. G., et al. (2006). The global abundance and size distribution of lakes, ponds, and impoundments. Limnol. Oceanogr. 51, 2388–2397. doi: 10.4319/lo.2006.51.5.2388
Dris, R., Gasperi, J., Saad, M., Mirande, C., and Tassin, B. (2016). Synthetic fibers in atmospheric fallout: a source of microplastics in the environment? Mar. Pollut. Bull. 104, 290–293. doi: 10.1016/j.marpolbul.2016.01.006
Ebbesmeyer, C., and Scigliano, E. (2009). Flotsametrics and the Floating World: How One Man's Obsession with Runaway Sneakers and Rubber Ducks Revolutionized Ocean Science. London: Collins.
ECHA European Chemicals Agency. (2019). ANNEX XV. Restriction Report Proposal for a Restriction. Intentionally Added Microplastics. Available online at: https://echa.europa.eu/documents/10162/05bd96e3-b969-0a7c-c6d0-441182893720 (accessed June 3, 2020).
Eichler, A., Tobler, L., Eyrikh, S., Gramlich, G., Malygina, N., Papina, T., et al. (2012). Three centuries of Eastern European and Altai lead emissions recorded in a Belukha ice core. Environ. Sci. Technol. 46, 4323–4330. doi: 10.1021/es2039954
Enders, K., Lenz, R., Ivar do Sul, J. A., Tagg, A. S., and Labrenz, M. (2020). When every particle matters: a QuEChERS approach to extract microplastics from environmental samples. Methods X 7:100784. doi: 10.1016/j.mex.2020.100784
Eriksen, M., Mason, S., Wilson, S., Box, C., Zellers, A., Edwards, W., et al. (2013). Microplastic pollution in the surface waters of the Laurentian Great Lakes. Mar. Pollut. Bull. 77, 177–182. doi: 10.1016/j.marpolbul.2013.10.007
Erni-Cassola, G., Gibson, M. I., Thompson, R. C., and Christie-Oleza, J. A. (2017). Lost, but found with Nile red: a novel method for detecting and quantifying small microplastics (1 mm to 20 μm) in environmental samples. Environ. Sci. Technol. 51, 13641–13648. doi: 10.1021/acs.est.7b04512
Erni-Cassola, G., Zadjelovic, V., Gibson, M. I., and Christie-Oleza, J. A. (2019). Distribution of plastic polymer types in the marine environment: a meta-analysis. J. Haz. Mat. 369, 691–698. doi: 10.1016/j.jhazmat.2019.02.067
Fischer, E. K., Paglialonga, L., Czech, E., and Tamminga, M. (2016). Microplastic pollution in lakes and lake shoreline sediments – a case study on Lake Bolsena and Lake Chiusi (central Italy). Environ. Pollut. 213, 648–657. doi: 10.1016/j.envpol.2016.03.012
Free, C. M., Jensen, O. P., Mason, S. A., Eriksen, M., Williamson, N. J., and Boldgiv, B. (2014). High-levels of microplastic pollution in a large, remote, mountain lake. Mar. Pollut. Bull. 85, 156–163. doi: 10.1016/j.marpolbul.2014.06.001
Frias, J. P. G. L., Gago, J., Otero, V., and Sobral, P. (2016). Microplastics in coastal sediments from Southern Portuguese shelf waters. Mar. Environ. Res. 114, 24–30. doi: 10.1016/j.marenvres.2015.12.006
Frias, J. P. G. L., and Nash, R. (2019). Microplastics: finding a consensus on the definition. Mar. Pollut. Bull. 138, 145–147. doi: 10.1016/j.marpolbul.2018.11.022
Gabrieli, J., and Barbante, C. (2014). The Alps in the age of the Anthropocene: the impact of human activities on the cryosphere recorded in the Colle Gnifetti glacier. Rend. Lincei. 25, 71–83. doi: 10.1007/s12210-014-0292-2
Gabrielli, P., and Vallelonga, P. (2015). “Contaminant records in ice cores,” in Environmental Contaminants. Developments in Paleoenvironmental Research, Vol. 18, eds J. Blais, M. Rosen, and J. Smol (Dordrecht: Spriger), 393–430.
Gabrielli, P., Wegner, A., Sierra-Hernández, M. R., Beaudon, E., Davis, M., Barker, J. D., et al. (2020). Early atmospheric contamination on the top of the Himalayas since the onset of the European Industrial Revolution. Proc. Natl. Acad. Sci. U.S.A. 117, 3967–3973. doi: 10.1073/pnas.1910485117
Gajewski, K., Hamilton, P. B., and McNeely, R. (1997). A high resolution proxy-climate record from an arctic lake with annually-laminated sediments on Devon Island, Nunavut, Canada. J. Paleolimnol. 17, 215–225. doi: 10.1023/A:1007984617675
Galgani, F., Burgeot, T., Bocquene, G., Vincent, F., Leaute, J. P., Labastie, J., et al. (1995). Distribution and abundance of debris on the continental shelf of the Bay of Biscay and in Seine Bay. Mar. Pollut. Bull. 30, 58–62. doi: 10.1016/0025-326X(94)00101-E
Galgani, F., Souplet, A., and Cadiou, Y. (1996). Accumulation of debris on the deep sea floor off the French Mediterranean coast. Mar. Ecol. Progr. Ser. 142, 225–234. doi: 10.3354/meps142225
Galloway, T. S., Cole, M., and Lewis, C. (2017). Interactions of microplastic debris throughout the marine ecosystem. Nat. Ecol. Evol. 1, 1–8. doi: 10.1038/s41559-017-0116
Gałuszka, A., Migaszewski, Z. M., and Namieśnik, J. (2017). The role of analytical chemistry in the study of the Anthropocene. Trends Anal. Chem. 97, 146–152. doi: 10.1016/j.trac.2017.08.017
Geyer, R., Jambeck, J. R., and Law, K. L. (2017). Production, use, and fate of all plastics ever made. Sci. Adv. 3:e1700782. doi: 10.1126/sciadv.1700782
Gore, A. J. P. (1983). Ecosystems of the World—Mires: Swamps, Bog, Fen, and Moor. Amsterdam; Oxford; New York, NY: Elsevier.
