- 1School of Geographic and Environmental Sciences, Guizhou Normal University, Guiyang, China
- 2State Key Laboratory of Environmental Geochemistry, Institute of Geochemistry, Chinese Academy of Sciences, Guiyang, China
- 3University of Chinese Academy of Sciences, Beijing, China
- 4College of Biological and Environmental Engineering, Guiyang University, Guiyang, China
Macrophyte-dominated eutrophication (MDE) lakes have attracted wide attention due to the high phosphorus (P) loading in sediments that poses a wide spread risk for P release and pollution management. However, because of the superior productivity characteristics, the role of organic P mineralization in sediments in the internal P loading of MDE lake is still under debate. This study investigated the release dynamic of P in the sediments of Lake Caohai, a MDE lake in southwest of China, using a combination of the modified Huffer sequential extraction method, 31P nuclear magnetic resonance spectroscopy (NMR), and composite diffusive gradient in thin films (DGT) technology. Results showed that the apparent P diffusion flux at the sediment-water interface was remarkably high, with a mean value of 0.37 mg m−2 d−1. The phosphate ester organophosphorus components (i.e., Mono-P and Diester-P) continuously deposited and degraded in the sediments maintained the high productivity of the lake, and the mineralization process plays a critical role in the release of internal P. Although the content of inorganic P in sediment is relatively high (accounting for approximately 60% of total P), the reductive mechanism based on P-containing iron oxide/hydroxide has a low contribution to the internal P loading, as was indicated by the low release rate of P-combination iron-manganese (Fe-Mn)/iron-aluminum (Fe-Al) (BD-P and NaOH-P) and the insignificant positive correlations between DGT-labile P and DGT-labile Fe in the sediment cores. Additionally, organic P in sediments could transfer to P-combination Fe-Al/Fe-Mn. However, in severely expropriated environments, the enrichment of P-combination Fe-Al/Fe-Mn in surface sediments inhibited the mineralization of monophosphate to some degree. Taken together, this study emphasized the impact of sediment organic P loading on the release of internal P in lake, highlighting that organic P is also the valuable objects for avoiding eutrophication of MDE lakes.
1 Introduction
Phosphorus (P), as a limiting nutrient, is very important to lake ecosystems, but its excessive enrichment leads to eutrophication (Álvarez et al., 2017; Lin et al., 2021). Sediments is an important source and sink of P in water, and plays a critical role in the deposition, transformation, and recycling of nutrients in the lake system (Chen et al., 2020; Pu et al., 2020). Studies have substantiated that the release of P in sediments significantly affected the concentration of P in the lake water as well as the migration and transformation of P at the sediment-water interface (SWI) (Recknagel et al., 1995; Wang et al., 2021). Even when the input of external P is reduced, the internal P loading could lead to continuous eutrophication of the lake (Zhang et al., 2013; Wang et al., 2021). Therefore, understanding the biological availability and release dynamic of sediment P is of profound significance for the management and restoration of eutrophic lake ecosystems.
Different P forms in sediments have different bioavailability, and are released by changes in lake environmental conditions, to become a potential source contributing to lake eutrophication. Typically, sediment P can be divided into inorganic P (Pi) and organic P (Po) (Hupfer et al., 1995; Pu et al., 2020). Among these, biologically available P and detrital Po are considered as potential P reservoirs of phosphate in water, and their migration and transformation at the SWI have been the focus of research (Rydin 2000; Yang et al., 2020). However, although Po is the main component of lake sedimentary P, it has received much less attention than Pi in the past owing to the limitations of state-of-the art measurement methods (Bai et al., 2009; Lü et al., 2016). Therefore, a thorough understanding of the chemical dynamic of Po and Pi in sediments is vital for predicting the eutrophication process of lakes and the biogeochemical cycle of P.
The biogeochemical process of P at the SWI of macrophyte-dominant shallow lakes (a shallow lake aquatic ecosystem dominated by submerged plants) is controlled by the stability of different forms of P that was affected by various physical, chemical, and biological processes (Slomp et al., 2013; Barik et al., 2019). Prior studies documented that the reductive dissolution of P-containing iron oxide/hydroxide was the main mechanism controlling P migration in sediments (Spears et al., 2007; Tammeorg et al., 2020). However, due to the large amounts of organophosphorus components in the sediments of macrophyte-dominant shallow lakes, the degraded Po may also be an important source of P in the overlying water (Ryuichiro et al., 2017; Zhu et al., 2018). The combination of sequential P fractionation and solution 31P NMR spectroscopy proved that soluble organophosphates (mainly phosphate esters and phospholipids), which are susceptible to biological or chemical decomposition, are the preferred source of P for algae and bacteria in water (Bai et al., 2009; Zhu et al., 2018). Although the release of internal P in macrophyte-dominant shallow lakes has been studied, there is still a lack of quantitative understanding of the source of P released from the sediments and the contribution of P to the overlying water.
In the current study, we aimed at improving the understanding of the mechanisms responsible for the sediments P release in a macrophyte-dominant shallow lake, Lake Caohai. To this end, we collected 22 surface sediments and 3 core samples from Lake Caohai to identify whether sediment P release caused by the mineralization and degradation of Po, using a combination of modified Huffer sequential extraction method and 31P nuclear magnetic resonance spectroscopy (NMR). We further used in-situ, dynamic, high-resolution composite diffusive gradient in thin films (DGT) technology to quantify the release rate of internal P and evaluate the effectiveness of P released into lake water.
2 Materials and Methods
2.1 Study Area
Lake Caohai (26°47'∼26°52'N, 104°10'∼104°20'E) is located at the top of the central plateau of the foothills of the Guizhou Wumeng hinterland, with Guizhou being the largest natural freshwater lake in China (Figure 1). The area of the lake is about 25 km2, and the water storage capacity is about 0.6 × 108 m3 (Sun et al., 2020). The surface sediments environment of different lake areas is relatively stable, and the carbon-nitrogen ratio in the sediment cores profile change little (Yang et al., 2017). Lake Caohai is situated in a subtropical humid monsoon climate, with prevailing southwesterly wind, average annual rainfall of about 950.9 mm, and a relative humidity of 79%. Maximum lake depth is 5.3 m, with an average of 1.6 m. The organic matter content in the sediments is rich (average: 233.0 mg g−1), and the underlying bedrock is composed of Carboniferous carbonate rock (Yin et al., 2020).
The selection of Caohai because the aquatic ecosystem of Lake Caohai is gradually transformed into an algae-type lake aquatic ecosystem, and the content of organic matter in the sediments is higher (Xia et al., 2020b; Xia et al., 2021). The eastern part of Lake Caohai (including the upper reaches of the river into the lake) is a turbid water area with serious eutrophication, while the west (including the outlet) is a macrophyte-dominant area with abundant aquatic plants. There is serious pollution from heavy metals such as Pb, Zn and Cd in the sediments, which is mainly concentrated in the southern part of the lake (Xia et al., 2020a). Most of the rivers entering the lake are in the east and west, and the only outlet is located in the northwest. Among them, upstream Zhonghe River and Baima River are the main sources of external pollution (domestic and sewage production). Slow-dissolved mounds of the semi-closed plateau lead to a longer retention time (85.6 days), meaning that pollutants are more likely to be adsorbed and precipitated into sediments (Cao et al., 2016).
2.2 Sampling and Analysis
In July 2020, a stainless-steel water sampler was used to collect 22 water samples (Sl∼S22) from the surface of Lake Caohai (0.5 m below the surface). All water samples were stored in clean polyethylene bottles and kept at low temperature (<4°C) before being sent to the laboratory for analysis as soon as possible. The network method is used for the layout of sample points, and GPS is used to determine the coordinates of the sample points on site. Water quality parameters such as pH, Dissolved oxygen (DO) and Conductivity (EC) needed to be measured in situ using a portable multi-parameter water quality analyzer (model YSI EXO-2).
