- 1Institut für Biologie, Freie Universität Berlin, Berlin, Germany
- 2Berlin-Brandenburg Institute of Advanced Biodiversity Research, Berlin, Germany
Microplastic as an anthropogenic pollutant accumulates in terrestrial ecosystems over time, threatening soil quality and health, for example by decreasing aggregate stability. Organic matter addition is an efficient approach to promote aggregate stability, yet little is known about whether microplastic can reduce the beneficial effect of organic matter on aggregate stability. We investigated the impacts of microplastic fibers in the presence or absence of different organic materials by carrying out a soil incubation experiment. This experiment was set up as a fully factorial design containing all combinations of microplastic fibers (no microplastic fiber addition, two different types of polyester fibers, and polyacrylic) and organic matter (no organic matter addition, Medicago lupulina leaves, Plantago lanceolata leaves, wheat straw, and hemp stems). We evaluated the percentage of water-stable aggregates (WSA) and activities of four soil enzymes (β-glucosidase, β-D-celluliosidase, N-acetyl-b-glucosaminidase, phosphatase). Organic matter addition increased WSA and enzyme activities, as expected. In particular, Plantago or wheat straw addition increased WSA and enzyme activities by 224.77 or 281.65% and 298.51 or 55.45%, respectively. Microplastic fibers had no effect on WSA and enzyme activities in the soil without organic matter addition, but decreased WSA and enzyme activities by 26.20 or 37.57% and 23.85 or 26.11%, respectively, in the presence of Plantago or wheat straw. Our study shows that the effects of microplastic fibers on soil aggregation and enzyme activities are organic matter dependent. A possible reason is that Plantago and wheat straw addition stimulated soil aggregation to a greater degree, resulting in more newly formed aggregates containing microplastic, the incorporated microplastic fibers led to less stable aggregates, and decrease in enzyme activities This highlights an important aspect of the context dependency of microplastic effects in soil and on soil health. Our results also suggest risks for soil stability associated with organic matter additions, such as is common in agroecosystems, when microplastics are present.
Introduction
Microplastics as a group of anthropogenic contaminants are pervasive and persistent. Microplastic is widely studied in marine ecosystems (Eriksen et al., 2014; Bergmann et al., 2015; Jambeck et al., 2015), and only in recent years has attention shifted to terrestrial ecosystems (Rillig, 2012; Bläsing and Amelung, 2018; de Souza Machado et al., 2018a; Lozano and Rillig, 2020; Zhou et al., 2020). In fact, microplastic has been proposed as a new global change factor (Rillig and Lehmann, 2020). We adopted the definition that microplastics are plastics with size smaller than 5 mm (Moore, 2008; Barnes et al., 2009), with various shapes (e.g., fiber, fragment, film) and polymer types (e.g., polyester, polyethylene, polyacrylic, polypropylene), which are intentionally produced (e.g., microplastic beads in cosmetics) or fragmented into micro-sized plastics by natural or anthropogenic factors, such as photooxidation (Yakimets et al., 2004; Gewert et al., 2015), microbial degradation (Zettler et al., 2013; Moharir and Kumar, 2019) or plowing (Hann et al., 2016).
In microplastic polluted soil, microplastic fibers are a dominant shape (Singh et al., 2020; Zhou et al., 2020). Microplastic fibers derived from textiles are commonly discovered in wastewater (Pirc et al., 2016; Athey et al., 2020), they can span a length range of 0.3–25.0 mm. These microplastic fibers derived from textiles are therefore present in sludge and biosolids, which are applied on agricultural fields as fertilizer (Henry et al., 2019; Crossman et al., 2020; Zhang et al., 2020), likely leading to the accumulation of microplastic fibers in agricultural soils (Corradini et al., 2019; van den Berg et al., 2020). It is estimated that 1.56 × 1014 microplastic particles could enter the soil and other natural environments through sludge per year in China alone (Li et al., 2018). The majority of those fibers are made of polyester and polyacrylic. Moreover, atmospheric deposition of microplastic fibers is an important source of soil contamination, as hundreds of particles are deposited from the atmosphere per square meter per day (Cai et al., 2017; Dris et al., 2017; Brahney et al., 2020). Further anthropogenic activities, such as tillage, and also movement by soil animals can accelerate the incorporation of microplastic fibers into the soil (Huerta Lwanga et al., 2017; Rillig et al., 2017).
Microplastic fibers have been shown to influence soil quality and health by being detrimental to soil aggregate stability (de Souza Machado et al., 2018b; Lehmann et al., 2019a) and altering microbial activity (Liu et al., 2017; de Souza Machado et al., 2018b; Huang et al., 2019). Stability of soil aggregates, a fundamental soil physical property, is crucial to resist erosion, support water infiltration, water retention, aeration, and fertility (Bryan, 1968; Tisdall and Oades, 1982; Boix-Fayos et al., 2001; Li et al., 2016). Soil is a dynamic and complex system, and in particular the stability of soil aggregates is influenced by many factors including organic matter input, soil texture, clay mineralogy, and microbial populations (Seta and Karathanasis, 1996; Bossuyt et al., 2001; Wagner et al., 2007). Among these, organic matter is one of the most important factors determining aggregation (Abiven et al., 2008). The addition of organic matter promotes stable aggregation for example by stimulating microbial growth and metabolism, leading to increases in microbially derived metabolites such as polysaccharides and proteins, which, together with plant-derived polysaccharides act as gluing agents that facilitate aggregate stabilization (Tisdall, 1994; Wright and Upadhyaya, 1998; Caesar-Tonthat, 2002). The degradability of organic matter/litter is an inherent property of the material, leading to different breakdown products being released. Therefore, the effects of organic matter on aggregation varied among different types of organic matter (Abiven et al., 2008). We have previously shown that under soil conditions favorable to the formation of aggregates, microplastic fiber addition could reduce the stability of aggregates (Liang et al., 2019), because newly formed aggregates are likely to have incorporated microplastic fibers which can reduce stability of aggregates, likely by introducing fracture points. Given that organic matter addition facilitates the formation of aggregates, we assume that microplastic fibers have a more detrimental effect on WSA when organic matter is added, and that the magnitude of the effect depends on the type of organic matter.
Soil enzyme activity is key to biological processes, driving nutrient cycles in terrestrial ecosystems. Enzyme activity as an important indicator of microbial activity is frequently altered by microplastics (de Souza Machado et al., 2018b; Liang et al., 2019). As microplastic fibers tend to reduce bulk density and increase soil porosity (de Souza Machado et al., 2018b; Zhang et al., 2019a), microplastic fibers are expected to improve aeration, and thus increase enzyme activity. Moreover, microplastics themselves as organic carbon might introduce an artificial carbon source (Rillig, 2018), possibly influencing enzyme activity by being a potential substrate. However, the overall outcome of the interactive effects of microplastic fibers and organic matter on aggregates and enzyme activity remains unknown, and is the subject of our investigation here.
We carried out a soil incubation experiment with all combinations of 3 types of microplastic fibers and 4 types of organic matter. We hypothesize: (1) soil aggregation will be decreased by microplastic fiber, but effects will depend on the type of organic matter; (2) microplastic fibers will increase soil enzymatic activities.
Materials and Methods
Microplastic Fibers
Two polyester products (polyester1, PE1: Rope Paraloc 137 Mamutec polyester white, item number, 8,442,172, Hornbach.de, diameter: 0.03 mm, density: 1.45 g cm–3; polyester 2, PE2: Dolphin Fine 5 × 100 g Himalaya Knitting Wool, Baby Wool, 500 g Super Bulky Wool, diameter: 0.008 mm, density: 1.37 g cm––3) and one polyacrylic, PA (100% acrylic ‘‘Bravo’’ yarn1, diameter: 0.026 mm, density: 1.31 g cm–3) product were used in this study (Supplementary Figure 1). These types of plastics are widely used in textiles (Carney Almroth et al., 2018), and have previously been shown to decrease soil aggregation (de Souza Machado et al., 2018b). We produced microplastic fibers by manually cutting them into fragments of approximately 5 mm in length, the average lengths of PE1, PE2, and PA are 4.56 ± 0.94, 4.20 ± 1.37, 4.05 ± 0.1.14 mm, respectively, the size distributions are given in Supplementary Figure 2. The estimated particle numbers of PE1, PE2, and PA are 193, 2.9 × 104, 2.87 × 103 items g–1 dry soil, respectively These lengths were chosen to match the criteria of microplastic upper limit and the general size range of secondary microplastic fibers produced by washing of clothes made from synthetic fibers (Pirc et al., 2016). We rinsed the fibers with tap water for 5 min to remove soluble chemicals, then dried them at 60°C for 24 h, and subsequently microwaved them for 3 min to reduce any microbial populations adhering to the material. The microwaving does not alter the physical appearance of the treated microplastic products (de Souza Machado et al., 2018b). The microplastic fibers were mixed into the soil at a concentration of 0.3% (w/w), toward the upper limit of concentrations used in a previous study (de Souza Machado et al., 2018b), such concentration was also applied in other study (Zhang et al., 2019b). The concentration 0.3% we used is within the contamination range in a plastic industrial area, the soil in which contained 0.03– 6.7% of microplastic (Fuller and Gautam, 2016).
