- 1Department of General Biology and Genetics, Institute of Biochemical Technologies, Ecology and Pharmacy, V.I. Vernadsky Crimean Federal University, Simferopol, Russia
- 2Laboratory of Entomology and Phytopathology, Dendrology and Landscape Architecture, Nikita Botanical Gardens – National Scientific Centre of the Russian Academy of Sciences, Yalta, Russia
Each new class of insecticides that emerged during the development of plant protection gradually found the most suitable group of insect pests for application. At the same time, for each individual insecticide, a balance was sought between its effectiveness, on the one hand, and its safety for non-target organisms and the ecosystem as a whole, on the other hand. Neonicotinoids, diamides and pyrethroids, as effective control agents, dominate the insecticide market, but do not have outstanding performance in selectivity and biodegradation. The biodegradation of insecticides is one of the most important indicators, representing what will be said about the hidden costs for the resulting harvest paid by the environment and human health. Oligonucleotide insecticides (contact unmodified antisense DNA (CUAD) biotechnology, or ‘genetic zipper’ method) and RNA biocontrols (double-stranded RNA technology) as natural polymers and the next-generation classes of insecticides possess unique characteristics in fast biodegradation and high selectivity in action. While current chemical insecticides require days, months and even years for biodegradation by bacteria and fungi, oligonucleotide insecticides and RNA biocontrols are substantially biodegraded within hours in the presence of nucleases. Nucleic acid-based insecticides have the potential to complement the existing insecticide market and set an eco-precedent for crop protection products where the effectiveness of the insecticide will be determined by its safety for non-target organisms, and other factors being equal, the choice of a particular control agent will be determined by its biodegradability. It should be noted that not a single class of insecticides that once appeared has completely disappeared; rather, it has occupied its niche, gradually declining under the pressure of new classes of insecticides. At the same time, the common trend in plant protection is towards use of insecticides with higher biodegradability, which gives hope for a safer future of the planet.
1 Introduction
Insecticides, like any other substances that enter insect cell, are subject to biodegradation and disposal. Substances of natural origin undergo rapid biodegradation, while xenobiotics degrade slowly. The rate of decomposition of xenobiotics depends on how many functional groups they contain found in natural molecules, how they are arranged and depends on activity of enzymes that can catalyze the transformation reactions with the participation of these functional groups (Miglani et al., 2022). Microorganisms due to their short life cycle are able to quickly adapt to an environment that accumulated xenobiotics (Miglani et al., 2022; Mishra et al., 2021). During their rapid microevolution microorganisms form strains that can quickly degrade xenobiotics reducing the ecotoxicological load in ecosystems (Huang et al., 2018). Should also be noted that omics approaches (genomics, transcriptomics, metabolomics, proteomics) addressing microbial metabolism and physiology could be of immense help towards unravelling the mechanism behind the phenomenon and even developing novel biotechnological tools (Rodríguez et al., 2020). Genera Pseudomonas, Bacillus, Alcaligenes, Cladosporium, Actinomyces, Nocardia, Aspergillus, Fusarium, Trichoderma, Mortierella, etc., are considered as perfect “biodegradation micro machines” for many classes of insecticides. Just like a hundred years ago, when creating insecticides, scientists first of all focus on effectiveness and only then consider the possibilities of minimizing the negative effect of new insecticides on non-target organisms (Sánchez-Bayo, 2021). Of course, it is impossible to calculate all the environmental risks of an insecticide being created, otherwise it will never reach the market. However, it is the effectiveness of an insecticide that most often brings it to the market (Kalyabina et al., 2021), while the safety of the insecticide and its low potential for biodegradation most often influences its ban (Damalas and Eleftherohorinos, 2011). Majority of insecticides were banned in one way or another after decades or years of use in plant protection, when effective competitors with proven or supposed greater safety appeared (Donley, 2019).
Any insecticide can be evaluated in terms of its effectiveness, affordability, half-life (biodegradation) and safety. Biodegradation and the safety of an insecticide are almost always related: the safer the insecticide, the shorter its half-life (Pathak et al., 2022). Nature does not slow down the degradation of compounds that have natural origin; they are broken down to water, carbon dioxide, ammonia, acetic acid, phosphoric acid, and other simple substances serving as precursors for more complex natural compounds. Fortunately, later classes of insecticides reduced their hazard for non-target organisms and increased their biodegradability potential (Araújo et al., 2023). However, using the example of neonicotinoids, as one of the later class of insecticides, and their dangerous effect on pollinators and birds, we see that the achieved level is still insufficient (Muth and Leonard, 2019). Today, a new generation of plant protection products is being developed, based on biopolymers, DNA (contact unmodified antisense DNA (CUAD) biotechnology) and RNA (double-stranded RNA technology) (Oberemok et al., 2019; Oberemok et al., 2022; Oberemok et al., 2023; Gal’chinsky et al., 2023; Puzanova et al., 2023; Rank and Koch, 2021; De Schutter et al., 2022). The function of these natural molecules in the cell primarily emphasizes the exceptional selectivity of the action of such compounds, which depends on the combination of nitrogenous bases. Both CUAD biotechnology and double-stranded RNA technology are essentially based on the mechanism of gene silencing using antisense DNA and RNA fragments and DNA- or RNA-guided nucleases (Oberemok et al., 2018). Both DNA and RNA are natural molecules that eventually are biodegraded by ubiquitous nucleases in ecosystems (Yang, 2011). If in the near future a balance is found between the effectiveness and cost of such pest control agents, then the insecticide market will be replenished in abundance with two new classes of insecticides possessing high level of biodegradability. Obviously, such insecticides will not become a panacea, but will be effective against certain orders of insect pests. In the medium term (5–10 years) insecticides based on nucleic acids will not be able to dominate the insecticide market, but their appearance on the market will be another quantum leap towards safe and sustainable plant protection.
In this article we will consider biodegradability of chemical insecticides including novel pest control agents based on CUAD biotechnology (oligonucleotide insecticides) and double-stranded RNA technology (RNA biocontrols). Priority of consideration of classes of insecticides in this article will follow rank of total sales worldwide of a particular class of pest control agents.
1.1 Neonicotinoids
The age of neonicotinoids (neonics) began with nitromethylene nithiazine, but really was inspired by Shinzo Kagabu’s important discoveries (Casida, 2018), culminating in 1990s with the introduction of chloropyridinyl and nitroimine moieties to generate imidacloprid, the best-selling insecticide for many years (Nugnes et al., 2023). Today, the neonicotinoid class accounts for 20% of total pesticide sales worldwide (King, 2022).
Neonicotinoids are used on a wide range of crops, such as horticultural and industrial crops, grapes, citrus fruits, pome fruits, stone fruits, flower and ornamental plants (Kundoo et al., 2018). Neonicotinoids can control a wide range of insect pests (Matsuda et al., 2020), including representatives of orders Hemiptera (Li et al., 2018; Li L. et al., 2020; Rizwan et al., 2022; Barbosa et al., 2018) and Lepidoptera (Krishnan et al., 2021); soil insects (Labrie et al., 2020) and Colorado potato beetle (CPB) Leptinotarsa decemlineata (Jeschke et al., 2011) are included among the target pests (Kundoo et al., 2018).
It was found that insecticides of this group are able to bioaccumulate and persist in the environment after period of their use, which is due to the low bioavailability and ability of their residues to bind to the soil matrix (Hussain et al., 2016; Zamule et al., 2021). The persisting chemicals in this group, clothianidin and imidacloprid, have the highest half-lives of about 1,250–6,931 days in sandy loam soil, having slower rates of decomposition in temperate climates (Farahy et al., 2021; Goulson, 2013). In general, the half-life of neonicotinoids in soil typically ranges from 200 to more than 1,000 days. The moderate half-life (7–353 days) is shown for thiamethoxam in soil under dry conditions (Goulson, 2013; Bonmatin et al., 2015). Relatively lower persistence in soil was observed for thiacloprid and dinotefuran with half-lives of approximately 90 days (Gautam and Dubey, 2023). Half-life appears to be shorter for the N-cyanoamidines (for cetamiprid it comprises ca. 450 days) (Goulson, 2013). Traces of hazardous neonicotinoid residues have been found dispersed in parts per million and parts per billion amounts in open environments (Bonmatin et al., 2015). Clothianidin, imidacloprid and thiamethoxam in water have half-life of 365–408 days (Borsuah et al., 2020).
Epidemiological studies are sparse, but they do relate neonicotinoid exposure to oxidative stress, neurological symptoms, metabolic alterations, osteoporosis, and liver cancer (Li A. J. et al., 2020; Marfo et al., 2015; Vuong et al., 2022; Carmichael et al., 2014), as well as imidacloprid exposure to developmental, congenital, hematologic, hepatic, and renal consequences (Carmichael et al., 2014; Khan et al., 2010; Shaw et al., 2014; Yang et al., 2014). Neonicotinoids have also caused huge losses in bee populations around the world (colony collapse syndrome) (Zee Van Der et al., 2012). Research has shown that trace amounts of neonicotinoids used in agriculture usually do not kill bees directly (lethal effect), but have an indirect (sublethal) effect, as bees exhibit all the symptoms of insecticide poisoning–uncoordinated movements, tremors and seizures (Buszewski et al., 2019). In 2019, the European Union prohibited two main neonicotinoids, imidacloprid and clothianidin, later thiamethoxam and thiacloprid were banned in 2020 because they were used on crops attractive to bees. Also due to side effects on bees, the sulfoxaflor was banned in 2022 (Strouhova et al., 2023).
Neonicotinoid insecticides are cleared from the environment through various processes of biotic (microbe- and plant-mediated) and abiotic (physicochemical) transformation (Gautam and Dubey, 2023). Microbes play an indispensable role in ultimate fate of neonicotinoid pollutants. The biocatalytic activity of microbes enables them to act as powerful degraders of neonicotinoids (Bilal et al., 2019). As an example, there are some powerful microorganisms such as genera Ensifer, Phanerochaete, Bacillus, Ochrobactrum, Trametes, Rhodococcus and Pseudomonas, which have enormous potential in degradation of neonicotinoids (Gautam and Dubey, 2023). Cytochrome P450 enzymes have been shown to actively mediate the degradation of neonicotinoids in various organisms. In bacteria, P450s were found to closely relate to hydroxylation (Wei et al., 2023). For instance, a study by Guo et al. (2020) using Hymenobacter latericoloratu CGMCC 16346 to reduce imidacloprid from surface water has shown that the process of the hydroxylation of imidacloprid to 5-hydroxy imidacloprid and olefin imidacloprid was inhibited by the presence of piperonyl butoxide, an inhibitor of cytochrome P450 monooxygenases (Guo et al., 2020). In a report, Sun et al. (2018) isolated Ensifer adhaerens CGMCC 6315 whose resting cells achieved ∼95% of 200 mg⋅L–1 acetamiprid degradation within 12 h and quickly eliminated ∼88% of 5 mg/kg of residual soil acetamiprid within 2 days (Sun et al., 2018). Phanerochaete chrysosporium (CCTCC AF 96007) can degrade ∼98% of thiamethoxam (10 mg⋅L–1) within 25 days (Gautam and Dubey, 2023; Sharma et al., 2014). Within 2 days, strain Ochrobactrum sp. D-12 was able to degrade acetamiprid with initial concentrations of 250–3,000 mg⋅L–1 (Wang et al., 2013). Specifically, Trametes versicolor could remove 20% and 64.7% of acetamiprid and imidacloprid (4 mg⋅L–1), respectively, in 7 days (Hu et al., 2022). Actinomycete Rhodococcus ruber (CGMCC 17550) can degrade neonicotinoid insecticide nitenpyram (25 mg⋅L–1) by 71.85% and 87.11% after 3 and 8 days of incubation, respectively (Dai et al., 2021). Bacillus brevis, Pseudomonas sp. F1, Bacillus subtilis and Rhizobia degraded 25%–45% of imidacloprid (25 g⋅L−1) in a carbon-limited minimum salt environment in 25 days (Sabourmoghaddam et al., 2015).
1.2 Pyrethroids
The next class we will consider is pyrethroids, its world market share accounts for 19% of total pesticide sales worldwide, worth approximately US$ 2.8 billion (King, 2022). Pyrethroids, like neonicotinoids, are effectively degraded by many bacteria and fungi. Historically, pyrethroids are a group of derivatives of naturally occurring compounds, pyrethrins, isolated from the flowers of the plant Chrysanthemum cinerariaefolium alias Tanacetum cinerariaefolium (Dalmatian pyrethrum) (Hołyńska-Iwan and Szewczyk-Golec, 2020; Hodoșan et al., 2023). Plant materials containing pyrethrins have been sold in Europe since the mid-18th century (Ahmed et al., 2021). The early research that laid the foundation for the discovery of pyrethroids has a significant historical trajectory dating back to the mid-20th century and characterized by a number of important advances in the development of synthetic insecticides.
