- 1Wetsus—European Centre of Excellence for Sustainable Water Technology, Leeuwarden, Netherlands
- 2Membrane Science and Technology Cluster, MESA+ Institute, University of Twente, Enschede, Netherlands
- 3Department of Environmental Technology, Wageningen University and Research, Wageningen, Netherlands
Soluble Extracellular Polymeric Substances (sEPS) are a mixture of microbial soluble polymers produced during aerobic or anaerobic treatment of wastewater. Gel layers mainly consisting of sEPS are usually present in the fouling layers of membrane bioreactors (MBRs) and in the dynamic membranes (DMs) of dynamic membrane bioreactors (DMBRs), and their properties have not been thoroughly investigated over the years. In this study, sEPS fractions recovered from aerobic and anaerobic reactors were analyzed and tested to build-up EPS gel layers on a 0.2 µm pore size polycarbonate carrier. Dead-end filtration experiments showed that anaerobic sEPS layers, which have a low polysaccharide:protein (PS:PN) ratio, had a lower hydraulic resistance than the aerobic sEPS gel layers, which show a high PS:PN ratio. Optical Coherence Tomography (OCT) and Confocal Laser Scanning Microscopy (CLSM) analyses highlighted that both layers had similar thicknesses and 3D structural organizations. Fluorescent staining of organics and biovolume analysis revealed that for the anaerobic sEPS (low PS:PN), the abundance of proteins appears to destabilize the polysaccharide network increasing the water permeability through the layer. Additionally, the polysaccharides present in the anaerobic sEPS were mainly of the α-linked type, contributing to a more open crosslinked network within the layer, resulting in the low filtration resistance measured. The filtration characteristics observed in this study for the sEPS layers from anaerobic mixed cultures are of interest for possible future application of those layers as dynamic membranes within anaerobic reactors.
1 Introduction
Anaerobic digestion is a widely applied biotechnological process that converts organics from waste(water) streams into energy-rich biogas (Appels et al., 2008; van Lier et al., 2015; Mancini and Raggi, 2021). Unfortunately, this process’s efficiency is often limited by washout of the slow-growing anaerobic microorganisms and/or by insufficient retention of (biodegradable) wastewater solids and colloidal matter. These issues can be tackled by combining the biological process with a membrane in an anaerobic membrane bioreactor (AnMBR), which allows for higher volumetric loading rates and a better effluent quality (Lin et al., 2013; De Vela, 2021).
Long-term operation of membrane bioreactors such as AnMBRs results in the formation of a gel and/or cake layer on the membrane’s surface. The gel layer mostly consists of biopolymers of microbial origin (colloidal and soluble), while the cake layer consists of particles and suspended solids (Wang et al., 2008; Lin et al., 2013; De Vela, 2021). These layers can act as a secondary membrane and dictate filtration resistance and particle retention (Meng et al., 2007; Hu et al., 2016). In this case, the membrane acts merely as a carrier structure for the gel and/or cake layer.
These layers, also called dynamic membranes (DM) were previously used in standard non-biological separation processes, with outstanding removal of fine particles, for example, in the juice clarification process (Kishihara et al., 1989), and in the ultrafiltration separation of aqueous solutions containing poly(ethylene glycol)s with a wide range of molecular weights (Tsapiuk, 1996). In the non-biological DM the layer formed can be categorized as gel or cake layer depending on the layer composition/formation. Cake layers are formed by the deposition of particles in carriers with big pore sizes (10–500 µm). Gel layers instead are shaped by the formation of a polymer network near the carrier interface due to the retention of soluble and colloidal polymers, using carriers with small pore sizes (0.01–1 µm) (Tanny, 1978; Ersahin et al., 2012; Anantharaman et al., 2020).
Dynamic membrane bioreactors (DMBRs) use DM to replace the commonly used micro- or ultra-filtration membranes. The biological DM uses a low-cost material such as an open mesh, which acts as a carrier for the deposition and growth of a layer made of suspended solids, already present or added in the reactor, and constituting the actual filtration medium (Ersahin et al., 2012; Ersahin et al., 2017; Sabaghian et al., 2018; Millanar-Marfa et al., 2021). DMBRs have shown remarkable retention capacity for suspended solids, macromolecules, and flocs, and the DM can be easily back washed and re-formed in situ (Zhang et al., 2014; Pollice and Vergine, 2020; Siddiqui et al., 2021).
Opposite to the well-defined layer type in non-biological DM, the active layer present in the DMBR is categorized as cake plus gel layer, due to the deposition of particles/aggregates, and soluble microbial polymers on the carrier material.
While the cake layer’s role in the DM has been studied extensively (Liu et al., 2009; Ersahin et al., 2016; Xiong et al., 2016), studies on the main characteristics and filtration properties of gel layers are scarce.
Three studies (Fan and Huang, 2002; Chu and Li, 2006; Lei et al., 2021) observed that gel layer formation within a DMBR was a key event for removing both suspended particles and COD during the process.
There is no consensus in the literature in terms of the gel layer’s contribution to the hydraulic resistance of the DMBR. On the one hand, the gel layer is reported to give high filtration resistance to the DM and is therefore considered a fouling layer (Huang et al., 2019). In contrast, other studies reported DMBR with low filtration resistance in the gel layer’s presence (Chu and Li, 2006). Substantial differences in gel layers composition probably cause the inconsistency in the literature concerning their hydraulic resistances. The relation between composition and hydraulic resistance of the gel layer needs to be studied in more detail. The main component present in the gel layer of the DMBR, is soluble or clustered extracellular polymeric substances (EPS) (Zhang et al., 2010; Yu et al., 2015; Lei et al., 2021). Additionally, soluble EPS (sEPS) are often related to the initial stages of forming the gel layer in MBR (Lin et al., 2013; Hong et al., 2014).
sEPS, reported in literature also as soluble microbial products (SMP), are a mixture of moderate molecular weight and biodegradable soluble components. sEPS are released during cell lysis or excreted by microorganisms during biological treatment of wastewater (Laspidou and Rittmann, 2002; Kunacheva and Stuckey, 2014), and are mainly composed of polysaccharides and proteins (Flemming and Wingender, 2010; Seviour et al., 2018).The polysaccharide to protein ratio (PS:PN) of microbial EPSs affects properties such as hydrophilicity, ion adsorption, swelling, complexation, and aggregation (Jørgensen et al., 2017; Shi et al., 2017). For example, the presence of higher amounts of polysaccharides, the hydrophilic fraction of EPS, was correlated with faster clogging of membrane pores and higher irreversible fouling in MBRs (Hong et al., 2018).
In aerobic treatment reactors, sEPS mainly consist of polysaccharides (40–60 w/w %) (Liu and Fang, 2002; Rusanowska et al., 2019), which are considered to be the main fouling agents in aerobic MBRs (Chu and Li, 2005; Wang and Waite, 2008; Shi and Liu, 2021). In anaerobic systems, instead, the polysaccharide fraction of EPS (10–30 w/w %) generally is much lower than the protein fraction (50–80 w/w %) (Berkessa et al., 2018; Hu Y. et al., 2018). A study comparing the chemical composition of different fractions of sEPS collected from aerobic and anaerobic sources showed that sEPS collected from aerobic sources have PS:PN ratios near 1, while anaerobic sources have lower PS:PN ratios 0.5–0.1 (Liu and Fang, 2002). Other studies reported similar sEPS PS:PN ratios in aerobic and anaerobic treatment reactors (Hu D. et al., 2018; Rusanowska et al., 2019).
Yao et al. (2010), who carried out filtration experiments with mixtures of the model polysaccharide sodium alginate and the model protein bovine serum albumin, showed that a higher protein fraction gave lower fouling rates (Yao et al., 2010). This indicates that sEPS rich in protein, such as in anaerobic reactors, may have a beneficial effect on gel layers’ filtration process.