Surface sediments (S1∼S22) were collected with a Petersen grab sampler, and sediment cores were collected with the undisturbed lake SWI barycenter sampler (120 cm in length, 5.9 cm in diameter) and DGT columnar sediment sampler (Easy Sensor Ltd., Nanjing, China, Zr-Oxide fixed membrane (membrane length: 15 cm; width: 2 cm; thickness: 0.04 cm), agarose diffusion membrane (membrane length: 15 cm; width: 2 cm; thickness: 0.08 cm)) in the western macrophyte-dominant area (S2), the central transition area (S11) and the eastern eutrophic area (S13). The cylinder cores were cut on site at 1 cm interval, and all sediment samples were packed in 50 ml centrifuge tubes at low temperature (<4°C). After transforming to the laboratory, a portion of surface sediment samples were taken in order to determine the porosity, and the remaining sediments were centrifuged (MKE-VCK-22R, 4000 r/min) to obtain sediments pore water. The resulting solid samples were freeze-dried (Techconp FD-3-85-MP) followed by grinding to a powder using an agate mortar (below 120 meshs). For DGT measurements, the Zr-Oxide-DGT membrane probe was inserted into the DGT cores. After 24 h of reaction the membrane was removed, washed with ultrapure water, sealed at 4°C, and sent to the laboratory for analysis.
The concentrations of total P (TP) and soluble reactive P (SRP) in the lake’s overlying water and surface sediments pore water were determined by ammonium molybdate spectrophotometry (Murphy and Riley 1962), with the instrument detection limit of 0.01 mg L−1. The sediment P components were extracted by the modified Huffer sequential extraction method (Hupfer et al., 1995), which included 1) loosely adsorbed P (NH4Cl-P); 2) iron-manganese combined state P (BD-P); 3) iron-aluminum combined state P (NaOH-Pi); 4) calcium-bound P (HCl-P); 5) biological detrital organic P (NaOH-Po); and 6) residual P (Res-P). To ensure the reliability of the data in the determination process, we tested the samples by inserting standard materials with the results suggested that the extraction recovery rate of the standard materials and sediment samples was both greater than 86%. The P content in these extracts was determined by ammonium molybdate spectrophotometry after the samples had been centrifuged and filtered through a 0.45 m acetate fiber membrane (Murphy and Riley 1962). The porosity of the core sediments is determined based on the wet weight and dry weight of the top sample, and the specific method is referred to (Crusius and Anderson 1991). The concentrations of one-dimensional labile P (DGT-labile P) were determined by fixed film section-extraction microcalorimetry method, and the one-dimensional labile Fe (DGT-labile Fe) were determined using the phenanthroline colorimetric method, with an average error of less than 4% (Ding et al., 2015).
In 31P NMR, sediment samples were analyzed at room temperature with 0.25 mol L−1 NaOH and 50 mmol L−1 EDTA mixed solution was extracted; the sediments mass ratio of the extraction and the extraction time were 1:10 and 16 h, respectively. After the extract was refrigerated and centrifuged for 40 min, part of the supernatant (5 ml) was diluted to determine the concentration of TP and inorganic P (Pi) in the extract (Murphy and Riley 1962), and the concentration of organic P (Po) was determined by the difference between TP and Pi. The remaining extracted liquid was freeze-dried, and weighed 2 g of the sample was dissolved in a mixed solution of 10 mol L−1 NaOH and D2O, followed by ultrasound treatment at room temperature (frequency 40 kHz, time 5 min) and high-speed centrifugation (speed 8000 r/min, time 40 min), before being sent to the Center for Nuclear Magnetic Resonance of Peking University (BRKERAV 400) for testing (Cade-Menun and Lin 2013).
The pulse of the 31P NMR spectrum was 12 usec, the cycle delay was dl = 3.6 s, the measurement temperature was 25°C, the sweep was about 20,000 times, and the resonance frequency was 242.925 MHz. All 31P-NMR chemical shifts were based on 85% orthophosphate acid.
2.3 Estimation of P Release Flux at the SWI
According to Fick’s first law, the concentration of labile P at the SWI measured by DGT was calculated using the equation:
where CDGT is the concentration of target matter (mg·L−1); M is the accumulated amount of ZrO-Chelex DGT thin film within the sampling time (μg); ∆g is the thickness of the diffusive layer (cm); D is the molecular diffusive coefficient of the phosphate in the diffusion layer (cm2 s−1); A represents the sampling area (cm2); and t is the sample standing time (s) (Chen et al., 2019).
According to the concentration profile of DGT-labile P in the vicinity of the SWI, the apparent diffusion flux of P (Fd) in the sediments can be calculated as follows:
where Jw and Js are the diffusive fluxes of P from the overlying water to the SWI and from the sediments to the SWI, respectively; Dw and Ds are the diffusion coefficients of phosphates in the overlying water and sediments, respectively; φ is the porosity of the Lake surface sediments; and
In order to obtain an accurate diffusion flux value, we used the concentration rake ratio of DGT-labile P at the SWI to fit the concentration gradients. The Ds can be calculated as following:
where F is the resistivity coefficient of the stratum; and the F can be determined by the φ calculation, when φ ≥ 0.7, F = 1 /φ3; φ < 0.7, F = 1 /φ2 (Ullman and Aller 1982).
2.4 Analysis of Sediments P Loading in Lake Caohai
Assuming that molecular diffusion is the main transport pathway of phosphate release in the sediments of Lake Caohai, and the water in the basin is well mixed (Ding et al., 2018), the total dissolved phosphate concentration (Mp) and contribution rate (f) from the sediments interstitial water to the overlying water can be calculated as follows:
where Fd is the release flux of P in sediments; A is the lake area represented by each sediments core; Tw is the residence time of the water; C is the concentration of total dissolved phosphate in the water; and H is the water depth.
3 Results and Discussion
3.1 Water Properties
Since the water depth of Lake Caohai is relatively shallow (1.6 m on average) and the hydrodynamic effect is strong, the profile change of lake water quality parameters is not obvious (Table 1). The high DO and pH in the lake are mainly due to the intense photosynthesis of aquatic plants in summer (Welch and Cooke 2005). The eastern lake area (S7, S13, S19) had higher EC and lower DO and pH, which were associated to the direct discharge of domestic sewage in the lake area. Concentrations of TP and SRP in the overlying water and pore water of surface sediments were higher in the east and lower in the west (Figure 2), which was consistent with the water quality report of Liu et al. (2020). It is worth noting that there was a large difference in TP and SRP between the overlying water and pore water, showing the sharp gradient diffusion of P at the sediment-overlying water interfaces. This creates the necessary conditions for the release of internal P into the overlying water.
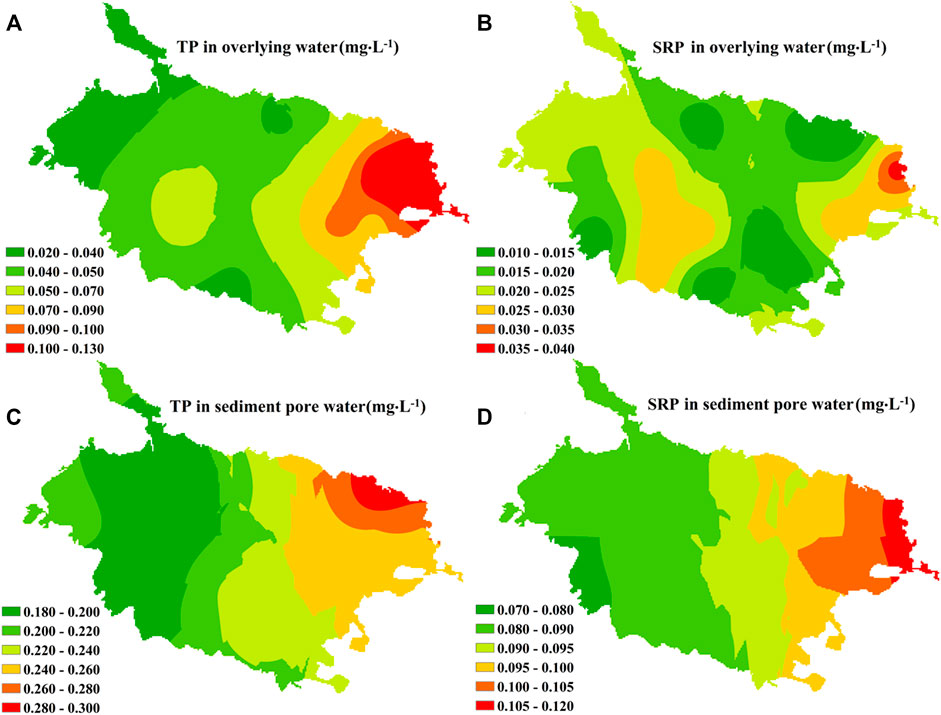
FIGURE 2. Concentrations and spatial distribution of TP and SRP in overlying waters and surface sediment pore waters of Lake Caohai. (A, B) are the overlying water TP and SRP, respectively. (C, D) are the surface sediment pore water TP and SRP, respectively.