Currently, pyrethroids are widely used in crop protection; in the forestry, woodworking, and textile industries; in medicine and veterinary medicine for the treatment of infections by parasitic crustaceans (Chrustek et al., 2018; Banks et al., 2014; Barlow et al., 2001; Orsborne et al., 2016; WHO, 2016). Pyrethroids are effective against a wide range of insect pests of the order Coleoptera (Athanassiou et al., 2015; Arthur et al., 2018; Stará and Kocourek, 2019), Hemiptera (Anderson and Cowles, 2012; Menger et al., 2020), Diptera (Önder and Huseyin, 2019; Santamaría and Marceló, 2019), Hymenoptera (Pereira Costa et al., 2020), Lepidoptera (Rabelo et al., 2020; Moreno et al., 2017), Orthoptera (Mullié et al., 2023), and Thysanoptera (Ahamad and Kumar, 2023a). As a rule, they are also used for personal protection against insects in the form of impregnated mosquito nets, sprays, or gels (Banks et al., 2014; Wylie et al., 2016; Ranjkesh et al., 2013).
Based on the vast amount of published research regarding the toxic effects of pyrethroids on non-target organisms and humans, some of these pyrethroids are banned in developed countries. Some prohibited pyrethroid insecticides such as fenvalerate, permethrin and other compounds, including certain metabolites, cause DNA damage in human (Jurewicz et al., 2015) and animal sperm, including decreased sperm count and concentration, decreased sperm motility, and increased abnormal sperm morphology (Cremonese et al., 2017; Orlu, 2014; Shi et al., 2011). Pyrethroids are neurotoxic pesticides and affect neurotransmitters (Shafer et al., 2005; Gammon et al., 2019). Additionally, a cohort study documented that some exposure to pyrethroids in pregnant women resulted in autism spectrum disorder in their children (Barkoski et al., 2021). At the animal experimental level, it has been reported that certain pyrethroids, i.e., deltamethrin (Gasmi, 2020) and cypermethrin (Abd El-Moneim Ibrahim et al., 2020), caused a decrease in serotonin and dopamine levels in rats (Bayoumi, 2022). Unfortunately, it should be emphasized that, despite the proven negative impact of pyrethroids on human health, at the current stage of knowledge it is not possible to offer a safer measure of personal protection against insects (WHO, 2014; WHO, 2015). However, for example, fenvalerate is banned in Sweden due to side effects in workers caused by occupational poisoning (Hadnagy et al., 2003; Muller-Mohnssen and Hahn, 1995).
Pyrethroids are currently emerging pollutants due to their ecotoxicological effects on aquatic systems even at extremely low concentrations (Geissen et al., 2015). Frequent use of pyrethroids in agriculture and forestry leads to the accumulation of their residues in the environment, especially in topsoil and water (Bragança et al., 2019; DeMars et al., 2021; Deng et al., 2020; Budd et al., 2020). These pyrethroids can enter rivers through a variety of routes, such as agricultural drainage, atmospheric deposition, surface runoff, and wastewater discharges (Alonso et al., 2012; Prusty et al., 2015; Luo and Zhang, 2011). In the natural environment, pyrethroids are degraded through biotic and abiotic pathways, including photooxidation, chemical oxidation, and biodegradation (Abraham and Silambarasan, 2014; Abraham and Silambarasan, 2016).
Today, there is a fairly large number of studies confirming that bacteria and fungi can quite effectively decompose the traces of pyrethroids in water and soil (Cycoń and Piotrowska-Seget, 2016; Huang et al., 2022; Huang et al., 2023). Microbiological degradation is considered a reliable and inexpensive method of recovery compared to common physicochemical methods (adsorption, photolysis and ozonation). It is important to note that microorganisms can use pyrethroids as a sole source of energy or with the help of other nutrients to remove pyrethroids through co-metabolism (Zhao et al., 2019). The primary way that pyrethroids are degraded by microorganisms is via ester-bond hydrolysis by carboxylesterases (carboxylic-ester hydrolase, EC 3.1.1.1), which yields carboxylate and alcohol (Sogorb and Vilanova, 2002; Aranda et al., 2014). A variety of potent pyrethroid-degrading bacteria have been reported. Bacillus cereus GW-01 (Jiang et al., 2023), Pseudomonas aeruginosa CH7 (Zhang et al., 2011), and Brevibacillus parabrevis BCP-09 (Tang et al., 2018a) can degrade beta-cypermethrin; Bacillus sp. DG-02 can degrade 61% of extremely high concentration of bifenthrin within 7 days (Chen et al., 2012); Ochrobactrum anthropi strain JCm1 (Akbar et al., 2015), Ochrobactrum lupini strain DG-S-01 (Chen et al., 2011a), Ochrobactrum tritici strain pyd-1 (Wang et al., 2011), and Serratia nematodiphila strain CB2 (Tyagi and Prashar, 2015) was found to be able to directly utilize more than 90% of the initial dose of cypermethrin within 5–10 days; Bacillus subtilis 1D has been reported to hydrolyze 95% of cypermethrin within 15 days; Paracoccus acridae SCU-M53 can metabolize 79.84% of cyhalothrin in 2 days (Tian et al., 2018); Photobacterium ganghwense can grow with cyfluthrin as the sole carbon source and degrade 60% of cyfluthrin in 3 days (Wang et al., 2019). Serratia marcescens DeI-1 (Cycoń and Piotrowska-Seget, 2016) and Lysinibacillus sp. ZJ6 (Jiang et al., 2023) can degrade deltamethrin; and Bacillus flexus XJU-4 (Mulla et al., 2017) and Bacillus licheniformis CY-012 (Tang et al., 2018a) can degrade fenvalerate. Also, fungi have been found as the effective degraders of pyrethroids, for example, Cladosporium sp. strain HU can degrade fenvalerate within 5 days (Chen et al., 2011b). Most pyrethroids are similar in structure, which opens the possibility for one strain to degrade many different pyrethroids (Bhatt et al., 2020).
1.3 Diamides
Next, we will look at the increasingly popular class of insecticides, diamides, with a global market share of 16% (King, 2022). Diamides like neonicotinoids and pyrethroids are degraded by many microorganisms. In 1993, the Japanese company Nihon Nohyaku began an insecticide program that led to a breakthrough in 1998 with the development of phthalimides, which have the aniline moiety replaced by a perfluoroalkyl side chain (Seo et al., 2008; Hamaguchi et al., 2012).
The spectrum of action of diamides is wide: flubendiamide mainly affects Lepidoptera, chlorantraniliprole controls whiteflies, leaf miners, beetles, and termite species in addition to lepidopterans, while cyantraniliprole is effective in controlling a large number of Lepidoptera, Hemiptera, Coleoptera, Diptera and Thysanoptera species (Teixeira and Andaloro, 2013; Li W. et al., 2019; Kadala et al., 2020). The insecticidal activities of some synthesized chlorantraniliprole derivatives were evaluated using a lepidopteran harmful pest of crops worldwide, diamondback moth Plutella xylostella (El-Sheikh and Ashour, 2021). Recent studies have found that broflanilide poses a potential risk to lady beetles Coccinella septempunctata (Cong et al., 2023).
When used extensively and incorrectly, diamides have a significant impact on the ecological environment (plant development and non-target organisms) (Ma et al., 2021). Phase I and phase II metabolism of xenobiotic compounds is thought to be mediated by three enzyme classes, cytochrome P450s (P450), carboxylesterases (CE) and glutathione S-transferases (GST) (Li et al., 2007). Metabolism of diamides in mammals has been shown to occur by oxidation, e.g., hydroxylation of alkyl groups (Yoshida, 2014). Although diamide metabolism in insects is not well elucidated, several studies have found upregulation of P450, CE or GST genes as well as enzyme activity in response to insecticide exposure, suggesting that the mechanism is potentially similar (Richardson et al., 2020). Reports have shown that the insecticide flubendiamide severely affects the antennal neurons of honey bees by disrupting calcium homeostasis (Kadala et al., 2020). Chlorantraniliprole and cyantraniliprole inhibit the growth rate, weight and reproduction of the earthworm Eisenia fetida (Qiao et al., 2019). It was also found that chronic exposure to chlorantraniliprole 20SC in pollen suppressed bumblebee reproduction and increased lethargy in the worker population for the duration of exposure (Smagghe et al., 2013). In addition, it was found that bees exposed to topical chlorantraniliprole had a decrease in locomotor activity (Kadala et al., 2019). It has also been reported that flubendiamide has been restricted or banned in some countries due to its toxicity to aquatic invertebrates (Zhao et al., 2020; Zhang H. et al., 2022).
The half-life of chlorantraniliprole was found to be 1.36 days after its use at recommended doses (Kar et al., 2013). The half-life of chlorantraniliprole was 51.3 and 62.5 days for low (1 mg kg−1) and high (10 mg kg−1) application dose, respectively, in a silty-loam paddy soil in subtropical China (Meng et al., 2018). Cyantraniliprole was found to be a relatively persistent compound in the soil, with a half-life of 16–89 days (EFSA, 2014). Half-life of tetraniliprole in maize plants and soil is ∼9 days (Ma et al., 2021). Flubendiamide degrades to des-iodoflubendiamide by laboratory photolysis of soil with a half-life of 135.5 days (Das et al., 2012). Flubendiamide degradation in soils was reported to follow first-order kinetics, and its average half-life in three types of soil ranged from 37.62 to 60.21 days. The persistence of flubendiamide in soils significantly increased in the following order: coastal soil > red and lateritic soil > new alluvial soils (Paramasivam and Banerjee, 2012). The half-life of photolysis of tetrachlorantraniliprole in natural water and pH buffers was 1.4–2.8 h, compared with 1.2–231 days of the half-life for hydrolysis (Zhou et al., 2022). Although diamide insecticides have been promising insecticides, their excessive use will result in their deposition into the soil (Zhang et al., 2020). Soil bioremediation is an environmentally friendly, cost-effective and efficient method compared to physical and chemical methods. Bhatt et al. (2021) showed that soil microorganisms can help degrade pesticides and be a source of energy, carbon and other nutrients (Bhatt et al., 2021). Recent results from Fahmy et al. (2022) showed that P. aeruginosa strain KZFS4 (LC599404.1) produced the highest CO2 content, about 1.226 mg CO2/16 days, with an efficiency in the biodegradation of flubendiamide-chlorantraniliprole (78.6%). Additionally, the lowest average CO2 production rate was recorded at 0.81 mg CO2/16 days (51.9%) for Bacillus mojavensis AZFS15 (Fahmy et al., 2022), while for B. subtilis strain AZFS3 produced 0.901 mg CO2/16 days (57.7%). Isolated Chryseobacterium sp. strain SSJ1 removed 89.06% of 1,000 mg⋅L–1 flubendiamide at optimum temperature of 35°C and pH 7.0 within 5 days of incubation period (Jadhav and David, 2016).
1.4 Organophosphates
Next, we will look at the organophosphate class of insecticides, which ranks fourth in popularity with a niche of 14% in the global market (King, 2022). Like diamides, organophosphate pesticides are degraded relatively quickly through hydrolysis on exposure to photolysis and microbial consortia. The basics of obtaining organophosphates (OP) started in the mid-19th century (Gammon et al., 2019; Laskowski, 2002), and they began to be used as insecticides in the first half of the 20th century (Abraham and Silambarasan, 2014). Among the characteristic representatives of organophosphates, the following insecticides can be distinguished: chlorpyrifos (Bragança et al., 2019; DeMars et al., 2021), monocrotophos (Li W. G. et al., 2019), malathion (Barkoski et al., 2021; Gasmi, 2020), dimethoate (Orlu, 2014; Shi et al., 2011), (Luo and Zhang, 2011; Gajendiran and Abraham, 2018) and quinalphos (Cycoń and Piotrowska-Seget, 2016). Organophosphate compounds target mostly pests such as termites (Paul et al., 2018), lice (Sanhueza-Guevara et al., 2018), flies (Galil et al., 2021; Van Laerhoven, 2008), mosquitoes (Rowland et al., 2013), and roundworms (de Albuquerque et al., 2020).
Organophosphates are capable of bioaccumulation and biomagnification, which leads to ecotoxicological effects (Jurewicz et al., 2015; Shafer et al., 2005). Chlorpyrifos was previously shown to be resistant to biodegradation and has now been shown to undergo enhanced microbial breakdown to produce less harmful and non-toxic metabolites. To date, research activity in this area has shown that a wide range of microorganisms are responsible for the degradation of chlorpyrifos (DeMars et al., 2021). The half-life of chlorpyrifos in soil is typically 60–120 days but can vary from 2 weeks to more than 1 year, depending on soil type, climate, and other conditions (Budd et al., 2020). For example, monocrotophos has a half-life of 17–96 days and results in surface and groundwater contamination, neurotoxicity, genotoxicity, and stress effects on various organisms (Alonso et al., 2012).
The insecticide dimethoate is decomposed by microbes under anaerobic conditions. It is known that the main product of its decomposition, omethoate, has been identified. Dimethoate has been found to have adverse effects on many organisms. In plants, photosynthesis and growth are severely affected, while in birds, brain enzyme activity is suppressed (Bhatt et al., 2019). Residues of organophosphates are washed out, accumulate in the soil, and groundwater contaminates terrestrial and aquatic food webs (Geissen et al., 2015). The insecticide quinalphos acts as a neurotoxicant that inhibits acetylcholinesterase, affecting the respiratory system, skin and eyes. Quinalphos affects aquatic organisms, especially freshwater fish (Ahamad and Kumar, 2023b). To date, there are many countries which have banned the usage of greater than 10 organophosphates: Mauritania (12), Thailand (12), Guinea (12), China (15), Saudi Arabia (15), Cambodia (15) and USA (26) (Hertz-Picciotto et al., 2018). In the US, the nation of Hawaii currently banned the distribution, sale, transportation and use of all insecticides containing chlorpyrifos (Reese, 2018).
