- 1WasserCluster Lunz—Biological Station, Lunz am See, Austria
- 2Institute of Hydrobiology and Aquatic Ecosystem Management, University of Natural Resources and Life Sciences, Vienna, Austria
- 3National Institute of Biology, Ljubljana, Slovenia
- 4Department of Functional and Evolutionary Ecology, University of Vienna, Vienna, Austria
- 5Institute of Soil Research (IBF), Department of Forest- and Soil Sciences, University of Natural Resources and Life Sciences, Vienna, Austria
Introduction: Woodchips as a source of particulate organic carbon (POC) are proposed as a nature-based solution to enhance nutrient uptake and retention in agricultural streams. However, the effective implementation of woodchips for nutrient removal in streams requires an advanced understanding of their potential and limits, considering their performance under various environmental conditions. This study tested the efficiency of woodchips on the uptake of soluble reactive phosphorus (SRP) and ammonium (N-NH4) across different experimental scales and complexity. We investigated whether the presence of woodchips can increase SRP and N-NH4 uptake in laboratory flumes under controlled conditions, outdoor flumes under semi-controlled conditions, and agricultural streams. Additionally, we examined how the effects of woodchips will change over time via a 6-week incubation in the outdoor flumes.
Methods: The woodchips were pre-colonized for four weeks to allow the growth of biofilms. We performed short-term nutrient additions without (control) and with (treatment) woodchips in all three experimental setups. Uptake parameters were determined via concentration changes over time in the laboratory flumes and concentration changes over travel distance in the outdoor flumes and the stream channels. The effects of woodchips on SRP and N-NH4 uptake rates were analyzed using an effect size model.
Results: We found positive effects of woodchips on nutrient uptake only in the laboratory flumes but no or even negative effects in the outdoor flumes and the agricultural streams. Over the 6-week incubation in the outdoor flumes, we did not observe significant changes in the effects of woodchips on nutrient uptake.
Discussion: These findings highlight that considering experimental scales and influencing environmental conditions is crucial when testing the application of woodchips as nature-based solutions to mitigate nutrient loads in agricultural streams.
1 Introduction
While agricultural streams receive large inputs of reactive phosphorus (P) and nitrogen (N) from fertilizers and runoff, they are often limited in organic carbon (OC), especially when they have been deprived of riparian forests (Akbari et al., 2023; Ezzati et al., 2020). This results in OC:N:P ratios below microbial demand, leading to stoichiometrically constrained heterotrophic nutrient uptake (Stutter et al., 2018; O'Brien et al., 2017). The in-stream addition of natural refractory particulate organic carbon could serve as a nature-based solution to improve OC:N:P ratios until riparian forests have been restored or when replantation is impossible (Burbery and Abraham, 2022). Increasing the supply of particulate OC via, e.g., the addition of woodchips in stream channels can stimulate microbially mediated processes, such as denitrification, thereby reducing nitrate transport downstream (McGuire et al., 2023; Goeller et al., 2019; Christianson and Schipper, 2016). Woodchips also provide surfaces for microbial colonization and adsorption, thus potentially stimulating abiotic and biotic P uptake (Duggan DiDominic et al., 2024; McGuire et al., 2023).
Denitrifying woodchip-bioreactors have long been known as a nature-based solution to mitigate nitrate pollution in subsurface waters, with rising interest in their implementation during the last decades (Duggan DiDominic et al., 2024; Fan et al., 2023; Sanchez Bustamante-Bailon et al., 2022; Christianson et al., 2021; Goeller et al., 2019; Husk et al., 2018). Originally designed to enhance denitrification under anoxic conditions (McGuire et al., 2023; Fan et al., 2023; Mardani et al., 2020), woodchip bioreactors can also stimulate heterotrophic nutrient assimilation in the presence of oxygen by improving the OC:N:P ratios (Christianson et al., 2021). Furthermore, woodchips can be a nutrient adsorbent, especially for soluble reactive phosphorus (SRP) (Husk et al., 2018; Bock et al., 2018). However, only a few comprehensive studies have dealt with the potential stimulation of phosphorus (P) uptake by woodchips so far, with the main focus on subsurface waters (Perera et al., 2024; Sanchez Bustamante-Bailon et al., 2022; Povilaitis et al., 2020). There, the average effective SRP removal by woodchip bioreactors in both lab and field experiments has been reported to range between 10% (Fan et al., 2022; Von et al., 2018; Goodwin et al., 2015; Warneke et al., 2011; Zoski et al., 2013) and 90% (Sanchez Bustamante-Bailon et al., 2022; Rivas et al., 2019; Dougherty, 2018; Husk et al., 2018), highlighting the high variability across different experimental conditions.
In contrast to subsurface systems, streams are exposed to various environmental conditions, which are highly dynamic. The effective implementation of woodchips-based solutions for nutrient removal in surface waters requires a comprehensive understanding of the interactions with various environmental factors, showing the potential but also the limitations of this nature-based solution (Fan et al., 2022; McGuire et al., 2023; Schaefer et al., 2022; Sanchez Bustamante-Bailon et al., 2022; Soupir et al., 2018; Bock et al., 2018; Pluer et al., 2016). Performing experiments in a controlled laboratory setting facilitates the investigation of the removal efficiency of the measure and the underlying biogeochemical processes in a specific environment, focusing on only a few factors at a time. It is equally crucial to understand the performance, potential, and constraints of woodchip-based solutions under varying environmental conditions with multiple interacting factors. Field experiments are essential for this understanding and ensure that the application of this measure is aligned with the complexities of stream ecosystems (McGuire et al., 2023; Schaefer et al., 2022; Sanchez Bustamante-Bailon et al., 2022; Soupir et al., 2018; Bock et al., 2018; Pluer et al., 2016). However, some studies have reported discrepancies in results between laboratory experiments and field studies, indicating that nutrient removal rates can be 2.5 times higher on a laboratory scale than at the field scale (Fan et al., 2022; Ferreira et al., 2015). Fan et al. (2023) conducted a meta-analysis on woodchip bioreactors and highlighted that field-scale bioreactor design should not depend only on data from short-term laboratory studies. The interaction of environmental factors can add a level of complexity that is challenging to replicate in the lab (Fan et al., 2022; Ferreira et al., 2015). Additionally, uncontrollable factors may arise in the field that were not accounted for in laboratory experiments, potentially resulting in unexpected outcomes (Fan et al., 2023). It is vital to bridge the gap between laboratory studies and real-world conditions to ensure that findings from the lab are reliably applicable in an implementation context.
In this study, we aimed to analyze the efficiency of woodchips as OC source on nutrient uptake across different spatial and experimental scales and complexity. The experimental scales included fully controlled laboratory experiments, semi-controlled experiments in outdoor flumes, and experiments under field conditions. Specifically, we investigated whether the presence of woodchips can increase the SRP and N-NH4 uptake in laboratory flumes under controlled conditions, outdoor flumes under semi-controlled conditions, and agricultural streams. We expected that woodchips will enhance nutrient uptake in all settings, with the effects being strongest in the laboratory flumes and weakest in the streams.
Woodchip bioreactors have shown a gradual decline in performance during the initial years of implementation in subsurface waters (Fan et al., 2022). In an early stage, large amounts of bioavailable dissolved organic carbon (DOC) may be released, which has a high potential to stimulate the microbial uptake of nutrients in streams (McGuire et al., 2021; Aalto et al., 2020). However, the positive effects may be outbalanced by a concurrent initial release of reactive nutrients in the form of SRP, N-NO3, and N-NH4 from the woodchips (Ryu et al., 2023). With aging, the OC will undergo microbial mineralization, resulting in the accumulation of more refractory OC with potentially lower effects on nutrient uptake (Fork et al., 2020). As aging may be more crucial for the performance of woodchips in surface waters due to the faster microbial degradation, we decided to additionally investigate the effects of woodchips aging on their nutrient removal efficiency. We chose the outdoor flumes as the best setting for the aging experiment as they provided both a safe environment and semi-natural conditions for the long-term exposure of the woodchips. We expected that nutrient uptake will follow a hump shaped curve with an initial increase during early colonization (due to the release of bioavailable DOC) followed by a continuous decrease with ongoing aging due to the increased accumulation of refractory OC.
2 Materials and methods
2.1 Preparation of woodchip bags
We performed short-term nutrient additions with and without woodchips in small-scale laboratory flumes, medium-scale outdoor flumes, and agricultural headwater streams in Austria (Figure 1). We enclosed the woodchips in mesh bags designed to prevent feeding by macroinvertebrates but allow water to pass through. The bags consisted of commercially available woodchips with a grain size ranging from 5 to 10 mm. The woodchips were an untreated mixture of spruce (Picea sp) and fir (Abies sp), with a maximum water content of 11%. In the laboratory flumes, 10 g of pre-colonized woodchips were filled into flat plastic mesh bags measuring 8 × 9 cm and with a mesh size of 5 × 5 mm. For the outdoor flumes and the agricultural streams, larger mesh bags sized 15 × 25 cm were used and each contained 70 g of woodchips. The laboratory flumes were used to assess the potential leaching effect of fresh woodchips prior to their usage for the different experimental scales. The aging experiments in the outdoor flumes started with fresh, i.e., uncolonized, woodchip bags, with analyses of their performance conducted at the start and after 2, 4, and 6 weeks of colonization. For the experiments in the laboratory flumes and in the streams, colonized woodchip bags were used, which had been incubated for 4 weeks in “Oberer Seebach”, an almost pristine headwater stream directly adjacent to the outdoor flumes. In this environment, biofilms typically attain a stable growth stage within 3–4 weeks (Singer et al., 2010). We refer to them as “fully colonized woodchip bags” throughout the manuscript.