In addition, we used the modified Fick’s first diffusion law and the static release calculation method of P at the SWI to estimate the interstitial P diffusion rate in the surface sediments of Lake Caohai (see Ullman and Aller (1982); Tammeorg et al. (2020) for detail calculations, giving the diffusion value of 5.0 × 10–6 cm−2 s−1). Results showed that the interstitial P diffusion rate of Lake Caohai surface sediments to the overlying water was 0.03–0.28 mg m−2 d−1, and the average flux of TP and SRP were 0.09 mg m−2 d−1 and 0.07 mg m−2 d−1, respectively. The significant positive correlation between the internal P flux and the overlying water TP (r = 0.84, p < 0.05) and SRP (r = 0.74, p < 0.05) indicates that surface sediments are mainly one of the sources of P in the P cycle of Lake Caohai. Simultaneously, the differences in internal P fluxes between different aeras also indicate that the internal P load in the lake is related to the nutrient status of the lake, and high nutrient areas are usually accompanied by higher internal pollution (Supplementary Table S1). However, since we neglected the vertical difference of P concentration in the lake overlying water and the depth of the water in the calculation process, the result was far lower than the DGT technology described later (see Section 3.5).
3.2 Fractionation and Distribution of P
Synchronous variations were found between surface sediments TP and overlying water TP and SRP in the spatial distribution (Figure 3A). The eutrophic lake area in the eastern was significantly higher than other areas of the lake, with the maximum of 1236.8 mg kg−1, and the average content of the entire lake is 692.9 mg kg−1. Although the mean content of TP in sediments was much lower compared to shallow lakes in southwest China such as Dianchi Lake (2050.0 mg kg−1) and Erhai Lake (999.0 mg kg−1) (Zhang et al., 2013; Chen et al., 2020), the sediment TP content of most sample sites of Lake Caohai was higher than the minimum toxicity value (600.0 mg kg−1) of P in the sediment quality assessment standard of Ontario, Canada, which is a moderate pollution level. Pi components of NH4Cl-P, BD-P, NaOH-Pi and HCl-P are the main P forms, accounting for about 66% of the TP in the sediments, these four major P concentrations reached 15.4 mg kg−1, 227.2 mg kg−1, 207.2 mg kg−1 and 49.5 mg kg−1, respectively. The mean contents of NaOH-Po and Res-P organophosphorus components were 117.1 mg kg−1 and 138.9 mg kg−1, respectively, amounting to 15 and 18% of TP in sediments (Figure 4).
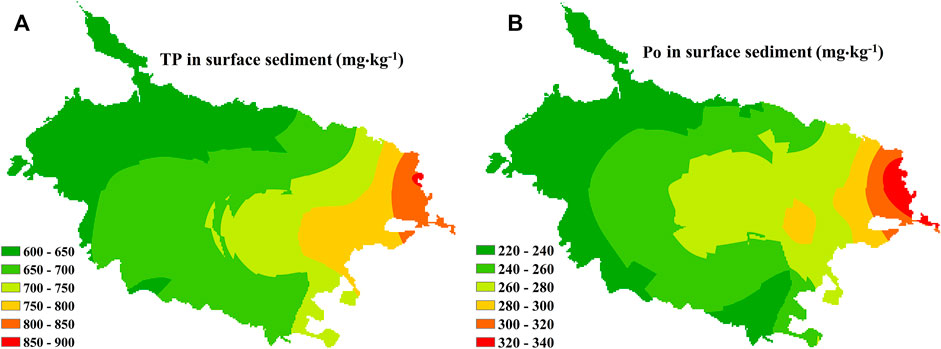
FIGURE 3. (A, B) are the content and spatial distribution of TP and Po in the surface sediments of Lake Caohai, respectively. Po is the sum of biological detrital organic P (NaOH-Po) and residual P (Res-P).
Bioavailable P (BAP), composed of NH4Cl-P, BD-P and NaOH-Pi, can characterize the content of internal-P available to primary producers in the lake (Kwak et al., 2018; Mu et al., 2020). The content of BAP in the surface sediments of Lake Caohai ranged from 335.7 mg kg−1 to 628.4 mg kg−1, accounting for about 60% of the TP in the sediments. Since BAP is relatively easy to release and is easily bio-utilized, the content of BAP is relatively lower in the sediments of macrophyte-dominant lakes with better water quality. However, in eutrophic lakes greatly affected by human activities, the content of BAP in the sediments is relatively high (Supplementary Figure S1), indicating that BAP content is related to the degree of disturbance by human activities and the type of aquatic ecosystem in the lakes. In other words, the more polluted the lake, the higher the TP content in the sediments, and the higher the content of BAP. It is worth noting that the spatial distribution of BD-P and NaOH-Pi in Lake Caohai showed a similar pattern, with high concentrations mainly distributed in the middle and on the south bank of the lake (Supplementary Figure S2). This distribution pattern is very similar to the variation of P (BD-P and NaOH-Pi) in the sediments of Lake Erie, and may be related to the interaction of the external P loading and the lake circulation model (Wang et al., 2021). In addition, the abundant accumulation of heavy metals such as Pb and Zn in the sediments of the southern lake area may also be an important reason for this phenomenon, because the P components adsorbed by such heavy metals are mainly in the form of Fe-Al/Fe-Mn combined P in the fractional extraction of sediments (Liang et al., 2018; He et al., 2019). As a calcium-bound type of P, HCl-P has strong stability and can be published to a certain extent under acidic conditions (Rydin 2000; Wang et al., 2021). Lake Caohai is a weakly alkaline water (pH > 8.8), and it is difficult for HCl-P to participate in the P cycle in the lake system. The high content of HCl-P in the sediments of the eastern lake area is mainly due to the co-precipitation of a large amount of calcium ions and phosphate caused by man-made discharge (Barik et al., 2019; Mu et al., 2020) (Supplementary Figure S2).
Organic phosphate mineralization has been shown to be one of the main mechanisms for P release from lake sediments and plays a critical role in the release of internal P in some lakes (Torres et al., 2014; Tu et al., 2019). The content of NaOH-Po and Res-P in sediments can be used to characterize the degree of incomplete mineralization and humification of organic matter loading deposition in water environments (Kleeberg and Dudel 1997). In this study, content distribution of the NaOH-Po and Res-P was also consistent with the nutritional level of the lake area, reflecting the influence of human activities and organic matter accumulation (Figure 3B). These Po components are a potential source of P concentration in the overlying water of the lake, because Po types such as magnolias and diesters of orthophosphate under anoxic conditions degraded to inorganic P that were subsequently sorbed and utilized by organisms (Joshi et al., 2015; Tu et al., 2019). In conclusion, the content differences in P forms between sediments of different lakes and different points in the same lake area are closely related to the nutrient state of the lake and the type of aquatic ecosystem in the lake area, and aquatic plants have a good controlling effect on the activity of P in sediments.
3.3 Release and Variation of P Fraction
The biogeochemical cycle of P in lake sediments and overlying water is closely related to the change in different P forms in sediments (Wang et al., 2006; Wang et al., 2021), and the content of P (BAP and Po) potentially available for biological absorption and utilization is considered to be the key factor affecting the P cycle in the lake (Xiang and Zhou 2011). According to the average deposition rate of Lake Caohai sediments (0.27 cm yr−1) measured by Zhu et al. (2013), the sedimentary depth of 0–14 cm in the study area could be used to characterize the sedimentary records of Lake Caohai since its impoundment in 1969. Our sediments TP data shows that the internal P loading of Lake Caohai shows a trend of increasing (Supplementary Figure S3).