Organic Matter
We chose four different types of organic matter: Medicago lupulina leaves, Plantago lanceolata leaves, wheat straw (MultiFit, Item no.: 1,008,159, Krefeld, Germany), and hemp stems (REAL NATURE, Item no.: 1,259,176, Krefeld, Germany) (Supplementary Figure 3). Medicago lupulina and Plantago lanceolata leaf material were chosen as typical species from the local grassland, which were collected from plants previously grown in our greenhouse, wheat straw was chosen as the type of organic matter widely applied in agriculture for soil amendments, hemp was used to represent woody plant litter. Medicago, Plantago, straw and hemp formed a gradient of litter quality with C:N ratios as 12.85 ± 0.11, 14.76 ± 0.29, 133.03 ± 2.18, 153.04 ± 0.70%, respectively, the decomposition of Medicago was the fastest, followed by Plantago, and straw, with hemp being the slowest to decompose (Supplementary Figure 4). Before adding organic matter to our experiment, we ground the material using a blender (Philips Pro Blend 6 RD, Germany) and sieved to keep size between 0.5 and 2 mm. We added organic matter with a concentration of 0.8% (w/w) to our test systems. The concentration of 0.8% was below the saturation level of organic matter addition in our test soil (Supplementary Figure 5).
Experimental Design
This experiment was set up as a fully factorial design and contained all combinations of microplastic fibers [no microplastic fiber addition (No mf), PE1, PE2, PA) and organic matters (no organic matter addition (No OM), Medicago, Plantago, wheat straw, hemp stems], resulting in 20 treatments including the controls. Each treatment had 8 replicates for a total of 160 experimental units.
Soil Incubation
Fresh soil was collected from a local grassland (Berlin, Germany) with a sandy loam texture, an Albic Luvisol (Rillig et al., 2010). Soil was sieved < 0.5 mm in order to reduce the amount of larger soil aggregates, thus intensifying the effect of organic matter addition on aggregate formation. Reducing aggregates beforehand is commonly used to measure macroaggregate formation in laboratory incubations (De Gryze et al., 2005). We mixed 20 g of the dry soil with 60 mg of microplastic fibers by steel spoon for 3 min, achieving a homogeneous distribution of fibers as no obvious fiber clusters could be observed by eye, then we mixed the prepared soil with 160 mg of organic matter of the different types for 30 s which is sufficient time to achieve an even distribution of organic matter. We applied the 3.5 min of mixing time to the soil without microplastic or organic matter addition aiming for the same level of disturbance. The soil mixture was transferred to a 50 ml falcon tube by using a steel spoon, the soil mixture was placed into the tube carefully in order to maintain the distribution of microplastic fiber in soil we achieved after mixing. Then we slowly wetted soil with distilled water by injecting water into soil by using a syringe, the water passively spread throughout our test system. We kept water content at 60% water holding capacity. Tubes were then closed with a hydrophobic vented cap to allow gas exchange. We centrifuged all tubes with soils at 100 rpm/s for 1 min to minimize any cracks in the soil (Brackin et al., 2013), and then incubated them at 25°C in the dark for 42 days, we assumed organic matter might achieve an intensive effect on aggregate stability after 42 days (Abiven et al., 2008).
Aggregate Stability Measurement
To measure soil aggregate stability, we followed the protocol by Kemper and Rosenau (2018): the air-dried soils were sieved through a 2 mm sieve and 4.0 g of soils were placed into sieves for capillary rewetting in deionized water for 5 min. We used 0.25 mm sieves to test the stability of the soil fraction > 0.25 mm (macroaggregates) against water as a disintegrating force. For the test, sieves carrying the soil samples were placed in a wet-sieving machine (Eijkelkamp, Netherlands) and moved vertically (stroke = 1.3 cm, 34 times min–1 for 3 min. The fractions left on the sieves were dried at 60°C for 24 h. After weighing the dry fractions, sand particles and organic debris larger than 0.25 mm were extracted from the fractions as coarse matter. The calculation of percent water-stable aggregates (WSA) was: % WSA = (water stable aggregates − coarse matter)/(4.0 g − coarse matter).
Enzyme Activity Measurement
To assess the ability of the microbial community to acquire nutrients, we measured activities of β-glucosidase (cellulose degradation), β-D-celluliosidase (cellulose degradation), N-acetyl-b-glucosaminidase (chitin degradation), and phosphatase (organic phosphorus mineralization) (Delgado-Baquerizo et al., 2017). These enzymes are key for microbes to acquire C, N, and P, and are thus at least partially indicative of the function of the microbial community in terms of organic matter processing and decomposition (Waldrop et al., 2000). Soil enzymatic activity was determined using a high throughput microplate assay, according to Jackson et al. (2013). Activities of above enzymes were measured using p-nitrophenyl (pNP) -linked model substrates: pNP-β-D-glucopyranoside (Sigma no. N7006), pNP-β-D-cellobioside (Sigma no. N5759), pNP-N-acetyl-β-D-glucosaminide (Sigma no. N9376), pNP- phosphate disodium salt hexahydrate (Sigma no. 71,768), respectively. Briefly, 3.5 g of frozen stored (−20°C) soil of each sample was placed in a sterile 50 ml centrifuge tube and mixed with 10 ml of 50 mM acetate buffer, and then the mixture was vortexed for 30 s to produce a soil slurry. After vortexing, the soil slurry was transferred to 96-well microplates. Reaction mixtures in each cell contained 0.150 mL soil slurry and 0.150 mL substrate dissolved in 50 mM sodium acetate buffer (pH 5.0). Reaction mixtures were incubated at 25°C for 2–4 h. After incubation, microplates were centrifuged at 3,000 rpm for 5 min, then 0.100 ml of suspension was transferred into a new microplate and mixed with 0.200 mL 0.1 M NaOH to stop the reaction. Absorbances were determined spectrophotometrically at 410 nm using a microplate reader (BioRad, Benchmark Plus, Japan). Enzyme activity was defined as the amount of released μmol of p-nitrophenol per gram of dry soil per hour.
Statistical Analysis
All statistics were conducted in R 3.5.3 (R Core Team, 2017). We analyzed the effect size of microplastic fibers on WSA and enzyme activity using the package “dabestr” (Ho et al., 2019) to generate unpaired mean differences and 95% confidence interval (CI) by a bootstrapping approach (5,000 iterations). This type of analysis estimates the magnitude and precision of an effect. To support our findings, we additionally applied two-way ANOVA by using generalized least square models in the “nlme” package (Pinheiro et al., 2020). The plots were created with the graphic package “ggplot2” (Wickham, 2016).
Results
Stability of Soil Aggregates
All types of organic matter increased WSA substantially compared to the control, with wheat straw having the most positive effect on WSA (30.7% [95%-CI: 26–35.1]), followed by Plantago (24.4% [95%-CI: 20.6–29.1]), hemp stems (24.2% [95%-CI: 19–28.4]), and Medicago (16.4% [95%-CI: 11–22.8]).
The effects of microplastic fibers on WSA strongly depended on the type of added organic matter (Figure 1 and Table 1). Microplastic fibers had neutral effects on WSA in soil without organic matter addition and soil with Medicago addition. By contrast, all types of microplastic fibers exerted negative effects on WSA in soil with Plantago and wheat straw; PE2 had negative effects in soil with hemp stem. The most negative effect of microplastic fibers on WSA was found in soil with PE1 and Plantago (−13.3%, [95%-CI: −18.7 to −6.55]), followed by PE1 and wheat straw (−10.9%, [95%-CI: −16.2 to −6.43]). Generally, microplastic fibers were more detrimental for WSA in soils with added organic matter, while organic matter addition was more beneficial to WSA (Figure 2).