In this study, a more concrete evaluation of the impact of the PS:PN ratio on the gel layer formation and its filtration properties was explored based on real sEPS harvested from lab-scale aerobic and anaerobic reactors.
A lab-scale anaerobic reactor was used to produce and harvest anaerobic sEPS with a low PS:PN ratio. The latter was compared to sEPS with a high PS:PN ratio, sampled from an aerobic system. The two different sEPS sources were applied in dead-end filtration cells to form a sEPS gel layer on a porous polycarbonate carrier (0.2 µm pore size). Gel layers formation and performance were evaluated based on flux decline during the layer build-up and hydraulic resistance after the layer formation. The sEPS layer thickness, structure, and macromolecular arrangement were further investigated via fluorescent staining techniques coupled with confocal laser scanning microscopy (CLSM) and optical coherence tomography (OCT).
2 Materials and Methods
2.1 Anaerobic and Aerobic Bioreactors Operation for sEPS Production
2.1.1 Anaerobic Bioreactor
An anaerobic continuous stirred tank reactor (CSTR) of 11 L was inoculated with 1 L of sludge (55 g volatile suspended solids L−1) from an anaerobic digester of the municipal wastewater treatment plant (WWTP) of the city of Leeuwarden, the Netherlands. The sludge was acclimated for 4 months to synthetic wastewater consisting of a mixture of macro- and micro-nutrients and a combination of glucose, acetate, and tryptone as substrate in a 3:2:1 chemical oxygen demand (COD) ratio (Sudmalis et al., 2018). This soluble substrate mixture was chosen because it was reported that such a substrate ratio would stimulate EPS production (Gagliano et al., 2018). The organic loading rate (OLR) was 1 g COD L−1 d−1, with a hydraulic retention time (HRT) of 11 d. After the acclimation period, the CSTR was operated for 100 days at 37 ± 1°C, at an average pH of 7.4 ± 0.2, and monitored for its sEPS production. Supplementary Table S1 of the supplementary information provides more details about the operation and performance of the anaerobic CSTR.
2.1.2 Aerobic Bioreactor
The aerobic membrane bioreactor (MBR) (3.3 L) was inoculated with activated sludge from the same WWTP. The aerobic reactor was kept at a temperature of 20 ± 1°C and operated at a solid retention time (SRT) of 15 days and an HRT of 5 h. The system was fed with nutrients and the readily biodegradable substrates glycerol and ethanol. The aerobic MBR was specifically designed and operated to produce EPS with a high polysaccharide content. More details are reported by Ajao et al. (Ajao et al., 2018; Ajao et al., 2019).
2.2 sEPS Recovery and Analysis
The mixed liquor of the aerobic MBR and effluent of the anaerobic reactor were used to collect the sEPS fractions by centrifugation for 20 min at 10,000 g, following a procedure described in detail Ajao et al. (Ajao et al., 2018). The supernatants were dialyzed using a tubular dialysis membrane with 12–14 kDa molecular weight cut-off (Spectra/Por 2, United States) against demi water for 5 days with demi water changes every 24 h. The supernatant produced by the centrifugation step was mainly composed of sEPS (Nielsen and Jahn, 1999). sEPS fractions were subsequently recovered by lyophilization (Alpha 2-4 LSCplus, Germany) in triplicate (for the anaerobic CSTR) or duplicate (for the aerobic MBR). Lyophilized samples were used to prepare solutions in Milli-Q water (demineralized water purified by the Milli-Q advantage A10 water purification system) at a concentration of 1 g sEPS L−1 to characterize and compare anaerobic and aerobic sEPS.
The total polysaccharide concentration of these solutions was determined in duplicates by the phenol-sulfuric acid method, with glucose as standard (Dubois et al., 1956). Protein content was determined in triplicate with the Pierce Bicinchoninic Acid Protein Assay Kit (Thermo Scientific, United States) using bovine serum albumin as standard. The COD was determined with Hach Lange LCK514 kits (HACH GMBH, Germany).
2.3 Gel Layer Formation Experiments With sEPS
The sEPS solutions were subsequently diluted with Milli-Q water to achieve a concentration of 100 mg COD L−1. CaCl2 was added to obtain a concentration of 30 mg L−1 Ca2+ to induce gel formation during the filtration process (van den Brink et al., 2009; Wang and Waite, 2009). The sEPS solutions were applied in dead-end filtration cells, as depicted in Figure 1, to test the gel layers’ formation and properties. The cells were stirred at 100 rpm with a magnetic stirrer and operated at a pressure of 0.2 bar. A circular 45.3 cm2 track-etched polycarbonate (PC) membrane with a nominal pore size of 0.2 µm (Sterlitech, United States) was used as the carrier material. The relatively small pore diameter of 0.2 µm was chosen to increase sEPS retention and allow for faster sEPS gel layer formation. Moreover, the use of PC membranes is well described in the literature for fouling studies due to the defined pore size distribution and architecture, allowing the research to be focus mainly on the gel layer properties.
In each experiment, approximately 0.6 L of the sEPS feed solution was filtered. Over time, the cumulative permeate volume was recorded with a digital mass balance (KERN PLJ, Germany) connected to a BalanceConnection software package (KERN SCD 4.0, Germany). The filtration experiments were performed in triplicate (Supplementary Figure S1). Flux profiles during the layer formation and hydraulic resistances after gel layer formation were calculated to monitor the sEPS gel layers’ formation. The total hydraulic filtration resistance was calculated from Darcy’s law (Grenier et al., 2008). The hydraulic resistance is measured before and after gel layer formation to calculate the clean carrier’s hydraulic filtration resistance and the total hydraulic filtration resistance after gel layer formation, respectively. The filtration resistance of the sEPS gel layer was obtained by subtracting the clean carrier filtration resistance from the total filtration resistance. After the filtration runs, the sEPS layers on their carrier were carefully removed from the filtration chamber under fully hydrated conditions for subsequent microscopy and OCT analysis.
2.4 Characterization of the sEPS Gel Layers
2.4.1 Crystal Violet Staining and Bright-Field Stereomicroscopy
The sEPS gel layers were visualized with crystal violet 0.1% (v/v) staining (O'Toole, 2011). The stained layer was observed using a Leica MZ95 Stereomicroscope equipped with a DFC320 camera (Leica Microsystems, Germany).
2.4.2 Optical Coherence Tomography
The sEPS gel layers’ thicknesses were accessed by a spectral-domain OCT (Thorlabs Ganymede OCT System, United States). The OCT was equipped with a 5x telecentric scan lens (Thorlabs LSM03BB, United States) with a maximum scan area of 100 mm2. The OCT provided high-resolution images with a sensitivity of 106 dB at 1.25 kHz A-scan rate.
2.4.3 Fluorescence Microscopy Analyses
The distribution of polysaccharides and proteins in the EPS gel layer was analyzed with specific fluorescent dyes and CLSM. For this purpose, immediately after the formation of the sEPS gel layer in the dead-end filtration system, a solution of 0.1 M sodium bicarbonate buffer, pH 8.2, was filtered to keep the amine groups of proteins in deprotonated form. Afterward, three staining solutions of 0.5 g L−1 of fluorescein isothiocyanate (FITC) (Thermo Fisher Scientific, United States), 0.3 g L−1 of calcofluor white (CW) (Fluka, Canada), and 0.25 g L−1 of Concanavalin A (Con A)—rhodamine, (Thermo Fisher Scientific, United States) were sequentially filtered through the gel layer to stain proteins, β-linked D-glucopyranose sugars, and α-polysaccharides rich in mannose and glucose, respectively. The staining solutions were allowed to stay at least 30 min in static contact with the gel layer. After each staining step, the sample was washed twice with a phosphate buffer solution (0.25 X).