The contents of NH4Cl-P, NaOH-Po, HCl-P and Res-P decreased significantly with the increase in deposition depth, and their average fast release and sedimentation rates (If the release and sedimentation rates is positive, it indicates that this form of P is mainly released; otherwise, it is mainly retained.), which were calculated according to Hupfer and Lewandowski (2005), were 2, 16, 0.5 and 5% respectively (Figure 5). These results indicate that these P forms are to some extent engaged in the P cycle of the lake. The contents of NaOH-Pi and BD-P in the profile increased at first and then decreased or remained stable, indicating that BD-P and NaOH-Pi were the main forms of P deposition in the sediments of Lake Caohai. This may be related to oxygen transport behavior of submerged plant roots to the rhizosphere sediments and the DO and organic-rich environment in Lake Caohai (Stephen et al., 1997; Xu et al., 2018). Because oxygen secreted by plant roots can lead to the oxidation of low-state metal compounds such as Fe and Mn into high-state compounds in the rhizosphere sediments, insoluble high-state metal oxides such as Fe/Al-P and Fe/Mn-P are more likely to accumulate in the rhizosphere sediments (Søndergaard et al., 2003). Further, the high DO concentration in the Lake water (>6.0 mg L−1) will also inhibit the reduction of Fe/Al-P, Fe/Mn-P and other metal oxides in the sediments (Stephen et al., 1997; Xu et al., 2018), and the organic matter mineralization can also cause changes in the redox conditions and pH values, thus affecting the migration and transformation of BD-P and NaOH-Pi in sediments (Joshi et al., 2015; Lü et al., 2016).
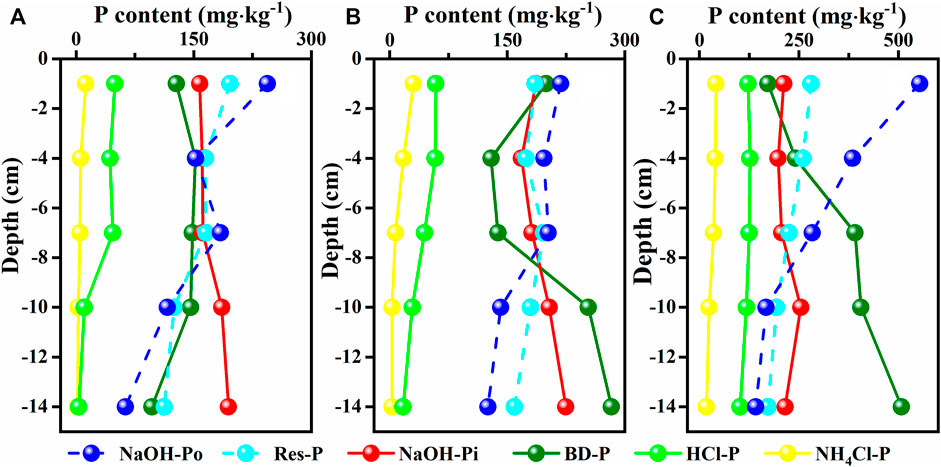
FIGURE 5. Vertical profiles of P forms in the sediments from Lake Caohai. (A–C) represent the changes of P content in the macrophyte-dominant zone of Lake Caohai, the central transition zone, and the eastern eutrophic zone in the high flux season, respectively.
The Po mineralization of sediments may play a role in the biogeochemical cycle of P in the lake. In general, due to Po mineralization, the Po content in sediment decreases with increasing sedimentary depth (Pu et al., 2020; Wang et al., 2021). The average contents of NaOH-Po and Res-P in the 1–14 cm sediment of Lake Caohai were 211.7 mg kg−1 and 186.0 mg kg−1, respectively. The contents of potentially reversible NaOH-Po and Res-P in the top two layers of the cores (1 and 4 cm) accounted for about 55 and 14% of the internal TP released in the lake. The eutrophic area even reached 82 and 10%. In particular, the rate of Po mineralization in sediment profiles of eutrophicated lakes is significantly higher than that in macrophyte-dominant lakes and transition areas, due to a positive feedback mechanism between eutrophication-driven hypoxia and internal P release (Carey and Rydin 2011). On the other hand, the variation of Pi contents in the core could not explain the high internal P flux in the lake (see Section 3.5 for details). Although about 78% NH4Cl-P and 60% HCl-P in 1–14 cm sediments can be released into the overlying water, their contents are relatively low, accounting for only 7 and 13% of internal TP release on average. However, the high content of BD-P and NaOH-Pi in the surface sediments showed an accumulation trend, with average slow release and sedimentation rates of −19% and −7%, respectively. It is worth noting that the differences in BD-P and NaOH-Pi contents between sediment with different depths (1–10 cm) increased with increasing nutrient level. This difference is obviously related to the distribution of aquatic plants and the difference in hydrodynamic conditions for different lakes. In lakes with abundant aquatic plants, macrophytes have a great demand for P in the water and sediments during the growing season, which leads to the available P in the sediments, such as BD-P and NaOH-Pi, being absorbed by aquatic plants (Søndergaard et al., 2003; Zhu et al., 2006). This suggests that aquatic plants play an important role in the biogeochemical cycle of P in macrophyte-dominant lakes such as Lake Caohai, and the release and sedimentation rates of BD-P and NaOH-Pi in sediments may be underestimated.
3.4 31P NMR Spectroscopy
As shown in Table 2, the low TP extraction rate (average 45%) and high Po recovery rate (average 74%) in the extract demonstrated that NaOH-EDTA could effectively extract Po components from the sediments, and indicated that the information on P forms extracted by NMR could basically characterize the actual nature of the variation in Po in the sediments of Lake Caohai. Almost all 31P NMR P in the profile decreased with increasing deposition depth, indicating that all forms of P mineralized, degraded or transformed in the deep sediments (Golterman 1996; Zhu et al., 2018). However, the increase of orthophosphate (Ortho-P, 5–7 ppm) content in deep sediments may be related to the high contents of orthophosphate monoester (Mono-P, 3–6 ppm), orthophosphate diester (Diester-P, 2.5 to −1 ppm) and pyrophosphate (Pyro-P, −3 to −5 ppm) in the sediment profiles as well as the mineralization rate (Figure 6). Mono-P, Diester-P, and Pyro-P are rapidly converted to orthophosphates under anoxic environments and released into the overlying water (Golterman et al., 1998; Zhang et al., 2014; Zhu et al., 2018).
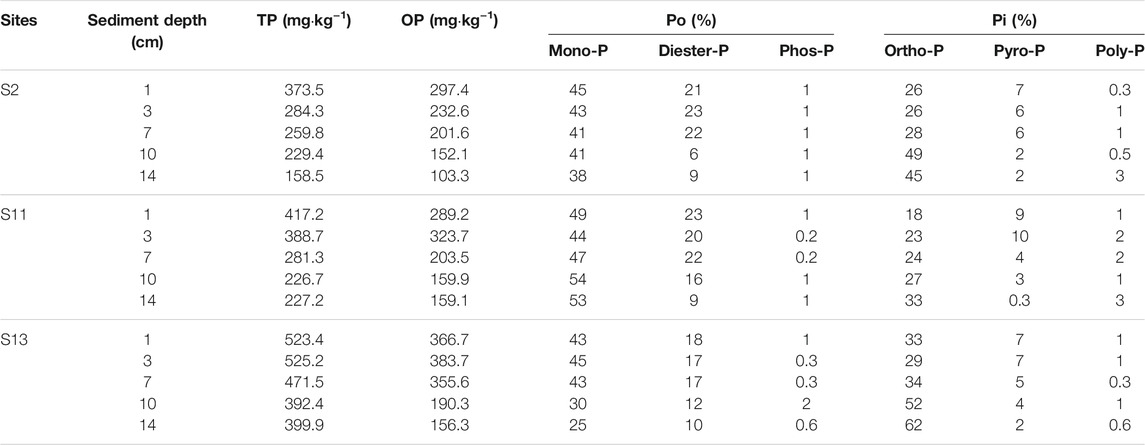
TABLE 2. Content and proportion of different forms of phosphorus in Lake Caohai Sediments detected by 31P NMR spectroscopy.
