- 1Systems Medicine, Clinical Pharmacology and Safety Sciences, R&D BioPharmaceuticals, AstraZeneca, Gaithersburg, MD, United States
- 2BioPharmaceuticals Medical, AstraZeneca, Baar, Switzerland
- 3Global Safety, Health and Environment, AstraZeneca, Macclesfield, United Kingdom
- 4Clinical Pharmacology and Safety Sciences, R&D BioPharmaceuticals, AstraZeneca, Hertfordshire, United Kingdom
Pressurized metered-dose inhalers (pMDIs) deliver life-saving medications to patients with respiratory conditions and are the most used inhaler delivery device globally. pMDIs utilize a hydrofluoroalkane (HFA), also known as an F-gas, as a propellant to facilitate the delivery of medication into the lungs. Although HFAs have minimal impact on ozone depletion, their global warming potential (GWP) is more than 1,000 times higher than CO2, bringing them in scope of the F-Gas Regulation in the European Union (EU). The pharmaceutical industry is developing solutions, including a near-zero GWP “next-generation propellant,” HFO-1234ze(E). At the same time, the EU is also evaluating a restriction on per-and polyfluoroalkyl substances (PFAS) under the Registration, Evaluation, Authorization, and Restriction of Chemicals (REACH) regulation. Trifluoroacetic acid (TFA) is a persistent PFAS and a potential degradation product of HFO-1234ze(E). We quantified yield of TFA from HFO-1234ze(E) using a computational model under Europe-relevant atmospheric conditions. The modeling suggests that most HFO-1234ze(E) degrades into formyl fluoride within 20 days (≥85%) even at the highest examined altitude. These results suggest that TFA yield from HFO-1234ze(E) varies between 0%–4% under different atmospheric conditions. In 2022, France represented the highest numbers of pMDI units sold within the EU, assuming these pMDIs had HFO-1234ze(E) as propellant, we estimate an annual rainwater TFA deposition of ∼0.025 μg/L. These results demonstrate negligible formation of TFA as a degradation product of HFO-1234ze(E), further supporting its suitability as a non-persistent, non-bioaccumulative, and non-toxic future propellant for pMDI devices to safeguard access for patients to these essential medicines.
Background
Asthma and chronic obstructive pulmonary disease (COPD) are serious, potentially life-threatening diseases, affecting more than 550 million people worldwide (Soriano et al., 2020). Pharmacotherapy delivered to the lung by inhalation is the standard of care and recommended for treating people living with respiratory diseases. Selecting the right inhaler regimen for an individual patient is crucial to reduce symptoms and exacerbation risk. Numerous factors need to be considered when selecting the correct inhaled therapy such as the active pharmaceutical ingredient (API), inspiratory capacity, cognition and dexterity, preference for a device type, affordability, among others. Device type is a particularly important consideration with pressurized metered dose inhalers (pMDIs), dry-powder inhalers (DPIs), and soft-mist inhalers as often prescribing options.
Of the three delivery device types, pMDIs are most used globally (∼78%) with greater than 90% use in some countries (Bell et al., 2022). Historically, pMDIs used chlorofluorocarbon (CFC) as a propellant, which was known to deplete the ozone layer in the atmosphere. The Montreal protocol called for the phase-out of the CFC propellants, prompting the pharmaceutical industry to develop hydrofluoroalkanes (HFAs) as a replacement propellant. HFAs do not deplete ozone but have high global warming potential (GWP), e.g., HFA-134a has GWP100 of 1,530 (The Earth’s Energy Budget, 2023). In addition to pMDIs, HFAs have many other usages and can substantially affect global surface temperatures over time due to their high GWP (Song et al., 2023); to mitigate global warming, parties that had previously committed to the Montreal Protocol made an amendment (Kigali Amendment) to phase down the production and consumption of HFAs over 30 years (Heath, 2017). The search for alternative partially fluorinated compounds with low GWP led to identification of hydrofluoroolefin (HFO) compounds. In particular, HFO-1234ze(E), hereafter referred to as HFO-1234ze, was identified as a potential next-generation propellant for pMDIs as it has near-zero GWP (The Earth’s Energy Budget, 2023); has physicochemical properties like HFA-134a and HFA-227ea; and is non-bioaccumulative and non-toxic (Giffen et al., 2022). Using a cradle-to-grave life cycle assessment, Hargreaves et al. (2022) demonstrated that replacement of HFA-134a with HFO-1234ze reduced the carbon footprint of pMDI device by ∼92%, akin to that of a DPI.