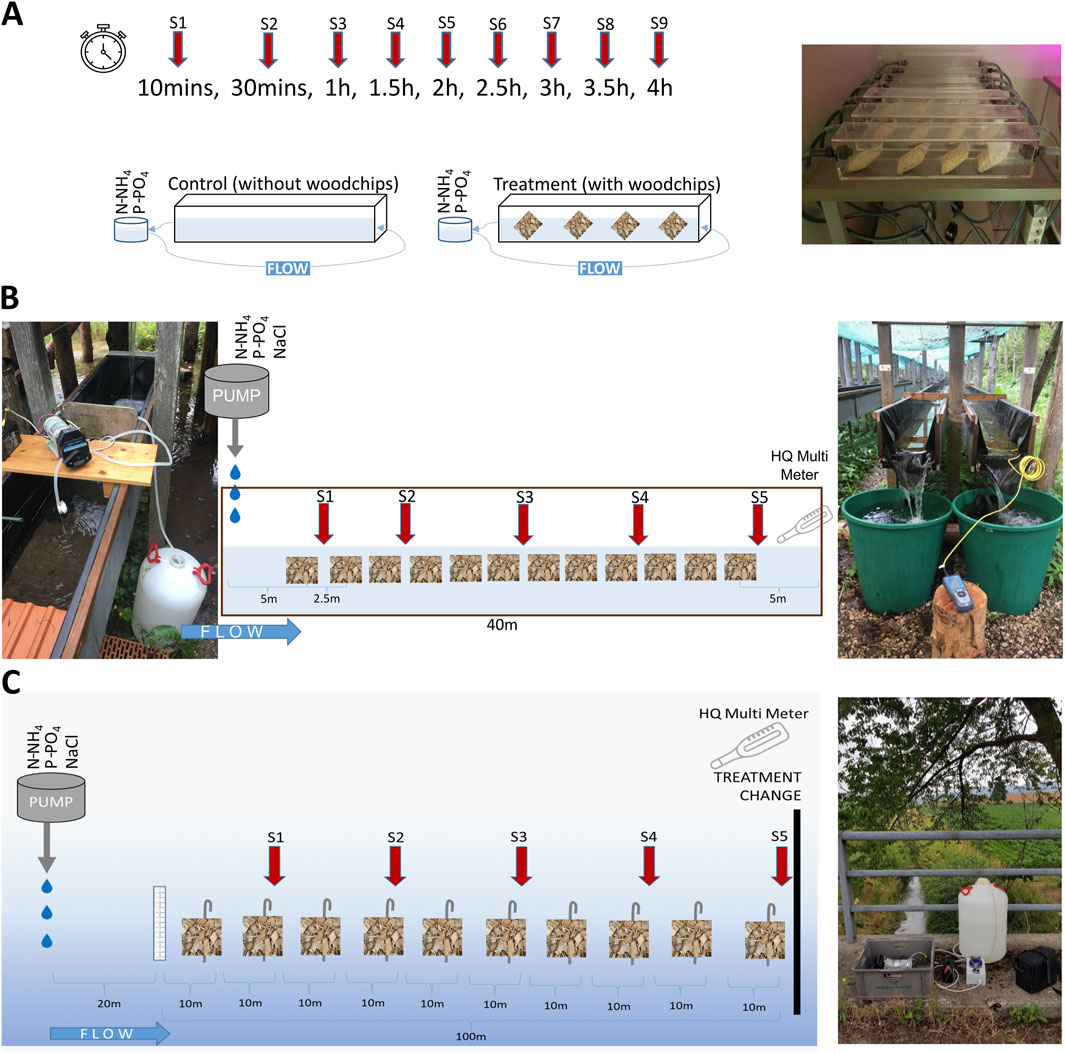
Figure 1. Experimental setups in the laboratory flumes (A) running in circulation flow mode using aquarium pumps, outdoor flumes (B) and agricultural streams (C) with sketches of woodchip bags, sampling points, Hach Lange HQ conductivity meter and the peristaltic pump (at the top of the flume and a selected stream reach) releasing the nutrient solution at a constant rate. The figures are not drawn to scale.
2.2 Experimental set-up
2.2.1 Laboratory flumes
The laboratory flumes were made of acrylic glass with 55 cm length, 9 cm width, and 10.5 cm depth. For the experiments, a total of 12 flumes were operated in circulation flow mode with a flow velocity of approximately 6 cm s−1 using aquarium pumps (EDEN, 126, 800 Lh−1; for details, see Akbari et al., 2023). The nutrient uptake experiments were performed at 20°C in a climate chamber in summer 2021.Four flumes were run without woodchip bags as controls and six other flumes were run with four fully colonized woodchip bags each, which were placed in 0.14 m intervals within the flumes (Figure 1). In the laboratory flumes, a solution of SRP and N-NH4 was added at the start of the experiments and nutrient uptake was calculated via the decrease of nutrient concentrations over time. The nutrient solution consisted of 5 L of filtered well-water (Acrodisc Syringe Filters, 0.22 μm), resembling the ionic composition of streams in this area (Table 1), with additions of SRP (as NaH2PO4) and N-NH4 (as NH4Cl) to achieve end concentrations of 200 μg L−1 each, typical for agriculturally influenced streams in Lower Austria. Water samples were collected in triplicates starting from 10 min after the addition (start point t0), and then every 30 min over 4 h, resulting in nine samplings in total. Water samples were filtered immediately on-site with pre-combusted GF/F Filters (Whatman, 0.75 µm) and stored in pre-combusted glass vials at 4°C in the dark over 24 h. Nutrient concentrations (SRP, N-NO3, N-NH4, and N-NO2) were determined with a continuous flow analyser (CFA, Systema Analytical Technology) and DOC concentrations were analysed with a Shimadzu TOC-Analyser (Shimadzu TOC-LCSH FA; Japan). Water samples from the outdoor flumes and the streams were treated in the same way. Additionally, approximately 3 g of woodchips were randomly removed from woodchip bags after the nutrient additions and analyzed for chlorophyll-a (Chl-a) concentrations, bacterial abundances, and total phosphorus (TP). The same analyses were performed on woodchip bags from both the outdoor flumes and the streams.
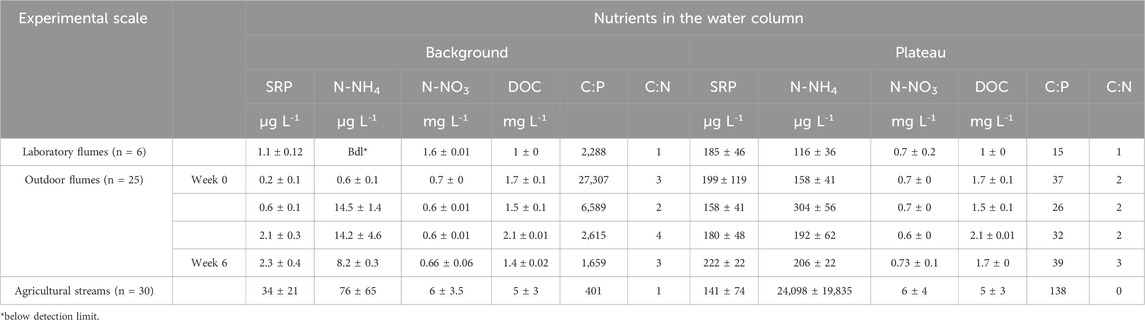
Table 1. Average nutrient and DOC concentrations in the woodchips treatments of the laboratory flumes, the outdoor flumes, and the agricultural streams during background and plateau conditions, showing means ± standard deviation.
2.2.2 Outdoor flumes
The outdoor flumes consisted of six artificial channels (40 m length, 0.4 m width and height; Figure 1). Five flumes were used for the nutrient addition treatments, while the sixth flume was used for the colonization and aging of the woodchip bags. All flumes were filled with a layer of gravel on the bottom as a substrate for colonization by benthic microbial biofilms. Pre-combusted ceramic tiles (5 × 5 cm; 4 h at 450°C) were additionally placed on the gravel sediments of each flume to facilitate standardized sampling of the biofilms. The flumes were continuously fed in a flow-through mode by stream water from “Oberer Seebach” with 0.62 L s−1 mean discharge. At the start of the experiment, the average background concentrations were 0.2 μg L−1 for SRP, 0.6 μg L−1 for N-NH4, 0.7 mg L−1 for N-NO3 and 1.7 mg L−1 for DOC (Table 1). During the experiments, light intensity, temperature and dissolved oxygen concentrations were measured continuously with HOBO Pendant Temperature/Light 64K Data loggers and HOBO Dissolved Oxygen Data loggers (Onset Computer Corporation) near the inlet and the outlet of each flume, respectively. The water temperature in the outdoor flumes ranged from 9.5°C to 11.8°C. Dissolved oxygen concentrations ranged from 10.9 to 12.1 mg L−1 in the outdoor flumes.
The colonization phase for the biofilms on the sediments and tiles lasted over 5 weeks from May to June 2022. For the uptake experiments, we conducted short-term nutrient plateau additions to calculate nutrient uptake via the decrease in concentrations along the flumes (Stream Solute Workshop, 1990; Weigelhofer et al., 2018). In total, we conducted four series of two consecutive short-term nutrient additions every second week over an entire period of 6 weeks. We considered the age of the woodchip bags as “Fresh” for week 0 (start), “Early colonized” after 2 weeks, “Fully colonized” after 4 weeks (Singer et al., 2010) and “Old age” after 6 weeks, respectively. Each series consisted of one nutrient addition experiment without woodchip bags (control) in each of the five treatment flumes (5 replicates), followed by an addition experiment with woodchip bags (treatment) on the next day. For the treatment on the second day, a total of 12 woodchip bags were placed 2.5 m apart within each flume (Figure 1). During each addition (control without woodchip bags and treatment with woodchip bags), a nutrient solution containing SRP (as NaH2PO4) and N-NH4 (as NH4Cl) with a final concentration of 200 μg L−1 each was injected at a constant rate via a peristaltic pump at the head of each flume for approximately 1 h. Sodium chloride (NaCl) was used as conservative tracer to determine plateau conditions. Electrical conductivity was recorded at the end of each flume at 10 s intervals using a Hach Lange HQ40d conductivity meter. About 10 min after reaching plateau conditions, water was sampled every 6 m between 5 and 30 m downstream of the injection point. Prior to the additions, water samples were collected at the inflow and outflow of each flume to determine nutrient and DOC background concentrations. After each addition series, five tiles were randomly selected from the upper and the lower end of each flume, respectively, to determine Chl-a concentrations, bacterial abundances, and TP (Section 2.3). Additionally, approximately 3 g of woodchips were removed randomly from five woodchip bags and analyzed for Chl-a concentrations, bacterial abundances, TP, respiration rates and alkaline phosphatase activity (Section 2.3). Finally, the woodchip bags were returned to the 6th flume to continue colonization.