After staining, the gel layers were maintained under fully hydrated conditions for further processing. Samples were examined by CLSM using the LSM 880 model (Zeiss, Germany). The sample scanning was carried out from the gel layers’ top surface to the PC membrane’s top surface, with a plan apochromat 40X/1.3 Oil DIC M27 objective. Visualization and analysis of samples were performed with Zen Black software (Zeiss, Germany). The FITC, CW, and rhodamine -Con A fluorophores were detected by excitation/emission at 488/500–540 nm, 405/410–480 nm, and 540/605–630 nm, respectively. The FIJI software package (version 1.51 g, Wayne Rasband, NIH, Bethesda, MD, United States) was used to merge the different color channels into single images and to construct three-dimensional (3D) objects from the CLSM stacks.
The gel layer’s biovolume was defined as the volume occupied by the polysaccharides and proteins in the sEPS layer. The biovolume was calculated by converting each CLSM stack pixel into individual voxels. A voxel was defined as the product of the squared pixel size and the scanning step size (1 µm) of the CLSM stack. The total number of voxels in the CLSM stacks defined the total volume of the gel layer. After pre-processing of each channel of the CLSM stacks, image segmentation was applied to calculate the biovolume of proteins, α-polysaccharides, and β-polysaccharides through the Voxel Counter plugin of the FIJI software.
3 Results and Discussion
3.1 Aerobic and Anaerobic sEPS Recovery and Analysis
Supplementary Table S1 gives the most important operational and performance parameters of the anaerobic CSTR used in this study to produce and harvest sEPS from anaerobic mixed cultures. The sEPS obtained from the reactor’s effluent increased in concentration during the operational period of 100 days and was between 100 and 170 mg L−1. The PS:PN ratio variation calculated for the sEPS collected in this period was small (Table 1). As expected, and in agreement with earlier studies on anaerobic sEPS samples (Liu and Fang, 2002; Berkessa et al., 2018; Hu Y. et al., 2018), an average PS:PN ratio of 0.1 ± 0.02 was found. The sEPS collected from the aerobic MBR had a much higher PS:PN ratio of 1.4 ± 0.4 (Table 1), which was expected as this reactor was specifically operated to produce high amounts of polysaccharides-rich EPS (Ajao et al., 2018). Note that the sum of the polysaccharides and proteins does not account for 100% of the sEPS because also other compounds are usually detected in sEPS mixtures, such as some complex organic colloids, nucleic acids, lipids, and humic-like substances (Janga et al., 2007; Ajao et al., 2018).

TABLE 1. Anaerobic and aerobic sEPS concentrations, their polysaccharide (PS) and protein (PN) content, and their ratio (PS:PN ratio).
3.2 Gel Layer Formation With Aerobic and Anaerobic sEPS
Aerobic sEPS (high PS:PN ratio) and anaerobic sEPS (low PS:PN ratio) were used in dead-end filtration experiments to form a gel layer on the 0.2 μm PC carrier. Due to the different EPS yields of the two lab-scale reactors used in this study, a single sample collected from the aerobic MBR was enough to carry out all the experiments, while multiple samples had to be taken from the anaerobic CSTR (as listed in Table 1). However, as indicated in Table 1, the PS:PN ratio of the anaerobic samples was similar. Lyophilized samples were used to prepare solutions in Milli-Q water at a concentration of 1 g sEPS L−1, which corresponding COD concentrations were 740 mg COD L−1 for anaerobic and of 1,137 mg COD L−1 for aerobic sEPS. These solutions were diluted to 100 mg COD L−1 in Milli-Q water to obtain approximately 152 ± 14 mg L−1 of anaerobic EPS and 93 mg L−1 of aerobic sEPS. The sEPS solutions were filtered through the 0.2 μm PC carrier to form the gel layers, which were then collected and evaluated via bright field stereomicroscopy and OCT (Figure 2). Both sEPS layers were positive to 0.1% crystal violet staining, which indicates the deposition of microbial biopolymers on the PC carrier’s surface (Figure 2A), forming a gel layer covering the carrier. OCT analysis showed an average thickness of approximately 100 ± 42 μm, but with an uneven non-uniform distribution of the sEPS on the carrier’s surface (Figure 2B). A considerable variation in thickness was observed for the high PS:PN sEPS layer, ranging between 26 and 202 μm. The filtration experiments with the aerobic and anaerobic sEPS solutions were performed in triplicate, as presented in Fig.S1. Figure 3 shows flux profiles in time and final filtration resistances of the layers that were formed, one representative experiment per each sample used. These profiles can be divided into two stages: formation stage I, with a fast-flux decline, and an operational stage II, with a slowly declining or stagnant flux (Figure 3A). For the aerobic produced, high PS:PN ratio sEPS, the flux decline was very fast, taking only 0.4 h for the flux to decrease from 300 to below 5 L m−2 h−1, indicating rapid deposition of sEPS on the carrier. Working with the anaerobic sEPS with a low PS:PN ratio, a much longer formation phase was observed, as the flux decline from 300 to below 5 L m−2 h−1 took more than 8 h.
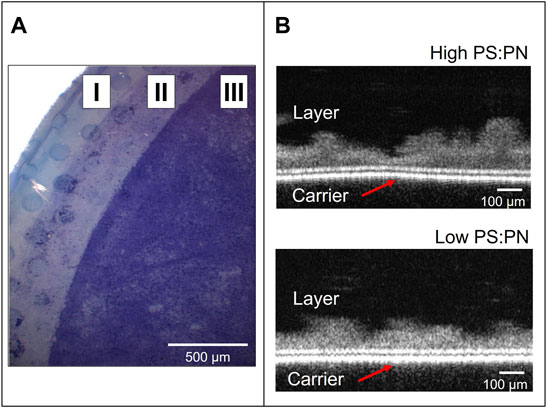
FIGURE 2. sEPS gel layers after the dead-end filtration experiments. (A) A bright field stereomicroscopy image of low PS:PN sEPS layer stained with crystal violet on the top of the polycarbonate carrier. Zone I and II are the carrier zones covered with a rubber o-ring; zone III is where the sEPS layer formed. (B) Static OCT images of the layers formed with sEPS with high (upper image, from the aerobic reactor) and low (lower image, from the anaerobic reactor) PS:PN ratios.
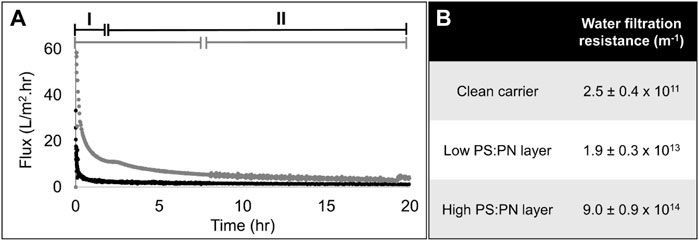
FIGURE 3. Filtration properties of sEPS gel layer. (A) Flux profiles as a function of filtration time at constant pressure (0.2 bar) of the sEPS with low PS:PN ratio (anaerobic, grey line) and high PS:PN ratio (aerobic, black line). A formation (I) and operational phase (II) are indicated. The data profile was obtained from the triplicate filtration experiment (Replicates are shown in Supplementary Figure S1). (B) Final filtration resistances of the layers.
Similar results observed in stage I (Figure 3A) were reported by Yao et al. when using a mixture of model compounds (sodium alginate and bovine serum albumin) filtered on 0.2 μm pore size PC microfiltration membranes, observing that the initial fast-flux decline was shortened at higher PS:PN ratios (Yao et al., 2010). Other studies that investigated membrane fouling in MBRs also discovered that an increase of filtration resistance correlates positively with the concentration of polysaccharides and the resulting increase of the viscosity in the feed solution (Lesjean et al., 2005; Drews et al., 2006). Yun et al. reported the formation of a uniform network of polysaccharides of low porosity as the main reason for the increase in the filtration resistance observed in aerobic MBRs (Yun et al., 2006).