Most microorganisms can degrade one OP or a narrow range of OP compounds (Singh, 2009). The biochemistry of organophosphorus compound degradation by most of the bacteria seems to be identical, in which a structurally similar enzyme called organophosphate hydrolase or phosphotriesterase catalyzes the first step of the degradation (Singh and Walker, 2006). Microbes such as Bacillus, Pseudomonas, Aspergillus, Anabaena and Nostoc at 25°C–37°C use monocrotophos as a source of nutrients and cause its complete or partial decomposition to dimethyl phosphate, phosphoric acid, and acetic acid (Alonso et al., 2012). Reported that Arthrobacter sp. can degrade chlorpyrifos and showed 99% (100 mg⋅L–1) decomposition within 10 h in modified mineral salt media (Mali et al., 2022). Strain Enterobacter aerogenes CP2 and Streptococcus pyogenes CP11 could remove 77% and 74% of chlorpyrifos, respectively (Lourthuraj et al., 2022). Strain Pseudomonas Ch1D degraded 98% of chlorpyrifos within 120 h of incubation at an initial pesticide concentration of 10 mg⋅L–1 (Singh, 2009). Two bacterial strains, Achromobacter xylosoxidans JCp4 and Ochrobactrum sp. FCp1 were capable to degrade 84.4% and 78.6% of the initial concentration of chlorpyrifos (100 mg⋅L–1) within 10 days, respectively (Akbar and Sultan, 2016). Moreover, it is also reported that 84.61% decomposition of quinalphos by Ochrobactrum sp. HZM strain can be achieved under optimum pH of seven at 27°C using response surface methodology (Mulla et al., 2020). In another study, three bacterial strains were isolated: P. aeruginosa PF3, P. aeruginosa PF2, and Pseudomonas plecoglossicida PF1, which have the ability to degrade profenofos. These bacterial strains individually decreased concentration of profenofos (20 mg⋅L–1) by 95.3%, 93.1% and 95.0% within 96 h, respectively (Siripattanakul-Ratpukdi et al., 2015).
Today, organophosphates are an integral part of agriculture; their excessive use leads to contamination of ground and surface waters. That is why the search for analogues and other types of insecticides that have more gentle effects and will not cause great harm to ecosystems continues.
1.5 Carbamates
The fifth place in popularity with a niche of 6% in the global insecticide market is occupied by carbamates (King, 2022), which will be discussed further. The first carbamic acid derivatives that have insecticidal properties were synthesized in 1947. These are N-methylcarbamates, which are derived from aminoformic acid (Tian et al., 2018; Wang et al., 2019).
Among carbamate insecticides, there are about 50 chemicals (Zhao et al., 2019; Chen et al., 2012; Bhatt et al., 2020). Some striking examples include the following insecticides: carbaryl is used for control of bark beetles Scolydidae (Dendroctonus ponderosae, D. adjunctus, D. rufipennis) (Akbar et al., 2015; Chen et al., 2011a); aldicarb is used as a systemic insecticide, acaricide and nematicide (Zhang et al., 2011; Tang et al., 2018a; Hao et al., 2018; Mulla et al., 2017); carbofuran is used as an insecticide and nematicide (Yan et al., 2018), exhibits broad-spectrum properties, affecting various pests such as soil insects, sap-feeding insects, chewing insects, and nematodes (Rizwan et al., 2022); tefluthrin can control lepidopteran and coleopteran pests (Wen et al., 2019).
Carbamates are typically transformed into different reaction products through various pathways, such as biotransformation, biodegradation, hydrolysis, bioaugmentation, oxidation, photolysis, and metabolic reactions in living organisms (Cai et al., 2015). There are few studies on the stability or migration of aldicarb in air. However, based on their research, scientists conclude that aldicarb or its decomposition products enter the vapor phase (Tang et al., 2018b). There are also early studies that indicate that aldicarb accumulates after its use in ground and drinking water (Nugnes et al., 2023; Buszewski et al., 2019; Kurwadkar and Evans, 2016). The half-life of aldicarb in soil is about 30 days; this may vary depending on microbial populations, soil composition, humidity, temperature (Casida, 2018; Mustapha et al., 2019). It should be noted that the half-life of a few carbamates in soil lasts from 1 to 210 days (for example, carbaryl – 4–72 days, carbosulfan – 1–2 days, propoxur – 80–210 days, pirimicarb – 53 days, and oxamyl – 2.5–4 days) (Mustapha et al., 2019). The insecticide carbofuran has a half-life of 16 years in acidic soil, whilst in normal, alkaline or alkaline soils, the half-life of carbofuran is 149 days (Krishnan et al., 2021). In addition, few microorganisms stay in acidic soil, so biodegradation does now no longer arise naturally (Jeschke et al., 2011; Gautam and Dubey, 2023).
Cases of carbamate poisoning are most often associated with intentional oral ingestion or occupational dermal exposure. Large outbreaks due to contaminated food and crops have been reported in developing countries (Tian et al., 2018). Long-term contamination from these pesticides is unlikely, but use of the runoff by marine animals may cause harm to them (Jiang et al., 2023). For example, carbamate aldicarb was banned in the European Union since 2003, plant protection products containing this compound benefited from exemptions in France until 2007 for use on beet and vines, but ultimately aldicarb was banned in Europe in 2007 (Berny et al., 2015; Boucaud-Maitre et al., 2019).
Today, quite a lot of isolated bacteria and fungi are known to be capable of degrading a wide range of carbamates in soil and water environments (Mishra et al., 2021; Malhotra et al., 2021). Various species of microorganisms involved in the transformation or degradation of carbamate pesticides have been reported to belong to the genera Pseudomonas, Stenotropomonas, Micrococcus, Enterobacter, Nocardioides, Pseudaminobacter, Serratia, Mucor, Trametes, Trichoderma, Pichia and Aspergillus, amongst others. The first step in the metabolic degradation of pesticide carbamates is their hydrolysis catalysed by carboxyl ester hydrolases (Ufarté et al., 2017). The isolated Sphingobacterium multiorum showed high carbofuran (50 mg⋅L–1) degradation ∼74% at pH 7°C and 25°C (Tien et al., 2017). Several Trichoderma spp., such as T. viride, T. harzianum can metabolize carbamate insecticide (Vydat®) 200 mg⋅Kg−1 up to 92% and 89.5%, respectively, within 14 days of incubation in soil (Helal and Abo-El-Seoud, 2015). The immobilized cells of Enterobacter cloacae strain TA7 showed high (100%) efficient in degradation for three carbamates: carbaryl (8.9 mg⋅L–1), carbofuran (17.87 mg⋅L–1), and aldicarb (22.6 mg⋅L–1) N-methylated carbamates (Fareed et al., 2017). Recent results showed that Sedum alfredii associated with 20.5 mg⋅Kg−1 of carbendazim-degrading bacterial strains enhanced carbendazim degradation up to 83.3% (Xiao et al., 2013).
However, the toxicity of carbamates entails consequences worse than people could imagine. That is why there is a search for different directions in the development and re-search of insecticides, thus their new forms and types appear from year to year. Carbamates are among the most toxic known pest control agents (Matsuda et al., 2020).
Although biodegradation of neonicotinoids, pyrethroids, diamides, OPs and carbamates are successfully performed by bacteria and fungi (Ensifer, Phanerochaete, Bacillus, Trichoderma, Trametes, Aspergillus, etc.), their fast biodegradation frequently depends on many factory (UV, pH, type of soil, etc.). Necessity of presence of multiple factors for successful biodegradation decreases speed of decomposition of carbamates and results in significant toxic load on ecosystems requiring months and even years for complete biodegradation. Obviously, use of natural molecules as insecticides can improve this situation. DNA and RNA insecticides in recent years made a huge progress in direction of creation of efficient end-products for plant protection whose biodegradation is limited to days and even hours.
1.6 Сontact unmodified antisense DNA (CUAD) biotechnology (oligonucleotide insecticides)
In 2008 it was discovered that unmodified antisense DNA may serve as contact insecticide on spongy moth Lymantria dispar (Oberemok, 2008). This surprising discovery revealed an entirely new dimension – DNA-programmable plant protection based on tiny antisense DNAs with profound insecticidal potential. Oligonucleotide insecticides (briefly, olinscides or DNA insecticides) as a new class of insecticides has its own peculiar characteristics. Olinscides are short unmodified antisense DNA fragments that use pre-RNA and rRNA of pests as target. A target rRNA and an olinscide interlock and resemble zipper mechanism performed by DNA-RNA duplex (‘genetic zipper’ method). Oligonucleotide insecticides act through DNA containment mechanism that consists of two steps: at first step antisense DNA oligonucleotide (oligonucleotide insecticide) complementarily interacts with target rRNA (in other words, ‘arrests’ target rRNA) and interferes with normal functioning of ribosomes (protein machinery and precise architecture of this step remains a mystery); at second step, RNase H cleaves target rRNA and substantial decrease in its concentration occurs (Gal’chinsky et al., 2024). Species-specific oligonucleotide insecticides are basic substances of DNA-programmed plant protection and CUAD (contact unmodified antisense DNA) platform (Figure 1). Our investigations suggest that short unmodified DNA sequences can play a crucial role in regulation of rRNA expression by complementary genomic DNA (direct rDNA master regulation) and replication of DNA viruses (rRNA switchboard mechanism) (Oberemok et al., 2024) and can turn to be essential in innate immunity system (Gal'chinsky et al,. 2024) against ssDNA viruses for which hemipteran insects serve as major vectors (Wang and Blanc, 2021; Wu et al., 2022) and also against DNA viruses that normally infect them (Guo et al., 2022).
Oligonucleotide insecticides represent new principle of action of pest control agents and have proven themselves most successful in the control of insect pests from the order Hemiptera; in average, mortality rate comprises 80%–90% in 3–14 days after single treatment (Gal’chinsky et al., 2024; Oberemok V. et al., 2024). The first successful experiment with olinscides within suborder Sternorrhyncha (Hemiptera) was carried out on the scale insect Unaspis euonymi in 2019 and pest 28S rRNA as a target for the oligonucleotide insecticide UE-11 was used (Gal’chinsky et al., 2020). Soft scale insects, armored scale insects, whiteflies, mealybugs, aphids, psyllids and other representatives of the suborder Sternorrhyncha show high mortality in response to the use of contact oligonucleotide insecticides targeting pest rRNAs (Oberemok et al., 2022; Gal’chinsky et al., 2023; Puzanova et al., 2023; Gal’chinsky et al., 2020; Useinov et al., 2020). Oligonucleotide insecticides and DNA-programmable plant protection is developing intensively (Oberemok V. et al., 2024; Oberemok V. V. et al., 2024) and it is not unrealistic to believe that ASO-based pesticides may become a reality sooner rather than later, advancing more quickly by leapfrogging past existing technical solutions (TriLink, 2024).
Degradation of nucleic acids mainly depends on abiotic factors (water temperature, pH, salinity, and ultraviolet (UV) radiation) and biotic factors (microbes and activity of extracellular enzymes) (Barnes and Turner, 2016). It is well known that most bacteria ingest environmental DNA (eDNA) through extracellular enzymes and ectoenzymes in search of nutrients in aquatic ecosystems. Active enzymes that degrade eDNA have been found in fractions of filtered water containing bacteria, algae, cyanobacteria, fungi, as well as unicellular and multicellular planktonic animals. (Siuda and Chróst, 2001; Mauvisseau et al., 2022). For example, extracellular DNase produced by P. aeruginosa, ensures the degradation of extracellular DNA into an available source of carbon, nitrogen and phosphate (Mulcahy et al., 2010); all members of the group A Streptococcus have been shown to produce at least one extracellular DNase, and most strains make more than one distinct enzyme (Cunningham, 2000; Allan et al., 2020). Additionally, degradation is likely to be related to genomic characteristics: target region, fragment length, genomic origin (nuclear or mitochondrial), and nucleic acid composition (DNA or RNA) (Barnes and Turner, 2016; Siuda and Chróst, 2001). However, studies of the nuclease activity of target insect pests (L. dispar, Icerya purchasi, L. decemlineata) and their host plants (Quercus pubescens, Pittosporum tobira, Solanum tuberosum) have shown that most of the used olinscides degrade within 24 h at 27°C (Oberemok et al., 2019; Oberemok et al., 2022; Oberemok et al., 2023; Gal’chinsky et al., 2023); and even faster, within 1 h, by DNases of Macrosiphoniella sanborni (Puzanova et al., 2023).