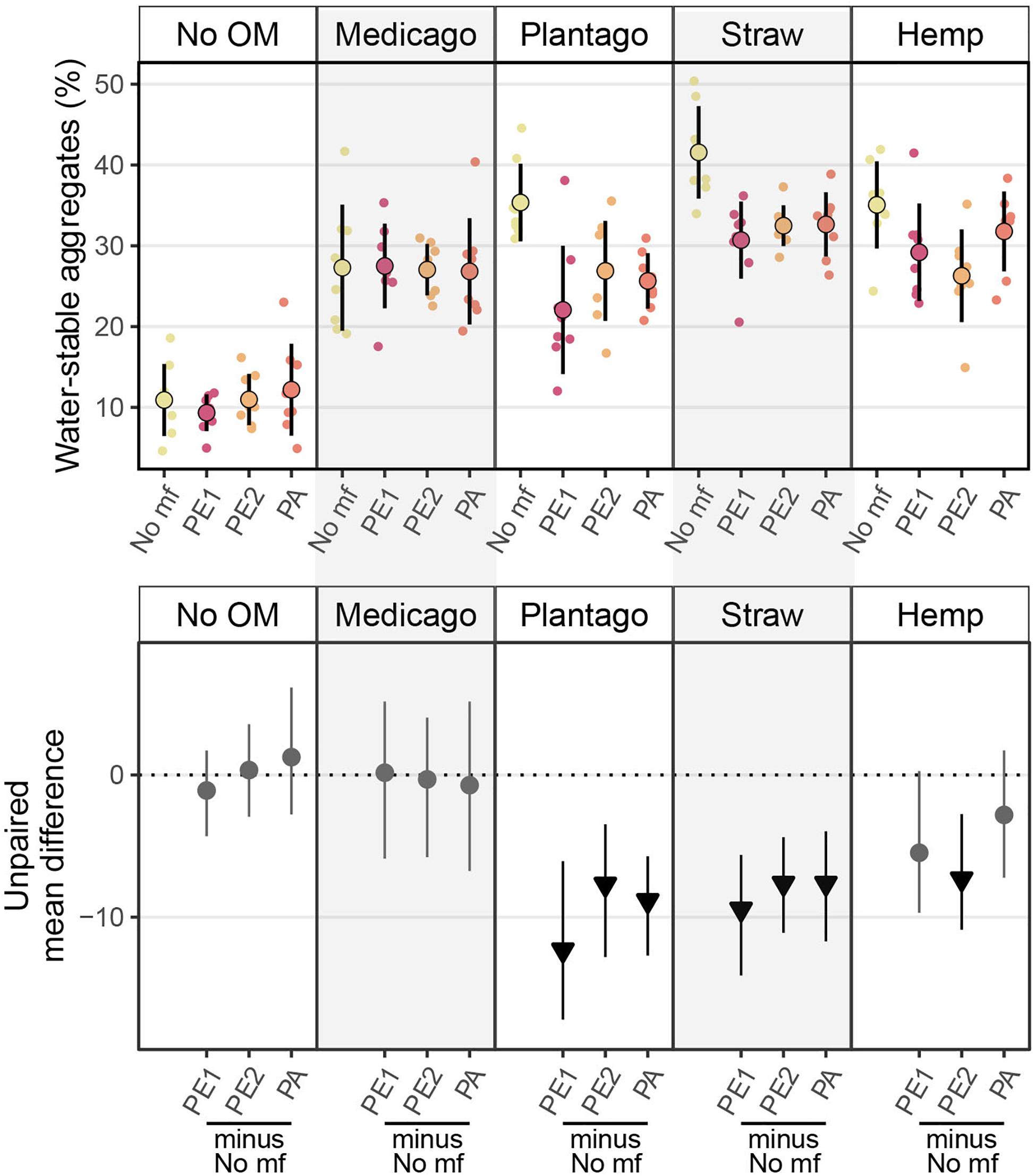
Figure 1. Effects of microplastic fibers on soil water stable aggregates (WSA in %) with the addition of different types of organic matter. The upper panel shows the raw data of water stable aggregates, data distributions are aligned with corresponding mean and standard deviation (n = 8 for each treatment). The lower panel shows the unpaired mean differences of the microplastic fiber addition and control under different organic matter addition. Circles and triangles represent the effect size mean (unpaired mean; effect magnitude) and the vertical lines the corresponding confidence intervals (effect precision). Negative (arrow head down) effect sizes and corresponding CIs of treatment compared to control are depicted in black while neutral effects (circle) are colored in gray; neutral effects occur when the CIs overlap the dashed zero line (line of no effect). “No OM” represents no organic matter addition, “No mf” represents no microplastic fiber addition.

Table 1. ANOVA results for the effects of organic matter, microplastic fibers, and the interaction of these factors on the stability of soil aggregates (WSA), β-Glucosidase (BG) activity, cellobiohydrolase (CB) activity, phosphatase (Phos) activity, and N-acetyl-glucosaminidase (NAG) activity.
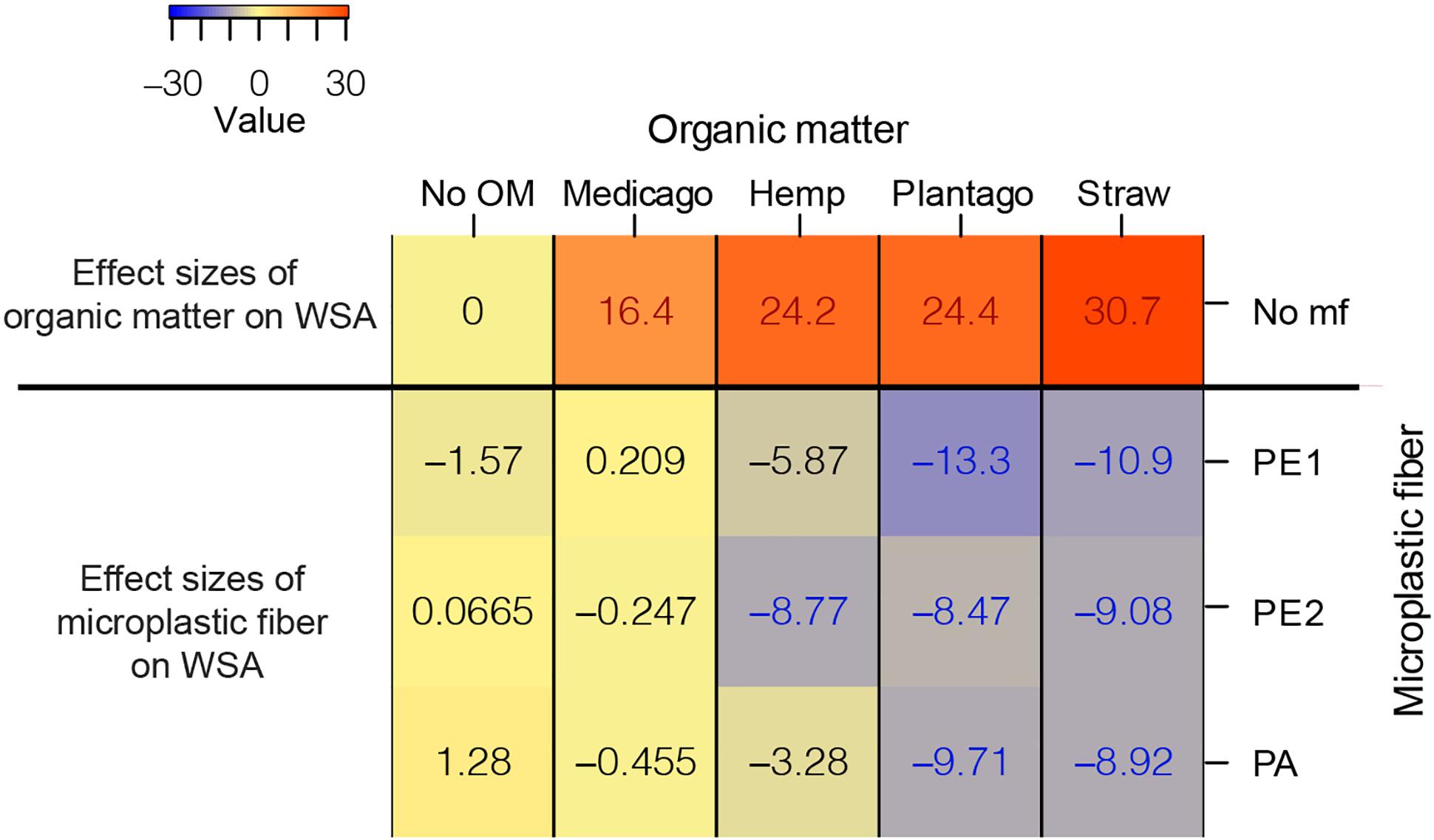
Figure 2. The heatmap of the effect sizes of microplastic fiber and organic matter on WSA. The effect sizes of organic matter on WSA were the unpaired mean differences of organic matter addition alone and control (WSAorganic matter – WSANo OM). The effect sizes of microplastic fiber are the unpaired mean differences of the microplastic fiber addition and control with the addition of the different types of organic matter. (WSAorganic matter_microplastic fiber – WSAorganic matter_No mf) The blue text represents the statistically significant negative effect of microplastic fiber on WSA, the red text represents the statistically significant positive effect on WSA. “No OM” represents no organic matter addition, “No mf” represents no microplastic fiber addition.