2.2.3 Agricultural streams
The studied headwater streams Schildbach (Si), Linsberggraben (Li), and Schlattenbach (SI) are situated in an area of Lower Austria which is dominated by agriculture, approximately 30 km north of the location of the outdoor flumes. All streams showed a straight and homogenous stream course with 20–30 cm water depth, 70–130 cm stream width, and 7–60 L s−1 discharge during the experimental period. The average background concentrations were 34 μg L−1 for SRP, 76 μg L−1 for N-NH4, 6 mg L−1 for N-NO3 and 5 mg L−1 for DOC (Table 1). The water temperatures in the agricultural streams showed a broader range, amounting from 15.6°C to 18.5°C. Dissolved oxygen concentrations ranged from 7.4 to 9.3 mg L−1 in the agricultural streams. All streams showed straightened homogeneous channels without riparian forests and silty to sandy sediments. Two 100 m homogenous reaches were selected in each stream for the experiments, of which one was used for the WBR treatment and the other as control without woodchip bags. The sequence of control and treatment reach was chosen randomly for each experiment to avoid a spatial bias (Figure 1).
Similar to the outdoor flumes, we performed short-term nutrient plateau additions in the streams in July 2021 over a period of 6 days under stable, sunny weather conditions. The experiments were carried out twice within two non-consecutive days in each of the three streams, resulting in six replicates (one stream per day in the sequence Si, Li, Sl, Si, Li, Sl). On the day before the nutrient additions, we installed ten fully colonized woodchip bags every 10 m apart in the treatment reach. Additionally, we placed a metal grid 20 m before the first sampling point to ensure mixing. The addition experiments followed the same procedure as for the outdoor flumes. The injection point was located 30 m upstream of the first sampling point, and a nutrient solution containing SRP (as NaH2PO4) and N-NH4 (as NH4Cl) with final concentrations of 200 μg L−1 and 25 mg L−1,respectively, was injected at a constant rate via a peristaltic pump. We used higher N-NH4 concentrations because we expected higher background concentrations in the streams. Water samples were taken every 20 m in both the control and the treatment reach directly before the addition and during plateau conditions (3 replicates). The water samples were processed in the same way as in all other experiments.
2.3 Biofilm analyses
Biofilm samples from woodchips from all experiments were processed as follows. Chl-a contents in woodchips were determined after extraction in 90% cold acetone via high-pressure liquid chromatography (HPLC; Hitachi Elite LaChrom, Hitachi High Technologies America, San Jose, CA, United States). For bacterial abundances, 1.3 g of woodchips were suspended in 3 mL GF/F filtered and autoclaved stream water (30 min at 121°C and Hirayama HG-50, California, USA) from the respective experiments and fixed with 37% formaldehyde at a final concentration of 2.5%. Bacterial abundances were determined after extraction with Tween 80 (10%) and staining with Sybr Green II on a flow cytometer (CytoFLEX, Beckman Coulter GmbH, Krefeld, Germany; Weigelhofer et al., 2020). The TP concentrations in woodchips samples was determined after digestion with 1 M sulfuric acid in the CEM-microwave (CEMMarsXpress; Malá and Lagová, 2014) and then measured using a continuous flow analyser (CFA, Alliance Instruments GmbH, Salzburg, Austria). Woodchips samples were dried at 75°C overnight to determine the dry mass (DW).
To get information about changing biofilm activities on the woodchips during aging, we additionally analysed biofilm respiration and alkaline phosphatase activity in the woodchip bags from the outdoor flumes. For microbial respiration, 1 g of woodchips were placed in 18 mL air-tight glass vials equipped with oxygen-sensitive optical sensor spots (Fibox 3 optode system, PreSens GmbH, Regensburg, Germany). Each vial was filled carefully with respective stream water, closed without air-bubbles, and incubated in the dark. The decrease in dissolved oxygen concentrations was measured at the beginning and after 4, 8, 12, 24, and 30 h. The respiration rates were calculated from the decrease in oxygen over time, corrected for the water volume and woodchips dry weight. The alkaline phosphatase activity was measured as enzymatic activity by adding substrate proxies and incubating the woodchips samples for 1 h in the dark before a buffer was inserted to stop the reaction. Then, fluorescence was determined at 365 nm excitation and 450 nm emission using a 96-well plate reader (VARIOSCAN FLASH, Thermo Fisher Scientific; Marx et al., 2001; Bell et al., 2013).
2.4 Calculation of uptake parameters
For the laboratory flumes, SRP and N-NH4 concentrations remaining in solution were plotted against time and a linear regression line was fitted using the lm function in R. Nutrient uptake rates (µg L−1 h −1) were determined by calculating the slope of the regression line. Positive numbers indicate an uptake and negative numbers a release of the respective nutrients. For the plateau additions, nutrient uptake lengths (m) were calculated via the longitudinal decline of the added nutrients in the outdoor flumes and the agricultural streams (Stream Solute Workshop, 1990; Weigelhofer et al., 2018). For this purpose, nutrient concentrations during plateau conditions were corrected for ambient concentrations. We calculated uptake rates (U) from the uptake lengths (Sw), water velocity, water depth, and nutrient concentrations as described in the Stream solute Workshop (1990).
2.5 Data analysis
We calculated the effect sizes to compare the effects of the woodchip bags on the nutrient uptake rates to a control without woodchip bags among all three experiments (Supplementary Table S1). For this purpose, we compared the experiments in the laboratory flumes and in the streams with the 4-weeks sampling of the outdoor flumes. Responses of SRP and N-NH4 uptake rates to the presence of woodchip bags were calculated for all three experiments as the log transformed response ratio (logR), given by the logarithm of the ratio of the mean of uptake/release rates after nutrient addition with woodchip bags to controls without woodchip bags. For handling the negative values (release rates), we added a constant value to the data prior to applying the log transform. A logR = 0 indicates no response. A logR <0 and logR >0 indicate decreased and increased uptake rates with woodchip bags versus controls, respectively (Hedges et al., 1999). The variance associated with logR was calculated using standard deviation and sample size. Effect sizes and variances were calculated using the “escal” function of the “metafor” package (Viechtbauer, 2010) in R (R Core Team, 2020). We quantified the effects of woodchip bags on nutrient uptake by fitting random-effects models using the “rma.uni” function of the “metafor” package for experimental scale (Viechtbauer, 2010). For the plateau additions, the average and minimum velocity were calculated from reach length divided by the time at which half of the maximum conductivity and the plateau were reached, respectively (Gordon et al., 2008).
The effects of aging of woodchips (fresh, early-, fully-colonized, old age) on the nutrient uptake (controls and addition) were analysed by two-way ANOVA followed by Tukey’s Honestly Significant Difference (HSD) post hoc tests after checking that equal sample size, the assumptions of normal distribution (Shapiro–Wilk test) and/or homogeneity of variance (Bartlett’s test) were met. Results at p < 0.05 were considered significant. All statistical analyses were performed using statistical software R v4.0.3.
3 Results
3.1 Effects of woodchips on nutrient uptake at different experimental scales
We found positive effects of fully colonized woodchips on SRP and N-NH4 uptake only in the laboratory flumes but no or even negative effects in the outdoor flumes and the agricultural streams (Figure 2; Supplementary Table S1). The global model across all experiments was non-significant (p > 0.05).
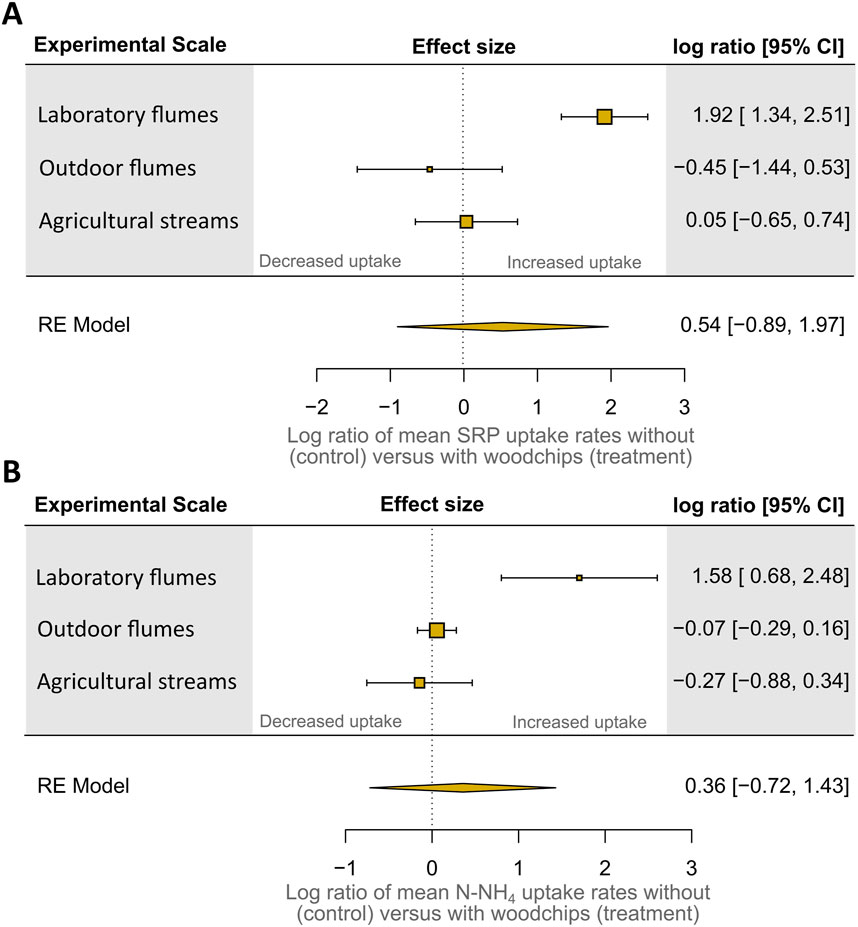
Figure 2. Responses of soluble reactive phosphorus (SRP) (A) and ammonium (N-NH4) (B) uptake rates to the presence of fully colonized (4 weeks) woodchips at different experimental scales. Responses were calculated as the log transformed response ratio (logR), given by the logarithm of the ratio of mean uptake rates in the presence of woodchips (treatment) to uptake rates in controls without woodchips. A logR = 0 indicates no response. A logR <0 and logR >0 indicate decreased and increased uptake rates with woodchips versus controls, respectively (Hedges et al., 1999).