The much slower flux decline during stage II in both cases was most likely dictated by a simultaneous accumulation and detachment of sEPS, probably due to the shear force imposed by the magnetic stirrer. Although both layers have a similar average thickness (Figure 2B), the gel layers’ mechanical stability to the shear force and their corresponding hydraulic resistances can be quite different. A common explanation reported in the literature is that the gel-like layers with higher PS:PN ratios have stronger polymeric networks than gels with low PS:PN ratios due to the high physical crosslinking density of the PS chains (Le and Turgeon, 2013; Zhang et al., 2018). The strength and the mechanical stability of the gel layer to the shear force most probably result in constant filtration properties. However, to the best of our knowledge, no studies have addressed the correlation between PS:PN ratio and mechanical stability of sEPS layers against shear force.
Although the average thickness of the two sEPS layers was similar, 100 ± 42 μm (Figure 2B), the hydraulic filtration resistance of the layer formed with the low PS:PN ratio anaerobic sEPS was 50 times lower than the resistance of the high PS:PN ratio aerobic sEPS layer (Figure 3B). Yao et al. (2010) observed the same trend when performing short-term dead-end filtration of solutions made by the model compounds alginate and bovine serum albumin, using PC and polyvinylidene fluoride membranes with a pore size of 0.2 µm (Yao et al., 2010).
The higher filtration resistance of the layer built with high PS:PN ratio sEPS can be explained firstly by the higher concentration of polysaccharides, as these not only form networks with a high physical crosslinking density between the polymer chains (Tual et al., 2000) but also retain large amounts of immobilized water in their network (Hong et al., 2014). The low PS:PN ratio sEPS layer contains fewer polysaccharides, having a lower degree of polymer chain crosslinking, and thus a lower filtration resistance. Another possible factor influencing the filtration resistance is the presence of a large fraction of proteins, such as in the low PS:PN ratio sEPS layer, which can disrupt the organization of the polysaccharide network, and thereby further opening the layer structure (Laneuville et al., 2006; Wang et al., 2009; Gentès et al., 2011). The anaerobic sEPS with a low PS:PN ratio appears to be more suitable for creating a gel layer with lower filtration resistance than aerobic sEPS, with a high PS:PN ratio. A third detail likely dictating the filtration resistance difference is related to the distinct polysaccharides’ composition between aerobic and anaerobic sEPS, and will be discussed in the next section.
3.3 Spatial Distribution of Polysaccharides and Proteins
The effect of polysaccharides and proteins on the filtration resistance and their distribution within the sEPS layers were further elucidated by fluorescent staining. As expected from the different PS:PN ratios, the density of each compound in the two layers was different, as shown by the CLSM and biovolume analyses (Figure 4; Supplementary Figure S2). β-polysaccharides (in blue) were the higher organic fraction in the layer formed with the aerobic, high PS:PN ratio sEPS (Figure 4A), while proteins (in green) were dominant in the layer formed with the anaerobic, low PS:PN ratio sEPS (Figure 4C). It should be noted that the thickness determined with CLSM (between 60 and 80 µm) was lower than the thickness determined with OCT (100 ± 42 μm). A possible explanation of such difference is the non-uniform deposition of the layer on the carrier (Figure 2B) and the utilization of a glass coverslip to analyze the samples via CLSM. The Z-axis resolution of OCT is in the order of 10–15 μm, while CLSM allows for a resolution of approximately 0.8 µm.
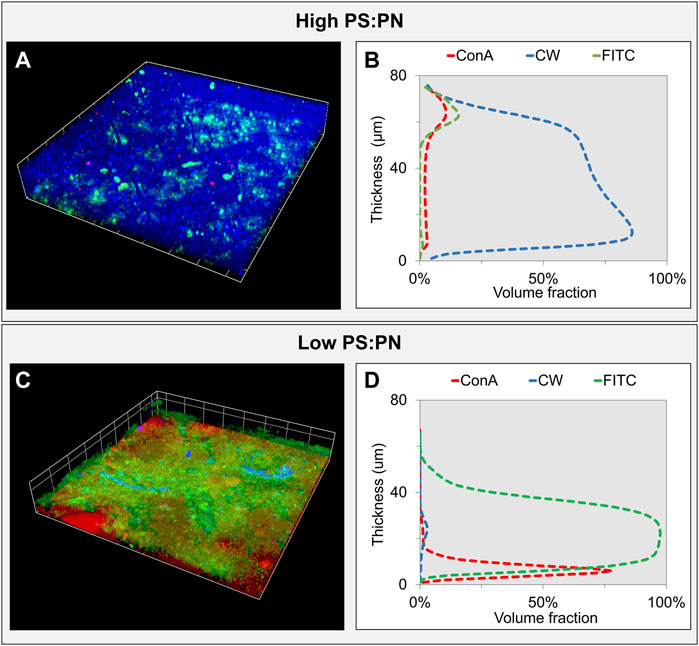
FIGURE 4. Confocal laser scanning microscopy (CLSM) analysis of the sEPS layers—3D volume reconstruction of CLSM stacks of the sEPS layers formed with high (aerobic) (A) and low (anaerobic) (C) PS:PN sEPS after fluorescent staining of biopolymers. In blue, the β-polysaccharides were stained with calcofluor white, in red the α-polysaccharides stained with Concanavalin A, and in green, the proteins stained with FITC. The biovolume analysis of each stained biopolymer within the gel layer formed with high and low PS:PN sEPS is presented in (B) and (D), respectively.
The PS:PN ratios calculated by biovolume analysis (Figures 4B,D) were similar to those determined by chemical analysis of the sEPS solution (Table 1), with a ratio of 1.9 for the high PS:PN sEPS layer and a ratio of 0.17 for the low PS:PN ratio sEPS layer. These results confirm that there was no preferential accumulation or adsorption of polysaccharides neither proteins on the carrier.
CLSM images highlighted that in the low PS:PN ratio sEPS layer, both β-polysaccharides (Figure 4C, in blue) and α-polysaccharides (Figure 4C, in red) were present, while in the high PS:PN sEPS layer, only β-polysaccharides were detected (Figure 4A, in blue). This variation in polymer composition between EPS from different microbial origins was reported by other researchers (Park and Helm, 2008; Simon et al., 2009). This is important because different polysaccharides have different degrees of crosslinking (Coviello et al., 2007; Meng et al., 2018), which influence filtration resistances in EPS layers. The layer formed with aerobic, high PS:PN ratio sEPS mainly contained β-polysaccharides, that have a higher degree of entanglement due to the more flexible chains (Lee and Mooney, 2012), compared to α-polysaccharides, with lower flexibility, predominately present in the anaerobic, low PS:PN ratio sEPS.
The polysaccharides and proteins’ location through the layer thickness was not affected by the type of polysaccharides (α or β) or by the PS:PN ratio. Both layers showed a polysaccharides rich zone, one as α-polysaccharide, the other as β-polysaccharides close to the carrier surface (Supplementary Figure S2), while the proteins accumulated on top of the polysaccharides (Figures 4A,B; Supplementary Figure S2). Hong and colleagues (Hong et al., 2018) showed that the polysaccharides fraction within an MBR fouling layer was located close to the membrane surface. The clogging of the membrane pores by the long hydrophilic polysaccharides’ chains started the formation of the layer, which later on was able to retain the small-sized, more hydrophobic macromolecules such as proteins (Hong et al., 2018).
Biovolume analyses showed that the high PS:PN ratio sEPS layer was mainly composed of β-polysaccharides (Figure 4B; Supplementary Figure S2, CW–blue signal), while the proteins were located at ≈ 60 µm from the surface of the carrier (Figure 4B, FITC–green signal). The α-polysaccharide portion in the biovolume of the low PS:PN ratio sEPS layer was ≈40 µm thinner than the β-polysaccharides portion in the other layer, while the proteins were uniformly distributed over the whole width of the layer (Figure 4D; Supplementary Figure S2). Similar observations were made for the spatial organization of polysaccharides and proteins on the surface of membranes materials such as mixed cellulose ester or cellulose triacetate (Chen et al., 2006; Yuan et al., 2015; Wang et al., 2016), suggesting that the nature of the carrier material can influence the polymers deposition during the filtration experiments. Further investigations with different carrier materials are needed to clarify this point.