On 22 March 2023, Germany, Netherlands, Sweden, Norway, and Denmark (Dossier submitters) submitted a restriction proposal, under the Registration, Evaluation, Authorization, and Restriction of Chemicals (REACH) regulation, to the European Chemical Agency (ECHA) for a ban on more than 10,000 per- and polyfluoroalkyl substances (PFAS) chemicals, allowing exceptions for human and veterinary APIs, biocides, pesticides, and a few fully degradable PFAS subgroups. As per the Organization for Economic Cooperation and Development definition (Wang et al., 2021), “PFASs are defined as fluorinated substances that contain at least one fully fluorinated methyl (–CF3) or methylene (–CF2–) carbon atom (without any H/Cl/Br/I atom attached to it.” The carbon-fluorine bond is often highly stable and resistant to degradation via natural processes, which has led to the moniker of ‘forever chemicals’ when referring to the class. Although the parent molecule only has an environmental exposure of 19 days (Neale et al., 2021), the novel propellant HFO-1234ze may fall under the purview of the “PFAS proposal” because of the presence of a trifluoromethyl group (–CF3) in its structure, which is associated with formation of trifluoracetic acid (TFA)–a virtually non-degradable compound found naturally in the environment (Scott et al., 2005). The Dossier submitters argued that chemicals having the trifluoromethyl group (–CF3) “are potential precursors to trifluoroacetic acid (TFA)” and their unchecked environmental release could lead to accumulation of toxic-levels of TFA in the environment.
The toxicology of TFA has been explored in a range of non-clinical rodent (rat) studies, which revealed non-adverse mild liver hypertrophy following acute exposure of up to 2,400 parts-per-million sodium TFA for 14 days (Dekant and Dekant, 2023). No adverse effects were detected when TFA was administered to rats for 90 days, or in dedicated reproductive and developmental toxicology studies, or in vitro genetic toxicity experiments (Dekant and Dekant, 2023). Based on the levels of TFA assessed in the toxicology studies and the potential for human environmental exposure to TFA, it was concluded that the “margin of exposures” of TFA do not indicate human health risks (Dekant and Dekant, 2023; Madronich et al., 2023). To assess the impact of HFO-1234ze-propelled pMDIs to environmental TFA depositions, we first outlined the possible degradation routes of HFO-1234ze in the atmosphere, and then estimated the maximum TFA yield by varying atmospheric conditions using a box-modeling approach. Afterwards, we assessed the annual TFA rainwater concentration assuming all pMDIs in France have transitioned to the next-generation propellant HFO-1234ze.
Effect of next-generation propellant on TFA deposition in the environment
To simulate the HFO-1234ze degradation kinetics at different altitudes, we implemented the chemical degradation mechanism (Supplementary Figure S1) in the box model (Wolfe et al., 2016) based on physicochemical data available in the literature (Lightfoot et al., 1992; Wallington et al., 1994; Tyndall et al., 2001; Sulbaek Andersen et al., 2004a; Sulbaek Andersen et al., 2004b; Chiappero et al., 2006; Søndergaard et al., 2007; Ammann et al., 2016). In the model, we captured the effect of different temperatures; pressures; and different abundances of reactants that play a central role in controlling the yield of TFA. We obtained the concentrations of these important reactants OH•, NO, NO2, HO2• from a global atmospheric chemistry model (Reifenberg et al., 2022) averaged over Europe (Supplementary Figure S2). For chemical reactions with missing rate constants, we used their values from analogous reactions. In the text below, we describe the major steps involved in chemical degradation of HFO-1234ze and formation of TFA.