Gregory, M. R. (2009). Environmental implications of plastic debris in marine settings–entanglement, ingestion, smothering, hangers-on, hitch-hiking and alien invasions. Philos. Trans. R. Soc. Lond. B Biol. Sci. 364, 2013–2025. doi: 10.1098/rstb.2008.0265
Gregory, M. R., and Andrady, A. L. (2003). “Plastics in the marine environment,” in Plastics and the Environment, ed A. L. Andrady (New Jersey NJ: John Wiley and Sons), 379–400.
Haave, M., Lorenz, C., Primpke, S., and Gerdts, G. (2019). Different stories told by small and large microplastics in sediment - first report of microplastic concentrations in an urban recipient in Norway. Mar. Pollut. Bull. 141, 501–513. doi: 10.1016/j.marpolbul.2019.02.015
Hansson, S. V., Bindler, R., and De Vleeschouwer, F. (2015). “Using peat records as natural archives of past atmospheric metal deposition,” in Environmental Contaminants. Developments in Paleoenvironmental Research, Vol. 18, eds J. Blais, M. Rosen, and J. Smol (Dordrecht: Springer), 35–60.
Hardesty, B. D., Harari, J., Isobe, A., Lebreton, L., Maximenko, N., Potemra, J., et al. (2017). Using numerical model simulations to improve the understanding of micro-plastic distribution and pathways in the marine environment. Front. Mar. Sci. 4:30. doi: 10.3389/fmars.2017.00030
Hardy, B. L., Moncel, M. H., Kerfant, C., Lebon, M., Bellot-Gurlet, L., and Mélard, N. (2020). Direct evidence of Neanderthal fibre technology and its cognitive and behavioral implications. Scient. Rep. 10:8167. doi: 10.1038/s41598-020-65143-5
Hartmann, N. B., Hüffer, T., Thompson, R. C., Hassellöv, M., Verschoor, A., Daugaard, A. E., et al. (2019). Are we speaking the same language? Recommendations for a definition and categorization framework for plastic debris. Environ. Sci. Technol. 53, 1039–1047. doi: 10.1021/acs.est.8b05297
Hartwig, E., Clemens, T., and Heckroth, M. (2007). Plastic debris as nesting material in a Kittiwake-(Rissa tridactyla)-colony at the Jammerbugt, Northwest Denmark. Mar. Pollut. Bull. 54, 595–597. doi: 10.1016/j.marpolbul.2007.01.027
Helcoski, R., Yonkos, L. T., Sanchez, A., and Baldwin, A. H. (2020). Wetland soil microplastics are negatively related to vegetation cover and stem density. Environ. Pollut. 256:113391. doi: 10.1016/j.envpol.2019.113391
Hidalgo-Ruz, V., Gutow, L., Thompson, R. C., and Thiel, M. (2012). Microplastics in the marine environment: a review of the methods used for identification and quantification. Environ. Sci. Technol. 46, 3060–3075. doi: 10.1021/es2031505
Hoffmann, G., and Reicherter, K. (2014). Reconstructing Anthropocene extreme flood events by using litter deposits. Glob. Planet. Change 122, 23–28. doi: 10.1016/j.gloplacha.2014.07.012
Holden, J., Chapman, P. J., Lane, S. N., and Brookes, C. (2006). “Impacts of artificial drainage of peatlands on runoff production and water quality,” in Peatlands: Evolution and Records of Environmental and Climate Changes, eds I. P. Martini, A. M. Cortizas, and W. Chesworth (Oxford: Elsevier B.V.), 501–528.
Hong, S., Lee, K., Hou, S., Hur, S. D., Ren, J., Burn, L. J., et al. (2009). An 800-year record of atmospheric As, Mo, Sn, and Sb in central Asia in high-altitude ice cores from Mt. Qomolangma (Everest), Himalayas. Environ. Sci. Technol. 43, 8060–8065. doi: 10.1021/es901685u
Horton, A. A., Svendsen, C., Williams, R. J., Spurgeon, D. J., and Lahive, E. (2017). Large microplastic particles in sediments of tributaries of the River Thames, UK – abundance, sources and methods for effective quantification. Mar. Pollut. Bull. 114, 218–226. doi: 10.1016/j.marpolbul.2016.09.004
Hurley, R., Woodward, J., and Rothwell, J. J. (2018a). Microplastic contamination of river beds significantly reduced by catchment-wide flooding. Nat. Geosci. 11, 251–257. doi: 10.1038/s41561-018-0080-1
Hurley, R. R., Lusher, A. L., Olsen, M., and Nizzetto, L. (2018b). Validation of a method for extracting microplastics from complex, organic-rich, environmental matrices. Environ. Sci. Technol. 52, 7409–7417. doi: 10.1021/acs.est.8b01517
Imhof, H. K., Ivleva, N. P., Schmid, J., Niessner, R., and Laforsch, C. (2013). Contamination of beach sediments of a subalpine lake with microplastic particles. Curr. Biol. 23, R867–R868. doi: 10.1016/j.cub.2013.09.001
Ivar do Sul, J. A., Costa, M. F., Silva-Cavalcanti, J. S., and Araújo, M. C. B. (2014). Plastic debris retention and exportation by a mangrove forest patch. Mar. Pollut. Bull. 78, 252–257. doi: 10.1016/j.marpolbul.2013.11.011
Ivar do Sul, J. A., and Labrenz, M. (2020). “Microplastics into the anthropocene: rise and fall of the human footprint,” in Handbook of Microplastics in the Environment, eds T. Rocha-Santos, M. Costa, and C. Mouneyrac (Springer). doi: 10.1007/978-3-030-10618-8
Jamieson, A. J., Malkocs, T., Piertney, S. B., Fujii, T., and Zhang, Z. (2017). Bioaccumulation of persistent organic pollutants in the deepest ocean fauna. Nat. Ecol. Evol. 1, 1–4. doi: 10.1038/s41559-016-0051