3.4 sEPS Gel Layers as a Dynamic Membrane for Anaerobic Treatments: Opportunities and Challenges
Our results have shown that sEPS produced in biological treatment reactors can be used to form a gel layer on a 0.2 μm PC membrane used as carrier material. Additionally, it was also proven that such a gel layer would only have an appreciably low filtration resistance if the PS:PN ratio of the sEPS is low, as observed for anaerobic sEPS. Usually, AnMBRs using microfiltration membranes showed filtration resistances 100 times higher than in anaerobic DMBRs (Lin et al., 2011). Previous studies of anaerobic DMBRs treating wastewater, where the DM consisted of a cake layer plus an underlying gel layer (Ersahin et al., 2016), showed filtration resistance values in the same order of magnitude as we obtained in the present work with only the anaerobic sEPS gel layer present. On the other hand, DM layers are usually prepared by deposition of particles and colloids, and can release smaller particles. Thus, when the deposited organic material accumulates over the filtration capacity, the membrane pores would be blocked, causing membrane fouling issues (Huang et al., 2020) This suggests that using a DM system based solely on a sEPS gel layer could be an attractive option for anaerobic bioreactors to improve fine particle (and biomass) retention, without further increase in the filtration resistance due to particle accumulation, and cake layer maturation.
In this work, a purified sEPS solution was used for the formation of the gel layer. In reality, it will be impossible to obtain such a “clean” sEPS solution from the biological reactor to form only active gel layer. The sEPS within the effluent will always go along with a low concentration of suspended and colloidal particles that may interfere with the gel layer formation. Generally, to prevent the deposition of suspended particles (typically >5 µm) and the formation of the cake layer, in DMBRs a certain level of shear could be provided by sparging with the produced biogas (Cui et al., 2003; Ersahin et al., 2012). The use of shear implies the sEPS gel layer should have sufficient mechanical stability to avoid its detachment from the carrier structure. The impact of shear and the PS:PN ratio in the sEPS gel layer’s mechanical stability need to be further evaluated and characterized experimentally.
In light of this, as a technological perspective for future experiments, the filtration step with the a gel-layer DM could be realized in a separate unit outside the main anaerobic reactor, including a settling step in between, with the aim to remove colloids and big particles, as depicted in Supplementary Figure S3. The effluent of the CSTR would be then be seen as a substrate for the recovery of resources, in this case the sEPS biopolymers used as building blocks of a dynamic membrane. These sEPS would otherwise be part of the waste effluent to be further treated/disposed. In an industrial world turning around over circular economy, the use of waste streams is starting to be highly valorized as alternative/secondary sustainable revenue outcome model (Gherghel et al., 2019)
In this study, we do propose a suitable application for protein-rich natural polymers, originating from anaerobic microorganisms. The production of EPS by mixed cultures is a natural, cheap, and environmentally friend process (More et al., 2014). EPS produced by mixed cultures microorganisms have important properties for the final industrial application, such as the ability to bind/adsorb organics through complicated interactions (Yan et al., 2019), and in particular sEPS has shown a higher tendency to establish such interactions (Comte et al., 2006; Pan et al., 2010).
Even though additional research is needed, this study made clear that the PS:PN ratio of sEPS impacts the gel layer structure, which affects the hydraulic resistance of the layer. The protein-rich sEPS from anaerobic mixed cultures may have a future application as a cheap active filtering medium with low hydraulic resistances to prevent the washout of fine particles (0.2–10 µm) such as slowly degradable compounds and microorganisms. Anaerobic mixed cultures are a reproducible source of natural polymers, that can be tuned in PS:PN ratio and polymer composition and can be applied to obtain high performance filtration systems, for different anaerobic wastewater treatment applications. Moreover, the findings of the present work can be of help for a better understanding of the importance and function of gel layers in dictating the performances of DMBRs (Chu and Li, 2006; Huang et al., 2019).
As per the technological application of sEPS filtration layers, the perspective is to integrate it in an industrial line, which already utilizes anaerobic digestion to treat waste streams, as intermediate step between anaerobic digestion and effluent disposal. This topic is currently under investigation together with leading companies in the development of new biotechnologies.
Data Availability Statement
The raw data supporting the conclusion of this article will be made available by the authors, without undue reservation.
Author Contributions
HT, AK, MG and ED contributed to the conception and design of the study. ED run the experiments and organized the database. ED, wrote the first draft of the manuscript. HT and MG wrote sections of the manuscript. All authors contributed to manuscript revision, read, and approved the submitted version.
Funding
This research was performed in the cooperation framework of Wetsus, European Centre of Excellence for Sustainable Water Technology (www.wetsus.nl). Wetsus is cofounded by the Dutch Ministry of Economic Affairs and Ministry of Infrastructure and Environment, the European Union Regional Development Fund, the Province of Fryslan, and the Northern Netherlands Provinces. This work is part of a project that has received funding from the European Union’s Horizon 2020 research and innovation programme under the Marie Sklodowska-Curie Grant Agreement 665874.
Conflict of Interest
The authors declare that the research was conducted in the absence of any commercial or financial relationships that could be construed as a potential conflict of interest.
Publisher’s Note
All claims expressed in this article are solely those of the authors and do not necessarily represent those of their affiliated organizations, or those of the publisher, the editors, and the reviewers. Any product that may be evaluated in this article, or claim that may be made by its manufacturer, is not guaranteed or endorsed by the publisher.
Acknowledgments
The authors thank the participants of the research themes “Advanced water treatment” and “Natural flocculants” for fruitful discussions and financial support. The authors also thank Victor Ajao for providing the waste sludge for aerobic sEPS recovery and Jan Jurjen Salverda for the realization of the lab-scale anaerobic reactors setup.