Recent results demonstrated remarkable specificity of oligonucleotide insecticides in action (Oberemok et al., 2019; Oberemok et al., 2018) and showed their safety for several non-target organisms: Quercus robur, Malus domestica (Zaitsev et al., 2015), Triticum aestivum (Oberemok et al., 2013; Nyadar et al., 2019), Manduca sexta, Agrotis ipsilon (Oberemok et al., 2015), Galleria mellonella (Oberemok et al., 2019). Use of insect pest pre-rRNA and rRNA as target leads to high efficiency of oligonucleotide insecticides, since pre-rRNA and rRNA comprise 80% of all RNA in the cell (Oberemok V. et al., 2024). Thousands of different mRNAs make up only 5% of all RNA and use of pre-rRNA and rRNA for targeting substantially increases signal-to-noise ratio, ca. 105:1 (rRNA vs. random mRNA). In this situation, even 11 nt long oligonucleotide insecticide provides sufficient level of selectivity in action, uniqueness frequency comprises ca. 1/4.19 · 10−6 (Oberemok et al., 2022). Moreover, recent research articles have shown that it is enough to replace one nucleotide in the sequence of an oligonucleotide insecticide to substantially reduce its effectiveness, in turn, this indicates high selectivity in action (Oberemok et al., 2019; Puzanova et al., 2023; Gal’chinsky et al., 2024). The advantage of using natural oligomers, unmodified antisense oligonucleotides, seems to be the safest way, since the cells of all living organisms contain ubiquitous nucleases that can neutralize them (Oberemok et al., 2019). Consequently, for oligonucleotide insecticides there is no need to look for methods of accelerated biodegradation. The principle of using oligonucleotide insecticides is that they must have enough time to act in the right place and on the right organism before their rapid biodegradation. In contrast, conventional chemical insecticides have too much time for their action not only in the right place and not only on the right organism. Thus, when using unmodified antisense DNA fragments, as well as double-stranded RNA fragments, in insect cells there will be competition between different types of nucleases for the substrate, mainly between exonucleases and nucleic acid-guided nucleases (Figure 2). Obviously, in the case of using DNA insecticides on sternorrhynchan pests, RNase H manages to successfully degrade the target rRNA in the DNA insecticide/rRNA duplex, which is ensured by the high concentration of the target rRNA in the insect cell (Oberemok V. et al., 2024). In addition, in the absence of nucleases, DNA is less susceptible to hydrolysis in the environment and insect cells than RNA molecules, due to the absence of an oxygen atom in the deoxyribose in the 2′ position (Thorp, 2000). This chemical parameter gives antisense DNA, rather than dsRNA, the opportunity to act before being degraded.
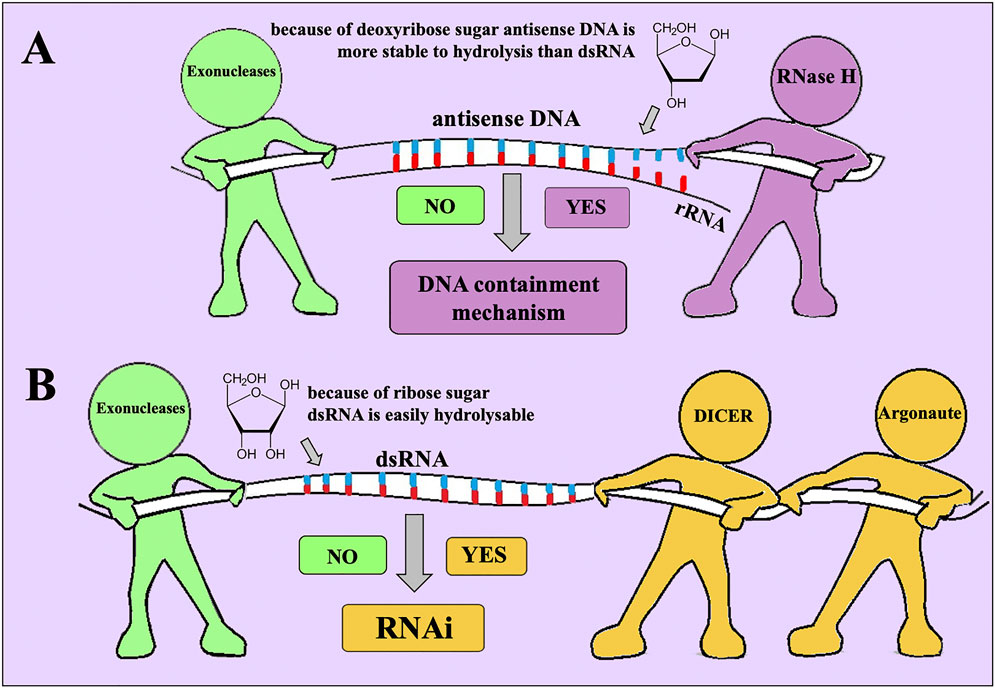
Figure 2. Competition between exonucleases and endonucleases together with nucleic acid-guided nucleases for their substrates: (A) exonucleases vs. DNA-guided RNase H; (B) exonucleases vs. endonuclease DICER and RNA-guided Argonaute.
The innovative strategy of managing pests using custom-made short oligonucleotides opens up a new horizon of “oligo-insecticides” (Patil et al., 2024) and DNA-programmable plant protection as an advanced technology. It should be noted that our research group has discovered a 50–100 nucleotides long DNA (mainly single-stranded) fraction on P. tobira leaves that has significant insecticidal activity against C. hesperidum (Oberemok et al., 2022). This fraction has been detected for four consecutive years (2021–2024) and is an example of DNA insecticides formed in nature. Thus, under natural conditions, plant DNA can be partially biodegraded and appear in the form of previously unknown nanolayer of single-stranded DNA insecticides on the surface of plant leaves and can be an example of adaptation to sap-feeding insects having sedentary lifestyle. Natural DNA insecticides act via DNA containment mechanism blocking expression of mRNAs and non-coding RNAs that have complementary sequences to them. It is amazing that ‘genetic zipper’ method, elaborated by scientists in the lab to benefit of mankind, has its previously unknown and strikingly similar analogue in nature. At the same time, there is no example in nature of insect pest control using plant dsRNA, at least none is known until now.
1.7 RNA biocontrols (double-stranded RNA technology)
In 1998, A. Fire and C. Mello discovered that the main antiviral immune system of the roundworm Caenorhabditis elegans is a mechanism of post-transcriptional gene silencing, known today as RNA interference (RNAi) (Fire et al., 1998). RNA interference is a mechanism for post-transcriptional gene silencing using a double-stranded RNA copy (usually >200 bp) of the target gene (Tomoyasu et al., 2008; Svoboda, 2020).
Recent studies on plant protection by RNAi have shown that in insects such as the tephritid fruit fly (Li et al., 2011) and the beet armyworm (Tian et al., 2009), feeding dsRNA produced in bacteria causes effective knockdown of target genes and mortality. dsRNA produced by yeast (Murphy et al., 2016) and algae (Fei et al., 2020; Fei et al., 2021; Fei et al., 2023) has also been reported to trigger RNAi in mosquitoes. Calantha™ is the first dsRNA biopesticide and currently the only one that was commercially available by GreenLight Biosciences in 2023. Calantha™ is a sprayable double-stranded ribonucleic acid (dsRNA) product that targets CPB (Rodrigues et al., 2021; Pallis et al., 2023; GreenLight Biosciences, 2023).
dsRNA is degraded quickly both in soil and in the aquatic environment (Dubelman et al., 2014; Bachman et al., 2020; Mogilicherla and Roy, 2023). For example, Fischer et al. (2017), demonstrated that unformulated dsRNA generally degrades quickly once in aquatic systems due to microbial activity and hydrolysis. Within the aquatic study performed by Fischer et al. (2017) the authors note that the degradation time for 50% (DT50) and 90% (DT90) values for aquatic system are less than 3 days and 4 days, respectively. The aerobic aquatic degradation study for Calantha™ calculated a DT90 of 6.18 and 4.2 days and DT50 values of 1.87 and 1.27 days for two representative aquatic environments (Fischer et al., 2016). The aerobic presentative agricultural soils degradation study for Calantha™ showed that degraded 80% dsRNA of the initial concentration for all soils tested (EPA, 2023). Rapid degradation is possible due to microbial nucleases and ultraviolet radiation present in the soil and on the leaves. Moreover, runoff from dew and rain can also significantly limit the availability of dsRNA for pests (Bachman et al., 2020; Parker et al., 2019). For example, Staphylococcus aureus uses environmental RNA as a building material in specific polysaccharide-dependent biofilms (Chiba et al., 2022); Streptococcus pneumoniae have extracellular nuclease EndA which degrading both DNA and RNA (Gonzalez and Hernandez, 2022); S. pyogenes can destroy both ssDNA and dsDNA, as well as RNA, due to the extracellular nucleases they actively produce (Remmington and Turner, 2018).
Studies of Spodoptera litura nuclease activity has shown that naked dsRNA (414 bp in the final concentration 0.05 μg/μL) is degraded by serum nucleases in 30 min, and saliva nucleases almost completely degrade dsRNA in 2 h (Peng et al., 2018). A high rate of dsRNA degradation (within 2–3 h) was also observed in the aphid Acyrthosiphon pisum (Christiaens et al., 2014) and the tobacco hornworm M. sexta (Garbutt et al., 2013). Scientists are trying to solve this problem with the help of improvements and recent discoveries in the field of chemistry and nanotechnology to hinder dsRNA biodegradation by non-specific RNases in insect cells (for example, use of dsRNA-protective agents, surfactants and diluents and nanoparticle) (Lucena-Leandro et al., 2022; Ma et al., 2024) (Figure 2). Unfortunately, such decisions can lead to more unexpected negative consequences for the environment. Some researchers showed that co-silencing of gene ZcdsRNase1 (dsRNase) along with gene ZcCOPI-alpha which plays a major role in insect development and survival, improved RNAi efficacy in Zeugodaus cucurbitae (led to 84% larval mortality) (Ahmad et al., 2024). A critical issue in assessing dsRNA’s persistence in environmental samples is the method of dsRNA detection. So far, the methods employed are based on probe assays (Quantigene) which are not very sensitive or accurate. More sensitive methods such as RT-qPCR for dsRNA detection coupled with small RNA sequencing for the detection of small RNAs (around 20 nt) which may accumulate as intermediate degradation products upon dsRNA degradation (but remain as such undetectable by conventional dsRNA detection methods while retaining their biological potential for RNAi) need to be developed for this purpose (Dalakouras et al., 2024).
There is a general expectation that by careful selection of dsRNA length and sequence (Chen et al.), RNAi can be limited to target insect pests without any direct harmful effects on non-target species, including beneficial insects such as pollinators and biological control agents (Cagliari et al., 2019; Zotti et al., 2018). However, the expectation that dsRNA will have high specificity can be refuted by two arguments (Arora et al., 2021). First, RNAi susceptibility to heterologous dsRNA can be obtained with a single matching 21-mer sequence, require several or many matching 21-mers, be achieved with as few as 15 contiguously matching bases and be tolerant of some sequence mismatches that vary with species, gene and dsRNA concentration (Chen et al., 2021; Powell et al., 2017). Second, animals, including insects, can respond to dsRNA in a sequence-non-specific manner, i.e., independent of the sequence of the dsRNA. In particular, various studies have shown that dsRNA with no matching 15–21-mer sequences in an insect genome can have substantial effects on antiviral immunity, gene expression and performance in insects (Chen et al., 2021; Brutscher et al., 2017; Flenniken and Andino, 2013; Hirai et al., 2004; Chen and De Schutter, 2024; Joga et al., 2021). Moreover, Organisation for Economic Cooperation and Development has published two guideline documents on the biodegradability of dsRNA pesticides and their overall risk assessment on the environment (OECD, 2020) and human health (OECD, 2023). Besides off-target effects to non-target organisms and concerns for the environment, an unexplored risk of dsRNA pesticides is that they may lead to epigenetic effects in the crops of applications, with the induced epimutations being trans-generationally inheritable (Dalakouras and Papadopoulou, 2020; Dalakouras and Ganopoulos, 2021). Therefore, it is obvious that further research is needed in this direction.
1.7.1 Biosafety of CUAD and RNAi
The double-stranded RNA molecules, like single-stranded DNA, biodegrade very fast and do not require any additional measures. Minimalist formulation containing only water and a target sequence of nucleic acids, unmodified antisense DNA or dsRNA, will have minimal negative effect on environment because of ubiquitous nucleases. Obviously, additional substances may enhance efficiency of nucleic acid-based insecticides on resistant species but can also pose additional environmental risks. To date, dsRNA-based technology does not have an easy algorithm for creation insecticides providing selectivity in action and high efficiency like CUAD biotechnology does on several groups of pests. Compared to short antisense DNA, long and fragile dsRNAs are more unpredictable and complex that is why it is not easy to use them as selective and efficient practical tool for plant protection. In addition to better target specificity, antisense oligonucleotides are easier and cheaper to synthesize than siRNAs or dsRNAs, and in the human system, antisense oligonucleotides have been shown to have lower immunoreactivity, which is also of significant importance for potential applications in edible plants that could accumulate this kind of means of insect pest control. It is important to note, from the point of view of environmental risks, there are no findings in the numerous human clinical studies that prove, for example, genomic integration events attributable to the use of antisense oligonucleotides (Gruber et al., 2023).