Enzyme Activities
Organic matter addition stimulated enzyme activities, while microplastic fibers decreased enzyme activities in some cases. The effects of microplastic fibers on enzyme activities were neutral in soil without organic matter addition, while with organic matter addition, negative effects of microplastic fibers appeared (Figure 3 and Table 1). We found the highest loss of β-Glucosidase activity in soil with PE1 and Plantago (−1.64 μmol pNP g–1 h–1 [95%-CI: −0.03 to −3.27]), cellobiohydrolase activity in soil with PE2 and hemp stem (−0.19 μmol pNP g–1 h–1 [95%-CI: −0.07 to −0.35]), phosphatase in soil with PE2 and wheat straw (−1.22 μmol pNP g–1 h–1 [95%-CI: −0.47 to −1.96]), N-acetyl-glucosaminidase in soil with PA and wheat straw (−0.65 μmol pNP g–1 h–1 [95%-CI: −0.07 to −1.24]).
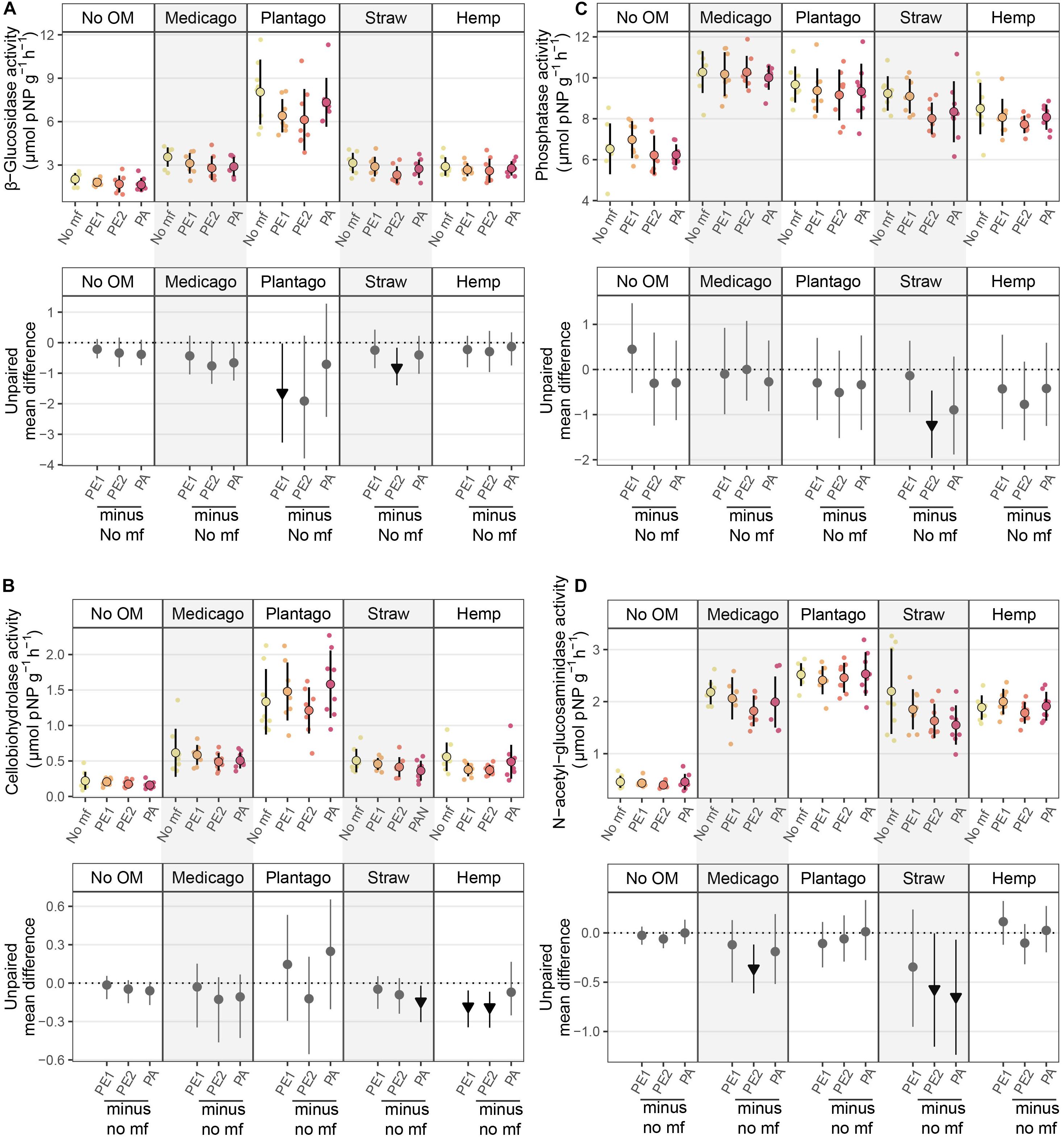
Figure 3. Effects of microplastic fibers on β-Glucosidase activity (A), cellobiohydrolase activity (B), phosphatase activity (C), and N-acetyl-glucosaminidase activity (D) in the presence of different types of organic matter. The upper panel shows the raw data of enzyme activity, data distributions are aligned with corresponding mean and standard deviation (n = 8 for each treatment). The lower panel shows the unpaired mean differences of the microplastic fiber addition and control with different types of organic matter addition. Circles and triangles represent the effect size mean (unpaired mean; effect magnitude) and the vertical lines the corresponding confidence intervals (effect precision). Negative (arrow head down) effect sizes and corresponding CIs of treatment compared to control are depicted in black while neutral effects (circle) are shown in gray; neutral effects occur when the CIs overlap the dashed zero line (line of no effect). “No OM” represents no organic matter addition, “No mf” represents no microplastic fiber addition.
We found a similar relationship between effects of microplastic fibers in the presence of different types of organic matter and effects of organic matter on β-Glucosidase activity as we found in WSA; meaning, the more positive an effect a specific organic material, the more detrimental was the impact of added microplastic fibers (Figure 4).