Supplementary Material
The Supplementary Material for this article can be found online at: https://www.frontiersin.org/articles/10.3389/fenvs.2022.774536/full#supplementary-material
References
Ajao, V., Bruning, H., Rijnaarts, H., and Temmink, H. (2018). Natural Flocculants from Fresh and saline Wastewater: Comparative Properties and Flocculation Performances. Chem. Eng. J. 349, 622–632. doi:10.1016/j.cej.2018.05.123
Ajao, V., Millah, S., Gagliano, M. C., Bruning, H., Rijnaarts, H., and Temmink, H. (2019). Valorization of Glycerol/ethanol-Rich Wastewater to Bioflocculants: Recovery, Properties, and Performance. J. Hazard. Mater. 375, 273–280. doi:10.1016/j.jhazmat.2019.05.009
Anantharaman, A., Chun, Y., Hua, T., Chew, J. W., and Wang, R. (2020). Pre-deposited Dynamic Membrane Filtration – A Review. Water Res. 173, 115558. doi:10.1016/j.watres.2020.115558
Appels, L., Baeyens, J., Degrève, J., and Dewil, R. (2008). Principles and Potential of the Anaerobic Digestion of Waste-Activated Sludge. Prog. Energ. Combust. Sci. 36 (6), 755–781. doi:10.1016/j.pecs.2008.06.002
Berkessa, Y. W., Yan, B., Li, T., Tan, M., She, Z., Jegatheesan, V., et al. (2018). Novel Anaerobic Membrane Bioreactor (AnMBR) Design for Wastewater Treatment at Long HRT and High Solid Concentration. Bioresour. Technol. 250, 281–289. doi:10.1016/j.biortech.2017.11.025
Chen, M. Y., Lee, D. J., and Tay, J. H. (2006). Extracellular Polymeric Substances in Fouling Layer. Sep. Sci. Technol. 41, 1467–1474. doi:10.1080/01496390600683597
Chu, H. P., and Li, X. Y. (2005). Membrane Fouling in a Membrane Bioreactor (MBR): Sludge Cake Formation and Fouling Characteristics. Biotechnol. Bioeng. 90, 323–331. doi:10.1002/bit.20409
Chu, L., and Li, S. (2006). Filtration Capability and Operational Characteristics of Dynamic Membrane Bioreactor for Municipal Wastewater Treatment. Sep. Purif. Technol. 51, 173–179. doi:10.1016/j.seppur.2006.01.009
Comte, S., Guibaud, G., and Baudu, M. (2006). Biosorption Properties of Extracellular Polymeric Substances (EPS) Resulting from Activated Sludge According to Their Type: Soluble or Bound. Process. Biochem. 41, 815–823. doi:10.1016/j.procbio.2005.10.014
Coviello, T., Matricardi, P., Marianecci, C., and Alhaique, F. (2007). Polysaccharide Hydrogels for Modified Release Formulations. J. Control Release 119, 5–24. doi:10.1016/j.jconrel.2007.01.004
Cui, Z. F., Chang, S., and Fane, A. G. (2003). The Use of Gas Bubbling to Enhance Membrane Processes. J. Memb. Sci. 221, 1–35. doi:10.1016/S0376-7388(03)00246-1
De Vela, R. J. (2021). A Review of the Factors Affecting the Performance of Anaerobic Membrane Bioreactor and Strategies to Control Membrane Fouling. Rev. Environ. Sci. Biotechnol. 20, 607–644. doi:10.1007/s11157-021-09580-2
Drews, A., Lee, C. H., and Kraume, M. (2006). Membrane Fouling - a Review on the Role of EPS. Desalination 200, 186–188. doi:10.1016/j.desal.2006.03.290
Dubois, M., Gilles, K. A., Hamilton, J. K., Rebers, P. A., and Smith, F. (1956). Colorimetric Method for Determination of Sugars and Related Substances. Anal. Chem. 28, 350–356. doi:10.1021/ac60111a017
Ersahin, M. E., Ozgun, H., Dereli, R. K., Ozturk, I., Roest, K., and van Lier, J. B. (2012). A Review on Dynamic Membrane Filtration: Materials, Applications and Future Perspectives. Bioresour. Technol. 122, 196–206. doi:10.1016/j.biortech.2012.03.086
Ersahin, M. E., Tao, Y., Ozgun, H., Gimenez, J. B., Spanjers, H., and van Lier, J. B. (2017). Impact of Anaerobic Dynamic Membrane Bioreactor Configuration on Treatment and Filterability Performance. J. Memb. Sci. 526, 387–394. doi:10.1016/j.memsci.2016.12.057
Ersahin, M. E., Tao, Y., Ozgun, H., Spanjers, H., and van Lier, J. B. (2016). Characteristics and Role of Dynamic Membrane Layer in Anaerobic Membrane Bioreactors. Biotechnol. Bioeng. 113, 761–771. doi:10.1002/bit.25841
Fan, B., and Huang, X. (2002). Characteristics of a Self-Forming Dynamic Membrane Coupled with a Bioreactor for Municipal Wastewater Treatment. Environ. Sci. Technol. 36, 5245–5251. doi:10.1021/es025789n
Flemming, H. C., and Wingender, J. (2010). The Biofilm Matrix. Nat. Rev. Microbiol. 8, 623–633. doi:10.1038/nrmicro2415
Gagliano, M. C., Neu, T. R., Kuhlicke, U., Sudmalis, D., Temmink, H., and Plugge, C. M. (2018). EPS Glycoconjugate Profiles Shift as Adaptive Response in Anaerobic Microbial Granulation at High Salinity. Front. Microbiol. 9, 1423. doi:10.3389/fmicb.2018.01423
Gentès, M. C., St-Gelais, D., and Turgeon, S. L. (2011). Gel Formation and Rheological Properties of Fermented Milk with In Situ Exopolysaccharide Production by Lactic Acid Bacteria. Dairy Sci. Technol. 91, 645. doi:10.1007/s13594-011-0039-0
Gherghel, A., Teodosiu, C., and De Gisi, S. (2019). A Review on Wastewater Sludge Valorisation and its Challenges in the Context of Circular Economy. J. Clean. Prod. 228, 244–263. doi:10.1016/j.jclepro.2019.04.240
Grenier, A., Meireles, M., Aimar, P., and Carvin, P. (2008). Analysing Flux Decline in Dead-End Filtration. Chem. Eng. Res. Des. 86, 1281–1293. doi:10.1016/j.cherd.2008.06.005
Hong, H., Zhang, M., He, Y., Chen, J., and Lin, H. (2014). Fouling Mechanisms of Gel Layer in a Submerged Membrane Bioreactor. Bioresour. Technol. 166, 295–302. doi:10.1016/j.biortech.2014.05.063
Hong, P.-N. N., Taing, C., Phan, P.-T. T., and Honda, R. (2018). Polarity-Molecular Weight Profile of Extracellular Polymeric Substances in a Membrane Bioreactor: Comparison between Bulk Sludge and Cake Layers. J. Water Environ. Technol. 16, 40–53. doi:10.2965/jwet.17-020
Hu, D., Li, X., Chen, Z., Cui, Y., Gu, F., Jia, F., et al. (2018). Performance and Extracellular Polymers Substance Analysis of a Pilot Scale Anaerobic Membrane Bioreactor for Treating Tetrahydrofuran Pharmaceutical Wastewater at Different HRTs. J. Hazard. Mater. 342, 383–391. doi:10.1016/j.jhazmat.2017.08.028
Hu, Y., Wang, X. C., Ngo, H. H., Sun, Q., and Yang, Y. (2018). Anaerobic Dynamic Membrane Bioreactor (AnDMBR) for Wastewater Treatment: A Review. Bioresour. Technol. 247, 1107–1118. doi:10.1016/j.biortech.2017.09.101
Hu, Y., Wang, X. C., Yu, Z. Z., Ngo, H. H., Sun, Q., and Zhang, Q. (2016). New Insight into Fouling Behavior and Foulants Accumulation Property of Cake Sludge in a Full-Scale Membrane Bioreactor. J. Memb. Sci. 510, 10–17. doi:10.1016/j.memsci.2016.02.058
Huang, C., Liu, H., Meng, S., and Liang, D. (2020). Effect of PAC on the Behavior of Dynamic Membrane Bioreactor Filtration Layer Based on the Analysis of Mixed Liquid Properties and Model Fitting. Membranes (Basel) 10, 1–14. doi:10.3390/membranes10120420