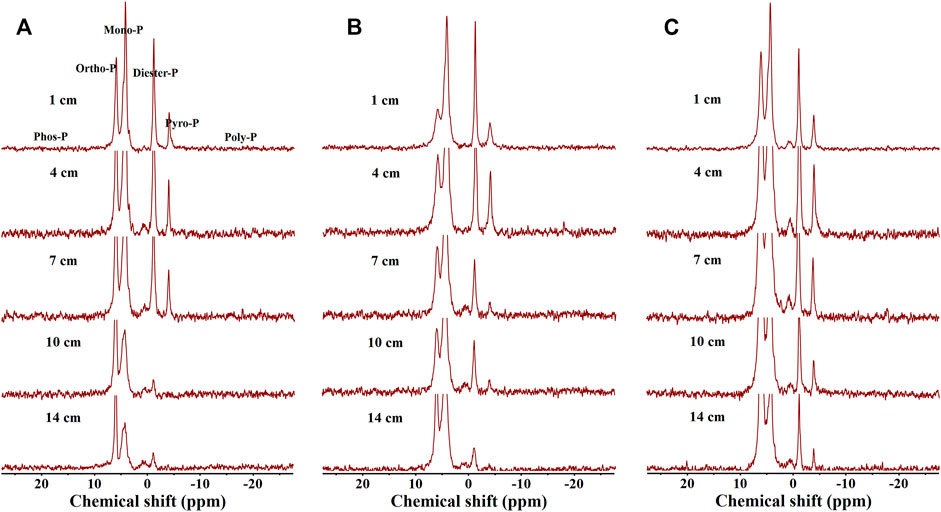
FIGURE 6. 31P NMR spectrums of NaOH-EDTA extracts from the different sediment profile in Lake Caohai. (A–C) are the 31P NMR vertical changes of the sediment cores macrophyte-dominant zone, the central transition zone, and the eastern eutrophic zone of Lake Caohai in the high flux season, respectively. The31P NMR chemical shifts suggest the presence of phosphonate (7–20 ppm), orthophosphate (5–7 ppm), phosphate monoester (3–6 ppm), phosphodiester (−1–2.5 ppm), DNA (0 to −1 ppm), pyrophosphate (−3 to −5 ppm) and polyphosphate (−20 to −5 ppm).
Diester-P, which is composed of inositol phosphoric acid, sugar phosphoric acid and phytic acid, and nucleic acid (DNA, RNA), phospholipids and teichoic acid, occupies an absolute dominance in 31P NMR organophosphorus forms, with an average content of 146.0 mg kg−1 and 58.4 mg kg−1, or 43 and 16% of the extracted TP, respectively. Although the spatial variation of such P content in sediments in the basin was consistent with the distribution of P in water, the overall contents of Mono-P and Diester-P in sediments in Lake Caohai were relatively high owing to the influence of water quality and the distribution of aquatic organisms. This is consistent with the large amount of bioclasts and DNA-P deposited in surface sediments. The content of DNA-P (0 to −1 ppm) in Diester-P can characterize the abundance of microorganisms in the sediments (Reitzel et al., 2006; Zhang et al., 2013). The vertical distribution and migration of P in the sediment profiles indicated that the mineralization and mineralization of Mono-P and Diester-P were the main source of authigenic P in the lake sediments. The content of Ortho-P in different sediment cores gradually became the dominant component with the increase in deposition depth (1–14 cm), and the average slow release and sedimentation rates of Mono-P and Diester-P reached 44 and 28%, respectively. According to the changes in P forms content, the Po mineralization in the sediments of Lake Caohai was mainly transferred to BD-P and NaOH-Pi. In the lake system, Pyro-P was easily decomposed and released by microorganisms (the average slow release and sedimentation rates was 12%). Due to its low content, which accounted for only 4% of TP, and had a maximum of 38.7 mg kg−1, decomposition of Pyro-P in sediments contributed little to the loading of internal P in the lake. In addition, trace amounts of phosphonate (Phos-P, 7–20 ppm) and polyphosphate (Ploy-P, −5 to −20 ppm) were detected, which accounted for only 2% of TP, and there was no obvious regular change in each column core. Since Phos-P and Poly-P are mainly produced by biosynthesis or metabolism, anaerobic conditions are not conducive to the accumulation of such P (Carman et al., 2000; Dong et al., 2012), so it was speculated that the hydrolysis of Po such as Mono-P and Diester-P during the extraction and concentration of NaOH-EDTA was the main reason for the small amount of Phos-P and Poly-P in the extraction solution (Cade-Menun 2005).
31P NMR results further suggested that the P content of almost all 31P NMR increased significantly with the increase in nutrient level in the lake, which was consistent with the classification results of the Huffer method described above. Except for a trace of Phos-P and Poly-P, the contents of various 31P NMR in surface sediments of eutrophication areas were significantly higher than those in macrophyte-dominant and transition areas, possibly due to the higher external P loading and relatively lower release of P from sediments in the early eutrophication period of the lake (Carey and Rydin 2011). Interestingly, with the increase in nutrient level in the lake, the release and sedimentation rates of various 31P NMRs in the surface sediments (1–7 cm) of Lake Caohai changed significantly, and the release and sedimentation rates of Diester-P (28%) and Pyro-P (20%) in the eutrophic area increased by 38 and 53%, respectively, compared with those in the macrophyte-dominant lakes area. Ortho-P (5%) and Mono-P (41%) decreased by 73 and 21%, respectively. Combined with the migration and transformation characteristics of P in the sediment profiles, it is suggested that the abundant enrichment of Fe-Al/Fe-Mn oxides in surface sediments at the severe eutrophication level may inhibit the mineralization of Mono-P.
3.5 Biogeochemical Behavior of P in Sediments
Vertical profile variations of the DGT-labile P (Fe) concentrations at the SWI are summarized in Figure 7. All DGT-labile P (Fe) in the cores showed the characteristics of first increasing and then stabilizing or decreasing, and there was a higher concentration gradient at the SWI. According to the DGT-labile P diffusion model (see Section 2.3) to the SWI, the release flux of DGT-labile P from sediments in the macrophyte-dominant lake area, the transition area and the eutrophic lake area were 0.23 mg m−2 d−1, 0.29 mg m−2 d−1 and 0.58 mg m−2 d−1, respectively. The contribution of sediments P release to TP in the overlying water reached 21, 24 and 40%, and the internal P flux was significantly higher than other lakes, such as Lake Taihu (0.15 mg m−2 d−1) and Lake Dongting (0.09 mg m−2 d−1) (Ding et al., 2015; Gao et al., 2016). But it is also close to some shallow lakes, such as Lake Apopka (average 0.30–0.70 mg m−2 d−1) and Lake Beaver (average 0.48 mg m−2 d−1) (Olila et al., 1997; Bhadha et al., 2010). The normal water storage area of Lake Caohai is about 25 km2, of which the surface area of the eastern eutrophic lake is about 3 km2, and the water retention time of Lake Caohai is about 85.6 days (Cao et al., 2016). According to molecular diffusion theory (see Section 2.4), the season of high internal P flux in Lake Caohai was estimated to be 1.4 tons, of which the release amount (0.3 tons) in the eutrophic parts of the lake (only 12% of the total area of the lake), accounted for 23% of the total. This indicated that highly eutrophic lakes are usually coupled with a high intensity of internal P release. Similarly, many studies have confirmed that higher nutrient levels in lakes were associated with increased sediments P release (Torres et al., 2014; Tu et al., 2019).
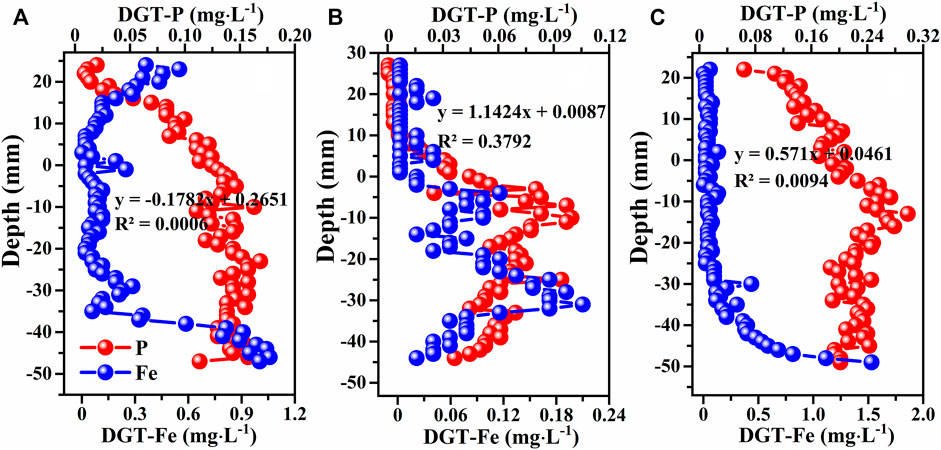
FIGURE 7. Variations of the DGT-labile P (Fe) concentrations in sediments of Lake Caohai. (A–C) represent the macrophyte-dominant zone, the central transition zone, and the eastern eutrophic zone DGT-labile P (Fe) concentration of Lake Caohai sediment cores during the high flux season, respectively. The location of the sediment-water interface is represented by zero. R2 represents the linear correlation coefficient between DGT-labile P and DGT-labile Fe.