Step 1: Oxidation of HFO-1234ze by hydroxy radicals
In the presence of O2, the hydroxyl radicals attack HFO-1234ze across the double bond, initiating its degradation and forming two peroxy radicals (1a, 1b; Table 1). In Figure 1A, we have depicted the possible fate of HFO-1234ze emissions within the boundary layer. Figure 1B shows simulation of HFO-1234ze degradation at various altitudes (0, 4, 6, and 10 km), suggesting that HFO-1234ze has a lifetime of ∼20 days, which is like the 19-day lifetime reported elsewhere (Neale et al., 2021). Given the short atmospheric lifetimes, these results also suggest that HFO-1234ze would have a weak vertical gradient, throughout the troposphere (0–10 km at mid-latitudes) and that its oxidation will take place at temperatures between 300 and 220 K.
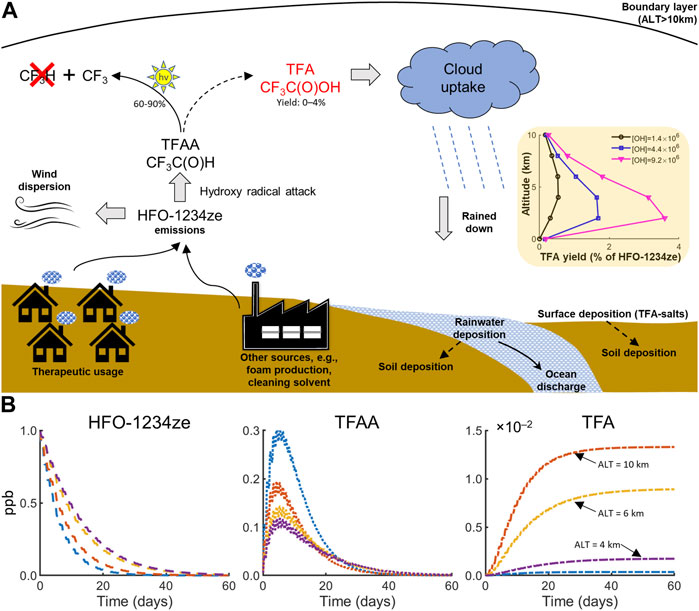
FIGURE 1. HFO-1234ze(E) emission and degradation in the atmosphere. (A) Schematics depicting possible fate of HFO-1234ze emissions within the boundary layer of the atmosphere. In addition to interacting with the atmospheric reactants within a region, HFO-1234ze emissions released in the atmosphere would experience wind dispersion. In the atmosphere, photolysis reaction dominates the removal of trifluoro-acetaldehyde (TFAA), formed after the hydroxy radical attack on the double bond of HFO-1234ze. Recent work (Andersen and Nielsen, 2022) has demonstrated that photolysis of TFAA does not produce CF3H (HFC-23; GWP100 12,960). A downstream product of TFAA’s interaction with hydroxyl radicals is the production of trifluoroacetic acid (TFA). TFA accumulated due to in-cloud hydrolysis of HFO-1234ze is removed from the atmosphere via rain, snow, and fog (Wang et al., 2014). The solid arrow indicates TFAA photodissociation forms CF3, whereas dotted arrow indicates TFA is a downstream product of TFAA degradation. Beige shaded inset: Computational estimate of TFA yield with altitude as a function of increasing concentration (black, blue, and pink) of hydroxy radicals. (B) Model simulations showing kinetics of HFO-1234ze degradation, TFAA formation and degradation, and TFA formation at different altitudes above the surface, i.e., 0 (blue), 4 (purple), 6 (yellow), and 10 (orange) km. All chemicals are in units of parts-per-billion (ppb).