Johannessen, S. C., and Macdonald, R. W. (2012). There is no 1954 in that core! Interpreting sedimentation rates and contaminant trends in marine sediment cores. Mar. Pollut. Bull. 64, 675–678. doi: 10.1016/j.marpolbul.2012.01.026
Jouzel, J., Raisbeck, G., Benoist, J. P., Yiou, F., Lorius, C., Raynaud, D., et al. (1989). A comparison of deep Antarctic ice cores and their implications for climate between 65,000 and 15,000 years ago. Quat. Res. 31, 135–150. doi: 10.1016/0033-5894(89)90003-3
Kaiser, D., Kowalski, N., and Waniek, J. J. (2017). Effects of biofouling on the sinking behavior of microplastics. Environ. Res. Lett. 12:124003. doi: 10.1088/1748-9326/aa8e8b
Kane, I. A., Clare, M. A., Miramontes, E., Wogelius, R., Rothwell, J. J., Garreau, P., et al. (2020). Seafloor microplastic hotspots controlled by deep-sea circulation. Science 368, 1140–1145. doi: 10.1126/science.aba5899
Kanhai, L. D. K., Katarina, G., Krumpen, T., and Thompson, R. C. (2020). Microplastics in sea ice and seawater beneath ice floes from the Arctic Ocean. Sci. Rep. 10:5004. doi: 10.1038/s41598-020-61948-6
Käppler, A., Fischer, D., Oberbeckmann, S., Schernewski, G., Labrenz, M., Eichhorn, K. J., et al. (2016). Analysis of environmental microplastics by vibrational microspectroscopy: FTIR, Raman or both? Anal. Bioanal. Chem. 408, 8377–8391. doi: 10.1007/s00216-016-9956-3
Katija, K., Choy, C. A., Sherlock, R. E., Sherman, A. D., and Robison, B. H. (2017). From the surface to the seafloor: how giant larvaceans transport microplastics into the deep sea. Sci. Adv. 3:e1700715. doi: 10.1126/sciadv.1700715
Kerrigan, J. F., Sandberg, K. D., Engstrom, D. R., La Para, T. M., and Arnold, W. A. (2018). Sedimentary record of antibiotic accumulation in Minnesota Lakes. Sci. Tot. Environ. 621, 970–979. doi: 10.1016/j.scitotenv.2017.10.130
Kinder, M., Tylmann, W., Bubak, I., Fiłoc, M., Gasiorowski, M., Kupryjanowicz, M., et al. (2019). Holocene history of human impacts inferred from annually laminated sediments in Lake Szurpiły, northeast Poland. J. Paleolimnol. 61, 419–435. doi: 10.1007/s10933-019-00068-2
Klein, S., Dimzon, I. K., Eubeler, J., and Knepper, T. P. (2018). “Analysis, occurrence, and degradation of microplastics in the aqueous environment,” in Freshwater Microplastics: Emerging Environmental Contaminants? eds M. Wagner and S. Lambert (Cham: Springer Open), 51–67.
Klein, S., Worch, E., and Knepper, T. P. (2015). Occurrence and spatial distribution of microplastics in river shore sediments of the Rhine-Main area in Germany. Environ. Sci. Technol. 49, 6070–6076. doi: 10.1021/acs.est.5b00492
Knight, M., Ballantyne, R., Robinson Zeki, I., and Gibson, D. (2019). The must farm pile-dwelling settlement. Antiquity 93, 645–663. doi: 10.15184/aqy.2019.38
Koelmans, A. A., Nor, N. H. M., Hermsen, E., Kooi, M., Mintenig, S. M., and De France, J. (2019). Microplastics in freshwaters and drinking water: Critical review and assessment of data quality. Water Res. 155, 410–422. doi: 10.1016/j.watres.2019.02.054
Kong, X., and Koelmans, A. A. (2019). Modeling decreased resilience of shallow lake ecosystems toward eutrophication due to microplastic ingestion across the food web. Environ. Sci. Technol. 53, 13822–13831. doi: 10.1021/acs.est.9b03905
Kooi, M., Besseling, E., Kroeze, C., Van Wezel, A. P., and Koelmans, A. A. (2018). “Modeling the fate and transport of plastic debris in freshwaters: Review and Guidance,” in Freshwater Microplastics: Emerging Environmental Contaminants? eds M. Wagner and S. Lambert (Cham: Springer Open), 125–152.
Laiho, R., Bhuiyan, R., Straková, P., Mäkiranta, P., Badorek, T., and Penttilä, T. (2014). Modified ingrowth core method plus infrared calibration models for estimating fine root production in peatlands. Plant Soil 385, 311–327. doi: 10.1007/s11104-014-2225-3
Law, K. L., Morét-Ferguson, S., Maximenko, N. A., Proskurowski, G., Peacock, E. E., Hafner, J., et al. (2010). Plastic accumulation in the North Atlantic subtropical gyre. Science 329, 1185–1188. doi: 10.1126/science.1192321
Lebreton, L., Egger, M., and Slat, B. (2019). A global mass budget for positively buoyant macroplastic debris in the ocean. Sci. Rep. 9:12922. doi: 10.1038/s41598-019-49413-5
Lebreton, L., Slat, B., Ferrari, F., Sainte-Rose, B., Aitken, J., Marthouse, R., et al. (2018). Evidence that the Great Pacific Garbage Patch is rapidly accumulating plastic. Sci. Rep. 8:4666. doi: 10.1038/s41598-018-22939-w
Lei, Y. D., and Wania, F. (2004). Is rain or snow a more efficient scavenger of organic chemicals? Atmos. Environ. 38, 3557–3571. doi: 10.1016/j.atmosenv.2004.03.039
Leinfelder, R., and Ivar do Sul, J. A. (2019). “The stratigraphy of plastics and their preservation in geological records,” in The Anthropocene as a Geological Time Unit: A Guide to the Scientific Evidence and Current Debate, eds J. A. Zalasiewicz, C. N. Waters, M. Williams, and C. P. Summerhayes (Cambridge: Cambridge University Press), 147–155.