In the laboratory flumes, controls showed net average SRP and N-NH4 uptake rates of 2.6 ± 1.7 and 1.4 ± 6.3 μg L−1 h−1. In the presence of woodchips, we measured mean net SRP and N-NH4 uptake rates of 24 ± 4.6 and 28.5 ± 6.8 μg L−1 h−1 in the laboratory flumes. In the outdoor flumes, controls showed net average SRP and N-NH4 uptake rates of 1.72 ± 2.12 and 1.80 ± 1.83 mg m−2 s−1, respectively. Interestingly, the addition of fully colonized woodchips resulted in slightly, albeit not significantly lower mean SRP and N-NH4 uptake rates of about 0.73 ± 1.40 and 1.33 ± 0.44 mg m−2 s−1. In the agricultural headwater streams, controls showed slight net SRP uptake of 1.16 ± 1.47 mg m−2 s−1 on average, while net N-NH4 uptake rates amounted to 6.10 ± 6.84 mg m−2 s−1. The addition of fully colonized woodchips had no effect on SRP uptake (mean 1.27 ± 1.23 mg m−2 s−1). Interestingly, net N-NH4 uptake rates decreased to 3.36 ± 4.36 mg m−2 s−1 after the addition of woodchips (p > 0.05). In general, we observed a high variability among the replicates of both the controls and treatments across all three different experimental scales.
The water chemistry data showed average background concentrations of SRP and N-NH4 in the laboratory flumes of 1.1 and <0.2 μg L−1, respectively (Table 1). Under plateau condition, these concentrations increased to 185 and 116 μg L−1, respectively. In the outdoor flumes, the average background concentrations of SRP and N-NH4 increased from 0.2 to 0.6 μg L−1 to 2.3 and 8.2 μg L−1, respectively, over the 6 weeks of the experiment. Plateau concentrations of SRP and N-NH4 ranged between 199 and 116 μg L−1 in week 0 and 222 and 206 μg L−1 in week 6. Thus, background and plateau conditions were comparable between lab flumes and outdoor flumes, with an average increase of approximately 1:100. However, the agricultural streams showed much higher average nutrient background concentrations of about 33.8 μg L−1 for SRP and 75.9 μg L−1 for N-NH4 than the flumes. Plateau concentrations were 141 and 24,098 μg L−1 for SRP and N-NH4, respectively (average increase 1:4 and 1:300).
In the laboratory flumes, the average background N-NO3 and DOC concentrations were 1.6 and 1 mg L−1, respectively. Following nutrient additions, the N-NO3 concentrations decreased to 0.7 mg L−1. In the outdoor flumes, the initial concentrations for N-NO3 and DOC were 0.7 and 1.7 mg L−1, respectively, and they decreased to 0.66 and 1.4 mg L−1 for N-NO3 and DOC, respectively, during plateau conditions. In the agricultural streams, the N-NO3 and DOC concentrations were 6 mg L−1 and 5 mg L−1, respectively, and the concentration of N-NO3 and DOC remained at the same level during plateau conditions (Table 1).
Fully-colonized (4-weeks) biofilms on the woodchips for the laboratory flumes and the agricultural streams showed 10 times higher Chl-a concentrations (12 ± 6 µg gDW−1) than those for the outdoor flumes (1.27 ± 2.7 µg gDW−1; p < 0.05, n = 25; Figure 3). In contrast, woodchips biofilms in the outdoor flumes showed approximately 20 times higher bacterial abundances (215 ± 93 cells 106 g DW−1 than those in the laboratory flumes and the agricultural streams (12.5 ± 6 cells 106 g DW−1; p < 0.05, n = 25). The TP concentrations of the fully colonized woodchips did not differ between experiments.
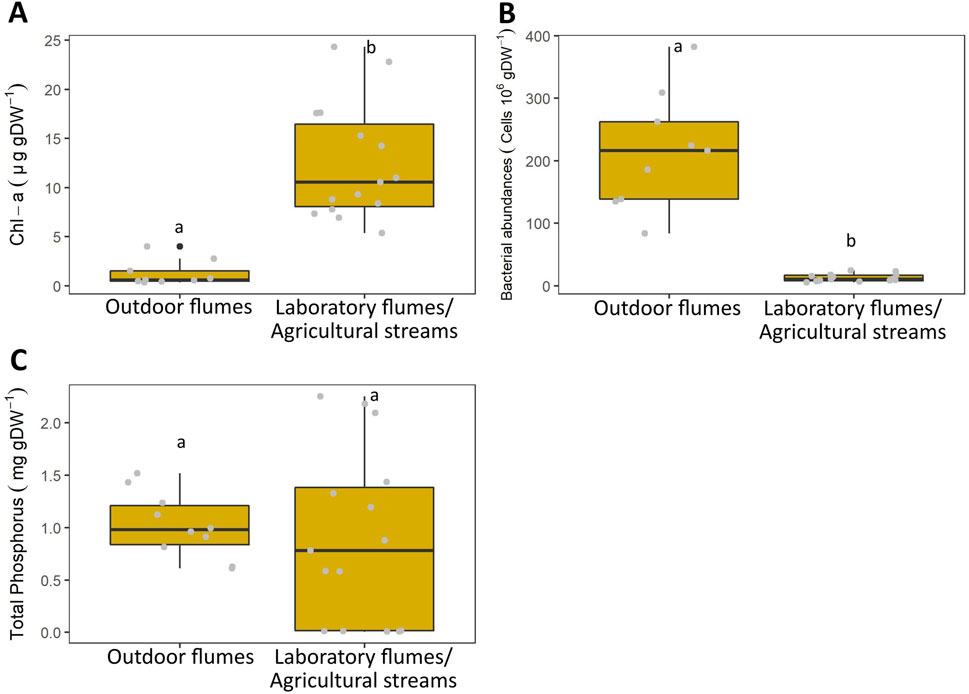
Figure 3. Chlorophyll-a (Chl-a) contents (A), bacterial abundances (B) and total phosphorus (TP) concentrations (C) in 4 weeks colonized woodchips at different experimental scales.
3.2 Effects of aging of woodchips on nutrient uptake rates
In general, nutrient uptake rather than release was observed in both treatment and control outdoor flumes during the addition experiments. The aging of the woodchips over 6 weeks did not significantly change this nutrient uptake (p > 0.05; Figure 4), although we observed a small increase in SRP uptake rates in the presence of woodchips over time from week 2 (1.2 times) to week 6 (2 times). For N-NH4, woodchips increased uptake rates only in week 2 (2 times) and a reduction in uptake was observed thereafter.
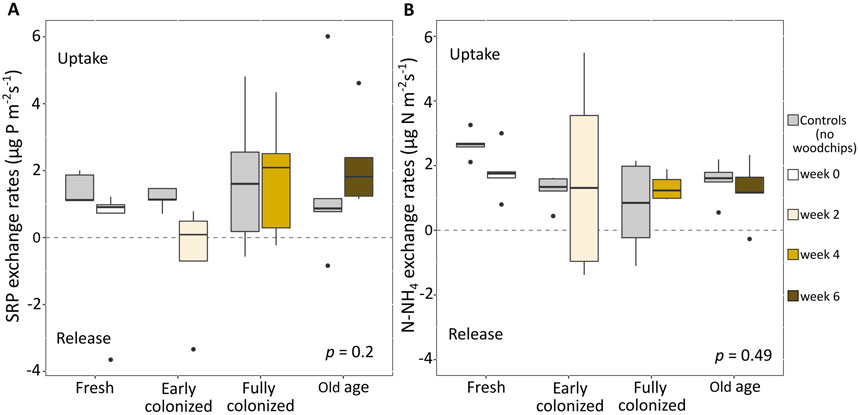
Figure 4. Aging effects of woodchips on soluble reactive phosphorus (SRP) (A) and ammonium (N-NH4) (B) rates in the outdoor flumes (ANOVA, p > 0.05, n = 40). Positive and negative values show uptake and release rates, respectively. The age of woodchips varied from 0 (fresh), 2 (early colonized), 4 (fully colonized), to 6 (Old age) week(s).