Step 2: Formation of trifluoro-acetaldehyde
The organic peroxy radicals (RO2) formed in Step 1 (above) can react with NO, NO2, other RO2, and HO2. We have not considered their reaction with NO2 because peroxynitrates formed after NO2 reaction are thermally unstable. In NO-rich experimental conditions, Javadi et al. (2008) found a ∼100% yield of trifluoro-acetaldehyde (TFAA) and formyl fluoride; however, under atmospheric conditions the TFAA yield would depend on i) relative concentration of NO and HO2 and ii) the rate constants of reactions 2a, 2b, 2c, and 2d. In our model, we have used temperature-independent generic rate constants for these reactions (Lightfoot et al., 1992; Tyndall et al., 2001) because their specific measurements are unavailable, which requires further studies.
Step 3: Atmospheric degradation of trifluoro-acetaldehyde
In the atmosphere, there are two main routes of TFAA degradation: i) OH• radicals and ii) photodissociation. The formation of TFA occurs via the first route through an acyl-peroxyl radical (4d; Table 1), which itself is formed when TFAA reacts with OH• radicals (3a; Table 1). The photodissociation rate constant works as a sink for TFA formation in the atmosphere; however, there is no wavelength-dependent data available in literature. In TFA calculations, we have used the photodissociation rate constants at different altitudes reported by Chiappero et al. (Chiappero et al., 2006).
Step 4: Possible fate of acyl-peroxyl radical
The acyl-peroxyl radical reaction (4d; Table 1) forming TFA occurs with HO2. However, as with other peroxyl radicals, this acyl-peroxyl radical has many competing reactants (e.g., NO, NO2) in the atmosphere (4a and 4b; Table 1). The rate constant of TFA forming reaction (4d; Table 1) is unknown. Andersen et al. (Sulbaek Andersen et al., 2004b), however, have determined the branching ratios of the three HO2 reactions with the acyl-peroxyl radical at room temperature, which were used in our TFA computations. The rate constant of the analogous non-fluorinated acyl-peroxyl radical was obtained from a paper (Ammann et al., 2016).
TFA yield and deposition due to novel pMDI usage
We implemented the chemical reactions and constants associated with HFO-1234ze degradation (Table 1) in a box model (Wolfe et al., 2016) to quantify average yield of TFA under different atmospheric conditions. In the Supplementary Material (Text S1), we have provided the atmospheric conditions and modeling assumptions used for generating the estimates shown in Figure 1A (inset) and 1B. To assess the impact of humidity (water vapor)—the primary source of hydroxyl radicals (Stevenson et al., 2020)—on HFO-1234ze degradation, we performed three independent simulations varying the atmospheric hydroxyl concentration between (units of 10−6 molecules/cm3) 1.0 to 10, ensuring its maximum is below the averaged diurnal maximum (Ma et al., 2022). We found that these hydroxyl concentration variations caused the TFA yield to vary between 0% and 4%, irrespective of the altitude. Our results are in agreement with a recent chemistry transport modeling study (Andersen et al., 2018), which reported an average TFA yield of 2% due to atmospheric breakdown of a structurally similar compound (HCFO-1233zd). In contrast to the 4% TFA yield of HFO-1234ze, APIs such as isoflurane and sevoflurane have an estimated TFA yield of 95% and 100%, respectively (Andersen et al., 2023).