Lennartz, B., and Liu, H. (2019). Hydraulic functions of peat soils and ecosystem service. Front. Environ. Sci. 7:92. doi: 10.3389/fenvs.2019.00092
Li, J., Liu, H., and Chen, J. P. (2018). Microplastics in freshwater systems: a review on occurrence, environmental effects, and methods for microplastics detection. Water Res. 137, 362–374. doi: 10.1016/j.watres.2017.12.056
Li, R., Zhang, L., Xue, B., and Wang, Y. (2019). Abundance and characteristics of microplastics in the mangrove sediment of the semi-enclosed Maowei Sea of the south China sea: New implications for location, rhizosphere, and sediment compositions. Environ. Pollut. 244, 685–692. doi: 10.1016/j.envpol.2018.10.089
Lin, T., Hu, L., Shi, X., Li, Y., Guo, Z., and Zhang, G. (2012). Distribution and sources of organochlorine pesticides in sediments of the coastal East China Sea. Mar. Pollut. Bull. 64, 1549–1555. doi: 10.1016/j.marpolbul.2012.05.021
Lovett, G. M., and Kinsman, J. D. (1990). Atmospheric pollutant deposition to high-elevation ecosystems. Atmos. Environ. Part A Gen. Top. 24, 2767–2786. doi: 10.1016/0960-1686(90)90164-I
Lyons, K. (2018, October 9) ‘Plastic bottle washes up looking 'almost new' after nearly 50 years at sea'. The Guardian. Available onlilne at: https://www.theguardian.com/environment/2018/oct/09/plastic-bottle-washes-up-looking-almost-new-after-nearly-50-years-at-sea (accessed June 16, 2020).
Maes, T., Barry, J., Leslie, H. A., Vethaak, A. D., Nicolaus, E. E. M., Law, R. J., et al. (2018). Below the surface: Twenty-five years of seafloor litter monitoring in coastal seas of North West Europe (1992–2017). Sci. Total Environ. 630, 790–798. doi: 10.1016/j.scitotenv.2018.02.245
Mai, L., Bao, L. J., Shi, L., Wong, C. S., and Zeng, E. Y. (2018). A review of methods for measuring microplastics in aquatic environments. Environ. Sci. Poll. Res. 25, 11319–11332. doi: 10.1007/s11356-018-1692-0
Mapstone, T. (2019, June 12). “Plastic bag found in Sunshine Coast waterway could be up to 40 years old and it's just the tip of the iceberg”. ABC News. Available online at: https://www.abc.net.au/news/2019-06-13/40-year-old-plastic-bag-found-in-waterway/11197892 (accessed June 16, 2020).
Martí, E., Martin, C., Galli, M., Echevarría, F., Duarte, C. M., and Cózar, A. (2020). The colours of the ocean plastics. Environ. Sci. Technol. 54, 6594–6601. doi: 10.1021/acs.est.9b06400
Martínez-Cortizas, A., Pontevedra-Pombal, X., Garcia-Rodeja, E., Novoa-Munoz, J. C., and Shotyk, W. (1999). Mercury in a Spanish peat bog: archive of climate change and atmospheric metal deposition. Science 284, 939–942. doi: 10.1126/science.284.5416.939
Martínez-Cortizas, A., and Weiss, D. (2002). Peat bog archives of atmospheric metal deposition. Sci. Tot. Environ. 292, 1–5. doi: 10.1016/S0048-9697(02)00024-4
Matsuguma, Y., Takada, H., Kumata, H., Kanke, H., Sakurai, S., Suzuki, T., et al. (2017). Microplastics in sediment cores from Asia and Africa as indicators of temporal trends in plastic pollution. Arch. Environ. Contam. Toxicol. 73, 230–239. doi: 10.1007/s00244-017-0414-9
McCall, P. L., and Fisher, J. B. (1980). “Effects of tubificid oligochaetes on physical and chemical properties of lake Erie sediments,” in Aquatic Oligochaete Biology, eds R. O. Brinkhurst and D. G. Cook (Boston, MA: Springer), 253–317.
McConnell, J. R., and Edwards, R. (2008). Coal burning leaves toxic heavy metal legacy in the Arctic. Proc. Natl. Acad. Sci. U.S.A. 105, 12140–12144. doi: 10.1073/pnas.0803564105
McConnell, J. R., Maselli, O. J., Sigl, M., Vallelonga, P., Neumann, T., Anschütz, H., et al. (2014). Antarctic-wide array of high-resolution ice core records reveals pervasive lead pollution began in 1889 and persists today. Sci. Rep. 4:5848. doi: 10.1038/srep05848
Mendoza, L. M. R., and Balcer, M. (2019). Microplastics in freshwater environments: a review of quantification assessment. Trends Anal. Chem. 113, 402–408. doi: 10.1016/j.trac.2018.10.020
Meng, Y., Kelly, F. J., and Wright, S. L. (2020). Advances and challenges of microplastic pollution in freshwater ecosystems: a UK perspective. Environ. Pollut. 256:113445. doi: 10.1016/j.envpol.2019.113445
Meyers, P. A., and Lallier-Vergès, E. (1999). Lacustrine sedimentary organic matter records of Late Quaternary paleoclimates. J. Paleolimnol. 21, 345–372. doi: 10.1023/A:1008073732192
Michels, J., Stippkugel, A., Lenz, M., Wirtz, K., and Engel, A. (2018). Rapid aggregation of biofilm-covered microplastics with marine biogenic particles. Proc. R. Soc. B. 285:20181203. doi: 10.1098/rspb.2018.1203
Miller, R. Z., Watts, A. J. R., Winslow, B. O., Galloway, T. S., and Barrows, A. P. W. (2017). Mountains to the sea: river study of plastic and non-plastic microfiber pollution in the northeast USA. Mar. Pollut. Bull. 124, 245–251. doi: 10.1016/j.marpolbul.2017.07.028
Mintenig, S. M., Int-Veen, I., Löder, M. G., Primpke, S., and Gerdts, G. (2017). Identification of microplastic in effluents of waste water treatment plants using focal plane array-based micro-Fourier-transform infrared imaging. Water Res. 108, 365–372. doi: 10.1016/j.watres.2016.11.015
Möhlenkamp, P., Purser, A., and Thomsen, L. (2018). Plastic microbeads: an experimental study of their hydrodynamic behaviour, vertical transport and resuspension in phytoplankton and sediment aggregates. Elem. Sci. Anth. 6:61. doi: 10.1525/elementa.317
Morritt, D., Stefanoudis, P. V., Pearce, D., Crimmen, O. A., and Clark, P. F. (2014). Plastic in the Thames: a river runs through it. Mar. Pollut. Bull. 78, 196–200. doi: 10.1016/j.marpolbul.2013.10.035
Muir, D. C. G., Grift, N. P., Lockhart, W. L., Wilkinson, P., Billeck, B. N., and Brunskill, G. J. (1995). Spatial trends and historical profiles of organochlorine pesticides in Arctic lake sediments. Sci. Total Environ. 160–161, 447–457. doi: 10.1016/0048-9697(95)04378-E
Mulder, T., Hüneke, H., and Van Loon, A. J. (2011). “Progress in deep-sea sedimentology,” in Developments in Sedimentology, eds H. Hüneke and T. Mulder (Amsterdam: Elsevier), 1–24.