1.7.2 Limitations and prospects of CUAD and RNAi in plant protection
At present, there are no absolute rules for improving dsRNA efficiency (Zhao et al., 2024). For dsRNA design, the three-dimensional structures of RNA molecules that are critical to their function should be considered. Despite decades of intense effort, few RNA structures are known, and predicting the structure of RNAs remains a great challenge (Townshend et al., 2021). Until today, DNA-programmable plant protection based on oligonucleotide pesticides is the only simple and successful approach for pest control based on nucleic acids. It is easy and efficient algorithm for plant protection at least against many sternorrhynchans and mites (Oberemok V. et al., 2024; Oberemok V. V. et al., 2024). Advances in the direction of CUAD biotechnology (‘genetic zipper’ method) will help to expand this approach on other groups of pests providing easy biodegradation for the pest control agents after efficient and selective action. Today, dsRNA insecticides are perceived as ‘difficult’ insecticides, whilst oligonucleotide insecticides (ssDNA insecticides) are considered as ‘easy’ insecticides. While dsRNA insecticides need easy and efficient algorithm and more groups of pests to show their effectiveness on, ssDNA insecticides require only the latter. Both technologies individually can become successful for insect pest control on distinct groups of pests and also can complement each other’s action in complex pest control formulations. In our opinion, in short-term perspective (5–10 years) insecticides based on CUAD and RNAi are supposed to occupy not more than 5% of global insecticide market but will help make a quantum leap towards eco-friendly plant protection.
2 Conclusion
It should be noted that in eukaryotic and prokaryotic cells, DNA and RNA consist about 1% of the mass. However, studies show that they do not accumulate in ecosystems and amount to only 1 millionth of the mass of soil or marine water. This suggests that nucleic acids do not accumulate in nature but are biodegraded to the simplest monomers and are then used to construct new sequences of DNA and RNA within living organisms. From this point of view, the use of DNA and RNA as insecticides fits into the framework of natural processes and is a safe strategy for controlling insect pests. It is obvious that the need to use insecticides will not decrease but will only increase against the backdrop of an increase in population and a reduction in areas for agriculture. Biodegradability, or in other words neutralization, of insect pest control agents introduced into ecosystems must be at a high level so as not to jeopardize non-target organisms and human health (Guerrero Ramírez et al., 2023). Back in the 20th century, the possibility of creating insecticides based on natural polymers (such as DNA and RNA) to control a specific insect pest with minimal negative effects on non-target organisms seemed fantastic. This was impossible either conceptually or economically. Today this is reality. Time will tell how far we can progress in this direction, but the prospect looks encouraging: natural molecules act on natural systems through natural mechanisms and are utilized by fast “biodegradation micro machines” (bacteria and fungi). The share of modern chemical insecticides (neonicotinoids, pyrethroids, diamides, organophosphates, etc.) will obviously decrease against the background of the expected increase in the popularity of insecticides based on CUAD biotechnology and double-stranded RNA technology. However, from the point of view of increasing the level of biodegradability of modern chemical insecticides, improvements are still possible by creating molecules with a biomimetic structure, as well as obtaining bacterial strains with a high potential for biodegradation of certain substances. Thus, it is obvious that all conventional classes of insecticides will continue to exist in the medium term (5–10 years), and one would like to hope that ways will be found for them to compete with insecticides based on DNA and RNA in the level of biodegradability. We are on the verge of creating such plant protection products, the effectiveness of which will be determined by their safety, and CUAD biotechnology and double-stranded RNA technology are on the way to show an eco-precedent.
Author contributions
VO: Conceptualization, Funding acquisition, Resources, Supervision, Validation, Writing–original draft, Writing–review and editing. KL: Writing–original draft, Writing–review and editing. OA: Writing–original draft, Writing–review and editing. NG: Supervision, Validation, Writing–original draft, Writing–review and editing.
Funding
The author(s) declare that financial support was received for the research, authorship, and/or publication of this article. This research results obtained within the framework of a state assignment V. I. Vernadsky Crimean Federal University for 2024 and the planning period of 2024–2026 No. FZEG-2024–0001.
Acknowledgments
We thank our many colleagues, too numerous to name, for the technical advances and lively discussions that have prompted us to write this review. We apologize to the many colleagues whose work has not been cited. We are very much indebted to all reviewers and our colleagues from the Lab on DNA technologies, PCR analysis and creation of DNA insecticides (V.I. Vernadsky Crimean Federal University, Institute of Biochemical Technologies, Ecology and Pharmacy, Department of General Biology and Genetics), and OLINSCIDE BIOTECH LLC. for valuable comments on our manuscript.
Conflict of interest
The authors declare that the research was conducted in the absence of any commercial or financial relationships that could be construed as a potential conflict of interest.
Publisher’s note
All claims expressed in this article are solely those of the authors and do not necessarily represent those of their affiliated organizations, or those of the publisher, the editors and the reviewers. Any product that may be evaluated in this article, or claim that may be made by its manufacturer, is not guaranteed or endorsed by the publisher.
References
Abd El-Moneim Ibrahim, K., Mohamed Abdelrahman, S., K A Elhakim, H., and Ali Ragab, E. (2020). Single or combined exposure to chlorpyrifos and cypermethrin provoke oxidative stress and downregulation in monoamine oxidase and acetylcholinesterase gene expression of the rat’s brain. Environ. Sci. Pollut. Res. 27, 12692–12703. doi:10.1007/s11356-020-07864-8
Abraham, J., and Silambarasan, S. (2014). Biomineralization and formulation of endosulfan degrading bacterial and fungal consortiums. Pestic. Biochem. Physiol. 116, 24–31. doi:10.1016/j.pestbp.2014.09.006
Abraham, J., and Silambarasan, S. (2016). Biodegradation of chlorpyrifos and its hydrolysis product 3,5,6-trichloro-2-pyridinol using a novel bacterium Ochrobactrum sp. JAS2: a proposal of its metabolic pathway. Pestic. Biochem. Physiol. 126, 13–21. doi:10.1016/j.pestbp.2015.07.001
Ahamad, A., and Kumar, J. (2023a). Pyrethroid pesticides: an overview on classification, toxicological assessment and monitoring. J. Hazard. Mater. Adv. 10, 100284. doi:10.1016/j.hazadv.2023.100284
Ahamad, A., and Kumar, J. (2023b). Pyrethroid pesticides: an overview on classification, toxicological assessment and monitoring. J. Hazard. Mater. Adv. 10, 100284. doi:10.1016/j.hazadv.2023.100284
Ahmed, N., Saeed, M., Ullah, H., Iqbal, T., Al-Mutairi, K. A., Shahjeer, K., et al. (2021) “Botanical insecticides are a non-toxic alternative to conventional pesticides in the control of insects and pests,” in Global decline of insects. Intech Open. doi:10.5772/intechopen.100416
Ahmad, S., Jamil, M., Jaworski, C. C., and Luo, Y. (2024). Double-stranded RNA degrading nuclease affects RNAi efficiency in the melon fly, Zeugodacus cucurbitae. J. Pest Sci. 97, 397–409. doi:10.1007/s10340-023-01637-1
Akbar, S., and Sultan, S. (2016). Soil bacteria showing a potential of chlorpyrifos degradation and plant growth enhancement. Braz J. Microbiol. 47 (3), 563–570. doi:10.1016/j.bjm.2016.04.009
Akbar, S., Sultan, S., and Kertesz, M. (2015). Bacterial community analysis of cypermethrin enrichment cultures and bioremediation of cypermethrin contaminated soils. J. Basic Microbiol. 55, 819–829. doi:10.1002/jobm.201400805
Allan, E. A., Zhang, W. G., Lavery, A. C., and Govindarajan, A. F. (2020). Environmental DNA shedding and decay rates from diverse animal forms and thermal regimes. Environ. DNA. 3, 492–514. doi:10.1002/edn3.141
Alonso, M. B., Feo, M. L., Corcellas, C., Vidal, L. G., Bertozzi, C. P., Marigo, J., et al. (2012). Pyrethroids: a new threat to marine mammals? Environ. Int. 47, 99–106. doi:10.1016/j.envint.2012.06.010
Anderson, J. F., and Cowles, R. S. (2012). Susceptibility of Cimex lectularius (Hemiptera: cimicidae) to pyrethroid insecticides and to insecticidal dusts with or without pyrethroid insecticides. J. Econ. Entomology 105 (5), 1789–1795. doi:10.1603/EC12089
Aranda, J., Cerqueira, N. M. F. S. A., Fernandes, P. A., Roca, M., Tuñon, I., and Ramos, M. J. (2014). The catalytic mechanism of carboxylesterases: a computational study. Biochemistry 53, 5820–5829. doi:10.1021/bi500934j
Araújo, M. F., Castanheira, E. M. S., and Sousa, S. F. (2023). The buzz on insecticides: a review of uses, molecular structures, targets, adverse effects, and alternatives. Molecules 28, 3641. doi:10.3390/molecules28083641
Arora, A. K., Chung, S. H., and Douglas, A. E. (2021). Non-target effects of dsRNA molecules in Hemipteran insects. Genes 12, 407. doi:10.3390/genes12030407
Arthur, F. H., Ghimire, M. N., Myers, S. W., and Phillips, T. W. (2018). Evaluation of pyrethroid insecticides and insect growth regulators applied to different surfaces for control of trogoderm тgranarium (Coleoptera: dermestidae) the khapra beetle. J. Econ. Entomology 111 (2), 612–619. doi:10.1093/jee/toy040
Athanassiou, C. G., Kavallieratos, N. G., Boukouvala, M. C., Mavroforos, M. E., and Kontodimas, D. C. (2015). Efficacy of alpha-cypermethrin and thiamethoxam against Trogoderma granarium everts (Coleoptera: dermestidae) and Tenebrio molitor L. (Coleoptera: tenebrionidae) on concrete. J. Stored Prod. Res. 62, 101–107. doi:10.1016/j.jspr.2015.04.003
Bachman, P., Fischer, J., Song, Z., Urbanczyk-Wochniak, E., and Watson, G. (2020). Environmental fate and dissipation of applied DsRNA in soil, aquatic systems, and plants. Front. Plant Sci. 11, 21. doi:10.3389/fpls.2020.00021
Banks, S. D., Murray, N., Wilder-Smith, A., and Logan, J. G. (2014). Insecticide-treated clothes for the control of vector-borne diseases: a review on effectiveness and safety. Med. Veter. Entomol. 28, 14–25. doi:10.1111/mve.12068
Barbosa, P. R. R., Oliveira, M. D., Barros, E. M., Michaud, J. P., and Torres, J. B. (2018). Differential impacts of six insecticides on a mealybug and its coccinellid predator. Ecotoxicol. Environ. Saf. 147, 963–971. doi:10.1016/j.ecoenv.2017.09.021