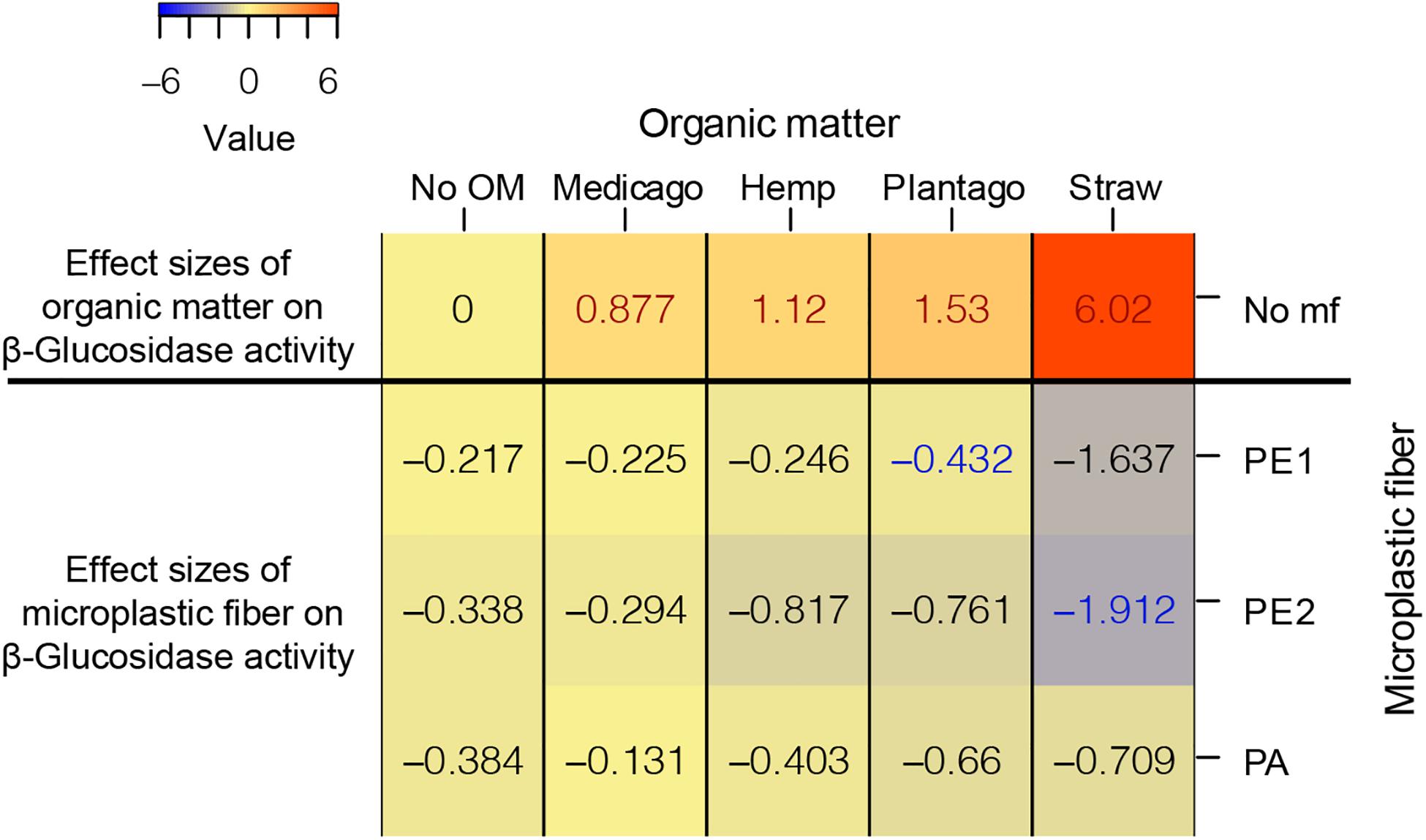
Figure 4. The heatmap of the effect sizes of microplastic fiber and organic matter on β-Glucosidase activity. The effect sizes of organic matter on β-Glucosidase activity were the unpaired mean differences of organic matter addition alone and control (β-Glucosidase activityorganic matter – β-Glucosidase activityNo OM). The effect sizes of microplastic fiber are the unpaired mean differences of the microplastic fiber addition and control with the addition of the different types of organic matter (β-Glucosidase activityorganic matter_microplastic fiber – β-Glucosidase activityorganic matter_No mf). The blue text represents the statistically significant negative effect of microplastic fiber on β-Glucosidase activity, the red text represents the statistically significant positive effect on β-Glucosidase activity. “No OM” represents no organic matter addition, “No mf” represents no microplastic fiber addition.
Discussion
Effects of Microplastic Fibers on the Stability of Soil Aggregates (WSA)
As we hypothesized, microplastic fibers reduced the stability of aggregates in the presence of specific types of organic matter, with the magnitude of the microplastic fiber effects dependent on the type of organic matter. This was likely because the addition and subsequent microbial processing of organic matter accelerated aggregation, which in turn led to more microplastic fibers being incorporated into newly formed aggregates. The concentration of microplastic fibers in aggregates was previously observed to have increased with the addition of one type of organic matter addition (Zhang and Zhang, 2020). In addition, the increased incorporation of microplastic fibers into soil aggregates might produce fracture points, therefore reducing the stability of the coarse, sandy soil that we used here. Given that the effects of organic matter on aggregation varied among different types of organic matter, the amount of increased incorporation of fibers into aggregates might depend on the type of added organic matter; this helps explain the phenomenon that microplastic fibers generally exerted more negative effects on WSA in soil to which a type of organic matter was added that favored soil aggregation.
In our study, microplastic fibers had no effects on WSA in soil without added organic matter. The sandy loam soil we used had limited intrinsic potential to form aggregates, thus there must have been only relatively few microplastic fibers that were incorporated into aggregates, resulting in no effects of microplastic fibers in soil without added organic matter. We assumed organic matter stimulated microbial activity, accelerating the formation of aggregates and the integration of fibers into those aggregates. A similar result was found in a previous study (Lehmann et al., 2019b): microplastic fiber had no effects on aggregate stability in sterile soil, while decreased aggregate stability in soil with a microbial community, indicating that microorganisms mediate effects of microplastic fibers on soil aggregate stability (Forster, 1990; Lehmann et al., 2017). A contrary result was found in a study using clayey non-sterile soil, in which polyester fiber increased macroaggregates (Zhang et al., 2019b). Soil mineralogy and clay content play important roles in the formation and stability of aggregates (Seta and Karathanasis, 1996), microplastic fibers may have different effects on soil aggregates in soils with different textures. Further studies are needed to explore the interaction between microplastic and soil minerals.
Our WSA results also reveal that predictions based on just the type of microplastic fibers alone would have failed: effects are dependent on the type of organic matter available for microbial processing—and thus on the rate of soil aggregate formation, and as such our study has uncovered an important aspect of context dependency of microplastic effects in soils. However, there is a limitation to our study. The temporal effects of organic matter input on soil aggregation are not covered in our study design. Medicago had the lowest C:N ratio, thus had the fastest decomposition rate. Therefore, we expected the greatest increase of WSA in soil amended with Medicago alone, and also the greatest loss in WSA caused by microplastic fibers in soil amended with Medicago. Nevertheless, neither was observed in our study. We assumed the Medicago as a rapidly degraded organic matter exhibited the maximum aggregate stability before we harvested the experiment, then the aggregate began to break down as the binding agents were decomposed (Abiven et al., 2008). Our findings could be relevant for agroecosystems: wheat straw is widely used as an organic amendment in agriculture due to the benefits it offers, such as increasing sequestration of soil organic carbon and improving soil structure (Han et al., 2018; Zhao et al., 2019). However, as agricultural fields are prone to microplastic contamination, our study suggests that this can lead to unforeseen effects of straw additions on soil properties; an assertion that should now be explicitly tested in the field.
Effects of Microplastic Fibers on Enzymatic Activities
Contrary to our hypothesis, microplastic fibers did not increase enzyme activities, but had even negative effects on enzyme activities with the addition of specific types of organic matter. The results depended on the microplastic type, but the pattern was not consistent across the organic matter treatments. PE2 decreased enzyme activities more frequently than PE1 and PAN, which might be attributed to the highest particle numbers of PE2 in each experimental unit. Moreover, those negative effects were more likely to appear in the presence of straw. This again emphasizes the context dependency of microplastic impacts on the soil environment. Such negative effects of microplastics are also found in other studies, as microplastics reduce soil nutrient levels (Yu et al., 2020). We do not know what caused this negative response in our study, but microplastic fibers might have caused shifts in the microbial community via changing soil physical properties (de Souza Machado et al., 2018b), releasing additives (Widén et al., 2004; Hahladakis et al., 2018) that were not water-soluble (fibers were washed before being mixed in the soil) that migrated into the soil (Kim et al., 2020), or serving as microbial habitats (Huang et al., 2019; Zhang et al., 2019c); the enzymatic activities could have changed as a consequence of such community shifts.
Microplastic fibers had negative effects on enzyme activities in the presence of organic matter, which could lead to decreased nutrient uptake into microbes and mineralization rates. The restricted nutrient transfer from organic matter to microbes could change the microbial community in the long term, potentially resulting in altered soil functions and processes (Waldrop et al., 2000). Additionally, assuming an accumulation of microplastic fibers in the future, decreased enzyme activities could lead to a reduction of plant available nutrients and thus ultimately diminish agricultural productivity or crop quality (Paz-Ferreiro et al., 2014).