Naidu, S. A., Ranga Rao, V., and Ramu, K. (2018). Microplastics in the benthic invertebrates from the coastal waters of Kochi, Southeastern Arabian Sea. Environ. Geochem. Health 40, 1377–1383. doi: 10.1007/s10653-017-0062-z
Ng, K. L., and Obbard, J. P. (2006). Prevalence of microplastics in Singapore's coastal marine environment. Mar. Pollut. Bull. 52, 761–767. doi: 10.1016/j.marpolbul.2005.11.017
Nieminen, T. M., Ukonmaanaho, L., and Shotyk, W. (2002). Enrichment of Cu, Ni, Zn, Pb and as in an ombrotrophic peat bog near a Cu–Ni smelter in Southwest Finland. Sci. Total Environ. 292, 81–89. doi: 10.1016/S0048-9697(02)00028-1
Nor, N. H. M., and Obbard, J. P. (2014). Microplastics in Singapore's coastal mangrove ecosystems. Mar. Pollut. Bull. 79, 278–283. doi: 10.1016/j.marpolbul.2013.11.025
Nuelle, M. T., Dekiff, J. H., Remy, D., and Fries, E. (2014). A new analytical approach for monitoring microplastics in marine sediments. Environ. Pollut. 184, 161–169. doi: 10.1016/j.envpol.2013.07.027
Obbard, R. W., Sadri, S., Wong, Y. Q., Khitun, A. A., Baker, I., and Thompson, R. C. (2014). Global warming releases microplastic legacy frozen in Arctic Sea ice. Earth's Fut. 2, 315–320. doi: 10.1002/2014EF000240
Ostle, C., Thompson, R. C., Broughton, D., Gregory, L., Wootton, M., and Johns, D. G. (2019). The rise in ocean plastics evidenced from a 60-year time series. Nat. Commun. 10:1622. doi: 10.1038/s41467-019-09506-1
Outridge, P. M., and Wang, F. (2015). “The stability of metal profiles in freshwater and marine sediments,” in Environmental contaminants. Developments in Paleoenvironmental Research, Vol. 18, eds J. Blais, M. Rosen, and J. Smol (Dordrecht: Springer), 35–60.
Pagter, E., Frias, J., and Nash, R. (2018). Microplastics in galway bay: a comparison of sampling and separation methods. Mar. Pollut. Bull. 135, 932–940. doi: 10.1016/j.marpolbul.2018.08.013
Peeken, I., Primpke, S., Beyer, B., Gütermann, J., Katlein, C., Krumpen, T., et al. (2018). Arctic sea ice is an important temporal sink and means of transport for microplastic. Nat. Commun. 9:1505. doi: 10.1038/s41467-018-03825-5
Peng, G., Xu, P., Zhu, B., Bai, M., and Li, D. (2018). Microplastics in freshwater river sediments in Shanghai, China: a case study of risk assessment in mega-cities. Environ. Pollut. 234, 448–456. doi: 10.1016/j.envpol.2017.11.034
Pietrelli, L., Di Gennaro, A., Menegoni, P., Lecce, F., Poeta, G., Acosta, A. T. R., et al. (2017). Pervasive plastisphere: first record of plastics in egagropiles (Posidonia spheroids). Environ. Pollut. 229, 1032–1036. doi: 10.1016/j.envpol.2017.07.098
Plastics Europe (2012). Plastics—The Facts 2012: An Analysis of European Plastics Production, Demand and Waste Data for 2011. Available online at: https://www.plasticseurope.org/download_file/force/1687/181 (accessed June 17, 2020).
Plastics Europe (2016). Plastics—The Facts 2016: An Analysis of European Plastics Production, Demand and Waste Data. Available online at: https://www.plasticseurope.org/application/files/4315/1310/4805/plastic-the-fact-2016.pdf (accessed June 15, 2020).
Plastics Europe (2019). Plastics – The Facts 2019: An Analysis of European Plastics Production, Demand and Waste Data. Available online at: https://www.plasticseurope.org/download_file/force/3183/181 (accessed June 15, 2020).