Huang, J., Wu, X., Cai, D., Chen, G., Li, D., Yu, Y., et al. (2019). Linking Solids Retention Time to the Composition, Structure, and Hydraulic Resistance of Biofilms Developed on Support Materials in Dynamic Membrane Bioreactors. J. Memb. Sci. 581, 158–167. doi:10.1016/j.memsci.2019.03.033
Janga, N., Ren, X., Kim, G., Ahn, C., Cho, J., and Kim, I. S. (2007). Characteristics of Soluble Microbial Products and Extracellular Polymeric Substances in the Membrane Bioreactor for Water Reuse. Desalination 202, 90–98. doi:10.1016/j.desal.2005.12.043
Jørgensen, M. K., Nierychlo, M., Nielsen, A. H., Larsen, P., Christensen, M. L., and Nielsen, P. H. (2017). Unified Understanding of Physico-Chemical Properties of Activated Sludge and Fouling Propensity. Water Res. 120, 117–132. doi:10.1016/j.watres.2017.04.056
Kishihara, S., Tamaki, H., Fujii, S., and Komoto, M. (1989). Clarification of Technical Sugar Solutions through a Dynamic Membrane Formed on a Porous Ceramic Tube. J. Memb. Sci. 41, 103–114. doi:10.1016/S0376-7388(00)82394-7
Kunacheva, C., and Stuckey, D. C. (2014). Analytical Methods for Soluble Microbial Products (SMP) and Extracellular Polymers (ECP) in Wastewater Treatment Systems: A Review. Water Res. 61, 1–18. doi:10.1016/j.watres.2014.04.044
Laneuville, S. I., Turgeon, S. L., Sanchez, C., and Paquin, P. (2006). Gelation of Native β-lactoglobulin Induced by Electrostatic Attractive Interaction with Xanthan Gum. Langmuir 22, 7351–7357. doi:10.1021/la060149+
Laspidou, C. S., and Rittmann, B. E. (2002). Non-steady State Modeling of Extracellular Polymeric Substances, Soluble Microbial Products, and Active and Inert Biomass. Water Res. 36, 1983–1992. doi:10.1016/S0043-1354(01)00414-6
Le, X. T., and Turgeon, S. L. (2013). Rheological and Structural Study of Electrostatic Cross-Linked Xanthan Gum Hydrogels Induced by β-lactoglobulin. Soft Matter 9, 3063–3073. doi:10.1039/c3sm27528k
Lee, K. Y., and Mooney, D. J. (2012). Alginate: Properties and Biomedical Applications. Prog. Polym. Sci. 37, 106–126. doi:10.1016/j.progpolymsci.2011.06.003
Lei, Z., Wang, J., Leng, L., Yang, S., Dzakpasu, M., Li, Q., et al. (2021). New Insight into the Membrane Fouling of Anaerobic Membrane Bioreactors Treating Sewage: Physicochemical and Biological Characterization of Cake and Gel Layers. J. Memb. Sci. 632, 119383. doi:10.1016/j.memsci.2021.119383
Lesjean, B., Rosenberger, S., Laabs, C., Jekel, M., Gnirss, R., and Amy, G. (2005). Correlation between Membrane Fouling and Soluble/colloidal Organic Substances in Membrane Bioreactors for Municipal Wastewater Treatment. Water Sci. Technol. 51, 1–8. doi:10.2166/wst.2005.0615
Lin, H., Liao, B. Q., Chen, J., Gao, W., Wang, L., Wang, F., et al. (2011). New Insights into Membrane Fouling in a Submerged Anaerobic Membrane Bioreactor Based on Characterization of Cake Sludge and Bulk Sludge. Bioresour. Technol. 102, 2373–2379. doi:10.1016/j.biortech.2010.10.103
Lin, H., Peng, W., Zhang, M., Chen, J., Hong, H., and Zhang, Y. (2013). A Review on Anaerobic Membrane Bioreactors: Applications, Membrane Fouling and Future Perspectives. Desalination 314, 169–188. doi:10.1016/j.desal.2013.01.019
Liu, H., and Fang, H. H. P. (2002). Extraction of Extracellular Polymeric Substances (EPS) of Sludges. J. Biotechnol. 95, 249–256. doi:10.1016/S0168-1656(02)00025-1
Liu, H., Yang, C., Pu, W., and Zhang, J. (2009). Formation Mechanism and Structure of Dynamic Membrane in the Dynamic Membrane Bioreactor. Chem. Eng. J. 148, 290–295. doi:10.1016/j.cej.2008.08.043
Mancini, E., and Raggi, A. (2021). A Review of Circularity and Sustainability in Anaerobic Digestion Processes. J. Environ. Manage. 291, 112695. doi:10.1016/j.jenvman.2021.112695
Meng, F., Zhang, H., Yang, F., and Liu, L. (2007). Characterization of Cake Layer in Submerged Membrane Bioreactor. Environ. Sci. Technol. 41, 4065–4070. doi:10.1021/es062208b
Meng, S., Fan, W., Li, X., Liu, Y., Liang, D., and Liu, X. (2018). Intermolecular Interactions of Polysaccharides in Membrane Fouling during Microfiltration. Water Res. 143, 38–46. doi:10.1016/j.watres.2018.06.027
Millanar-Marfa, J. M. J., Borea, L., Castrogiovanni, F., Hasan, S. W., Choo, K.-H., Korshin, G. V., et al. (2021). Self-forming Dynamic Membranes for Wastewater Treatment. Sep. Purif. Rev 51 (2), 195–211. doi:10.1080/15422119.2021.1887223
More, T. T., Yadav, J. S. S., Yan, S., Tyagi, R. D., and Surampalli, R. Y. (2014). Extracellular Polymeric Substances of Bacteria and Their Potential Environmental Applications. J. Environ. Manage. 144, 1–25. doi:10.1016/j.jenvman.2014.05.010
Nielsen, P. H., and Jahn, A. (1999). “Extraction of EPS,” in Microbial Extracellular Polymeric Substances. Editor Wingender, J., Neu, T. R., and Flemming, H. C. (Berlin, Heidelberg: Springer) 49–72. doi:10.1007/978-3-642-60147-7_3
O’Toole, G. A. (2011). Microtiter Dish Biofilm Formation Assay. J. Vis. Exp. 47, 2437. doi:10.3791/2437
Pan, X., Liu, J., Zhang, D., Chen, X., Song, W., and Wu, F. (2010). Binding of Dicamba to Soluble and Bound Extracellular Polymeric Substances (EPS) from Aerobic Activated Sludge: A Fluorescence Quenching Study. J. Colloid Interf. Sci. 345, 442–447. doi:10.1016/j.jcis.2010.02.011
Park, C., and Helm, R. F. (2008). Application of Metaproteomic Analysis for Studying Extracellular Polymeric Substances (EPS) in Activated Sludge Flocs and Their Fate in Sludge Digestion. Water Sci. Technol. 57, 2009–2015. doi:10.2166/wst.2008.620
Pollice, A., and Vergine, P. (2020). “Self-forming Dynamic Membrane Bioreactors (SFD MBR) for Wastewater Treatment: Principles and Applications,” in Current Developments in Biotechnology and Bioengineering (Elsevier) 235–258. doi:10.1016/b978-0-12-819854-4.00010-1
Rusanowska, P., Cydzik-Kwiatkowska, A., and Wojnowska-Baryła, I. (2019). Microbial Origin of Excreted DNA in Particular Fractions of Extracellular Polymers (EPS) in Aerobic Granules. Water Air Soil Pollut. 230, 203. doi:10.1007/s11270-019-4248-0
Sabaghian, M., Mehrnia, M. R., Esmaieli, M., and Noormohammadi, D. (2018). Formation and Performance of Self-Forming Dynamic Membrane (SFDM) in Membrane Bioreactor (MBR) for Treating Low-Strength Wastewater. Water Sci. Technol. 78, 904–912. doi:10.2166/wst.2018.368
Seviour, T., Derlon, N., Dueholm, M. S., Flemming, H.-C. C., Girbal-Neuhauser, E., Horn, H., et al. (2018). Extracellular Polymeric Substances of Biofilms: Suffering from an Identity Crisis. Water Res. 151, 1–7. doi:10.1016/j.watres.2018.11.020
Shi, Y., Huang, J., Zeng, G., Gu, Y., Chen, Y., Hu, Y., et al. (2017). Exploiting Extracellular Polymeric Substances (EPS) Controlling Strategies for Performance Enhancement of Biological Wastewater Treatments: An Overview. Chemosphere 180, 396–411. doi:10.1016/j.chemosphere.2017.04.042