Moreover, the decreased nutrient uptake could lead to decreased microbial biomass and metabolites, which are important for accumulating stabilized C in soil (Cotrufo et al., 2013; Cenini et al., 2015). Nevertheless, decreased enzyme activities accompanied by decreased transformation of organic matter could also lead to less C loss, the eventual fate of added C could not be predicted from our study due to the scope of our work here. Further studies should target long-term C dynamics of added organic matter, to enable prediction of the potential effects of microplastic fibers on C sequestration.
Though soils received different types of microplastic fibers, which varied in diameters, particle numbers per experimental unit, and probably varied in their additives, we did not find a consistent pattern of the different microplastic fibers across our response variables. In terms of WSA, as a previous study found microplastic fibers decreased WSA with increasing concentrations of microplastic fibers (de Souza Machado et al., 2018b), we assume the decreases in WSA already reached the upper limit response of soil to microplastic fibers in our study, resulting in no obvious different effects on WSA among the types of microplastic fibers.
In terms of enzyme activities, we found the decrease in enzyme activities appeared randomly among microplastic fibers. We assumed microplastics needed a longer time to reveal their effects on the microbial community and thus on microbial function, such as accumulation of additives released from aged plastic over time (Bandow et al., 2017).
Microplastic fibers we produced had higher average length than that microplastic fibers found in the environment, with most studies finding fibers of shorter than 1 mm (Cai et al., 2017; Zhou et al., 2018; Corradini et al., 2019). For our experimental work, it was impractical to manually cut fibers that small (but see Schmiedgruber et al., 2019; Frehland et al., 2020).
Conclusion
In our study, microplastic fibers affected soil aggregation by interfering with the formation of stable aggregates, with effects dependent on the type of added organic matter. It seems that greater soil aggregation activity leads to increased opportunities for microplastic to interact with this biological process; this is very likely due to microplastic fibers becoming integrated into aggregates to an increasing degree, leading to subsequent destabilization of these structures by as yet unknown mechanisms. Effects of microplastic additions on soil processes have been variable, in part likely due to the plastic material itself, but our study points to soil properties, in particular soil organic matter, as another important variable contributing to the context dependency of such effects in terrestrial ecosystems.
Data Availability Statement
All data for this paper have been deposited under https://doi.org/10.6084/m9.figshare.14270807.v3.
Author Contributions
YL and EL designed the research. YL performed the research, and conceived and performed the data analyses. YL, AL, GY, EL, and MR wrote the manuscript and contributed to the final version of the manuscript.
Conflict of Interest
The authors declare that the research was conducted in the absence of any commercial or financial relationships that could be construed as a potential conflict of interest.
Funding
We acknowledge support from an ERC Advanced Grant (694368), funding from the Federal Ministry of Education and Research (BMBF) for the projects μPlastic and BIBS, funding from Deutsche Forschungsgemeinschaft (LE 3859/1-1), and a scholarship from the China Scholarship Council.
Acknowledgments
We acknowledge the support by the OpenAccess Publication Initiative of Freie Universität Berlin.
Supplementary Material
The Supplementary Material for this article can be found online at: https://www.frontiersin.org/articles/10.3389/fenvs.2021.650155/full#supplementary-material
Footnotes
References
Abiven, S., Menasseri, S., and Chenu, C. (2008). The effects of organic inputs over time on soil aggregate stability – A literature analysis. Soil Biol. Biochem. 41, 1–12. doi: 10.1016/j.soilbio.2008.09.015
Athey, S. N., Adams, J. K., Erdle, L. M., Jantunen, L. M., Helm, P. A., Finkelstein, S. A., et al. (2020). The widespread environmental footprint of indigo denim microfibers from blue jeans. Environ. Sci. Technol. Lett. 7, 840–847. doi: 10.1021/acs.estlett.0c00498
Bandow, N., Will, V., Wachtendorf, V., and Simon, F.-G. (2017). Contaminant release from aged microplastic. Environ. Chem. 14:394. doi: 10.1071/EN17064
Barnes, D. K. A., Galgani, F., Thompson, R. C., and Barlaz, M. (2009). Accumulation and fragmentation of plastic debris in global environments. Philos. Trans. R. Soc. B Biol. Sci. 364, 1985–1998. doi: 10.1098/rstb.2008.0205
Bergmann, M., Gutow, L., and Klages, M. (2015). Marine anthropogenic litter. New York, NY: Springer International Publishing, doi: 10.1007/978-3-319-16510-3
Bläsing, M., and Amelung, W. (2018). Plastics in soil: Analytical methods and possible sources. Sci. Total Environ. 612, 422–435. doi: 10.1016/J.SCITOTENV.2017.08.086
Boix-Fayos, C., Calvo-Cases, A., Imeson, A. C., and Soriano-Soto, M. D. (2001). Influence of soil properties on the aggregation of some Mediterranean soils and the use of aggregate size and stability as land degradation indicators. Catena 44, 47–67. doi: 10.1016/S0341-8162(00)00176-4
Bossuyt, H., Denef, K., Six, J., Frey, S. D., Merckx, R., and Paustian, K. (2001). Influence of microbial populations and residue quality on aggregate stability. Appl. Soil Ecol. 16, 195–208. doi: 10.1016/S0929-1393(00)00116-5
Brackin, R., Robinson, N., Lakshmanan, P., and Schmidt, S. (2013). Microbial function in adjacent subtropical forest and agricultural soil. Soil Biol. Biochem. 57, 68–77. doi: 10.1016/j.soilbio.2012.07.015
Brahney, J., Hallerud, M., Heim, E., Hahnenberger, M., and Sukumaran, S. (2020). Plastic rain in protected areas of the United States. Science 368, 1257–1260. doi: 10.1126/science.aaz5819
Bryan, R. B. (1968). The development, use and efficiency of indices of soil erodibility. Geoderma 2, 5–26. doi: 10.1016/0016-7061(68)90002-5
Caesar-Tonthat, T. C. (2002). Soil binding properties of mucilage produced by a basidiomycete fungus in a model system. Mycol. Res. 106, 930–937. doi: 10.1017/S0953756202006330
Cai, L., Wang, J., Peng, J., Tan, Z., Zhan, Z., Tan, X., et al. (2017). Characteristic of microplastics in the atmospheric fallout from Dongguan city, China: preliminary research and first evidence. Environ. Sci. Pollut. Res. 24, 24928–24935. doi: 10.1007/s11356-017-0116-x
Carney Almroth, B. M., Åström, L., Roslund, S., Petersson, H., Johansson, M., and Persson, N. K. (2018). Quantifying shedding of synthetic fibers from textiles; a source of microplastics released into the environment. Environ. Sci. Pollut. Res. 25, 1191–1199. doi: 10.1007/s11356-017-0528-7
Cenini, V. L., Fornara, D. A., McMullan, G., Ternan, N., Lajtha, K., and Crawley, M. J. (2015). Chronic nitrogen fertilization and carbon sequestration in grassland soils: evidence of a microbial enzyme link. Biogeochemistry 126, 301–313. doi: 10.1007/s10533-015-0157-5
Corradini, F., Meza, P., Eguiluz, R., Casado, F., Huerta-Lwanga, E., and Geissen, V. (2019). Evidence of microplastic accumulation in agricultural soils from sewage sludge disposal. Sci. Total Environ. 671, 411–420. doi: 10.1016/j.scitotenv.2019.03.368
Cotrufo, M. F., Wallenstein, M. D., Boot, C. M., Denef, K., and Paul, E. (2013). The Microbial Efficiency-Matrix Stabilization (MEMS) framework integrates plant litter decomposition with soil organic matter stabilization: Do labile plant inputs form stable soil organic matter? Glob. Chang. Biol. 19, 988–995. doi: 10.1111/gcb.12113