Porter, A., Lyons, B. P., Galloway, T. S., and Lewis, C. (2018). Role of marine snows in microplastic fate and bioavailability. Environ. Sci. Technol. 52, 7111–7119. doi: 10.1021/acs.est.8b01000
Prata, J. C., da Costa, J. P., Duarte, A. C., and Rocha-Santos, T. (2019). Methods for sampling and detection of microplastics in water and sediment: a critical review. Trends Anal. Chem. 110, 150–159. doi: 10.1016/j.trac.2018.10.029
Primpke, S., Cross, R. K., Mintenig, S. M., Simon, M., Vianello, A., Gerdts, G., et al. (2020). EXPRESS: toward the systematic identification of microplastics in the environment: evaluation of a new independent software tool (siMPle) for spectroscopic analysis. Appl. Spectrosc. 3702820917760. doi: 10.1177/0003702820917760
Quinn, B., Murphy, F., and Ewins, C. (2017). Validation of density separation for the rapid recovery of microplastics from sediment. Anal. Meth. 9, 1491–1498. doi: 10.1039/C6AY02542K
Quinton, W., Elliot, T., Price, J., Rezanezhad, F., and Heck, R. (2009). Measuring physical and hydraulic properties of peat from X-ray tomography. Geoderma 153, 269–277. doi: 10.1016/j.geoderma.2009.08.010
Rausch, N., Nieminen, T., Ukonmaanaho, L., Le Roux, G., Krachler, M., Cheburkin, A. K., et al. (2005). Comparison of atmospheric deposition of copper, nickel, cobalt, zinc, and cadmium recorded by Finnish peat cores with monitoring data and emission records. Environ. Sci. Technol. 39, 5989–5998. doi: 10.1021/es050260m
Redondo-Hasselerharm, P. E., Falahudin, D., Peeters, E. T., and Koelmans, A. A. (2018). Microplastic effect thresholds for freshwater benthic macroinvertebrates. Environ. Sci. Technol. 52, 2278–2286. doi: 10.1021/acs.est.7b05367
Redondo-Hasselerharm, P. E., Gort, G., Peeters, E. T. H. M., and Koelmans, A. A. (2020). Nano- and microplastics affect the composition of freshwater benthic communities in the long term. Sci. Adv. 6:eaay4054. doi: 10.1126/sciadv.aay4054
Renberg, I., Bindler, R., and Brännvall, M.-L. (2001). Using the historical atmospheric lead-deposition record as a chronological marker in sediment deposits in Europe. Holocene 11, 511–516. doi: 10.1191/095968301680223468
Reynolds, C., and Ryan, P. G. (2018). Micro-plastic ingestion by waterbirds from contaminated wetlands in South Africa. Mar. Pollut. Bull. 126, 330–333. doi: 10.1016/j.marpolbul.2017.11.021
Rezaei, M., Riksen, M. J. P. M., Sirjani, E., Sameni, A., and Geissen, V. (2019). Wind erosion as a driver for transport of light density microplastics. Sci. Tot. Environ. 669, 273–281. doi: 10.1016/j.scitotenv.2019.02.382
Rezanezhad, F., Price, J. S., Quinton, W. L., Lennartz, B., Milojevic, T., and Van Cappellen, P. (2016). Structure of peat soils and implications for water storage, flow and solute transport: a review update for geochemists. Chem. Geol. 429, 75–84. doi: 10.1016/j.chemgeo.2016.03.010
Ribeiro-Claro, P., Nolasco, M. M., and Araújo, C. (2017). Characterization of microplastics by Raman spectroscopy. Compr. Anal. Chem. 75, 119–151. doi: 10.1016/bs.coac.2016.10.001
Rillig, M. C. (2018). Microplastic disguising as soil carbon storage. Environ. Sci. Technol. 52, 6079–6080. doi: 10.1021/acs.est.8b02338
Robbins, J. A. (1978). “Geochemical and geophysical applications of radioactive lead,” in The Biogeochemistry of Lead in the Environment, ed J. O. Nriagu (Amsterdam: Elsevier/North-Holland Biomedical), 285–393.
Rochman, C. M., Brookson, C., Bikker, J., Djuric, N., Earn, A., Bucci, K., et al. (2019). Rethinking microplastics as a diverse contaminant suite. Environ. Toxicol. Chem. 38, 703–711. doi: 10.1002/etc.4371
Rose, N. L. (2015). Spheroidal carbonaceous fly-ash particles provide a globally synchronous stratigraphic marker for the Anthropocene. Environ. Sci. Technol. 49, 4155–4162. doi: 10.1021/acs.est.5b00543
Ryan, P. G., Moore, C. J., van Franeker, J. A., and Moloney, C. L. (2009). Monitoring the abundance of plastic debris in the marine environment. Philos. Trans. R. Soc. B. Biol. Sci. 364, 1999–2012. doi: 10.1098/rstb.2008.0207
Sanchez, W., Bender, C., and Porcher, J.-M. (2014). Wild gudgeons (Gobio gobio) from French rivers are contaminated by microplastics: preliminary study and first evidence. Environ. Res. 128, 98–100. doi: 10.1016/j.envres.2013.11.004
Sander, L. (2016). Date-prints on stranded macroplastics: inferring the timing and extent of overwash deposition on the Skallingen peninsula, Denmark. Mar. Pollut. Bull. 109, 373–377. doi: 10.1016/j.marpolbul.2016.05.051
Scherer, C., Brennholt, N., Reifferscheid, G., and Wagner, M. (2017). Feeding type and development drive the ingestion of microplastics by freshwater invertebrates. Sci. Rep. 7:17006. doi: 10.1038/s41598-017-17191-7
Scheurer, M., and Bigalke, M. (2018). Microplastics in swiss floodplain soils. Environ. Sci. Technol. 52, 3591–3598. doi: 10.1021/acs.est.7b06003
Scholz, C. A. (2001). “Applications of seismic sequence stratigraphy in lacustrine basins,” in Tracking Environmental Change Using Lake Sediments. Volume 1: Basin Analysis, Coring, and Chronological Techniques, eds W. M. Last and J. P. Smol (Dordrecht: Kluwer Academic Publishers), 7–22.
Schotterer, U., Stichler, W., and Ginot, P. (2004). “The Influence of post-depositional effects on ice core studies: examples from the Alps, Andes, and Altai,” in Earth Paleoenvironments: Records Preserved in Mid- and Low-Latitude Glaciers. eds L. Cecil, J. R. DeWayne, L. G. Green Thompson (Dordrecht: Springer), 39–59.