Barkoski, J. M., Philippat, C., Tancredi, D., Schmidt, R. J., Ozonoff, S., Barr, D. B., et al. (2021). In utero pyrethroid pesticide exposure in relation to autism spectrum disorder (ASD) and other neurodevelopmental outcomes at 3 years in the MARBLES longitudinal cohort. Environ. Res. 194, 110495. doi:10.1016/j.envres.2020.110495
Barlow, S., Sullivan, F., and Lines, J. (2001). Risk assessment of the use of deltamethrin on bednets for the prevention of malaria. Food Chem. Toxicol. 39, 407–422. doi:10.1016/S0278-6915(00)00152-6
Barnes, M. A., and Turner, C. R. (2016). The ecology of environmental DNA and implications for conservation genetics. Conserv. Genet. 17, 1–17. doi:10.1007/s10592-015-0775-4
Bayoumi, E. A. (2022) “Deleterious effects of banned chemical pesticides on human health in developing countries,” in Pesticides - updates on toxicity, efficacy and risk assessment. IntechOpen. doi:10.5772/intechopen.104571
Berny, P., Vilagines, L., Cugnasse, J.-M., Mastain, O., Chollet, J.-Y., Joncour, G., et al. (2015). Vigilance poison: illegal poisoning and lead intoxication are the main factors affecting avian scavenger survival in the Pyrenees (France). Ecotoxicol. Environ. Saf. 118, 71–82. doi:10.1016/j.ecoenv.2015.04.003
Bhatt, P., Bhatt, K., Sharma, A., Zhang, W., Mishra, S., and Chen, S. (2021). Biotechnological basis of microbial consortia for the removal of pesticides from the environment. Crit. Rev. Biotechnol. 41, 317–338. doi:10.1080/07388551.2020.1853032
Bhatt, P., Huang, Y., Zhan, H., and Chen, S. (2019). Insight into microbial applications for the biodegradation of pyrethroid insecticides. Front. Microbiol. 10, 1778. doi:10.3389/fmicb.2019.01778
Bhatt, P., Rene, E. R., Kumar, A. J., Zhang, W., and Chen, S. (2020). Binding interaction of allethrin with esterase: bioremediation potential and mechanism. Bioresour. Technol. 315, 123845. doi:10.1016/j.biortech.2020.123845
Bilal, M., Iqbal, H. M., and Barceló, D. (2019). Persistence of pesticides-based contaminants in the environment and their effective degradation using laccase-assisted biocatalytic systems. Sci. Total Environ. 695, 133896. doi:10.1016/j.scitotenv.2019.133896
Bonmatin, J. M., Giorio, C., Girolami, V., Goulson, D., Kreutzweiser, D. P., Krupke, C., et al. (2015). Environmental fate and exposure; neonicotinoids and fipronil. Environ. Sci. Pollut. Res. 22 (1), 35–67. doi:10.1007/s11356-014-3332-7
Borsuah, J. F., Messer, T. L., Snow, D. D., Comfort, S. D., and Mittelstet, A. R. (2020). Literature review: global neonicotinoid insecticide occurrence in aquatic environments. Water 12, 3388. doi:10.3390/w12123388
Boucaud-Maitre, D., Rambourg, M. O., Sinno-Tellier, S., Puskarczyk, E., Pineau, X., Kammerer, M., et al. (2019). Human exposure to banned pesticides reported to the French Poison Control Centers: 2012-2016. Environ. Toxicol. Pharmacol. 69, 51–56. doi:10.1016/j.etap.2019.03.017
Bragança, I., Lemos, P. C., Delerue-Matos, C., and Domingues, V. F. (2019). Assessment of pyrethroid pesticides in topsoils in northern Portugal. Water Air Soil Pollut. 230, 166. doi:10.1007/s11270-019-4209-7
Brutscher, L. M., Daughenbaugh, K. F., and Flenniken, M. L. (2017). Virus and dsRNA-triggered transcriptional responses reveal key components of honey bee antiviral defense. Sci. Rep. 7 (3), 6448. doi:10.1038/s41598-017-06623-z
Budd, R., Wang, D., Ensminger, M., and Phillips, B. (2020). An evaluation of temporal and spatial trends of pyrethroid concentrations in California surface waters. Sci. Total Environ. 718, 137402. doi:10.1016/j.scitotenv.2020.137402
Buszewski, B., Bukowska, M., Ligor, M., and Staneczko-Baranowska, I. A. (2019). A holistic study of neonicotinoids neuroactive insecticides—properties, applications, occurrence, and analysis. Environ. Sci. Pollut. Res. Int. 26 (34), 34723–34740. doi:10.1007/s11356-019-06114-w
Cagliari, D., Dias, N. P., Galdeano, D. M., dos Santos, E. Á., Smagghe, G., and Zotti, M. J. (2019). Management of pest insects and plant diseases by non-transformative RNAi. Front. Plant Sci. 10, 1319. doi:10.3389/fpls.2019.01319
Cai, Z., Wang, J., Ma, J., Zhu, X., Cai, J., and Yang, G. (2015). Anaerobic degradation pathway of the novel chiral insecticide paichongding and its impact on bacterial communities in soils. J. Agric. Food Chem. 63 (32), 7151–7160. doi:10.1021/acs.jafc.5b02645
Carmichael, S. L., Yang, W., Roberts, E., Kegley, S. E., Padula, A. M., English, P. B., et al. (2014). Residential agricultural pesticide exposures and risk of selected congenital heart defects among offspring in the San Joaquin Valley of California. Environ. Res. 135, 133–138. doi:10.1016/j.envres.2014.08.030
Casida, J. E. (2018). Neonicotinoids and other insect nicotinic receptor competitive modulators: progress and prospects. Annu. Rev. Entomol. 63, 125–144. doi:10.1146/annurev-ento-020117-043042
Chen, J., Peng, Y., Zhang, H., Wang, K., Zhao, C., Zhu, G., et al. (2021). Off-target effects of RNAi correlate with the mismatch rate between dsRNA and non-target mRNA. RNA Biol. 18, 1747–1759. doi:10.1080/15476286.2020.1868680
Chen, S., Hu, M., Liu, J., Zhong, G., Yang, L., Rizwan-ul-Haq, M., et al. (2011a). Biodegradation of beta-cypermethrin and 3-phenoxybenzoic acid by a novel Ochrobactrum lupini DG-S-01. J. Hazard. Mater. 187, 433–440. doi:10.1016/j.jhazmat.2011.01.049
Chen, S., Hu, Q., Hu, M., Luo, J., Weng, Q., and Lai, K. (2011b). Isolation and characterization of a fungus able to degrade pyrethroids and 3-phenoxybenzaldehyde. Bioresour. Technol. 102, 8110–8116. doi:10.1016/j.biortech.2011.06.055
Chen, S., Luo, J., Hu, M., Geng, P., and Zhang, Y. (2012). Microbial detoxification of bifenthrin by a novel yeast and its potential for contaminated soils treatment. PLoS One 7, e30862. doi:10.1371/journal.pone.0030862
Chen, Y., and De Schutter, K. (2024). Biosafety aspects of RNAi-based pests control. Pest Manag. Sci. 80 (8), 3697–3706. doi:10.1002/ps.8098
Chen, Y., Shi, Y., Wang, Z., An, X., Wei, S., Andronis, C., et al. (2024). J. dsRNAEngineer: a web-based tool of comprehensive dsRNA design for pest control. bioRxiv. doi:10.1101/2024.07.22.604585
Chiba, A., Seki, M., Suzuki, Y., Kinjo, Y., Mizunoe, Y., and Sugimoto, S. (2022). Staphylococcus aureus utilizes environmental RNA as a building material in specific polysaccharide-dependent biofilms. npj Biofilms Microbiomes 8, 17. doi:10.1038/s41522-022-00278-z
Christiaens, O., Swevers, L., and Smagghe, G. (2014). DsRNA degradation in the pea aphid (Acyrthosiphon pisum) associated with lack of response in RNAi feeding and injection assay. Peptides 53, 307–314. doi:10.1016/j.peptides.2013.12.014
Chrustek, A., Hołyńska-Iwan, I., Dziembowska, I., Bogusiewicz, J., Wróblewski, M., Cwynar, A., et al. (2018). Current research on the safety of pyrethroids used as insecticides. Medicina 54, 61. doi:10.3390/medicina54040061
Cong, Y., Chen, J., Xie, Y., Wang, Y., and Cheng, C. (2023). Toxicity and sublethal effects of diamide insecticides on key non-target natural predators, the larvae of Coccinella septempunctata L. (Coleoptera: coccinellidae). Toxics 11 (3), 270. doi:10.3390/toxics11030270
Cremonese, C., Piccoli, C., Pasqualotto, F., Clapauch, R., Koifman, R., Koifman, S., et al. (2017). Occupational exposure to pesticides, reproductive hormone levels and sperm quality in young Brazilian men. Reprod. Toxicol. 67, 174–185. doi:10.1016/j.reprotox.2017.01.001
Cunningham, M. W. (2000). Pathogenesis of group A streptococcal infections. Clin. Microbiol. Rev. 13, 470–511. doi:10.1128/cmr.13.3.470
Cycoń, M., and Piotrowska-Seget, Z. (2016). Pyrethroid-degrading microorganisms and their potential for the bioremediation of contaminated soils: a review. Front. Microbiol. 7, 1463. doi:10.3389/fmicb.2016.01463
Dai, Z.-L., Yang, W.-L., Fan, Z.-X., Guo, L., Liu, Z.-H., and Dai, Y.-J. (2021). Actinomycetes Rhodococcus ruber CGMCC 17550 degrades neonicotinoid insecticide nitenpyram via a novel hydroxylation pathway and remediates nitenpyram in surface water. Chemosphere 270, 128670. doi:10.1016/j.chemosphere.2020.128670
Dalakouras, A., and Ganopoulos, I. (2021). Induction of promoter DNA methylation upon high-pressure spraying of double-stranded RNA in plants. Agronomy 11, 789. doi:10.3390/agronomy11040789
Dalakouras, A., Koidou, V., and Papadopoulou, K. (2024). DsRNA-based pesticides: considerations for efficiency and risk assessment. Chemosphere 352, 141530. doi:10.1016/j.chemosphere.2024.141530
Dalakouras, A., and Papadopoulou, K. K. (2020). Epigenetic modifications: an unexplored facet of exogenous RNA application in plants. Plants 9, 673. doi:10.3390/plants9060673
Damalas, C. A., and Eleftherohorinos, I. G. (2011). Pesticide exposure, safety issues, and risk assessment indicators. Int. J. Environ. Res. Public Health 8 (5), 1402–1419. doi:10.3390/ijerph8051402
Das, S. K., Mukherjee, I., and Das, S. K. (2012). Dissipation of flubendiamide in/on okra [Abelmoschus esculenta (L.) moench] fruits. Bull. Environ. Contam. Toxicol. 88, 381–384. doi:10.1007/s00128-011-0491-9
de Albuquerque, N. C. P., Carrão, D. B., Habenschus, M. D., Fonseca, F. S., Moreira da Silva, R., Lopes, N. P., et al. (2020). Risk assessment of the chiral pesticide fenamiphos in a human model: cytochrome P450 phenotyping and inhibition studies. Food Chem. Toxicol. 146, 111826. doi:10.1016/j.fct.2020.111826
DeMars, C., Wang, R., Grieneisen, M. L., Steggall, J., and Zhang, M. (2021). Assessment of pyrethroid contamination and potential mitigation strategies in California Central Coast surface waters. J. Environ. Manag. 278, 111507. doi:10.1016/j.jenvman.2020.111507
Deng, F., Sun, J., Dou, R., Yu, X., Wei, Z., Yang, C., et al. (2020). Contamination of pyrethroids in agricultural soils from the yangtze river delta, China. Sci. Total Environ. 731, 139181. doi:10.1016/j.scitotenv.2020.139181
De Schutter, K., Taning, C. N. T., Van Daele, L., Van Damme, E. J. M., Dubruel, P., and Smagghe, G. (2022). RNAi-based biocontrol products: market status, regulatory aspects, and risk assessment. Front. Insect Sci. 1, 818037. doi:10.3389/finsc.2021.818037
Donley, N. (2019). The USA lags behind other agricultural nations in banning harmful pesticides. Environ. Health 18, 44. doi:10.1186/s12940-019-0488-0
Dubelman, S., Fischer, J., Zapata, F., Huizinga, K., Jiang, C., Uffman, J., et al. (2014). Environmental fate of double-stranded RNA in agricultural soils. PLoS ONE 9, e93155. doi:10.1371/journal.pone.0093155
EFSA (2014). Conclusion on the peer review of the pesticide risk assessment of the active substance cyantraniliprole. Eur. Food Saf. Auth. 12. doi:10.2903/j.efsa.2014.3814
El-Sheikh, E.-S., and Ashour, M.-B. (2021). Diamide insecticides: efficacy, toxicity and analytical methods for residue monitoring in food samples. Egypt. J. Chem. 0, 0. doi:10.21608/ejchem.2021.96445.4513
EPA (2023). Environmental risk assessment for a FIFRA section 3 registration of the new product GS2 formulation (Calantha) containing ledprona. Available at: https://www.regulations.gov/document/EPA-HQ-OPP-2021-0271-0006.