Crossman, J., Hurley, R. R., Futter, M., and Nizzetto, L. (2020). Transfer and transport of microplastics from biosolids to agricultural soils and the wider environment. Sci. Total Environ. 724:138334. doi: 10.1016/j.scitotenv.2020.138334
De Gryze, S., Six, J., Brits, C., and Merckx, R. (2005). A quantification of short-term macroaggregate dynamics: Influences of wheat residue input and texture. Soil Biol. Biochem. 37, 55–66. doi: 10.1016/j.soilbio.2004.07.024
de Souza Machado, A. A., Kloas, W., Zarfl, C., Hempel, S., and Rillig, M. C. (2018a). Microplastics as an emerging threat to terrestrial ecosystems. Glob. Chang. Biol. 24, 1405–1416. doi: 10.1111/gcb.14020
de Souza Machado, A. A., Lau, C. W., Till, J., Kloas, W., Lehmann, A., Becker, R., et al. (2018b). Impacts of Microplastics on the Soil Biophysical Environment. Environ. Sci. Technol. 52, 9656–9665. doi: 10.1021/acs.est.8b02212
Delgado-Baquerizo, M., Eldridge, D. J., Ochoa, V., Gozalo, B., Singh, B. K., and Maestre, F. T. (2017). Soil microbial communities drive the resistance of ecosystem multifunctionality to global change in drylands across the globe. Ecol. Lett. 20, 1295–1305. doi: 10.1111/ele.12826
Dris, R., Gasperi, J., Mirande, C., Mandin, C., Guerrouache, M., Langlois, V., et al. (2017). A first overview of textile fibers, including microplastics, in indoor and outdoor environments. Environ. Pollut. 221, 453–458. doi: 10.1016/j.envpol.2016.12.013
Eriksen, M., Lebreton, L. C. M., Carson, H. S., Thiel, M., Moore, C. J., Borerro, J. C., et al. (2014). Plastic Pollution in the World’s Oceans: More than 5 Trillion Plastic Pieces Weighing over 250,000 Tons Afloat at Sea. PLoS One 9:e111913. doi: 10.1371/journal.pone.0111913
Forster, S. M. (1990). The role of microorganisms in aggregate formation and soil stabilization: Types of aggregation. Arid Soil Res. Rehabil. 4, 85–98. doi: 10.1080/15324989009381236
Frehland, S., Kaegi, R., Hufenus, R., and Mitrano, D. M. (2020). Long-term assessment of nanoplastic particle and microplastic fiber flux through a pilot wastewater treatment plant using metal-doped plastics. Res. 182:115860. doi: 10.1016/j.watres.2020.115860
Fuller, S., and Gautam, A. (2016). A Procedure for Measuring Microplastics using Pressurized Fluid Extraction. Environ. Sci. Technol. 50, 5774–5780. doi: 10.1021/acs.est.6b00816
Gewert, B., Plassmann, M. M., and Macleod, M. (2015). Pathways for degradation of plastic polymers floating in the marine environment. Environ. Sci. Process. Impacts 17, 1513–1521. doi: 10.1039/c5em00207a
Hahladakis, J. N., Velis, C. A., Weber, R., Iacovidou, E., and Purnell, P. (2018). An overview of chemical additives present in plastics: Migration, release, fate and environmental impact during their use, disposal and recycling. J. Hazard. Mater. 344, 179–199. doi: 10.1016/j.jhazmat.2017.10.014
Han, X., Xu, C., Dungait, J. A. J., Bol, R., Wang, X., Wu, W., et al. (2018). Straw incorporation increases crop yield and soil organic carbon sequestration but varies under different natural conditions and farming practices in China: a system analysis. Biogeosciences 15, 1933–1946. doi: 10.5194/bg-15-1933-2018
Hann, S., Ettlinger, S., Gibbs, A., and Hogg, D. (2016). The impact of the use of “oxo-degradable” plastic on the environment. Luxembourg: European Union, doi: 10.2779/992559
Henry, B., Laitala, K., and Klepp, I. G. (2019). Microfibres from apparel and home textiles: Prospects for including microplastics in environmental sustainability assessment. Sci. Total Environ. 652, 483–494. doi: 10.1016/j.scitotenv.2018.10.166
Ho, J., Tumkaya, T., Aryal, S., Choi, H., and Claridge-Chang, A. (2019). Moving beyond P values: data analysis with estimation graphics. Nat. Methods 16, 565–566. doi: 10.1038/s41592-019-0470-3
Huang, Y., Zhao, Y., Wang, J., Zhang, M., Jia, W., and Qin, X. (2019). LDPE microplastic films alter microbial community composition and enzymatic activities in soil. Environ. Pollut. 254:112983. doi: 10.1016/j.envpol.2019.112983
Huerta Lwanga, E., Gertsen, H., Gooren, H., Peters, P., Salánki, T., van der Ploeg, M., et al. (2017). Incorporation of microplastics from litter into burrows of Lumbricus terrestris. Environ. Pollut. 220, 523–531. doi: 10.1016/j.envpol.2016.09.096
Jackson, C. R., Tyler, H. L., and Millar, J. J. (2013). Determination of Microbial Extracellular Enzyme Activity in Waters, Soils, and Sediments using High Throughput Microplate Assays. J. Vis. Exp. 2013, 1–9. doi: 10.3791/50399
Jambeck, J. R., Geyer, R., Wilcox, C., Siegler, T. R., Perryman, M., Andrady, A., et al. (2015). Plastic waste inputs from land into the ocean. Science 347, 768–771. doi: 10.1126/science.1260352
Kemper, W. D., and Rosenau, R. C. (2018). “Aggregate Stability and Size Distribution,” in Methods of Soil Analysis. Hoboken, NJ: John Wiley & Sons, Ltd, 425–442. doi: 10.2136/sssabookser5.1.2ed.c17
Kim, S. W., Waldman, W. R., Kim, T. Y., and Rillig, M. C. (2020). Effects of different microplastics on nematodes in the soil environment: Tracking the extractable additives using an ecotoxicological approach. Environ. Sci. Technol. 2020:0c04641. doi: 10.1021/acs.est.0c04641
Lehmann, A., Fitschen, K., and Rillig, M. (2019a). Abiotic and Biotic Factors Influencing the Effect of Microplastic on Soil Aggregation. Soil Syst. 3:21. doi: 10.3390/soilsystems3010021
Lehmann, A., Fitschen, K., Rillig, M., Lehmann, A., Fitschen, K., and Rillig, M. C. (2019b). Abiotic and Biotic Factors Influencing the Effect of Microplastic on Soil Aggregation. Soil Syst. 3:21. doi: 10.3390/soilsystems3010021
Lehmann, A., Zheng, W., and Rillig, M. C. (2017). Soil biota contributions to soil aggregation. Nat. Ecol. Evol. 1, 1828–1835. doi: 10.1038/s41559-017-0344-y
Li, S., Gu, X., Zhuang, J., An, T., Pei, J., Xie, H., et al. (2016). Distribution and storage of crop residue carbon in aggregates and its contribution to organic carbon of soil with low fertility. Soil Tillage Res. 155, 199–206. doi: 10.1016/j.still.2015.08.009
Li, X., Chen, L., Mei, Q., Dong, B., Dai, X., Ding, G., et al. (2018). Microplastics in sewage sludge from the wastewater treatment plants in China. Water Res. 142, 75–85. doi: 10.1016/j.watres.2018.05.034
Liang, Y., Lehmann, A., Ballhausen, M.-B., Muller, L., and Rillig, M. C. (2019). Increasing Temperature and Microplastic Fibers Jointly Influence Soil Aggregation by Saprobic Fungi. Front. Microbiol. 10:2018. doi: 10.3389/fmicb.2019.02018
Liu, H., Yang, X., Liu, G., Liang, C., Xue, S., Chen, H., et al. (2017). Response of soil dissolved organic matter to microplastic addition in Chinese loess soil. Chemosphere 185, 907–917. doi: 10.1016/j.chemosphere.2017.07.064
Lozano, Y. M., and Rillig, M. C. (2020). Effects of Microplastic Fibers and Drought on Plant Communities. Environ. Sci. Technol. 54, 6166–6173. doi: 10.1021/acs.est.0c01051
Moharir, R. V., and Kumar, S. (2019). Challenges associated with plastic waste disposal and allied microbial routes for its effective degradation: A comprehensive review. J. Clean. Prod. 208, 65–76. doi: 10.1016/j.jclepro.2018.10.059
Moore, C. J. (2008). Synthetic polymers in the marine environment: A rapidly increasing, long-term threat. Environ. Res. 108, 131–139. doi: 10.1016/j.envres.2008.07.025
Paz-Ferreiro, J., Fu, S., Méndez, A., and Gascó, G. (2014). Interactive effects of biochar and the earthworm Pontoscolex corethrurus on plant productivity and soil enzyme activities. J. Soils Sediments 14, 483–494. doi: 10.1007/s11368-013-0806-z
Pinheiro, J., Bates, D., DebRoy, S., Sarkar, D., and R Core Team. (2020). nlme: Linear and nonlinear mixed effects models. R package version 3.1–150.