In 2022, as per IQVIA STAR/MIDAS 2023 sales data of the EU, the greatest number of pMDI units (∼21 million) were sold in France, which is equivalent to about 300 tons of propellant usage. Assuming HFO-1234ze was the propellant in these pMDIs and there was zero wastage of the propellant, this roughly equals to 2.673 × 106 mol/year of propellant emissions in the atmosphere. Using the total precipitation volume of 4.76 × 1014 L for France (Knoema, 2021) and the maximally estimated 4% TFA-yield, this suggests that the maximum concentration of TFA in rainwater could be 2.2 × 10−10 M (or 0.025 μg/L). On the other hand, if we consider the IQVIA STAR/MIDAS 2023 sales data of the entire Europe, the greatest number of pMDI units (∼56 million) were sold in the United Kingdom (UK). By repeating our TFA calculations and using the total precipitation volume of 2.97 × 1014 L for United Kingdom (Knoema, 2021), we found that maximum concentration of TFA in rainwater could be ∼0.107 μg/L, which is about 100,000-fold smaller than the no observed effect concentration of TFA for aquatic species (Solomon et al., 2016). Our results clearly demonstrate that even under this UK-case scenario, wherein the pMDI sales were 2-fold higher and precipitation was 2-fold lower than France, pMDI devices using the HFO-1234ze propellant would form negligible amount of rainwater TFA, suggesting no threat to the human health and the ecosystem.
Limitations of the study
At different altitudes, the TFA yield depends on the availability of other atmospheric gases and the rate constants of associated reactions, which may have temperature, pressure, and ultraviolet wavelength dependence. Currently, detailed experimental characterization of all reactions (Table 1) is unavailable, which may affect our TFA yield and deposition estimates. Furthermore, in our calculations, we have treated acyl-peroxy nitrate product (APN) CF3C(O)OONO2 as a thermally stable product (4b; Table 1), whereas it is thermally unstable, and, at low-mid altitudes, this product can influence TFA yields by forming the acyl-peroxyl radical CF3C(O)OO• again. However, the CF3C(O)OO• radical will also react with RO2 (e.g., from the degradation of the hundreds of hydrocarbons in the air) by competing with its HO2 reactions (4c-e; Table 1), leading to decrease in the TFA yield. Lastly, the RO2 formed in Step 1 (1a-b; Table 1) would react with other RO2 in the atmosphere which we have not considered in the model. These limitations warrant a global chemical-transport modeling study to obtain more robust estimate of the TFA yield by accounting for transport of the chemical species (including HFO-1234ze, TFA, APNs, and hydrocarbons) into other regions with region-specific meteorological data. Nevertheless, our present calculations provide a broad demonstration of the range of the TFA yield with atmospheric conditions, within which the true TFA yield is likely to fall.
Epilogue
In this article, we first computed the range of TFA yield formed during the chemical degradation of HFO-1234ze under Europe-relevant atmospheric conditions. Afterwards, we used the estimated maximum of the TFA yield to calculate annual rainwater concentrations of TFA in France, assuming HFO-1234ze as the propellant of all pMDIs sold in a year. Our analysis supports the following:
1. HFO-1234ze, which is non-bioaccumulative and non-toxic, has an approximately 20 days lifetime in the atmosphere and leads to negligible, if any, yield of TFA, which is persistent but non-bioaccumulative and non-toxic.
2. Restricting HFO limits options for HFA transition to HFA-152a only, currently, which itself is subjected to future down-phasing because of its GWP, under the EU F-Gas Regulation, risking the viability of future pMDI supply.
3. Any evaluation of HFO-1234ze restriction must take into consideration the urgent need to identify innovative solutions to reduce greenhouse gas emissions across societies whilst safeguarding access to essential medicines.
Medications delivered by pMDIs are relied upon by millions of patients globally and they are the most used device type. Therefore, considering HFO-1234ze non-persistence and its infinitesimal contribution to rainwater TFA, the PFAS proposal should exempt the next-generation propellant HFO-1234ze to maintain the future supply of pMDI devices to people living with chronic respiratory diseases.
Data availability statement
The original contributions presented in the study are included in the article/Supplementary Material, further inquiries can be directed to the corresponding author.
Author contributions
ST: Conceptualization, Formal analysis, Investigation, Methodology, Software, Visualization, Writing–original draft, Writing–review and editing. JB: Writing–review and editing. NB: Data curation, Methodology, Writing–review and editing. SP: Funding acquisition, Supervision, Writing–review and editing. MG: Funding acquisition, Supervision, Writing–review and editing. PN: Supervision, Writing–review and editing. HK: Funding acquisition, Supervision, Visualization, Writing–review and editing.