Fahmy, M. A., Salem, S. H., Qattan, S. Y. A., Abourehab, M. A. S., Ashkan, M. F., Al-Quwaie, D. A., et al. (2022). Biodegradation of chlorantraniliprole and flubendiamide by some bacterial strains isolated from different polluted sources. Processes 10, 2527. doi:10.3390/pr10122527
Farahy, O., Laghfiri, M., Bourioug, M., and Aleya, L. (2021). Overview of pesticide use in Moroccan apple orchards and its effects on the environment. Curr. Opin. Environ. Sci. Health 19, 100223. doi:10.1016/j.coesh.2020.10.011
Fareed, A., Zaffar, H., Rashid, A., Maroof Shah, M., and Naqvi, T. A. (2017). Biodegradation of N-methylated carbamates by free and immobilized cells of newly isolated strain Enterobacter cloacae strain TA7. Bioremediation J. 21 (3-4), 119–127. doi:10.1080/10889868.2017.1404964
Fei, X. W., Xiao, S., Huang, X. D., Li, Z. J., Li, X. H., He, C. H., et al. (2023). Control of Aedes mosquito populations using recombinant microalgae expressing short hairpin RNAs and their effect on plankton. PloS Negl. Trop. Dis. 17 (1), e0011109. doi:10.1371/journal.pntd.0011109
Fei, X. W., Zhang, Y., Ding, L. L., Li, Y. J., and Deng, X. D. (2020). Controlling the development of the dengue vector Aedes aegypti using HR3 RNAi transgenic Chlamydomonas. PloS One 15 (10), e0240223. doi:10.1371/journal.pone.0240223
Fei, X. W., Zhang, Y., Ding, L. L., Xiao, S., Xie, X. Q., Li, Y. J., et al. (2021). Development of an RNAi-based microalgal larvicide for the control of Aedes aуgypti. Parasites Vectors 14 (1), 387. doi:10.1186/s13071-021-04885-1
Fire, A., Xu, S., Montgomery, M. K., Kostas, S. A., Driver, S. E., and Mello, С. С. (1998). Potent and specific genetic interference by double-stranded RNA in Caenorhabditis elegans. Nature 391, 806–811. doi:10.1038/35888
Fischer, J. R., Zapata, F., Dubelman, S., Mueller, G. M., Uffman, J. P., Jiang, C., et al. (2016). Aquatic fate of double stranded RNA in sediment-water system following an over-water application. Environ. Toxicol. 36, 3. doi:10.1002/etc.3577
Flenniken, M. L., and Andino, R. (2013). Non-specific dsRNA-mediated antiviral response in the honey bee. PLoS ONE 8, e77263. doi:10.1371/journal.pone.0077263
Gajendiran, A., and Abraham, J. (2018). An overview of pyrethroid insecticides. Front. Biol. 13, 79–90. doi:10.1007/s11515-018-1489-z
Gal’chinsky, N. V., Useinov, R. Z., Yatskova, E. V., Laikova, K. V., Novikov, I. A., Gorlov, M. V., et al. (2020). A breakthrough in the efficiency of contact DNA insecticides: rapid high mortality rates in the sap-sucking insects Dynaspidiotus britannicus Comstock and Unaspis euonymi Newstead. J. Plant Prot. Res 60 (2), 220–223. doi:10.24425/jppr.2020.133315
Gal’chinsky, N. V., Yatskova, E. V., Novikov, I. A., Sharmagiy, A. K., Plugatar, Y. V., and Oberemok, V. V. (2024). Mixed insect pest populations of Diaspididae species under control of oligonucleotide insecticides: 3′-end nucleotide matters. Pesticide Biochem. Physiology 200, 105838. doi:10.1016/j.pestbp.2024.105838
Gal’chinsky, N. V., Yatskova, E. V., Novikov, I. A., Useinov, R. Z., Kouakou, N. J., Kouame, K. F., et al. (2023). Icerya purchasi maskell (Hemiptera: monophlebidae) control using low carbon footprint oligonucleotide insecticides. Int. J. MolSci 24 (14), 11650. doi:10.3390/ijms241411650
Guo, Y., Ji, N., Bai, L., Ma, J., and Li, Z. (2022). Aphid viruses: a brief view of a long history. Front. Insect. Sci. 2, 846716. doi:10.3389/finsc.2022.846716
Galil, F. M. A. A., Zambare, S. P., Al-Mekhlafi, F. A., Al-Keridis, L. A., and Al-Khalifa, M. S. (2021). Effects of insecticide dimethoate on the developmental rate of forensic importance sarcophagid flies. J. King Saud Univ. – Sci. 33 (2), 101349. doi:10.1016/j.jksus.2021.101349
Gammon, D. W., Liu, Z., Chandrasekaran, A., El-Naggar, S. F., Kuryshev, Y. A., and Jackson, S. (2019). Pyrethroid neurotoxicity studies with bifenthrin indicate a mixed Type I/II mode of action. Pest Manag. Sci. 75 (4), 1190–1197. doi:10.1002/ps.5300
Garbutt, J. S., Bellés, X., Richards, E. H., and Reynolds, S. E. (2013). Persistence of double-stranded RNA in insect hemolymph as a potential determiner of RNA interference success: evidence from Manduca sexta and Blattella germanica. J. Insect Physiol. 59, 171–178. doi:10.1016/j.jinsphys.2012.05.013
Gasmi, S. (2020). Neurotransmission dysfunction by mixture of pesticides and preventive effects of quercetin on brain, hippocampus and striatum in rats. Toxicol. Environ. Health Sci. 12, 203–212. doi:10.1007/s13530-020-00012-2
Gautam, P., and Dubey, S. K. (2023). Biodegradation of neonicotinoids: current trends and future prospects. Curr. Pollut. Rep. 9, 410–432. doi:10.1007/s40726-023-00265-8
Geissen, V., Mol, H., Klumpp, E., Umlauf, G., Nadal, M., van der Ploeg, M., et al. (2015). Emerging pollutants in the environment: a challenge for water resource management. Int. Soil Water Conse 3, 57–65. doi:10.1016/j.iswcr.2015.03.002
Gonzalez, J. G., and Hernandez, F. J. (2022). Nuclease activity: an exploitable biomarker in bacterial infections. Expert Rev. Mol. Diagnostics 22 (3), 265–294. doi:10.1080/14737159.2022.2049249
Goulson, D.REVIEW (2013). REVIEW: an overview of the environmental risks posed by neonicotinoid insecticides. J. Appl. Ecol. 50 (4), 977–987. doi:10.1111/1365-2664.12111
GreenLight Biosciences (2023). Available at: https://www.greenlightbiosciences.com/international-crop-network-validates-ledprona-as-a-new-mode-of-action-group/
Gruber, C., Gursinsky, T., Gago-Zachert, S., Pantaleo, V., and Behrens, S. E. (2023). Effective antiviral application of antisense in plants by exploiting accessible sites in the target RNA. Int. J. Mol. Sci. 24 (24), 17153. doi:10.3390/ijms242417153
Guerrero Ramírez, J. R., Ibarra Muñoz, L. A., Balagurusamy, N., Frías Ramírez, J. E., Alfaro Hernández, L., and Carrillo Campos, J. (2023). Microbiology and biochemistry of pesticides biodegradation. Int. J. Mol. Sci. 24 (21), 15969. doi:10.3390/ijms242115969
Guo, L., Dai, Z., Guo, J., Yang, W., Ge, F., and Dai, Y. (2020). Oligotrophic bacterium Hymenobacter latericoloratus CGMCC 16346 degrades the neonicotinoid imidacloprid in surface water. Amb. Express 10 (7), 7. doi:10.1186/s13568-019-0942-y
Hadnagy, W., Leng, G., Sugiri, D., Ranft, U., and Idel, H. (2003). Pyrethroids used indoors–immune status of humans exposed to pyrethroids following a pest control operation a one year follow-up study. Inter. J. hygie. Environ. heal. 206 (2), 93–102. doi:10.1078/1438-4639-00201
Hamaguchi, H., and Hirooka, T. (2012). Insecticides affecting calcium homeostasis – flubendiamide, in Modern crop protection compounds. 2nd edn, ed. by W. Kramer, U. Schirmer, P. Jeschke, and M. Witschel Wiley VCH, Weinheim, Germany, 1396–1409.
Hao, X., Zhang, X., Duan, B., Huo, S., Lin, W., Xia, X., et al. (2018). Screening and genome sequencing of deltamethrin-degrading bacterium ZJ6. Curr. Microbiol. 75 (11), 1468–1476. doi:10.1007/s00284-018-1546-5
Helal, I. M., and Abo-El-Seoud, M. A. (2015). “Fungal biodegradation of pesticide vydate insoil and aquatic system,” in 4th international conference on radiation Sciences and applications (Egypt: Taba), 87–94.
Hertz-Picciotto, I., Sass, J. B., Engel, S., Bennett, D. H., Bradman, A., Eskenazi, B., et al. (2018). Organophosphate exposures during pregnancy and child neurodevelopment: recommendations for essential policy reforms. PLOS Med. 15 (10), e1002671. doi:10.1371/journal.pmed.1002671
Hirai, M., Terenius, O., Li, W., and Faye, I. (2004). Baculovirus and dsRNA induce Hemolin, but no antibacterial activity, in Antheraea pernyi. Insect Mol. Biol. 13, 399–405. doi:10.1111/j.0962-1075.2004.00497.x
Hodoșan, C., Gîrd, C. E., Ghica, M. V., Dinu-Pîrvu, C.-E., Nistor, L., Bărbuică, I. S., et al. (2023). Pyrethrins and pyrethroids: a comprehensive review of natural occurring compounds and their synthetic derivatives. Plants 12, 4022. doi:10.3390/plants12234022
Hołyńska-Iwan, I., and Szewczyk-Golec, K. (2020). Pyrethroids: how they affect human and animal health? Med. Kaunas. 56 (11), 582. doi:10.3390/medicina56110582
Hu, K., Barbieri, M. V., López-García, E., Postigo, C., López de Alda, M., Caminal, G., et al. (2022). Fungal degradation of selected medium to highly polar pesticides by Trametes versicolor: kinetics, biodegradation pathways, and ecotoxicity of treated waters. Anal. Bioanal. Chem. 414 (1), 439–449. doi:10.1007/s00216-021-03267-x
Huang, Y., and Chen, S. (2022). “Pyrethroid-degrading microorganisms and their potential application for the bioremediation of contaminated environments,”. Enzymes for pollutant degradation. Microorganisms for sustainability. Editors S. I. Mulla, and R. N. Bharagava (Singapore: Springer), 30, 119–137. doi:10.1007/978-981-16-4574-7_6
Huang, Y., Chen, S.-F., Chen, W.-J., Zhu, X., Mishra, S., Bhatt, P., et al. (2023). Efficient biodegradation of multiple pyrethroid pesticides by Rhodococcus pyridinivorans strain Y6 and its degradation mechanism. Chem. Eng. J. 469, 143863. doi:10.1016/j.cej.2023.143863
Huang, Y., Xiao, L., Li, F., Xiao, M., Lin, D., Long, X., et al. (2018). Microbial degradation of pesticide residues and an emphasis on the degradation of cypermethrin and 3-phenoxy benzoic acid: a review. Molecules 23 (9), 2313. doi:10.3390/molecules23092313
Hussain, S., Hartley, C. J., Shettigar, M., and Pandey, G. (2016). Bacterial biodegradation of neonicotinoid pesticides in soil and water systems. FEMS Microbiol. Lett. 363 (23), fnw252. doi:10.1093/femsle/fnw252
Jadhav, S. S., and David, M. (2016). Biodegradation of flubendiamide by a newly isolated Chryseobacterium sp. strain SSJ1. 3 Biotech. 6, 31. doi:10.1007/s13205-015-0347-9
Jeschke, P., Nauen, R., Schindler, M., and Elbert, A. (2011). Overview of the status and global strategy for neonicotinoids. J. Agric. Food Chem. 59 (7), 2897–2908. doi:10.1021/jf101303g
Jiang, Y., Liao, Y., Si, C., Du, J., Xia, C., Wang, Y., et al. (2023). Oral administration of Bacillus cereus GW-01 alleviates the accumulation and detrimental effects of β-cypermethrin in mice. Chemosphere 312 (1), 137333. doi:10.1016/j.chemosphere.2022.137333
Joga, M. R., Mogilicherla, K., Smagghe, G., and Roy, A. (2021). RNA interference-based forest protection products (FPPs) against wood-boring Coleopterans: hope or hype? Front. Plant Sci. 12, 733608. doi:10.3389/fpls.2021.733608
Jurewicz, J., Radwan, M., Wielgomas, B., Sobala, W., Piskunowicz, M., Radwan, P., et al. (2015). The effect of environmental exposure to pyrethroids and DNA damage in human sperm. Syst. Biol. Reproductive Med. 61 (1), 37–43. doi:10.3109/19396368.2014.981886
Kadala, A., Charreton, M., Charnet, P., and Collet, C. (2019). Honey bees long-lasting locomotor deficits after exposure to the diamide chlorantraniliprole are accompanied by brain and muscular calcium channels alterations. Sci. Rep. 9, 2153. doi:10.1038/s41598-019-39193-3
Kadala, A., Charreton, M., and Collet, C. (2020). Flubendiamide, the first phthalic acid diamide insecticide, impairs neuronal calcium signalling in the honey bee’s antennae. J. Insect Physiology 125, 104086. doi:10.1016/j.jinsphys.2020.104086
Kalyabina, V. P., Esimbekova, E. N., Kopylova, K. V., and Kratasyuk, V. A. (2021). Pesticides: formulants, distribution pathways and effects on human health – a review. Toxicol. Rep. 8, 1179–1192. doi:10.1016/j.toxrep.2021.06.004
Kar, A., Mandal, K., and Singh, B. (2013). Environmental fate of chlorantraniliprole residues on cauliflower using QuEChERS technique. Environ. Monit. Assess. 185, 1255–1263. doi:10.1007/s10661-012-2629-6
Khan, D. A., Hashmi, I., Mahjabeen, W., and Naqvi, T. A. (2010). Monitoring health implications of pesticide exposure in factory workers in Pakistan. Environ. Monit. Assess. 168 (1–4), 231–240. doi:10.1007/s10661-009-1107-2
King, Q. (2022). The case for Pyrethroid pesticides, then and now. Available at: https://www.kingquenson.com/Industry_News/Pyrethroids.html-:∼:text=TheCaseforPyrethroids,ThenandNow&text=Pyrethroidsfirstmadetheirmark.
Krishnan, N., Jurenka, R. A., and Bradbury, S. P. (2021). Neonicotinoids can cause arrested pupalecdysis in Lepidoptera. Sci. Rep. 11, 15787. doi:10.1038/s41598-021-95284-0
Kundoo, A. A., Dar, S. A., Mushtaqm, M., Dar, M. S., Gul, S., Ali, M. T., et al. (2018). Role of neonicotinoids in insect pest management: a review. J. Entomol. Zool. 6, 333–339.