From the spatial perspective, the concentration of P in the eutrophic area of Lake Caohai was much higher than that in the macrophyte-dominant lake area and the transition area, and the release flux of P in the sediments of this area was the highest. This indicated that the release of P in the sediments contributed significantly to the TP in the overlying water, and was related to the degree of internal pollution in different area of the lake. In previous studies, due to the limited depth of oxygen penetration into the sediments (only a few millimeters), the lower and middle sediments have been in a state of hypoxia (Cui et al., 2018; Chen et al., 2019). Therefore, the redox-controlled iron and P release mechanism is considered to be the main pathway for the dissolution and release of orthophosphates from anoxic lake sediments (Christophoridis and Fytianos 2006; Chen et al., 2019). However, the significantly irrelevant between DGT-labile P and DGT-labile Fe in the sediment profiles (R2 < 0.4, p < 0.05) confirms that the reductive dissolution of iron-bound P may not be the main source of P release from the sediments, combined with the migration and transformation characteristics of P in the sediment profiles, and the significant correlation between the concentrations of TP and SRP in the overlying water and the different P forms in the surface sediments (Supplementary Table S2, p < 0.05) indicate that the mineralization of organophosphorus may play a vital role in the release of internal P in Lake Caohai. This is similar to the results of Joshi et al. (2015). The Mono-P and Diester-P deposited and desorbed in the surface sediments effectively compensated for the phosphate needed for the growth of aquatic plants. At the same time, part of the residual phosphate was released into the overlying water, and part of the residual phosphate was adsorbed and stored by metal compounds in the sediments. Further analysis showed that the TP concentration in the overlying water of Lake Caohai was significantly positively correlated with NH4Cl-P, HCl-P, Res-P and NaOH-Po in the surface sediments (p < 0.05, Supplementary Table S2). In the sediment profiles, NH4Cl-P, HCl-P, Res-P and NaOH-Po were significantly positively correlated with Mono-P, Diester-P and Pyro-P (p < 0.05, Supplementary Table S3). In conclusion, the forms of Pi and Po in sediments play different roles in the MDE lakes. In comparison, the contribution of Po mineralization in sediments to lake eutrophication is greater than that of Pi in sediments. However, release flux of different P forms in sediments and their contribution to the TP in the overlying water needs further study.
4 Conclusion
This study reports the dynamic changes of P in the surface sediments of MDE lakes with high DO and organic-rich environment. A comparison the migration and transformation of P forms in the sediment profiles indicated that these compounds were involved in the P recycling to different extents and diagenetic transformation in this lake. According to the analysis of different P forms, the release and sedimentation rates of P-combination iron-manganese (Fe-Mn)/iron-aluminum (Fe-Al) (BD-P and NaOH-P) was low, and there are no significant positive correlations between DGT-labile P and DGT-labile Fe in the sediment profiles. In addition, the mineralization of organic P in the sediments could transfer to P-combination Fe-Al/Fe-Mn. However, in severely eutrophicated environments, the enrichment of P-combination Fe-Al/Fe-Mn in surface sediments inhibited the mineralization of monophosphate to some degree. Even though the data presented in this study are limited and cover a relatively shallow lake sediment depths of the examined lake, some useful conclusions can be drawn. The phosphate ester organophosphorus components (i.e., Mono-P and Diester-P) continuously deposited and degraded in the sediments maintained the high productivity of the lake, and the mineralization process plays a critical role in the release of internal P. Therefore, the organic P loading in sediments should be paid attention to in the internal P restoration of MDE lakes.
Data Availability Statement
The original contributions presented in the study are included in the article/Supplementary Material, further inquiries can be directed to the corresponding authors.
Author Contributions
WY, HY, and YL contributed to conception and design of the study. WY and QC organized the database and statistical analysis. WY wrote the first draft of the manuscript. HY, JC, PL, and YY guided the writing of thesis. All authors contributed to manuscript revision, read, and approved the submitted version.
Funding
This study was supported by the National Natural Science Foundation of China (No. 41807394, No. U1612441 and No. 41907279), the Science and Technology Project of Guizhou Province (QianKeHe Support (2020)4Y015), the Central Government Leading Local Science and Technology Development QianKeZhongYinDi (Grant number (2021)4028). 31P NMR measurements were performed at the mass spectrometry facility of National Center for Protein Sciences at Peking University.
Conflict of Interest
The authors declare that the research was conducted in the absence of any commercial or financial relationships that could be construed as a potential conflict of interest.
Publisher’s Note
All claims expressed in this article are solely those of the authors and do not necessarily represent those of their affiliated organizations, or those of the publisher, the editors and the reviewers. Any product that may be evaluated in this article, or claim that may be made by its manufacturer, is not guaranteed or endorsed by the publisher.
Supplementary Material
The Supplementary Material for this article can be found online at: https://www.frontiersin.org/articles/10.3389/fenvs.2021.812834/full#supplementary-material
References
Álvarez, X., Valero, E., Santos, R. M. B., Varandas, S. G. P., Fernandes, L. F. S., and Pacheco, F. A. L. (2017). Anthropogenic Nutrients and Eutrophication in Multiple Land Use Watersheds: Best Management Practices and Policies for the protection of Water Resources. Land Use Policy 69, 1–11. doi:10.1016/j.landusepol.2017.08.028
Bai, X., Ding, S., Fan, C., Liu, T., Shi, D., and Zhang, L. (2009). Organic Phosphorus Species in Surface Sediments of a Large, Shallow, Eutrophic lake, Lake Taihu, China. Environ. Pollut. 157 (8-9), 2507–2513. doi:10.1016/j.envpol.2009.03.018
Barik, S. K., Bramha, S., Bastia, T. K., Behera, D., Kumar, M., Mohanty, P. K., et al. (2019). Characteristics of Geochemical Fractions of Phosphorus and its Bioavailability in Sediments of a Largest Brackish Water lake, South Asia. Ecohydrology & Hydrobiology 19 (3), 370–382. doi:10.1016/j.ecohyd.2019.02.002
Bhadha, J. H., Jawitz, J. W., and Min, J. H. (2010). Phosphorus Mass Balance and Internal Load in an Impacted Subtropical Isolated Wetland. Water Air Soil Pollut. 218 (1-4), 619–632. doi:10.1007/s11270-010-0673-9
Cade-Menun, B. J. (2005). Characterizing Phosphorus in Environmental and Agricultural Samples by 31P Nuclear Magnetic Resonance Spectroscopy. Talanta 66 (2), 359–371. doi:10.1016/j.talanta.2004.12.024