Pirc, U., Vidmar, M., Mozer, A., and Kržan, A. (2016). Emissions of microplastic fibers from microfiber fleece during domestic washing. Environ. Sci. Pollut. Res. 23, 22206–22211. doi: 10.1007/s11356-016-7703-0
R Core Team. (2017). R: A Language and Environment for Statistical Computing. Available online at: http://www.r-project.org [accessed February 26, 2019]
Rillig, M. C. (2012). Microplastic in Terrestrial Ecosystems and the Soil? Environ. Sci. Technol. 46, 6453–6454. doi: 10.1021/es302011r
Rillig, M. C. (2018). Microplastic Disguising As Soil Carbon Storage. Environ. Sci. Technol. 52, 6079–6080. doi: 10.1021/acs.est.8b02338
Rillig, M. C., and Lehmann, A. (2020). Microplastic in terrestrial ecosystems. Science 368, 1430–1431. doi: 10.1126/science.abb5979
Rillig, M. C., Mardatin, N. F., Leifheit, E. F., and Antunes, P. M. (2010). Mycelium of arbuscular mycorrhizal fungi increases soil water repellency and is sufficient to maintain water-stable soil aggregates. Soil Biol. Biochem. 42, 1189–1191. doi: 10.1016/j.soilbio.2010.03.027
Rillig, M. C., Ziersch, L., and Hempel, S. (2017). Microplastic transport in soil by earthworms. Sci. Rep. 7:1362. doi: 10.1038/s41598-017-01594-7
Schmiedgruber, M., Hufenus, R., and Mitrano, D. M. (2019). Mechanistic understanding of microplastic fiber fate and sampling strategies: Synthesis and utility of metal doped polyester fibers. Water Res. 155, 423–430. doi: 10.1016/j.watres.2019.02.044
Seta, A. K., and Karathanasis, A. D. (1996). Water dispersible colloids and factors influencing their dispersibility from soil aggregates. Geoderma 74, 255–266. doi: 10.1016/S0016-7061(96)00066-3
Singh, R. P., Mishra, S., and Das, A. P. (2020). Synthetic microfibers: Pollution toxicity and remediation. Chemosphere 257:127199. doi: 10.1016/j.chemosphere.2020.127199
Tisdall, J. M. (1994). Possible role of soil microorganisms in aggregation in soils. Plant Soil 159, 115–121. doi: 10.1007/BF00000100
Tisdall, J. M., and Oades, J. M. (1982). Organic matter and water-stable aggregates in soils. J. Soil Sci. 33, 141–163. doi: 10.1111/j.1365-2389.1982.tb01755.x
van den Berg, P., Huerta-Lwanga, E., Corradini, F., and Geissen, V. (2020). Sewage sludge application as a vehicle for microplastics in eastern Spanish agricultural soils. Environ. Pollut. 261:114198. doi: 10.1016/j.envpol.2020.114198
Wagner, S., Cattle, S. R., and Scholten, T. (2007). Soil-aggregate formation as influenced by clay content and organic-matter amendment. J. Plant Nutr. Soil Sci. 170, 173–180. doi: 10.1002/jpln.200521732
Waldrop, M. P., Balser, T. C., and Firestone, M. K. (2000). Linking microbial community composition to function in a tropical soil. Soil Biol. Biochem. 32, 1837–1846. doi: 10.1016/S0038-0717(00)00157-7
Widén, H., Leufvén, A., and Nielsen, T. (2004). Migration of model contaminants from PET bottles: influence of temperature, food simulant and functional barrier. Food Addit. Contam. 21, 993–1006. doi: 10.1080/02652030400009217
Wright, S. F., and Upadhyaya, A. (1998). A survey of soils for aggregate stability and glomalin, a glycoprotein produced by hyphae of arbuscular mycorrhizal fungi. Plant Soil 198, 97–107. doi: 10.1023/A:1004347701584
Yakimets, I., Lai, D., and Guigon, M. (2004). Effect of photo-oxidation cracks on behaviour of thick polypropylene samples. Polym. Degrad. Stab. 86, 59–67. doi: 10.1016/j.polymdegradstab.2004.01.013
Yu, H., Fan, P., Hou, J., Dang, Q., Cui, D., Xi, B., et al. (2020). Inhibitory effect of microplastics on soil extracellular enzymatic activities by changing soil properties and direct adsorption: An investigation at the aggregate-fraction level. Environ. Pollut. 267:115544. doi: 10.1016/j.envpol.2020.115544
Zettler, E. R., Mincer, T. J., and Amaral-Zettler, L. A. (2013). Life in the “plastisphere”: Microbial communities on plastic marine debris. Environ. Sci. Technol. 47, 7137–7146. doi: 10.1021/es401288x
Zhang, G. S., and Zhang, F. X. (2020). Variations in aggregate-associated organic carbon and polyester microfibers resulting from polyester microfibers addition in a clayey soil. Environ. Pollut. 258:113716. doi: 10.1016/j.envpol.2019.113716
Zhang, G. S., Zhang, F. X., and Li, X. T. (2019a). Effects of polyester microfibers on soil physical properties: Perception from a field and a pot experiment. Sci. Total Environ. 670, 1–7. doi: 10.1016/j.scitotenv.2019.03.149
Zhang, G. S., Zhang, F. X., and Li, X. T. (2019b). Effects of polyester microfibers on soil physical properties: Perception from a field and a pot experiment. Sci. Total Environ. 670, 1–7.
Zhang, L., Xie, Y., Liu, J., Zhong, S., Qian, Y., and Gao, P. (2020). An Overlooked Entry Pathway of Microplastics into Agricultural Soils from Application of Sludge-Based Fertilizers. Environ. Sci. Technol. 54, 4248–4255. doi: 10.1021/acs.est.9b07905
Zhang, M., Zhao, Y., Qin, X., Jia, W., Chai, L., Huang, M., et al. (2019c). Microplastics from mulching film is a distinct habitat for bacteria in farmland soil. Sci. Total Environ. 688, 470–478. doi: 10.1016/j.scitotenv.2019.06.108
Zhao, H., Jiang, Y., Ning, P., Liu, J., Zheng, W., Tian, X., et al. (2019). Effect of Different Straw Return Modes on Soil Bacterial Community, Enzyme Activities and Organic Carbon Fractions. Soil Sci. Soc. Am. J. 83, 638–648. doi: 10.2136/sssaj2018.03.0101
Zhou, Q., Zhang, H., Fu, C., Zhou, Y., Dai, Z., Li, Y., et al. (2018). The distribution and morphology of microplastics in coastal soils adjacent to the Bohai Sea and the Yellow Sea. Geoderma 322, 201–208. doi: 10.1016/j.geoderma.2018.02.015
Keywords: microplastic, organic matter, soil aggregate stability, enzyme activity, soil structure, soil health, plastic pollution
Citation: Liang Y, Lehmann A, Yang G, Leifheit EF and Rillig MC (2021) Effects of Microplastic Fibers on Soil Aggregation and Enzyme Activities Are Organic Matter Dependent. Front. Environ. Sci. 9:650155. doi: 10.3389/fenvs.2021.650155
Received: 06 January 2021; Accepted: 17 March 2021;
Published: 06 April 2021.
Edited by:
C. Marjorie Aelion, University of Massachusetts Amherst, United StatesReviewed by:
Denise M. Mitrano, ETH Zürich, SwitzerlandXiaomei Yang, Wageningen University and Research, Netherlands
Joana Correia Prata, University of Aveiro, Portugal
Copyright © 2021 Liang, Lehmann, Yang, Leifheit and Rillig. This is an open-access article distributed under the terms of the Creative Commons Attribution License (CC BY). The use, distribution or reproduction in other forums is permitted, provided the original author(s) and the copyright owner(s) are credited and that the original publication in this journal is cited, in accordance with accepted academic practice. No use, distribution or reproduction is permitted which does not comply with these terms.
*Correspondence: Matthias C. Rillig, cmlsbGlnQHplZGF0LmZ1LWJlcmxpbi5kZQ==