At the start of the experiment (week 0), we observed relatively high SRP and N-NH4 uptake in the controls (i.e., without woodchip bags), averaging 1.4 ± 0.5 and 2.7 ± 0.4 mg m−2 s−1, respectively. When fresh woodchips were added, mean nutrient uptake was reduced to 0.04 ± 2.1 mg m−2 s−1 for SRP and 1.8 ± 0.8 mg m−2 s−1 for N-NH4. One flume even showed net SRP release of −3.65 mg m−2 s−1. After 2 weeks, the controls still showed an average SRP uptake of 1.18 ± 0.3 mg m−2 s−1, while N-NH4 varied between uptake and release among the individual flumes, with an average release rate of −0.5 ± 1.7 mg m−2 s-1. The addition of early colonized woodchips did not affect the SRP uptake (mean rates 1.24 ± 0.5 mg m−2 s−1), while N-NH4 switched to an average uptake of 1.6 ± 2.9 mg m−2 s−1. In week 4, we observed considerable variability in SRP uptake/release patterns among replicates in both the controls and the woodchips treatments. On average, SRP uptake dominated, showing rates of 1.7 ± 2.1 mg m−2 s−1 for the controls and 0.7 ± 1.4 mg m−2 s−1 for the treatments. We observed uptake of N-NH4 in all controls except one (mean 1.8 ± 1.8 mg m−2 s−1) as well as in all WBR treatments (mean 1.3 ± 0.4 mg m−2 s−1). In week 6, mean SRP uptake rates were 1.6 ± 2.6 mg m−2 s−1 and 1.5 ± 0.6 mg m−2 s−1 in the controls and the woodchips treatments, respectively. The WBR treatments showed lower uptake of N-NH4, amounting to 1.2 ± 1 mg m−2 s−1, than the controls with an average of 2.24 ± 1.4 mg m−2 s−1.
The largest differences were observed between fresh woodchips (week 0) and colonized ones (week 2–6; Figure 5). On average, fresh woodchips showed ∼5 times lower respiration rates (4.4 ± 1.6 µg O2 g DW−1 h−1), ∼ 6–40 times lower phosphatase activities (10.3 ± 1 μmol g−1 h−1), ∼3 times lower bacterial abundances (82.2 ± 5.2 cells 106 g DW−1), and ∼100–190 times lower TP levels (0.01 ± 0 mg g DW-1) than colonized woodchips that are older than 2 weeks (p < 0.001, n = 40). The mean Chl-a concentrations increased from below detection limit in fresh woodchips to 0.9–1.3 μg g DW−1 in colonized woodchips that are older than 2 weeks.
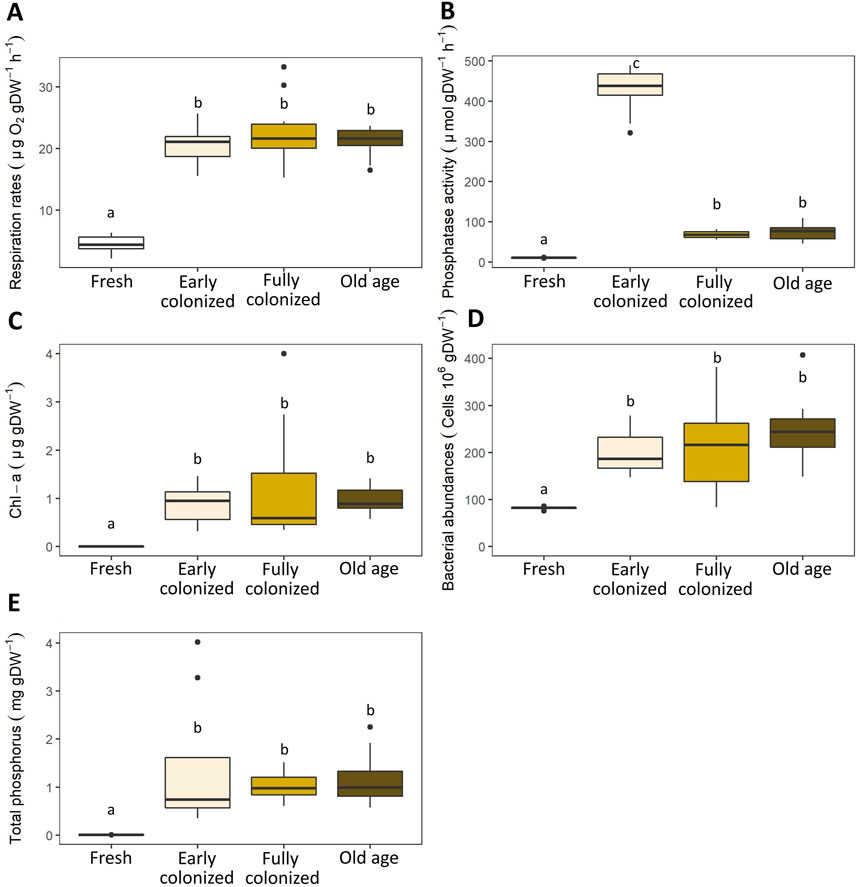
Figure 5. Respiration rates (A), phosphatase activity (B), chlorophyll-a (Chl-a) contents (C), bacterial abundances (D) and total phosphorus (TP) concentrations (E) in woodchips at different temporal scales. The age of woodchips ranged from 0, 2, 4, to 6 weeks(s) for Fresh, Early colonized, Fully colonized and Old age, respectively.
4 Discussion
Our study investigated the potential of woodchips in stimulating nutrient uptake in nutrient loaded surface waters by comparing uptake experiments at three different experimental scales: in laboratory flumes, outdoor flumes, and agricultural streams. As expected, we found strong stimulation of nutrient uptake in the presence of woodchips in our laboratory flumes experiments. We observed a notable 90% removal of SRP and 77% removal of N-NH4, indicating the efficacy of woodchips on nutrient retention at the laboratory scale. Over the past 2 decades, numerous laboratory and field studies investigated the nutrient mitigation potential of woodchips under mainly anoxic conditions, focusing primarily on nitrate removal via denitrification but also including effects on other N species and SRP (Christianson et al., 2021; Kouanda and Hua, 2021; Povilaitis et al., 2020; Li et al., 2018; Hua et al., 2016). While laboratory-scale studies with woodchips-only bioreactor columns reported low average SRP removal rates of <10% (Goodwin et al., 2015; Zoski et al., 2013), field studies on subsurface woodchip bioreactors observed high rates, ranging from 60% to 100% (Perera et al., 2024; Husk et al., 2018; Dougherty, 2018; Rivas et al., 2019), depending on various environmental conditions such as hydrology. Perera et al. (2024) and Husk et al. (2018), for example, observed 75%–100% and 60% SRP removal in their woodchips-only treatment during the first study year, respectively, which decreased to 3%–67% and 10%–20% during the next 2 years. In contrast, SRP release during the first year turned into an 89% SRP removal during the second year in a study by Rivas et al. (2019) when hydraulic retention times were significantly reduced. However, most of these studies focused on denitrification under anoxic conditions, with P removal as potential positive side-effect. Reported P removal rates of 75% to >99% in such studies refer mostly to P adsorption or Ca/Mg-associated precipitation (Sanchez Bustamante-Bailon et al., 2022; Povilaitis et al., 2020; Husk et al., 2018) and are thus not comparable to our study. To date, only a limited number of studies have investigated oxic nutrient uptake in woodchips, with the majority focusing on the coupling of bioavailable C and N rather than P (McGuire et al., 2023; 2021; Hartfiel et al., 2022; Maxwell et al., 2019; Aalto et al., 2020).
The release of labile OC from woodchips under oxic conditions may boost heterotrophic activity and facilitate in-stream nutrient uptake as demonstrated by McGuire et al. (2021) in a laboratory woodchip bioreactors study. Consistent with our laboratory results, other laboratory studies also show positive effects of bioavailable OC from different sources on microbial nutrient uptake (Akbari et al., 2023; Graeber et al., 2021; Stutter et al., 2020a). Akbari et al. (2023) observed enhanced phosphate uptake by heterotrophic biofilms with increased OC supply in a laboratory experiment using fresh and leached leaves. In another laboratory study using leaf leachate as carbon source, Graeber et al. (2021) found a strong correlation between heterotrophic P uptake and molar DOC:P ratios between 100 and 400. These findings suggest that an active stoichiometric shift in streams can result in coupled cycling of P and OC and enhancing heterotrophic microbial nutrient uptake, thus improving nutrient retention and ecosystem health. However, the complexity of coupling between autotrophs and heterotrophs, as well as their reliance on either the use of externally supplied or internally recycled nutrients, can significantly influence microbial nutrient uptake depending on their dominance within stream biofilms (Akbari et al., 2023; Stutter et al., 2020b; Wagner et al., 2017).
In the agricultural streams, our study found no significant and consistent positive effect of woodchips on nutrient uptake. Although we expected to find lower effects in the field, we did not expect to find none, and this is also contrary to other field studies. For example, O’Brien et al. (2017) observed increased microbial activity and enhanced in-stream nutrient uptake following a 6-week addition of OC via leaf packs in agricultural headwater streams. We assume that the extremely high nutrient background concentrations in the water column of our streams may have saturated the systems (Sunjidmaa et al., 2022; Tank et al., 2017; O’Brien et al., 2007) and that the amount of added woodchips were thus too low to achieve any measurable effects. The chronic exposure to increased nutrient loads can lead to saturation of the microbial community, resulting in decreased uptake efficiency and eventually even high nutrient release from streambed sediments (Sunjidmaa et al., 2022; Bechtold et al., 2003). In our agricultural streams, the woodchip biofilms were dominated by autotrophs, similar to the lab experiment. Thus, one would assume increased SRP uptake, albeit not necessarily stimulated by the increased OC availability (Akbari et al., 2023). However, several experimental studies have shown that especially thick and autotrophs-dominated biofilms may rely more on internal nutrient cycling rather than external nutrient supply (Kamjunke et al., 2015; Brailsford et al., 2019; Graeber et al., 2018; Wagner et al., 2017; Akbari et al., 2023). Consequently, their nutrient uptake is primarily controlled by the availability of dissolved inorganic N and/or P (Jarvie et al., 2018), which may be directly delivered by the woodchips. Another plausible explanation for the lack of consistent responses to nutrient additions in the agricultural streams could be the relatively short duration of the addition experiments (i.e., approximately less than 1 hour), which may not have been long enough to trigger an ecosystem response to the increased OC availability (Griffiths and Johnson, 2018; Rier et al., 2016). As a result, biofilm communities in the systems had insufficient time to adapt to both the increased OC availability and the enhanced nutrient supply. The short-term response of P uptake depends on the uptake kinetics of the existing microbial community and this may temporarily saturate at SRP concentrations above 5 μg L−1 (Mulholland et al., 1990). Conversely, long-term nutrient additions (over days to weeks) have the potential to increase the nutrient uptake by stream biofilms as biomass grows and nutrient demand increases over time (Griffiths and Johnson, 2018; Tank et al., 2017; Mulholland et al., 1990). Prolonged nutrient plateau conditions in the streams may have yielded different results but were not possible due to environmental issues. Future studies should consider the duration of nutrient additions as an important factor in laboratory and field experiments. Finally, the water flowing through the woodchip bags strongly affects their efficiency in adsorbing nutrients (Husk et al., 2018; Soupir et al., 2018) and microbial nutrient uptake. Soupir et al. (2018) observed a significant increase of approximately 36% in SRP removal by woodchips when the hydraulic retention time was 24 h compared to 12 h. Due to the larger dimensions of the streams compared to the laboratory flumes, there was less possibility to force the water flow through the woodchip bags in the first ones. Consequently, this may have led to nutrients increasingly bypassing the woodchip bags.