Funding
The author(s) declare financial support was received for the research, authorship, and/or publication of this article. AstraZeneca supported this study.
Conflict of interest
All authors are employees of AstraZeneca and hold shares/share options in AstraZeneca.
The author(s) declared that they were an editorial board member of Frontiers, at the time of submission. This had no impact on the peer review process and the final decision.
Publisher’s note
All claims expressed in this article are solely those of the authors and do not necessarily represent those of their affiliated organizations, or those of the publisher, the editors and the reviewers. Any product that may be evaluated in this article, or claim that may be made by its manufacturer, is not guaranteed or endorsed by the publisher.
Author disclaimer
IQVIA™ did not provide any support for the analysis or interpretation of the data. The statements, findings, conclusions, views, and opinions contained and expressed in this article are based, in part, on data obtained under license from the following IQVIA™ information service: IQVIA™ MIDAS inhaler sales data across all respiratory conditions (2023) in European countries, reflecting estimates of real-world activity. These statements, findings, conclusions, views, and opinions are not necessarily those of IQVIA™ or any of its affiliated or subsidiary entities.
Supplementary material
The Supplementary Material for this article can be found online at: https://www.frontiersin.org/articles/10.3389/fenvs.2023.1297920/full#supplementary-material
References
Andersen, M. P. S., and Nielsen, O. J. (2022). Tropospheric photolysis of CF3CHO. Atmos. Environ. 272, 118935. doi:10.1016/j.atmosenv.2021.118935
Andersen, M. P. S., Nielsen, O. J., and Sherman, J. D. (2023). Assessing the potential climate impact of anaesthetic gases. Lancet Planet. Health 7 (7), e622–e629. doi:10.1016/s2542-5196(23)00084-0
Andersen, M. P. S., Schmidt, J. A., Volkova, A., and Wuebbles, D. J. (2018). A three-dimensional model of the atmospheric chemistry of E and Z-CF3CH= CHCl (HCFO-1233 (zd)(E/Z)). Atmos. Environ. 179, 250–259. doi:10.1016/j.atmosenv.2018.02.018
Bell, J., et al. (2022)“An assessment of pressurized metered-dose inhaler use in countries in Europe and the rest of the world,” in D22. Hot topics in behavioral sciences and health services research. 2023 (American: American Thoracic Society), A6315.
Chiappero, M. S., Malanca, F. E., Argüello, G. A., Wooldridge, S. T., Hurley, M. D., Ball, J. C., et al. (2006). Atmospheric chemistry of perfluoroaldehydes (CxF2x+1CHO) and fluorotelomer aldehydes (CxF2x+1CH2CHO): quantification of the important role of photolysis. J. Phys. Chem. A 110 (43), 11944–11953. doi:10.1021/jp064262k
Dekant, W., and Dekant, R. (2023). Mammalian toxicity of trifluoroacetate and assessment of human health risks due to environmental exposures. Archives Toxicol. 97 (4), 1069–1077. doi:10.1007/s00204-023-03454-y
Giffen, P. S., et al. (2022) The nonclinical assessment of trans, tetrafluoropropene (HFO-1234ze (E)), a low global warming potential propellant for use in metered dose inhalation Products1,.
Hargreaves, C., et al. (2022), A new medical propellant HFO-1234ze(E): reducing the environmental impact of inhaled medicines. Under review.