Scopetani, C., Esterhuizen-Londt, M., Chelazzi, D., Cincinelli, A., Setälä, H., and Pflugmacher, S. (2020). Self-contamination from clothing in microplastics research. Ecotoxicol. Environ. Safety 189:110036. doi: 10.1016/j.ecoenv.2019.110036
Seddon, A. W. R., Mackay, A. W., Baker, A. G., Birks, H. J. B., Breman, E., Buck, C. E., et al. (2014). Looking forward through the past. Identification of fifty priority research questions in palaeoecology. J. Ecol. 102, 256–267. doi: 10.1111/1365-2745.12195
Setälä, O., Fleming-Lehtinen, V., and Lehtiniemi, M. (2014). Ingestion and transfer of microplastics in the planktonic food web. Environ. Pollut. 185, 77–83. doi: 10.1016/j.envpol.2013.10.013
Shotyk, W. (1996). Peat bog archives of atmospheric metal deposition: geochemical evaluation of peat profiles, natural variations in metal concentrations, and metal enrichment factors. Environ. Rev. 4, 149–183. doi: 10.1139/a96-010
Šilc, U., Küzmič, F., Caković, D., and Stešević, D. (2018). Beach litter along various sand dune habitats in the southern Adriatic (E Mediterranean). Mar. Pollut. Bull. 128, 353–360. doi: 10.1016/j.marpolbul.2018.01.045
Silva, A. B., Bastos, A. S., Justino, C. I. L., da Costa, J. P., Duarte, A. C., and Rocha- Santos, T. A. P. (2018). Microplastics in the environment: challenges in analytical chemistry - a review. Anal. Chim. Acta 1017, 1–19. doi: 10.1016/j.aca.2018.02.043
Souter, L., and Watmough, S. A. (2016). The impact of drought and air pollution on metal profiles in peat cores. Sci. Total Environ. 541, 1031–1040. doi: 10.1016/j.scitotenv.2015.09.137
Su, L., Xue, Y., Li, L., Yang, D., Kolandhasamy, P., Li, D., et al. (2016). Microplastics in Taihu Lake, China. Environ. Pollut. 216, 711–719. doi: 10.1016/j.envpol.2016.06.036
Tekman, M. B., Wekerle, C., Lorenz, C., Primpke, S., Hasemann, C., Gerdts, G., et al. (2020). Tying up loose ends of microplastic pollution in the arctic: distribution from the sea surface through the water column to deep-sea sediments at the HAUSGARTEN observatory. Environ. Sci. Technol. 54, 4079–4090. doi: 10.1021/acs.est.9b06981
Teuten, E. L., Saquing, J. M., Knappe, D. R. U., Barlaz, M. A., Jonsson, S., Björn, A., et al. (2009). Transport and release of chemicals from plastics to the environment and to wildlife. Philos. Trans. R. Soc. B Biol. Sci. 364, 2027–2045. doi: 10.1098/rstb.2008.0284
Thompson, R. C., Olsen, Y., Mitchell, R. P., Davis, A., Rowland, S. J., John, A. W. G., et al. (2004). Lost at sea: where is all the plastic? Science 304:838. doi: 10.1126/science.1094559
Tibbetts, J., Krause, S., Lynch, I., and Smith, G. H. S. (2018). Abundance, distribution, and drivers of microplastic contamination in urban river environments. Water 10:1597. doi: 10.3390/w10111597
Turner, S. D., Horton, A., Rose, N. L., and Hall, C. J. (2019). A temporal sediment record of microplastics in an urban lake, London, UK. J. Paleolimnol. 61, 449–462. doi: 10.1007/s10933-019-00071-7
Uglietti, C., Gabrielli, P., Cooke, C. A., Vallelonga, P., and Thompson, L. G. (2015). Widespread pollution of the South American atmosphere predates the Industrial Revolution by 240 y. Proc. Natl. Acad. Sci. U.S.A. 112, 2349–2354. doi: 10.1073/pnas.1421119112
Van Cauwenberghe, L., Devriese, L., Galgani, F., Robbens, J., and Janssen, C. R. (2015). Microplastics in sediments: a review oftechniques, occurrence and effects. Mar. Environ. Res. 111, 5–17. doi: 10.1016/j.marenvres.2015.06.007
Van Cauwenberghe, L., Vanreusel, A., Mees, J., and Janssen, C. R. (2013). Microplastic pollution in deep-sea sediments. Environ. Pollut. 182, 495–499. doi: 10.1016/j.envpol.2013.08.013
Vaughan, R., Turner, S. D., and Rose, N. L. (2017). Microplastics in the sediments of a UK urban lake. Environ. Pollut. 229, 10–18. doi: 10.1016/j.envpol.2017.05.057
Vosshage, A. T. L., Neu, T. R., and Gabel, F. (2018). Plastic alters biofilm quality as food resource of the freshwater gastropod Radix balthica. Environ. Sci. Technol. 52, 11387–11393. doi: 10.1021/acs.est.8b02470
Votier, S. C., Archibald, K., Morgan, G., and Morgan, L. (2011). The use of plastic debris as nesting material by a colonial seabird and associated entanglement mortality. Mar. Pollut. Bull. 62, 168–172. doi: 10.1016/j.marpolbul.2010.11.009
Waters, C. N., Zalasiewicz, J., Summerhayes, C., Fairchild, I. J., Rose, N. L., Loader, N. J., et al. (2018). Global Boundary Stratotype Section and Point (GSSP) for the anthropocene series: where and how to look for potential candidates. Earth-Sci. Rev. 178, 379–429. doi: 10.1016/j.earscirev.2017.12.016
Weinstein, J. E., Crocker, B. K., and Gray, A. D. (2016). From macroplastic to microplastic: degradation of high-density polyethylene, polypropylene, and polystyrene in a salt marsh habitat. Environ. Toxicol. Chem. 35, 1632–1640. doi: 10.1002/etc.3432
Williams, A. T., and Simmons, S. L. (1996). The degradation of plastic litter in rivers: Implications for beaches. J. Coast. Conserv. 2, 63–72. doi: 10.1007/BF02743038