Contrary to our hypothesis, we observed no significant and consistent positive effect of woodchips on nutrient uptake in the outdoor flumes despite the better control on environmental conditions such as lower background nutrient concentrations and replicability (Menczelesz et al., 2019) than in the streams. This is contrary to other mesocosm studies, where Allen et al. (2019), for example, reported a significant decrease in SRP concentrations in outdoor mesocosms containing leaf bags compared to the controls. One possible explanation for our results could be the comparatively high bacterial abundances on the woodchips, suggesting that P may have been primarily taken up from the organic substrate instead of the water column. Furthermore, it is plausible that the short duration of nutrient addition did not allow enough time for the microbial community to respond adequately to the increased nutrients supply, similar to what was observed in the agricultural streams (Griffiths and Johnson, 2018; Tank et al., 2017; Mulholland et al., 1990). However, net uptake was generally low in the outdoor flumes in both controls and treatments. A meta-analysis by Addy et al. (2016) found that N removal rates were higher with increasing temperatures ranging from 6 to >16.9°C. Low water temperatures of 9.5°C–11.8°C may have reduced microbial nutrient uptake in our outdoor flumes in contrast to the laboratory flumes, where room temperatures of 20°C likely contributed to higher nutrient removal rates due to stimulation of microbial metabolism (Grießmeier et al., 2021; Kouanda and Hua, 2021). However, this explanation is not full in line with what we would have expected as the bacterial colonization phase of the woodchip bags took place until a day before the experiment in the “Oberer Seebach,” which has similarly low water temperatures.
The lack of effects of woodchips on nutrient uptake also resulted in no significant effects of woodchips aging in the outdoor flumes. In the short term (maximum 2 weeks), leaching of nutrients from woodchips dominated the nutrient exchange rates and this could possibly diminish the positive effects of OC supply on nutrient uptake (Akbari et al., 2023). However, the addition of woodchipsto the flumes resulted in a distinct, albeit non-significant, average increase of SRP uptake from week two to week six. Ammonium also showed an average increase in the presence of fully colonized woodchips at week two. The increasing trend in nutrient uptake suggest that the negative effect of leaching is compensated after 2 weeks and indicates a potential positive effect of woodchips on nutrient uptake. This observation is supported by our data on woodchips biofilm properties, which indicate that the most significant differences in biofilm properties were observed between fresh and colonized woodchips. One possible explanation is that the woodchips biofilm has reached maturity, i.e., a stable growth, after 2 weeks, leading to an increase in their nutrient uptake capacity. Previous studies have shown that woodchip bioreactors may need a relatively long operating time to fully develop the capability for P removal. For example, Hua et al. (2016) noted that woodchips bioreactors exhibited an increasing ability to remove SRP within the first week of operation and reached a peak removal efficiency of 75% by the end of the 7th week. This extended time could be attributed to processes such as biofilm penetration and microbial degradation (Hua et al., 2016; Cameron and Schipper, 2010). Aging effects of woodchips on nutrient uptake could be observed in other laboratory studies that lasted for months. Kouanda and Hua (2021), for example, measured higher N removal in up-flow columns filled with fresh woodchips than in reactors filled with 12 months-old woodchips. The stimulation of SRP and N uptake by fresh woodchips can be due to a greater amount of labile OC during the initial stages, which increases the microbial demand for nutrients (Hathaway et al., 2017; Addy et al., 2016; Cameron and Schipper, 2010). As woodchips are gradually decomposed during aging, the OC becomes increasingly recalcitrant and less available over time. This may lead to reductions in the stimulation of microbial nutrient uptake. We decided to observe aging effects only over a relatively short time period of 6 weeks as aerobic decomposition may severely reduce the woodchips biomass, thus changing the experimental conditions. However, future research needs to include longer monitoring of the efficiency of woodchip bags in streams to elucidate the effectiveness of woodchip bags in stimulating nutrient removal over time.
Laboratory experiments are valuable for testing novel questions regarding woodchips efficiency and enhancing our understanding of processes such as microbial dynamics within these nature-based solutions. However, they often face limitations in scale and duration (Cameron and Schipper, 2010). The interaction of biotic and abiotic factors can change with an increase in complexity of the experimental system (Cooper et al., 1998). Using outdoor flumes or natural streams adds a level of complexity that is challenging to replicate in single-factor laboratory experiments (Ferreira et al., 2015). Results from laboratory experiments under controlled conditions may not be easily transferable to the field due to multiple influencing factors occurring in real-world environments (Schaefer et al., 2021). In our study, the variability observed in the field highlights the challenges of replicating controlled laboratory settings and the importance of careful interpretation of laboratory results. Additionally, our results suggest the need for field-scale verification involving multiple influencing factors. As we transition from lab-scale to field studies, our control over influencing factors such as water chemistry and temperature decreases (Schaefer et al., 2021; Addy et al., 2016; Pluer et al., 2016; Ferreira et al., 2015). Field-scale issues such as longevity and management may not be addressed through laboratory experiments alone, thus limiting the applicability of woodchips in field settings (Christianson and Schipper, 2016; Cameron and Schipper, 2010). Furthermore, there is a lack of research pairing laboratory experiments on woodchips with real-world field studies, limiting direct comparisons of the effectiveness of these nature-based solutions. Bridging the gap between laboratory studies and real-world field conditions is essential to ensure the applicability and reliability of findings in practical contexts, enabling researchers to examine and understand ecosystem processes and patterns at multiple scales. By comparing results from multi-scale experiments, researchers can assess their consistency and identify disparities, gaining insights into the scaling effects of the process under investigation.
5 Conclusion
In conclusion, our study has demonstrated the effectiveness of woodchips in the laboratory setting for enhancing nutrient uptake, particularly P, thereby supporting the general assumptions regarding the coupling of OC availability and P uptake. However, it also demonstrated that this coupling maybe covered by other influencing environmental factors, such as interactions between heterotrophs and autotrophs. Therefore, to gain insight to applicability of woodchip bags as a nature-based solution, it is essential to test their efficiency in both laboratory (potential efficiency) and field (actual efficiency) settings, with a high number of replicates. Moving forward, future research should place greater emphasis on examining the performance of woodchip bags, particularly regarding P removal, across various experimental scales in streams. This approach will provide valuable insights into the effectiveness of woodchips on a larger scale, facilitating a more comprehensive assessment of their efficacy in real-world scenarios.
Data availability statement
The original contributions presented in the study are included in the article/Supplementary Material, further inquiries can be directed to the corresponding author.
Author contributions
EA: Conceptualization, Investigation, Visualization, Formal analysis, Writing–original draft, Writing–review and editing. TM: Investigation, Formal analysis, Writing–review and editing. A-LD: Investigation, Formal analysis, Writing–review and editing. KA: Conceptualization, Formal analysis, Writing–review and editing. RH-N: Writing–review and editing. GW: Conceptualization, Formal analysis, Writing–original draft, Writing–review and editing, Supervision.
Funding
The author(s) declare that financial support was received for the research, authorship, and/or publication of this article. This study was funded by the states of Lower Austria as part of the RTI lead project RIBUST (Riparian BUffer STrips) (RIBUST, K3-F-130/005-2019; https://www.riparianbuffer.at).
Acknowledgments
Thanks to Theresa Reichenpfader, Annette Puritscher for their support in the laboratory and Sandra Schnabl and Maria Scharner for their support in the outdoor flume experiment.
Conflict of interest
The authors declare that the research was conducted in the absence of any commercial or financial relationships that could be construed as a potential conflict of interest.
Publisher’s note
All claims expressed in this article are solely those of the authors and do not necessarily represent those of their affiliated organizations, or those of the publisher, the editors and the reviewers. Any product that may be evaluated in this article, or claim that may be made by its manufacturer, is not guaranteed or endorsed by the publisher.