Heath, E. A. (2017). Amendment to the Montreal protocol on substances that deplete the ozone layer (kigali amendment). Int. Leg. Mater. 56 (1), 193–205. doi:10.1017/ilm.2016.2
Javadi, M. S., Søndergaard, R., Nielsen, O. J., Hurley, M. D., and Wallington, T. J. (2008). Atmospheric chemistry of trans-CF<sub>3</sub>CH=CHF: products and mechanisms of hydroxyl radical and chlorine atom initiated oxidation. Atmos. Chem. Phys. 8 (12), 3141–3147. doi:10.5194/acp-8-3141-2008
Lightfoot, P. D., Cox, R., Crowley, J., Destriau, M., Hayman, G., Jenkin, M., et al. (1992). Organic peroxy radicals: kinetics, spectroscopy and tropospheric chemistry. Atmos. Environ. Part A. General Top. 26 (10), 1805–1961. doi:10.1016/0960-1686(92)90423-i
Ma, X., Tan, Z., Lu, K., Yang, X., Chen, X., Wang, H., et al. (2022). OH and HO<sub>2</sub> radical chemistry at a suburban site during the EXPLORE-YRD campaign in 2018. Atmos. Chem. Phys. 22 (10), 7005–7028. doi:10.5194/acp-22-7005-2022
Madronich, S., Sulzberger, B., Longstreth, J. D., Schikowski, T., Andersen, M. P. S., Solomon, K. R., et al. (2023). Changes in tropospheric air quality related to the protection of stratospheric ozone in a changing climate. Photochem. Photobiological Sci. 22 (5), 1129–1176. doi:10.1007/s43630-023-00369-6
Neale, R. E., Barnes, P. W., Robson, T. M., Neale, P. J., Williamson, C. E., Zepp, R. G., et al. (2021). Environmental effects of stratospheric ozone depletion, UV radiation, and interactions with climate change: UNEP Environmental Effects Assessment Panel, Update 2020. Photochem. Photobiological Sci. 20 (1), 1–67. doi:10.1007/s43630-020-00001-x
Reifenberg, S. F., Martin, A., Kohl, M., Bacer, S., Hamryszczak, Z., Tadic, I., et al. (2022). Numerical simulation of the impact of COVID-19 lockdown on tropospheric composition and aerosol radiative forcing in Europe. Atmos. Chem. Phys. 22 (16), 10901–10917. doi:10.5194/acp-22-10901-2022
Scott, B., Macdonald, R. W., Kannan, K., Fisk, A., Witter, A., Yamashita, N., et al. (2005). Trifluoroacetate profiles in the arctic, atlantic, and pacific oceans. Environ. Sci. Technol. 39 (17), 6555–6560. doi:10.1021/es047975u
Solomon, K. R., Velders, G. J. M., Wilson, S. R., Madronich, S., Longstreth, J., Aucamp, P. J., et al. (2016). Sources, fates, toxicity, and risks of trifluoroacetic acid and its salts: relevance to substances regulated under the Montreal and Kyoto Protocols. J. Toxicol. Environ. Health B Crit. Rev. 19 (7), 289–304. doi:10.1080/10937404.2016.1175981
Søndergaard, R., Nielsen, O., Hurley, M., Wallington, T., and Singh, R. (2007). Atmospheric chemistry of trans-CF3CHCHF: kinetics of the gas-phase reactions with Cl atoms, OH radicals, and O3. Chem. Phys. Lett. 443 (4-6), 199–204. doi:10.1016/j.cplett.2007.06.084
Song, J., Tong, G., Chao, J., Chung, J., Zhang, M., Lin, W., et al. (2023). Data driven pathway analysis and forecast of global warming and sea level rise. Sci. Rep. 13 (1), 5536. doi:10.1038/s41598-023-30789-4
Soriano, J. B., Kendrick, P. J., Paulson, K. R., Gupta, V., Abrams, E. M., Adedoyin, R. A., et al. (2020). Prevalence and attributable health burden of chronic respiratory diseases, 1990-2017: a systematic analysis for the Global Burden of Disease Study 2017. Lancet Respir. Med. 8 (6), 585–596. doi:10.1016/s2213-2600(20)30105-3