Shi, Y., and Liu, Y. (2021). Evolution of Extracellular Polymeric Substances (EPS) in Aerobic Sludge Granulation: Composition, Adherence and Viscoelastic Properties. Chemosphere 262, 128033. doi:10.1016/j.chemosphere.2020.128033
Siddiqui, M. A., Biswal, B. K., Saleem, M., Guan, D., Iqbal, A., Wu, D., et al. (2021). Anaerobic Self-Forming Dynamic Membrane Bioreactors (AnSFDMBRs) for Wastewater Treatment – Recent Advances, Process Optimization and Perspectives. Bioresour. Technol. 332, 125101. doi:10.1016/j.biortech.2021.125101
Simon, S., Païro, B., Villain, M., D’Abzac, P., Hullebusch, E., Lens, P., et al. (2009). Evaluation of Size Exclusion Chromatography (SEC) for the Characterization of Extracellular Polymeric Substances (EPS) in Anaerobic Granular Sludges. Bioresour. Technol. 100, 6258–6268. doi:10.1016/j.biortech.2009.07.013
Sudmalis, D., Da Silva, P., Temmink, H., Bijmans, M. M., and Pereira, M. A. (2018). Biological Treatment of Produced Water Coupled with Recovery of Neutral Lipids. Water Res. 147, 33–42. doi:10.1016/j.watres.2018.09.050
Tanny, G. B. (1978). Dynamic Membranes in Ultrafiltration and Reverse Osmosis. Sep. Purif. Rev. 7, 183–220. doi:10.1080/03602547808066063
Tsapiuk, E. A. (1996). Ultrafiltration Separation of Aqueous Solutions of Poly(ethylene Glycol)s on the Dynamic Membrane Formed by Gelatin. J. Memb. Sci. 116, 17–29. doi:10.1016/0376-7388(95)00320-7
Tual, C., Espuche, E., Escoubes, M., and Domard, A. (2000). Transport Properties of Chitosan Membranes: Influence of Crosslinking. J. Polym. Sci. Part. B Polym. Phys. 38, 1521–1529. doi:10.1002/(SICI)1099-0488(20000601)38:11<1521::AID-POLB120>3.0.CO;2-%23
van den Brink, P., Zwijnenburg, A., Smith, G., Temmink, H., and van Loosdrecht, M. (2009). Effect of Free Calcium Concentration and Ionic Strength on Alginate Fouling in Cross-Flow Membrane Filtration. J. Memb. Sci. 345, 207–216. doi:10.1016/j.memsci.2009.08.046
van Lier, J. B., van der Zee, F. P., Frijters, C. T. M. J., and Ersahin, M. E. (2015). Celebrating 40 Years Anaerobic Sludge Bed Reactors for Industrial Wastewater Treatment. Rev. Environ. Sci. Biotechnol. 14, 681–702. doi:10.1007/s11157-015-9375-5
Wang, X. M., and Waite, T. D. (2008). Gel Layer Formation and Hollow Fiber Membrane Filterability of Polysaccharide Dispersions. J. Memb. Sci. 322, 204–213. doi:10.1016/j.memsci.2008.05.033
Wang, X. M., and Waite, T. D. (2009). Role of Gelling Soluble and Colloidal Microbial Products in Membrane Fouling. Environ. Sci. Technol. 43, 9341–9347. doi:10.1021/es9013129
Wang, X., Zhao, Y., Yuan, B., Wang, Z., Li, X., and Ren, Y. (2016). Comparison of Biofouling Mechanisms between Cellulose Triacetate (CTA) and Thin-Film Composite (TFC) Polyamide Forward Osmosis Membranes in Osmotic Membrane Bioreactors. Bioresour. Technol. 202, 50–58. doi:10.1016/j.biortech.2015.11.087
Wang, Z., Wu, Z., and Tang, S. (2009). Extracellular Polymeric Substances (EPS) Properties and Their Effects on Membrane Fouling in a Submerged Membrane Bioreactor. Water Res. 43, 2504–2512. doi:10.1016/j.watres.2009.02.026
Wang, Z., Wu, Z., Yin, X., and Tian, L. (2008). Membrane Fouling in a Submerged Membrane Bioreactor (MBR) under Sub-critical Flux Operation: Membrane Foulant and Gel Layer Characterization. J. Memb. Sci. 325, 238–244. doi:10.1016/j.memsci.2008.07.03510.2166/wst.2008.111
Xiong, J., Fu, D., Singh, R. P., and Ducoste, J. J. (2016). Structural Characteristics and Development of the Cake Layer in a Dynamic Membrane Bioreactor. Sep. Purif. Technol. 167, 88–96. doi:10.1016/j.seppur.2016.04.040
Yan, Z. R., Meng, H. S., Yang, X. Y., Zhu, Y. Y., Li, X. Y., Xu, J., et al. (2019). Insights into the Interactions between Triclosan (TCS) and Extracellular Polymeric Substance (EPS) of Activated Sludge. J. Environ. Manage. 232, 219–225. doi:10.1016/j.jenvman.2018.11.059
Yao, M., Zhang, K., and Cui, L. (2010). Characterization of Protein-Polysaccharide Ratios on Membrane Fouling. Desalination 259, 11–16. doi:10.1016/j.desal.2010.04.049
Yu, H., Wang, Z., Wu, Z., and Zhu, C. (2015). Dynamic Membrane Formation in Anaerobic Dynamic Membrane Bioreactors: Role of Extracellular Polymeric Substances. PLoS One 10, e0139703. doi:10.1371/journal.pone.0139703
Yuan, B., Wang, X., Tang, C., Li, X., and Yu, G. (2015). In Situ observation of the Growth of Biofouling Layer in Osmotic Membrane Bioreactors by Multiple Fluorescence Labeling and Confocal Laser Scanning Microscopy. Water Res. 75, 188–200. doi:10.1016/j.watres.2015.02.048
Yun, M. A., Yeon, K. M., Park, J. S., Lee, C. H., Chun, J., and Lim, D. J. (2006). Characterization of Biofilm Structure and its Effect on Membrane Permeability in MBR for Dye Wastewater Treatment. Water Res. 40, 45–52. doi:10.1016/j.watres.2005.10.035
Zhang, M., Sun, C., and Li, Q. (2018). “Interaction between the Polysaccharides and Proteins in Semisolid Food Systems,” in Encyclopedia of Food Chemistry. Editors Varelis, , Peter, , Melton, L., and Shahidi, F. (Elsevier) 439–445. doi:10.1016/B978-0-08-100596-5.21474-1
Zhang, X., Wang, Z., Wu, Z., Lu, F., Tong, J., and Zang, L. (2010). Formation of Dynamic Membrane in an Anaerobic Membrane Bioreactor for Municipal Wastewater Treatment. Chem. Eng. J. 165, 175–183. doi:10.1016/j.cej.2010.09.013
Keywords: anaerobic digestion, extracellular polymeric substances, membrane filtration, gel layer, dynamic membrane, confocal laser scanning microscopy
Citation: Dinis Costa EF, Gagliano MC, Kemperman A, Rijnaarts HHM, Lammertink RGH and Temmink H (2022) Hydraulic Resistance and Macromolecular Structure of Aerobic and Anaerobic Mixed-Culture Extracellular Polymeric Substances Gel Layers: Opportunities and Challenges. Front. Environ. Sci. 10:774536. doi: 10.3389/fenvs.2022.774536
Received: 12 September 2021; Accepted: 07 March 2022;
Published: 22 March 2022.
Edited by:
Susana Rodriguez-Couto, LUT University, FinlandReviewed by:
Mohamed Hassaan, National Institute of Oceanography and Fisheries (NIOF), EgyptPompilio Vergine, IRSA-CNR, Italy
Copyright © 2022 Dinis Costa, Gagliano, Kemperman, Rijnaarts, Lammertink and Temmink. This is an open-access article distributed under the terms of the Creative Commons Attribution License (CC BY). The use, distribution or reproduction in other forums is permitted, provided the original author(s) and the copyright owner(s) are credited and that the original publication in this journal is cited, in accordance with accepted academic practice. No use, distribution or reproduction is permitted which does not comply with these terms.
*Correspondence: Maria Cristina Gagliano, Q3Jpc3RpbmEuZ2FnbGlhbm9Ad2V0c3VzLm5s
†These authors have contributed equally to this work