Cade-Menun, B., and Liu, C. W. (2013). Solution Phosphorus-31 Nuclear Magnetic Resonance Spectroscopy of Soils from 2005 to 2013: A Review of Sample Preparation and Experimental Parameters. Soil Sci. Soc. America J. 78 (1), 19–37. doi:10.2136/sssaj2013.05.0187dgs
Cao, X., Wu, P., Han, Z., Zhang, S., and Tu, H. (2016). Sources, Spatial Distribution, and Seasonal Variation of Major Ions in the Caohai Wetland Catchment, Southwest China. Wetlands 36 (6), 1069–1085. doi:10.1007/s13157-016-0822-z
Carey, C. C., and Rydin, E. (2011). Lake Trophic Status Can Be Determined by the Depth Distribution of Sediment Phosphorus. Limnol. Oceanogr. 56 (6), 2051–2063. doi:10.4319/lo.2011.56.6.2051
Carman, R., Edlund, G., and Damberg, C. (2000). Distribution of Organic and Inorganic Phosphorus Compounds in Mrine and Lacustrine Sediments: a 31P NMR Study. Chem. Geology. 163 (1-4), 101–114. doi:10.1016/s0009-2541(99)00098-4
Chen, Q., Chen, J., Wang, J., Guo, J., Jin, Z., Yu, P., et al. (2019). In Situ, High-Resolution Evidence of Phosphorus Release from Sediments Controlled by the Reductive Dissolution of Iron-Bound Phosphorus in a Deep Reservoir, Southwestern China. Sci. Total Environ. 666, 39–45. doi:10.1016/j.scitotenv.2019.02.194
Chen, Q., Ni, Z., Wang, S., Guo, Y., and Liu, S. (2020). Climate Change and Human Activities Reduced the Burial Efficiency of Nitrogen and Phosphorus in Sediment from Dianchi Lake, China. J. Clean. Prod. 274, 122839. doi:10.1016/j.jclepro.2020.122839
Christophoridis, C., and Fytianos, K. (2006). Conditions Affecting the Release of Phosphorus from Surface lake Sediments. J. Environ. Qual. 35 (4), 1181–1192. doi:10.2134/jeq2005.0213
Crusius, J., and Anderson, R. F. (1991). Core Compression and Surficial Sediment Loss of Lake Sediments of High Porosity Caused by Gravity Coring. Limnol. Oceanogr. 36 (5), 1021–1030. doi:10.4319/lo.1991.36.5.1021
Cui, Y., Xiao, R., Xie, Y., and Zhang, M. (2018). Phosphorus Fraction and Phosphate Sorption-Release Characteristics of the Wetland Sediments in the Yellow River Delta. Phys. Chem. Earth, Parts A/B/C 103, 19–27. doi:10.1016/j.pce.2017.06.005
Ding, S., Chen, M., Gong, M., Fan, X., Qin, B., Xu, H., et al. (2018). Internal Phosphorus Loading from Sediments Causes Seasonal Nitrogen Limitation for Harmful Algal Blooms. Sci. Total Environ. 625, 872–884. doi:10.1016/j.scitotenv.2017.12.348
Ding, S., Han, C., Wang, Y., Yao, L., Wang, Y., Xu, D., et al. (2015). In Situ, High-Resolution Imaging of Labile Phosphorus in Sediments of a Large Eutrophic lake. Water Res. 74, 100–109. doi:10.1016/j.watres.2015.02.008
Dong, L., Yang, Z., Liu, X., and Liu, G. (2012). Investigation into Organic Phosphorus Species in Sediments of Baiyangdian Lake in China Measured by Fractionation and 31P NMR. Environ. Monit. Assess. 184 (9), 5829–5839. doi:10.1007/s10661-012-2550-z
Gao, Y., Liang, T., Tian, S., Wang, L., Holm, P. E., and Bruun Hansen, H. C. (2016). High-resolution Imaging of Labile Phosphorus and its Relationship with Iron Redox State in lake Sediments. Environ. Pollut. 219, 466–474. doi:10.1016/j.envpol.2016.05.053
Golterman, H., Paing, J., Serrano, L., and Gomez, E. (1998). Presence of and Phosphate Release from Polyphosphates or Phytate Phosphate in lake Sediments. Hydrobiologia 364, 99–104. doi:10.1023/A:1003212908511
Golterman, H. L. (1996). Fractionation of Sediment Phosphate with Chelating Compounds: a Simplification, and Comparison with Other Methods. Hydrobiologia 335, 87–95. doi:10.1007/bf00013687
He, Z., Li, F., Dominech, S., Wen, X., and Yang, S. (2019). Heavy Metals of Surface Sediments in the Changjiang (Yangtze River) Estuary: Distribution, Speciation and Environmental Risks. J. Geochemical Exploration 198, 18–28. doi:10.1016/j.gexplo.2018.12.015
Hupfer, M., Gfichter, R., and Giovanoli, R. (1995). Transformation of Phosphorus Species in Settling Seston and during Early Sediment Diagenesis. Aquat. Sci. 57 (4), 305–324. doi:10.1007/bf00878395
Hupfer, M., and Lewandowski, J. (2005). Retention and Early Diagenetic Transformation of Phosphorus in Lake Arendsee (Germany) - Consequences for Management Strategies. archiv_hydrobiologie 164 (2), 143–167. doi:10.1127/0003-9136/2005/0164-0143
Joshi, S. R., Kukkadapu, R. K., Burdige, D. J., Bowden, M. E., Sparks, D. L., and Jaisi, D. P. (2015). Organic Matter Remineralization Predominates Phosphorus Cycling in the Mid-Bay Sediments in the Chesapeake Bay. Environ. Sci. Technol. 49 (10), 5887–5896. doi:10.1021/es5059617
Kleeberg, A., and Dudel, G. E. (1997). Changes in Extent of Phosphorus Release in a Shallow lake (Lake Groβer Müggelsee; Germany, Berlin) Due to Climatic Factors and Load. Mar. Geology. 139, 61–75. doi:10.1016/s0025-3227(96)00099-0
Kwak, D. H., Jeon, Y. T., and Duck Hur, Y. (2018). Phosphorus Fractionation and Release Characteristics of Sediment in the Saemangeum Reservoir for Seasonal Change. Int. J. Sediment Res. 33 (3), 250–261. doi:10.1016/j.ijsrc.2018.04.008
Li, Y., and Gregory, S. (1974). Diffusion of Ions in Sea Water and in Deep-Sea Sediments. Geochimica et Comochiica Acta 38, 703–714.
Liang, X., Song, J., Duan, L., Yuan, H., Li, X., Li, N., et al. (2018). Source Identification and Risk Assessment Based on Fractionation of Heavy Metals in Surface Sediments of Jiaozhou Bay, China. Mar. Pollut. Bull. 128, 548–556. doi:10.1016/j.marpolbul.2018.02.008
Lin, S., Shen, S., Zhou, A., and Lyu, H. M. (2021). Assessment and Management of lake Eutrophication: A Case Study in Lake Erhai, China. Sci. Total Environ. 751, 141618. doi:10.1016/j.scitotenv.2020.141618
Liu, C., Yu, J., and Xiong, F. (2020). Spatial-temporal Variation of Water Quality and Eutrophication Status of Caohai Lake in Guizhou Province. Freshw. Fish. 50 (5), 106–112. doi:10.13721/j.cnki.dsyy.2020.05.015
Lü, C., He, J., Zuo, L., Vogt, R. D., Zhu, L., Zhou, B., et al. (2016). Processes and Their Explanatory Factors Governing Distribution of Organic Phosphorous Pools in lake Sediments. Chemosphere 145, 125–134. doi:10.1016/j.chemosphere.2015.11.038
Mu, Z., Wang, Y., Wu, J., Cheng, Y., Lu, J., Chen, C., et al. (2020). The Influence of cascade Reservoir Construction on Sediment Biogenic Substance Cycle in Lancang River from the Perspective of Phosphorus Fractions. Ecol. Eng. 158, 106051. doi:10.1016/j.ecoleng.2020.106051
Murphy, J., and Riley, J. P. (1962). A Modified Single Solution Method for the Determination of Phosphate in Natural Waters. Analytica Chim. Acta 27, 31–36. doi:10.1016/s0003-2670(00)88444-5
Olila, O. G., Reddy, K. R., and Stites, D. L. (1997). Influence of Draining on Soil Phosphorus Forms and Distribution in a Constructed Wetland. Ecol. Eng. 9 (3-4), 157–169. doi:10.1016/s0925-8574(97)10006-4
Pu, J., Ni, Z., and Wang, S. (2020). Characteristics of Bioavailable Phosphorus in Sediment and Potential Environmental Risks in Poyang Lake: The Largest Freshwater lake in China. Ecol. Indicators 115, 106409. doi:10.1016/j.ecolind.2020.106409
Recknagel, F., Hosomi, M., Fukushima, T., and Kong, D. (1995). Short- and Long-Term Control of External and Internal Phosphorus Loads in Lakes-A Scenario Analysis. Water Res. 29 (7), 1767–1779. doi:10.1016/0043-1354(94)00318-2
Reitzel, K., Ahlgren, J., DeBrabandere, H., Waldebäck, M., Gogoll, A., Tranvik, L., et al. (2006). Degradation Rates of Organic Phosphorus in lake Sediment. Biogeochemistry 82 (1), 15–28. doi:10.1007/s10533-006-9049-z