Stevenson, D. S., Zhao, A., Naik, V., O'Connor, F. M., Tilmes, S., Zeng, G., et al. (2020). Trends in global tropospheric hydroxyl radical and methane lifetime since 1850 from AerChemMIP. Atmos. Chem. Phys. 20 (21), 12905–12920. doi:10.5194/acp-20-12905-2020
Sulbaek Andersen, M., et al. (2004a). Atmospheric chemistry of n-C x F2 x+ 1CHO (x= 1, 3, 4): reaction with Cl atoms, OH radicals and IR spectra of C x F2 x+ 1C (O) O2NO2. J. Phys. Chem. A 108 (24), 5189–5196. doi:10.1021/jp0496598
Sulbaek Andersen, M., et al. (2004b). Atmospheric Chemistry of n-C x F2 x+ 1CHO (x= 1, 3, 4): mechanism of the C x F2 x+ 1C (O) O2+ HO2 Reaction. J. Phys. Chem. A 108 (30), 6325–6330. doi:10.1021/jp048849f
The Earth’s Energy Budget, (2023) The Earth’s Energy Budget, climate feedbacks and climate sensitivity, in Climate Change 2021 – the physical Science Basis: Working group I contribution to the Sixth assessment Report of the Intergovernmental Panel on Climate Change, C. Intergovernmental Panel on Climate, Editor. 2023, Cambridge University Press: Cambridge. p. 923–1054.
Tyndall, G., Cox, R. A., Granier, C., Lesclaux, R., Moortgat, G. K., Pilling, M. J., et al. (2001). Atmospheric chemistry of small organic peroxy radicals. J. Geophys. Res. Atmos. 106 (D11), 12157–12182. doi:10.1029/2000jd900746
Wallington, T. J., Sehested, J., and Nielsen, O. J. (1994). Atmospheric chemistry of CF3C (O) O2 radicals. Kinetics of their reaction with NO2 and kinetics of the thermal decomposition of the product CF3C (O) O2NO2. Chem. Phys. Lett. 226 (5-6), 563–569. doi:10.1016/0009-2614(94)00751-9
Wang, Q., Wang, X., and Ding, X. (2014). Rainwater trifluoroacetic acid (TFA) in Guangzhou, South China: levels, wet deposition fluxes and source implication. Sci. total Environ. 468, 272–279. doi:10.1016/j.scitotenv.2013.08.055
Wang, Z., Buser, A. M., Cousins, I. T., Demattio, S., Drost, W., Johansson, O., et al. (2021). A new OECD definition for per-and polyfluoroalkyl substances. Environ. Sci. Technol. 55 (23), 15575–15578. doi:10.1021/acs.est.1c06896
Keywords: hydrofluoroolefin, metered-dose inhalers, trifluoroacetic acid, environment, computational analyses
Citation: Tewari SG, Bell JP, Budgen N, Platz S, Gibbs M, Newham P and Kimko H (2023) Pressurized metered-dose inhalers using next-generation propellant HFO-1234ze(E) deposit negligible amounts of trifluoracetic acid in the environment. Front. Environ. Sci. 11:1297920. doi: 10.3389/fenvs.2023.1297920
Received: 20 September 2023; Accepted: 16 November 2023;
Published: 30 November 2023.
Edited by:
Xiaoxiang Wang, Shenzhen Polytechnic, ChinaReviewed by:
Keith Solomon, University of Guelph, CanadaBora Sul, Intelligent Fusion Technology, United States
Copyright © 2023 Tewari, Bell, Budgen, Platz, Gibbs, Newham and Kimko. This is an open-access article distributed under the terms of the Creative Commons Attribution License (CC BY). The use, distribution or reproduction in other forums is permitted, provided the original author(s) and the copyright owner(s) are credited and that the original publication in this journal is cited, in accordance with accepted academic practice. No use, distribution or reproduction is permitted which does not comply with these terms.
*Correspondence: Shivendra G. Tewari, c2hpdmVuZHJhLnRld2FyaUBhc3RyYXplbmVjYS5jb20=