Willis, K. A., Eriksen, R., Wilcox, C., and Hardesty, B. D. (2017). microplastic distribution at different sediment depths in an urban estuary. Front. Mar. Sci. 4, 1–8. doi: 10.3389/fmars.2017.00419
Wiltshire, P. E. J. (2006). Consideration of some taphonomic variables of relevance to forensic palynological investigation in the United Kingdom. Forensic Sci. Int. 163, 173–182. doi: 10.1016/j.forsciint.2006.07.011
Woodall, L. C., Sanchez-Vidal, A., Canals, M., Paterson, G. L., Coppock, R., Sleight, V., et al. (2014). The deep sea is a major sink for microplastic debris. R. Soc. Open Sci. 1:140317. doi: 10.1098/rsos.140317
Xiong, X., Zhang, K., Chen, X., Shi, H., Luo, Z., and Wu, C. (2018). Sources and distribution of microplastics in China's largest inland lake Qinghai Lake. Environ. Pollut. 235, 899–906. doi: 10.1016/j.envpol.2017.12.081
Xue, B., Zhang, L., Li, R., Wang, Y., Guo, J., Yu, K., et al. (2020). Underestimated microplastic pollution derived from fishery activities and “Hidden” in deep sediment. Environ. Sci. Technol. 54, 2210–2217. doi: 10.1021/acs.est.9b04850
Yang, C., Rose, N. L., Turner, S. D., Yang, H., Goldsmith, B., Losada, S., et al. (2016). Hexabromocyclododecanes, polybrominated diphenyl ethers and polychlorinated biphenyls in radiometrically dated sediment cores from English Lakes, ~1950 – present. Sci. Total Environ. 541, 721–728. doi: 10.1016/j.scitotenv.2015.09.102
Yang, H., Engstrom, D., and Rose, N. L. (2010). Recent changes in atmospheric mercury deposition recorded in the sediments of remote, equatorial lakes in the Rwenzori Mountains, Uganda. Environ. Sci. Technol. 44, 6570–6575. doi: 10.1021/es101508p
Yao, W., Di, D., Wang, Z., Liao, Z., Huang, H., Mei, K., et al. (2019). Micro- and macroplastic accumulation in a newly formed Spartina alterniflora colonized estuarine saltmarsh in southeast China. Mar. Pollut. Bull. 149:110636. doi: 10.1016/j.marpolbul.2019.110636
Zalasiewicz, J., Waters, C. N., Ivar do Sul, J. A., Corcoran, P. L., Barnosky, A. D., Cearreta, A., et al. (2016). The geological cycle of plastics and their use as a stratigraphic indicator of the Anthropocene. Anthropocene 13, 4–17. doi: 10.1016/j.ancene.2016.01.002
Zalasiewicz, J. A., Summerhayes, C. P., Head, M. J., Wing, S., Gibbard, P., and Waters, C. N. (2019). “Stratigraphy and the geological time scale,” in The Anthropocene as a Geological Time Unit: A Guide to the Scientific Evidence and Current Debate, eds J. A. Zalasiewicz, C. N. Waters, M. Williams, and C. P. Summerhayes (Cambridge: Cambridge University Press), 11–30.
Zarfl, C. (2019). Promising techniques and open challenges for microplastic identification and quantification in environmental matrices. Anal. Bioanal. Chem. 411, 3743–3756. doi: 10.1007/s00216-019-01763-9
Zhang, K., Su, J., Xiong, X., Wu, X., Wu, C., and Liu, J. (2016). Microplastic pollution of lakeshore sediments from remote lakes in Tibet plateau, China. Environ. Pollut. 219, 450–455. doi: 10.1016/j.envpol.2016.05.048
Zhao, S., Danley, M., Ward, J. E., Li, D., and Mincer, T. J. (2017). An approach for extraction, characterization and quantitation of microplastic in natural marine snow using Raman microscopy. Anal. Met. 9, 1470–1478. doi: 10.1039/C6AY02302A
Zhao, S., Zhu, L., Gao, L., and Li, D. (2018). “Limitations for microplastic quantification in the ocean and recommendations for improvement and standardization,” in Microplastic Contamination in Aquatic Environments, ed E. Y. Zeng (Amsterdam: Elsevier), 27–49.
Zobkov, M. B., Esiukova, E. E., Zyubin, A. Y., and Samusev, I. G. (2019). Microplastic content variation in water column: The observations employing a novel sampling tool in stratified Baltic Sea. Mar. Pollut. Bull. 138, 193–205. doi: 10.1016/j.marpolbul.2018.11.047
Keywords: Anthropocene, anthropogenic particles, chemostratigraphic units, ice cores, peats, sediment cores, taphonomy
Citation: Bancone CEP, Turner SD, Ivar do Sul JA and Rose NL (2020) The Paleoecology of Microplastic Contamination. Front. Environ. Sci. 8:574008. doi: 10.3389/fenvs.2020.574008
Received: 18 June 2020; Accepted: 14 August 2020;
Published: 24 September 2020.
Edited by:
Hans Peter Heinrich Arp, Norwegian Geotechnical Institute, NorwayReviewed by:
Clare Alexandra Wilson, University of Stirling, United KingdomSaija Maarit Saarni, University of Helsinki, Finland
Copyright © 2020 Bancone, Turner, Ivar do Sul and Rose. This is an open-access article distributed under the terms of the Creative Commons Attribution License (CC BY). The use, distribution or reproduction in other forums is permitted, provided the original author(s) and the copyright owner(s) are credited and that the original publication in this journal is cited, in accordance with accepted academic practice. No use, distribution or reproduction is permitted which does not comply with these terms.
*Correspondence: Neil L. Rose, bi5yb3NlQHVjbC5hYy51aw==