Rydin, E. (2000). Potentially mobile Phosphorus in Lake Erken Sediment. Water Res. 34 (7), 2037–2042. doi:10.1016/s0043-1354(99)00375-9
Ryuichiro, S., Mikiya, H., Ayato, K., Akio, I., Tetsunori, I., Eiichi, F., et al. (2017). Role of Organic Phosphorus in Sediment in a Shallow Eutrophic lake. Water Resour. Res. 53 (8). doi:10.1002/2017WR020486
Slomp, C. P., Mort, H. P., Jilbert, T., Reed, D. C., Gustafsson, B. G., and Wolthers, M. (2013). Coupled Dynamics of Iron and Phosphorus in Sediments of an Oligotrophic Coastal basin and the Impact of Anaerobic Oxidation of Methane. PLoS One 8 (4), e62386. doi:10.1371/journal.pone.0062386
Søndergaard, M., Jensen, J. P., and Jeppesen, E. (2003). Role of Sediment and Internal Loading of Phosphorus in Shallow Lakes. Hydrobiologia 506-509, 135–145. doi:10.1023/B:HYDR.0000008611.12704.dd
Spears, B. M., Carvalho, L., Perkins, R., Kirika, A., and Paterson, D. M. (2007). Sediment Phosphorus Cycling in a Large Shallow lake: Spatio-Temporal Variation in Phosphorus Pools and Release. Hydrobiologia 584 (1), 37–48. doi:10.1007/s10750-007-0610-0
Stephen, D., Moss, B., and Phillips, G. (1997). Do rooted Macrophytes Increase Sediment Phosphorus Release? Hydrobiologia 342, 27–34. doi:10.1007/978-94-011-5648-6_3
Sun, R., Yang, J., Xia, P., Wu, S., Lin, T., and Yi, Y. (2020). Contamination Features and Ecological Risks of Heavy Metals in the farmland along Shoreline of Caohai Plateau Wetland, China. Chemosphere 254, 126828. doi:10.1016/j.chemosphere.2020.126828
Tammeorg, O., Nürnberg, G., Horppila, J., Haldna, M., and Niemistö, J. (2020). Redox-related Release of Phosphorus from Sediments in Large and Shallow Lake Peipsi: Evidence from Sediment Studies and Long-Term Monitoring Data. J. Great Lakes Res. 46 (6), 1595–1603. doi:10.1016/j.jglr.2020.08.023
Torres, I. C., Turner, B. L., and Ramesh Reddy, K. (2014). The Chemical Nature of Phosphorus in Subtropical Lake Sediments. Aquat. Geochem. 20 (4), 437–457. doi:10.1007/s10498-014-9228-9
Tu, L., Jarosch, K. A., Schneider, T., and Grosjean, M. (2019). Phosphorus Fractions in Sediments and Their Relevance for Historical lake Eutrophication in the Ponte Tresa basin (Lake Lugano, Switzerland) since 1959. Sci. Total Environ. 685, 806–817. doi:10.1016/j.scitotenv.2019.06.243
Ullman, W. J., and Aller, R. C. (1982). Diffusion Coefficients in Nearshore marine Sediments1. Limnol. Oceanogr. 27 (3), 552–556. doi:10.4319/lo.1982.27.3.0552
Wang, S., Jin, X., Zhao, H., and Wu, F. (2006). Phosphorus Fractions and its Release in the Sediments from the Shallow Lakes in the Middle and Lower Reaches of Yangtze River Area in China. Colloids Surf. A: Physicochemical Eng. Aspects 273 (1-3), 109–116. doi:10.1016/j.colsurfa.2005.08.015
Wang, Y., Zhang, T., Zhao, Y., Ciborowski, J. J. H., Zhao, Y., O'Halloran, I. P., et al. (2021). Characterization of Sedimentary Phosphorus in Lake Erie and On-Site Quantification of Internal Phosphorus Loading. Water Res. 188, 116525. doi:10.1016/j.watres.2020.116525
Welch, E. B., and Cooke, G. D. (2005). Internal Phosphorus Loading in Shallow Lakes: Importance and Control. Lake Reservoir Manage. 21 (2), 209–217. doi:10.1080/07438140509354430
Xia, P., Ma, L., Sun, R., Yang, Y., Tang, X., Yan, D., et al. (2020a). Evaluation of Potential Ecological Risk, Possible Sources and Controlling Factors of Heavy Metals in Surface Sediment of Caohai Wetland, China. Sci. Total Environ. 740, 140231. doi:10.1016/j.scitotenv.2020.140231
Xia, P., Ma, L., Yi, Y., and Lin, T. (2021). Assessment of Heavy Metal Pollution and Exposure Risk for Migratory Birds- A Case Study of Caohai Wetland in Guizhou Plateau (China). Environ. Pollut. 275, 116564. doi:10.1016/j.envpol.2021.116564
Xia, P., Yan, D., Sun, R., Song, X., Lin, T., and Yi, Y. (2020b). Community Composition and Correlations between Bacteria and Algae within Epiphytic Biofilms on Submerged Macrophytes in a Plateau lake, Southwest China. Sci. Total Environ. 727, 138398. doi:10.1016/j.scitotenv.2020.138398
Xiang, S., and Zhou, W. (2011). Phosphorus Forms and Distribution in the Sediments of Poyang Lake, China. Int. J. Sediment Res. 26 (2), 230–238. doi:10.1016/s1001-6279(11)60089-9
Xu, Y., Han, F., Li, D., Zhou, J., and Huang, Y. (2018). Transformation of Internal Sedimentary Phosphorus Fractions by point Injection of CaO2. Chem. Eng. J. 343, 408–415. doi:10.1016/j.cej.2018.03.028
Yang, C., Yang, P., Geng, J., Yin, H., and Chen, K. (2020). Sediment Internal Nutrient Loading in the Most Polluted Area of a Shallow Eutrophic lake (Lake Chaohu, China) and its Contribution to lake Eutrophication. Environ. Pollut. 262, 114292. doi:10.1016/j.envpol.2020.114292
Yang, H., Chen, J., Song, Y., Wang, J., and Lu, D. (2017). The Distribution Characteristics and Sources of Organic Carbon in Sediments of Caohai Lake. J. Mianyang Teachers’College 36 (8), 1–9. doi:10.16276/j.cnki.cn51-1670/g.2017.08.001
Yin, C., Yang, H., Wang, J., Guo, J., Tang, X., and Chen, J. (2020). Combined Use of Stable Nitrogen and Oxygen Isotopes to Constrain the Nitrate Sources in a Karst lake. Agric. Ecosyst. Environ. 303, 107089. doi:10.1016/j.agee.2020.107089
Zhang, R., Wang, L., Wu, F., and Song, B. (2013). Phosphorus Speciation in the Sediment Profile of Lake Erhai, Southwestern China: Fractionation and 31P NMR. J. Environ. Sci. 25 (6), 1124–1130. doi:10.1016/s1001-0742(12)60163-6
Zhang, W., Shan, B., Zhang, H., and Tang, W. (2014). Phosphorus-31 Nuclear Magnetic Resonance Assignments of Biogenic Phosphorus Compounds in Sediment of an Artificial Fuyangxin River, China. Environ. Sci. Pollut. Res. 21 (5), 3803–3812. doi:10.1007/s11356-013-2322-5
Zhu, G., Qin, B., Zhang, L., and Luo, L. (2006). Geochemical Forms of Phosphorus in Sediments of Three Large, Shallow Lakes of China. Pedosphere 16 (6), 726–734. doi:10.1016/s1002-0160(06)60108-2
Zhu, Y., Feng, W., Liu, S., He, Z., Zhao, X., Liu, Y., et al. (2018). Bioavailability and Preservation of Organic Phosphorus in lake Sediments: Insights from Enzymatic Hydrolysis and 31P Nuclear Magnetic Resonance. Chemosphere 211, 50–61. doi:10.1016/j.chemosphere.2018.07.134
Keywords: organic phosphorus, sediment, 31P NMR, bioavailability, eutrophication, Lake Caohai
Citation: Yu W, Yang H, Chen J, Liao P, Chen Q, Yang Y and Liu Y (2022) Organic Phosphorus Mineralization Dominates the Release of Internal Phosphorus in a Macrophyte-Dominated Eutrophication Lake. Front. Environ. Sci. 9:812834. doi: 10.3389/fenvs.2021.812834
Received: 10 November 2021; Accepted: 27 December 2021;
Published: 14 January 2022.
Edited by:
Weiying Feng, Beihang University, ChinaReviewed by:
Kang Song, Institute of Hydrobiology (CAS), ChinaWenqiang Zhang, Research Center for Eco-environmental Sciences (CAS), China
Copyright © 2022 Yu, Yang, Chen, Liao, Chen, Yang and Liu. This is an open-access article distributed under the terms of the Creative Commons Attribution License (CC BY). The use, distribution or reproduction in other forums is permitted, provided the original author(s) and the copyright owner(s) are credited and that the original publication in this journal is cited, in accordance with accepted academic practice. No use, distribution or reproduction is permitted which does not comply with these terms.
*Correspondence: Haiquan Yang, eWFuZ2hhaXF1YW5AdmlwLnNrbGVnLmNu; Yong Liu, bHlvbmc4MjFtbW1AMTYzLmNvbQ==