References
Aalto, S. L., Suurnäkki, S., von Ahnen, M., Siljanen, H. M. P., Pedersen, P. B., Tiirola, M., et al. (2020). Nitrate removal microbiology in woodchip bioreactors: a case-study with full-scale bioreactors treating aquaculture effluents. Sci. Total Environ. 25 (723), 138093. doi:10.1016/j.scitotenv.2020.138093
Addy, K., Gold, A. J., Christianson, L. E., David, M. B., Schipper, L. A., and Ratigan, N. A. (2016). Denitrifying bioreactors for nitrate removal: a meta-analysis. J. Environ. Qual. 45, 873–881. doi:10.2134/jeq2015.07.0399
Akbari, E., Baldan, D., Watzinger, A., and Weigelhofer, G. (2023). Leaves stimulate aquatic phosphorus uptake by dark-grown but not by light-grown microbial communities in sediments: a laboratory study. Freshw. Biol. 69, 321–334. doi:10.1111/fwb.14213
Allen, J., Laviale, M., Cellamare, M., Bachelet, Q., Felten, V., and Danger, M. (2019). Do leaf-litter decomposers control biofilm primary production and benthic algal community structure in forest streams? Insights from an outdoor mesocosm experiment. Freshw. Biol. 65, 1256–1269. doi:10.1111/fwb.13475
Bechtold, J. S., Edwards, R. T., and Naiman, R. J. (2003). Biotic versus hydrologic control over seasonal nitrate leaching in a floodplain forest. Biogeochemistry 63, 53–72. doi:10.1023/A:1023350127042
Bell, C. W., Fricks, B. E., Rocca, J. D., Steinweg, J. M., McMahon, S. K., and Wallenstein, M. D. (2013). High-throughput fluorometric measurement of potential soil extracellular enzyme activities. J. Vis. Exp. 81, e50961. doi:10.3791/50961
Bock, E. M., Coleman, B. S. L., and Easton, Z. M. (2018). Performance of an under-loaded denitrifying bioreactor with biochar amendment. J. Environ. Manag. 217, 447–455. doi:10.1016/j.jenvman.2018.03.111
Brailsford, F. L., Glanville, H. C., Golyshin, P. N., Marshall, M. R., Lloyd, C. E., Johnes, P. J., et al. (2019). Nutrient enrichment induces a shift in dissolved organic carbon (DOC) metabolism in oligotrophic freshwater sediments. Sci. Total Environ. 690, 1131–1139. doi:10.1016/j.scitotenv.2019.07.054
Burbery, L. F., and Abraham, P. (2022). “Results from an in-stream woodchip denitrifying bioreactor field trial in South Canterbury,” in Adaptive strategies for future farming. Editors C. L. Christensen, D. J. Horne, and R. Singh (Palmerston North, New Zealand: Massey University), 10.
Cameron, S. G., and Schipper, L. A. (2010). Nitrate removal and hydraulic performance of organic carbon for use in denitrification beds. Ecol. Eng. 36, 1588–1595. doi:10.1016/j.ecoleng.2010.03.010
Christianson, L. E., Cooke, R. A., Hay, C. H., Helmers, M. J., Feyereisen, G. W., Ranaivoson, A. Z., et al. (2021). Effectiveness of denitrifying bioreactors on water pollutant reduction from agricultural areas. Trans. Am. Soc. Agric. Biol. Eng. 64 (2), 641–658. doi:10.13031/trans.14011
Christianson, L. E., and Schipper, L. A. (2016). Moving denitrifying bioreactors beyond proof of concept: introduction to the special section. J. Environ. Qual. 45, 757–761. doi:10.2134/jeq2016.01.0013
Cooper, S. D., Barmuta, L., Sarnelle, O., Kratz, K., and Diehl, S. (1998). Quantifying spatial heterogeneity in streams. J. North Am. Benthol. Soc. 16, 174–188. doi:10.2307/1468250
Dougherty, H. L. (2018). Hydraulic evaluation of a denitrifying bioreactor with baffles. Crop Sci. Master’s43.
Duggan DiDominic, K. L., Shapleigh, J. P., Walter, M. T., Wang, Y. S., Reid, M. C., and Regan, J. M. (2024). Microbial diversity and gene abundance in denitrifying bioreactors: a comparison of the woodchip surface biofilm versus the interior wood matrix. J. Environ. Qual. 53, 565–576. Epub ahead of print. PMID: 39014985. doi:10.1002/jeq2.20600
Ezzati, G., Fenton, O., Healy, M. G., Christianson, L., Feyereisen, G. W., Thornton, S., et al. (2020). Impact of P inputs on source-sink P dynamics of sediment along an agricultural ditch network. J. Environ. Manag. 257, 109988. doi:10.1016/j.jenvman.2019.109988
Fan, Y., Essington, M., Jagadamma, S., Zhuang, J., Schwartz, J., and Lee, J. (2022). The global significance of abiotic factors affecting nitrate removal in woodchip bioreactors. Sci. Total Environ. 848, 157739. doi:10.1016/j.scitotenv.2022.157739
Fan, Y., Zhuang, J., Essington, M., Jagadamma, S., Schwartz, J., and Lee, J. (2023). Global significance of substrates for nitrate removal in denitrifying bioreactors revealed by meta-analysis. Engineering 21, 214–226. doi:10.1016/j.eng.2022.08.017
Ferreira, V., Castagneyrol, B., Koricheva, J., Gulis, V., Chauvet, E., and Graça, M. A. S. (2015). A meta-analysis of the effects of nutrient enrichment on litter decomposition in streams. Biol. Rev. 90, 669–688. doi:10.1111/brv.12125
Fork, M. L., Osburn, C. L., and Heffernan, J. B. (2020). Bioavailability and compositional changes of dissolved organic matter in urban headwaters. Aquat. Sci. 82, 66–15. doi:10.1007/s00027-020-00739-7
Goeller, B. C., Burbery, L. F., Febria, C. M., Collins, K. E., Burrows, N. J., Simon, K. S., et al. (2019). Capacity for bioreactors and riparian rehabilitation to enhance nitrate attenuation in agricultural streams. Ecol. Eng. 134, 65–77. doi:10.1016/j.ecoleng.2019.03.014
Goodwin, G. E., Bhattarai, R., and Cooke, R. (2015). Synergism in nitrate and orthophosphate removal in subsurface bioreactors. Ecol. Eng. 84, 559–568. doi:10.1016/j.ecoleng.2015.09.051
Gordon, L. J., Peterson, G. D., and Bennett, E. M. (2008). Agricultural modifications ofhydrological flows create ecological surprises. Trends Ecol. Evol. 23, 211–219. doi:10.1016/j.tree.2007.11.011
Graeber, D., Poulsen, J. R., Heinz, M., Rasmussen, J. J., Zak, D., Gücker, B., et al. (2018). Going with the flow: planktonic processing of dissolved organic carbon in streams. Sci. Total Environ. 625, 519–530. doi:10.1016/j.scitotenv.2017.12.285
Graeber, D., Tenzin, Y., Stutter, M., Weigelhofer, G., Shatwell, T., von Tümpling, W., et al. (2021). Bioavailable DOC: reactive nutrient ratios control heterotrophic nutrient assimilation—an experimental proof of the macronutrient-access hypothesis. Biogeochemistry 155, 1–20. doi:10.1007/s10533-021-00809-4
Grießmeier, V., Wienhöfer, J., Horn, H., and Gescher, J. (2021). Assessing and modeling biocatalysis in field denitrification beds reveals key influencing factors for future constructions. Water Res. 188, 116467. doi:10.1016/j.watres.2020.116467
Griffiths, N. A., and Johnson, L. T. (2018). Influence of dual nitrogen and phosphorus additions on nutrient uptake and saturation kinetics in a forested headwater stream. Freshw. Sci. 37, 810–825. doi:10.1086/700700
Hartfiel, L. M., Schaefer, A., Howe, A. C., and Soupir, M. L. (2022). Denitrifying bioreactor microbiome: understanding pollution swapping and potential for improved performance. J. Environ. Qual. 51, 1–18. doi:10.1002/jeq2.20302
Hathaway, S. K., Bartolerio, N. A., Rodrìguez, L. F., Kent, A. D., and Zilles, J. L. (2017). Denitrifying bioreactors resist disturbance from fluctuating water levels. Front. Environ. Sci. 5, 1–8. doi:10.3389/fenvs.2017.00035
Hedges, L. V., Gurevitch, J., and Curtis, P. S. (1999). The meta-analysis of response ratios in experimental ecology. Ecology 80 (4), 1150–1156. doi:10.2307/177062
Hua, G., Salo, M. W., Schmit, C. G., and Hay, C. H. (2016). Nitrate and phosphate removal from agricultural subsurface drainage using laboratory woodchip bioreactors and recycled steel byproduct filters. Water Res. 102, 180–189. doi:10.1016/j.watres.2016.06.022
Husk, B. R., Sanchez, J. S., Anderson, B. C., Whalen, J. K., and Wootton, B. C. (2018). Removal of phosphorus from agricultural subsurface drainage water with woodchip and mixed-media bioreactors. J. Soil Water Conservation 73, 265–275. doi:10.2489/jswc.73.3.265
Jarvie, H. P., Smith, D. R., Norton, L. R., Edwards, F. K., Bowes, M. J., King, S. M., et al. (2018). Phosphorus and nitrogen limitation and impairment of headwater streams relative to rivers in Great Britain: a national perspective on eutrophication. Sci. Total Environ. 621, 849–862. doi:10.1016/j.scitotenv.2017.11.128
Kamjunke, N., Herzsprung, P., and Neu, T. R. (2015). Quality of dissolved organic matter affects planktonic but not biofilm bacterial produc-tion in streams. Sci. Total Environ. 506–507, 353–360. doi:10.1016/j.scitotenv.2014.11.043
Kouanda, A., and Hua, G. (2021). Determination of nitrate removal kinetics model parameters in woodchip bioreactors. Water Res. 195, 116974. doi:10.1016/j.watres.2021.116974
Li, S., Cooke, R. A., Huang, X., Christianson, L., and Bhattarai, R. (2018). Evaluation of fly ash pellets for phosphorus removal in a laboratory-scale denitrifying bioreactor. J. Environ. Mgmt. 207, 269–275. doi:10.1016/j.jenvman.2017.11.040
Malá, J., and Lagová, M. (2014). Comparison of digestion methods for determination of total phosphorus in river sediments. Chem. Pap. 68, 1015–1021. doi:10.2478/s11696-014-0555-5
Mardani, S., McDaniel, R., Bleakley, B. H., Hamilton, T. L., Salam, S., and Amegbletor, L. (2020). The effect of woodchip bioreactors on microbial concentration in subsurface drainage water and the associated risk of antibiotic resistance dissemination. Ecol. Eng. 6, 100017. doi:10.1016/j.ecoena.2020.100017
Marx, M. C., Wood, M., and Jarvis, S. C. (2001). A microplate fluorimetric assay for the study of enzyme diversity in soils. Soil Biol. Biochem. 33, 1633–1640. doi:10.1016/S0038-0717(01)00079-7
Maxwell, B. M., Birgand, F., Schipper, L. A., Christianson, L. E., Tian, S., Helmers, M. J., et al. (2019). Drying–rewetting cycles affect nitrate removal rates in woodchip bioreactors. J. Environ. Qual. 48, 93–101. doi:10.2134/jeq2018.05.0199
McGuire, P. M., Butkevich, N., Saksena, A. V., Walter, M. T., Shapleigh, J. P., and Reid, M. C. (2023). Oxic–anoxic cycling promotes coupling between complex carbon metabolism and denitrification in woodchip bioreactors. Environ. Microbiol. 25, 1696–1712. doi:10.1111/1462-2920.16387
McGuire, P. M., Dai, V., Walter, M. T., and Reid, M. C. (2021). Labile carbon release from oxic-anoxic cycling in woodchip bioreactors enhances nitrate removal without increasing nitrous oxide accumulation. Environ. Sci. Water Res. Technol. 7, 2357–2371. doi:10.1039/d1ew00446h
Menczelesz, N., Szivak, I., and Schmera, D. (2019). How do we construct and operate experimental streams? An overview of facilities, protocols, and studied questions. Hydrobiol 847, 1–10. doi:10.1007/s10750-019-04093-0
Mulholland, P. J., Steinman, A. D., and Elwood, J. W. (1990). Measurement of phosphorus uptake length in streams: comparison of radiotracer and stable PO4 releases. Can. J. Fish. Aquatic Sci. 47, 2351–2357. doi:10.119/f90-261
O’Brien, J. M., Dodds, W. K., Wilson, K. C., Murdock, J. N., and Eichmiller, J. (2007). The saturation of N cycling in Central Plains streams: 15N experiments across a broad gradi-ent of nitrate concentrations. Biogeochemistry 84, 31–49. doi:10.1007/s10533-007-9073-7
O'Brien, J. M., Warburton, H. J., Graham, S. E., Franklin, H. M., Febria, C. M., Hogsden, K. L., et al. (2017). Leaf litter additions enhance stream metabolism, denitrification, and restoration prospects for agricultural catchments. Ecosphere 8 (11), e02018. doi:10.1002/ecs2.2018
Perera, G. N., Rojas, D. T., Rivas, A., Barkle, G., Moorhead, B., Schipper, L. A., et al. (2024). Elucidating phosphorus removal dynamics in a denitrifying woodchip bioreactor. Sci. Total Environ. 917, 170478. doi:10.1016/j.scitotenv.2024.170478
Pluer, W. T., Geohring, L. D., Steenhuis, T. S., and Walter, M. T. (2016). Controls influencing the treatment of excess agricultural nitrate with denitrifying bioreactors. J. Environ. Qual. 45, 772–778. doi:10.2134/jeq2015.06.0271
Povilaitis, A., Matikienė, J., and Vismontienė, R. (2020). Effects of three types of amendments in woodchip-denitrifying bioreactors for tile drainage water treatment. Ecol. Eng. 158, 106054. doi:10.1016/j.ecoleng.2020.106054
R Core Team (2020). R: a language and environment for statistical computing. Vienna, Austria: R Foundation for Statistical Computing. Available at: https://www.R-project.org/.
Rier, S. T., Kinek, K. C., Hay, S. E., and Francoeur, S. N. (2016). Polyphosphate plays a vital role in the phosphorus dynamics of stream periphyton. Freshw. Sci. 35, 490–502. doi:10.1086/685859
Rivas, A., Barkle, G., Moorhead, B., Clague, J., and Stenger, R. (2019) “Nitrate removal efficiency and secondary effects of a woodchip bioreactor for the treatment of agricultural drainage,” in Nutrient loss mitigations for compliance in agriculture, 10p. Occasional Report No. 32.
Ryu, Y., Han, H., Na, T., Kim, G., Druffel, E. R. M., and Hwang, J. (2023). Transport of aged dissolved organic carbon via the surface current revealed by radiocarbon. Geophys. Res. Lett. 50, 1–9. doi:10.1029/2023GL105296
Sanchez Bustamante-Bailon, A. P., Margenot, A., Cooke, R. A. C., and Christianson, L. E. (2022). Phosphorus removal in denitrifying woodchip bioreactors varies by wood type and water chemistry. Environ. Sci. Pollut. Res. 29, 6733–6743. doi:10.1007/s11356-021-15835-w
Schaefer, A., Lee, J., Soupir, M. L., Moorman, T. B., and Howe, A. (2022). Comparison of microbial communities in replicated woodchip bioreactors. J. Environ. Qual. 51, 205–215. doi:10.1002/jeq2.20320
Schaefer, A., Werning, K., Hoover, N., Tschirner, U., Feyereisen, G., Moorman, T. B., et al. (2021). Impact of flow on woodchip properties and subsidence in denitrifying bioreactors. Agrosyst. Geosci. Environ. 4 (1), e20149. doi:10.1002/agg2.20149
Singer, G., Besemer, K., Schmitt-Kopplin, P., Hödl, I., and Battin, T. J. (2010). Physical heterogeneity increases biofilm resource use and its molecular diversity in stream mesocosms. PLoS ONE 5, e9988. doi:10.1371/journal.pone.0009988
Soupir, M. L., Hoover, N. L., Moorman, T. B., Law, J. Y., and Bearson, B. L. (2018). Impact of temperature and hydraulic retention time on pathogen and nutrient removal in woodchip bioreactors. Ecol. Eng. 112, 153–157. doi:10.1016/j.ecoleng.2017.12.005
Stream Solute Workshop (1990). Concepts and methods for assessing solute dynamics in stream ecosystems. J. North Am. Benthol. Soc. 9, 95–119. doi:10.2307/1467445
Stutter, M., Graeber, D., and Weigelhofer, G. (2020a). Available dissolved organic carbon alters uptake and recycling of phosphorus and nitrogen from river sediments. WaterSwitzerl. 12, 3321. doi:10.3390/w12123321
Stutter, M., Wyness, A., Watson, H., and Dodd, N. (2020b). Coupled macronutrient cycling in stream biofilms: effects of stoichiometry, light and temperature. Sci. Total Environ. 703, 134880. doi:10.1016/j.scitotenv.2019.134880
Stutter, M. I., Graeber, D., Evans, C. D., Wade, A. J., and Withers, P. J. A. (2018). Balancing macronutrient stoichiometry to alleviate eutrophication. Sci. Total Environ. 634, 439–447. doi:10.1016/j.scitotenv.2018.03.298
Sunjidmaa, N., Mendoza-Lera, C., Hille, S., Schmidt, C., Borchardt, D., and Graeber, D. (2022). Carbon limitation may override fine-sediment induced alterations of hyporheic nitrogen and phosphorus dynamics. Sci. Total Environ. 837, 155689. doi:10.1016/j.scitotenv.2022.155689
Tank, J. L., Reisinger, A. J., and Rosi, E. J. (2017) “Nutrient limitation and uptake,” in Methods in stream ecology. Elsevier Inc. doi:10.1016/B978-0-12-813047-6.00009-7
Viechtbauer, W. (2010). Conducting meta-analyses in R with the meta-for package. J. Stat. Softw. 1, 1–48. doi:10.18637/jss.v036.i03
Von, A. M., Pedersen, P. B., and Dalsgaard, J. (2018). Performance of full-scale woodchip bioreactors treating effluents from commercial RAS. Aquac. Eng. 83, 130–137. doi:10.1016/j.aquaeng.2018.10.004
Wagner, K., Bengtsson, M. M., Findlay, R. H., Battin, T. J., and Ulseth, A. J. (2017). High light intensity mediates a shift from allochthonous to autochthonous carbon use in phototrophic stream biofilms. J. Geophys. Res. Biogeosciences 122, 1806–1820. doi:10.1002/2016JG003727
Warneke, S., Schipper, L. A., Matiasek, M. G., Scow, K. M., Cameron, S., Bruesewitz, D. A., et al. (2011). Nitrate removal, communities of denitrifiers and adverse effects in different carbon substrates for use in denitrification beds. Water Res. 45, 5463–5475. doi:10.1016/j.watres.2011.08.007
Weigelhofer, G., Jirón, T. S., Yeh, T. C., Steniczka, G., and Pucher, M. (2020). Dissolved organic matter quality and biofilm composition affect microbial organic matter uptake in stream flumes. WaterSwitzerl. 12, 3246. doi:10.3390/w12113246
Weigelhofer, G., Ramião, J. P., Puritscher, A., and Hein, T. (2018). How do chronic nutrient loading and the duration of nutrient pulses affect nutrient uptake in headwater streams? Biogeochemistry 141, 249–263. doi:10.1007/s10533-018-0518-y
Keywords: phosphorus removal, agricultural streams, nature-based solutions, organic-carbon addition, biofilms
Citation: Akbari E, Matjašič T, Dittrich A-L, Attermeyer K, Hood-Nowotny R and Weigelhofer G (2024) Testing woodchips for their efficiency in stimulating aquatic nutrient uptake at different experimental and spatial scales. Front. Environ. Sci. 12:1419413. doi: 10.3389/fenvs.2024.1419413
Received: 18 April 2024; Accepted: 02 September 2024;
Published: 13 September 2024.
Edited by:
David William O’Connell, Trinity College Dublin, IrelandReviewed by:
Yuchuan Fan, University of Florida, United StatesSanni Leea Aalto, Technical University of Denmark, Denmark
Copyright © 2024 Akbari, Matjašič, Dittrich, Attermeyer, Hood-Nowotny and Weigelhofer. This is an open-access article distributed under the terms of the Creative Commons Attribution License (CC BY). The use, distribution or reproduction in other forums is permitted, provided the original author(s) and the copyright owner(s) are credited and that the original publication in this journal is cited, in accordance with accepted academic practice. No use, distribution or reproduction is permitted which does not comply with these terms.
*Correspondence: Elmira Akbari, ZWxtaXJhLmFrYmFyaUB3Y2wuYWMuYXQ=