Kurwadkar, S., and Evans, A. (2016). Neonicotinoids: systemic insecticides and systematic failure. Bull. Environ. Contam. Toxicol. 97, 745–748. doi:10.1007/s00128-016-1968-3
Labrie, G., Gagnon, A. È., Vanasse, A., Latraverse, A., and Tremblay, G. (2020). Impacts of neonicotinoid seed treatments on soil-dwelling pest populations and agronomic parameters in corn and soybean in Quebec (Canada). PLoS One 15 (2), e0229136. doi:10.1371/journal.pone.0229136
Laskowski, D. A. (2002). Physical and chemical properties of pyrethroids. Rev. Environ. Contam. Toxicol. 174, 49–170. doi:10.1007/978-1-4757-4260-2_3
Li, A. J., Martinez-Moral, M.-P., and Kannan, K. (2020b). Variability in urinary neonicotinoid concentrations in single-spot and first-morning void and its association with oxidative stress markers. Environ. Int. 135, 105415. doi:10.1016/j.envint.2019.105415
Li, L., Hu, Z., Dai, T., Liu, P., Chen, C., Liu, P., et al. (2020a). Improved efficacy of neonicotinoid in tablet formulation on the control of tomato chlorosis virus by controlling the vector Bemisia tabaci. Phytopathol. Res. 2, 2. doi:10.1186/s42483-019-0044-4
Li, W., Zhang, Y., Jia, H. R., Zhou, W. W., LiB, T., and Huang, H. J. (2019a). Residue analysis of tetraniliprole in rice and related environmental samples by HPLC/MS. Microchem. J. 150, 104168. doi:10.1016/j.microc.2019.104168
Li, W. G., Huang, D. Y., Chen, D., Wang, C., and Wei, G. L. (2019b). Temporal-spatial distribution of synthetic pyrethroids in overlying water and surface sediments in Guangzhou waterways: potential input mechanisms and ecological risk to aquatic systems. Environ. Sci. Pollut. Res. Int. 26 (17), 17261–17276. doi:10.1007/s11356-019-05013-4
Li, X., Schuler, M. A., and Berenbaum, M. R. (2007). Molecular mechanisms of metabolic resistance to synthetic and natural xenobiotics. Annu. Rev. Ent 52 (1), 231–253. doi:10.1146/annurev.ento.51.110104.151104
Li, X. X., Zhang, M. Y., and Zhang, H. Y. (2011). RNA interference of four genes in adult bactrocera dorsalis by feeding their dsRNAs. PloS One 6 (3), e17788. doi:10.1371/journal.pone.0017788
Li, Y.-F., An, J.-J., Dang, Z.-H., Pan, W.-L., and Gao, Z.-L. (2018). Systemic control efficacy of neonicotinoids seeds dressing on English grain aphid (Hemiptera: aphididae). J. Asia-Pacific Entomology 21 (1), 430–435. doi:10.1016/j.aspen.2018.01.003
Lourthuraj, A. A., Hatshan, M. R., and Hussein, D. S. (2022). Biocatalytic degradation of organophosphate pesticide from the wastewater and hydrolytic enzyme properties of consortium isolated from the pesticide contaminated water. Environ. Res. 205, 112553. doi:10.1016/j.envres.2021.112553
Lucena-Leandro, V. S., Abreu, E. F. A., Vidal, L. A., Torres, C. R., Junqueira, C. I. C. V. F., Dantas, J., et al. (2022). Current scenario of exogenously induced RNAi for Lepidopteran agricultural pest control: from dsRNA design to topical application. Int. J. Mol. Sci. 23, 15836. doi:10.3390/ijms232415836
Luo, Y., and Zhang, M. (2011). Environmental modeling and exposure assessment of sediment-associated pyrethroids in an agricultural watershed. PLoS One 6, e15794. doi:10.1371/journal.pone.0015794
Ma, D., Yang, S., Jiang, J., Zhu, J., Li, B., Mu, W., et al. (2021). Toxicity, residue and risk assessment of tetraniliprole in soil-earthworm microcosms. Ecotoxicol. Environ. Saf. 213, 112061. doi:10.1016/j.ecoenv.2021.112061
Ma, Y.-F., Zhao, Y.-Q., Zhou, Y., Feng, H.-Y., Gong, L.-L., Zhang, M.-Q., et al. (2024). Nanoparticle-delivered RNAi-based pesticide target screening for the rice pest white-backed planthopper and risk assessment for a natural predator. Sci. Total Environ. 926, 171286. doi:10.1016/j.scitotenv.2024.171286
Malhotra, H., Kaur, S., and Phale, P. S. (2021). Conserved metabolic and evolutionary themes in microbial degradation of carbamate pesticides. Front. Microbiol. 12, 648868. doi:10.3389/fmicb.2021.648868
Mali, H., Shah, C., Patel, D. H., Trivedi, U., and Subramanian, R. B. (2022). Degradation insight of organophosphate pesticide chlorpyrifos through novel intermediate 2,6-dihydroxypyridine by Arthrobacter sp. HM01. Bioresour. Bioprocess. 9, 31. doi:10.1186/s40643-022-00515-5
Marfo, J. T., Fujioka, K., Ikenaka, Y., Nakayama, S. M. M., Mizukawa, H., Aoyama, Y., et al. (2015). Relationship between urinary N-Desmethyl-Acetamiprid and typical symptoms including neurological findings: a prevalence case-control study. PLoS ONE 10 (11), e0142172. doi:10.1371/journal.pone.0142172
Matsuda, K., Ihara, M., and Sattelle, D. B. (2020). Neonicotinoid insecticides: molecular targets, resistance, and toxicity. Annu. Rev. Pharmacol. Toxicol. 60, 241–255. doi:10.1146/annurev-pharmtox-010818-021747
Mauvisseau, Q., Harper, L. R., Sander, M., Hanner, R. H., Kleyer, H., and Deiner, K. (2022). The multiple states of en vironmental DNA and what is known about their persistence in aquatic environments. Environ. Sci. and Technol. 56 (9), 5322–5333. doi:10.1021/acs.est.1c07638
Meng, Wu., Guilong, Li., Xiaofen, C., Jia, L., Ming, L., Chunyu, J., et al. (2018). Rational dose of insecticide chlorantraniliprole displays a transient impact on the microbial metabolic functions and bacterial community in a silty-loam paddy soil. Sci. Total Environ. 616–617, 236–244. doi:10.1016/j.scitotenv.2017.11.012
Menger, J., Beauzay, P., Chirumamilla, A., Dierks, C., Gavloski, J., Glogoza, P., et al. (2020). Implementation of a diagnostic-concentration bioassay for detection of susceptibility to pyrethroids in soybean aphid (Hemiptera: aphididae). J. Econ. Entomology 113 (2), 932–939. doi:10.1093/jee/toz351
Miglani, R., Parveen, N., Kumar, A., Ansari, M. A., Khanna, S., Rawat, G., et al. (2022). Degradation of xenobiotic pollutants: an environmentally sustainable approach. Metabolites 12 (9), 818. doi:10.3390/metabo12090818
Mishra, S., Pang, S., Zhang, W., Lin, Z., Bhatt, P., and Chen, S. (2021). Insights into the microbial degradation and biochemical mechanisms of carbamates. Chemosphere 279, 130500. doi:10.1016/j.chemosphere.2021.130500
Mogilicherla, K., and Roy, A. (2023). RNAi-chitosan biopesticides for managing forest insect pests: an outlook. Front. For. Glob. Change 6, 219685. doi:10.3389/ffgc.2023.1219685
Moreno, S. C., Silvério, F. O., Picanço, M. C., Alvarenga, E. S., Pereira, R. R., Santana Júnior, P. A., et al. (2017). New pyrethroids for use against tuta absoluta (Lepidoptera: gelechiidae): their toxicity and control speed. J. Insect Sci. 17 (5), 99. doi:10.1093/jisesa/iex072
Mulcahy, H., Charron-Mazenod, L., and Lewenza, S. (2010). Pseudomonas aeruginosa produces an extracellular deoxyribonuclease that is required for utilization of DNA as a nutrient source. Environ. Microbiol. 12, 1621–1629. doi:10.1111/j.1462-2920.2010.02208.x
Mulla, S. I., Ameen, F., Tallur, P. N., Bharagava, R. N., Bangeppagari, M., Eqani, SAMAS., et al. (2017). Aerobic degradation of fenvalerate by a Gram-positive bacterium, Bacillus flexus strain XJU-4. 3 Biotech. 7 (5), 320. doi:10.1007/s13205-017-0957-5
Mulla, S. I., Ameen, F., Talwar, M. P., Akber, S. A. M., Eqani, S., Bharagava, R. N., et al. (2020). “Organophosphate pesticides: impact on environment, toxicity, and their degradation,” in Bioremediation of industrial waste for environmental safety. Editors G. Saxena, and R. Bharagava (Singapore: Springer). doi:10.1007/978-981-13-1891-7_13
Muller-Mohnssen, H., and Hahn, K. (1995). A new method for early detection of neurotoxic diseases (exemplified by pyrethroid poisoning). Gesundheitswesen 57, 214–222.
Mullié, W. C., Prakash, A., Müller, A., and Lazutkaite, E. (2023). Insecticide use against desert locust in the horn of africa 2019–2021 reveals a pressing need for change. Agronomy 13, 819. doi:10.3390/agronomy13030819
Murphy, K. A., Tabuloc, C. A., Cervantes, K. R., and Chiu, J. C. (2016). Ingestion of genetically modified yeast symbiont reduces fitness of an insect pest via RNA interference. Sci. Rep. 6, 22587. doi:10.1038/srep22587
Mustapha, M. U., Halimoon, N., Johar, W. L. W., and Shukor, M. Y. A. (2019). An overview on biodegradation of carbamate pesticides by soil bacteria. Sci. and Technol. 27 (2), 547–563.
Muth, F., and Leonard, A. S. (2019). A neonicotinoid pesticide impairs foraging, but not learning, in free-flying bumblebees. Sci. Rep. 9, 4764. doi:10.1038/s41598-019-39701-5
Nugnes, R., Russo, C., Orlo, E., Lavorgna, M., and Isidori, M. (2023). Imidacloprid: comparative toxicity, DNA damage, ROS production and risk assessment for aquatic non-target organisms. Environ. Pollut. 316 (2), 120682. doi:10.1016/j.envpol.2022.120682
Nyadar, P. M., Oberemok, V., Omelchenko, A., Kerimova, S., Seidosmanova, E., Krasnodubiets, A., et al. (2019). DNA insecticides: the effect of concentration on non-target plant organisms such as wheat (Triticum aestivum L.). J. Plant Prot. Res. 59 (1), 60–68. doi:10.24425/jppr.2019.126038
Oberemok, V., Laikova, K., and Gal'chinsky, N. (2024). Contact unmodified antisense DNA (CUAD) biotechnology: list of pest species successfully targeted by oligonucleotide insecticides. Front. Agron. 6. doi:10.3389/fagro.2024.1415314
Oberemok, V. V. (2008). Method of elimination of phyllophagousinsects from order Lepidoptera. UA Pat. 36445.
Oberemok, V. V., Gal’chinsky, N. V., Useinov, R. Z., Novikov, I. A., Puzanova, Y. V., Filatov, R. I., et al. (2023). Four most pathogenic superfamilies of insect pests of suborder Sternorrhyncha: invisible superplunderers of plant vitality. Insects 14, 462. doi:10.3390/insects14050462
Oberemok, V. V., Laikova, K. V., Andreeva, O. A., and Gal'chinsky, N. V. (2024). Oligonucleotide insecticides and RNA-based insecticides: 16 years of experience in contact using of the next generation pest control agents. J. Plant Dis. Prot. doi:10.1007/s41348-024-00949-3
Oberemok, V. V., Laikova, K. V., Gal'chinsky, N. V., Useinov, R. Z., Novikov, I. A., Temirova, Z. Z., et al. (2019). DNA insecticide developed from the Lymantria dispar 5.8S ribosomal RNA gene provides a novel biotechnology for plant protection. Sci. Rep. 9, 6197. doi:10.1038/s41598-019-42688-8
Oberemok, V. V., Laikova, K. V., Repetskaya, A. I., Kenyo, I. M., Gorlov, M. V., Kasich, I. N., et al. (2018). A half-century history of applications of antisense oligonucleotides in medicine, agriculture and forestry: we should continue the journey. Molecules 23, 1302. doi:10.3390/molecules23061302
Oberemok, V. V., Laikova, V. K., Zaitsev, S. A., Nyadar, M. P., Shumskykh, N. M., and Gninenko, I. Y. (2015). DNA insecticides based on iap3 gene fragments of cabbage looper and gypsy moth nuclear polyhedrosis viruses show selectivity for non-target insects. Arch. Biol. Sci. 67, 785–792. doi:10.2298/abs141230037o
Oberemok, V. V., Nyadar, P., Zaytsev, O., Levchenko, N., Shiyntum, H., and Omelchenko, O. (2013). Pioneer evaluation of the possible side effects of the DNA insecticides on wheat (Triticum aestivum L.). Int. J. Biochem. Biophys. 1, 57–63. doi:10.13189/ijbb.2013.010302
Oberemok, V. V., Useinov, R. Z., Skorokhod, O. A., Gal’chinsky, N. V., Novikov, I. A., Makalish, T. P., et al. (2022). Oligonucleotide insecticides for green agriculture: regulatory role of contact DNA in plant–insect interactions. Int. J. Mol. Sci. 23, 15681. doi:10.3390/ijms232415681
OECD. (2020). Available at: https://one.oecd.org/document/ENV/JM/MONO(2020)25/en/pdf.
OECD. (2023). Available at: https://one.oecd.org/document/ENV/CBC/MONO(2023)26/en/pdf.
Önder, S., and Huseyin, C. (2019). Investigation of susceptibility levels of Culex pipiens L. (Diptera: Culicidae) populations to synthetic pyrethroids in antalya province of Turkey. J. Arthropod Borne Dis. 13 (3), 243–258.
Orlu, E. (2014). Deltamethrin-induced alterations in sperm morphology and spermatogenesis impairment in adult Sprague-dawley rats. Res. J. App. Sci. Eng. Technol. 7 (11), 2324–2331. doi:10.19026/rjaset.7.532
Orsborne, J., Banks, S. D., Hendy, A., Gezan, S. A., Kaur, H., Wilder-Smith, A., et al. (2016). Personal protection of permethrin-treated clothing against Aedesa egypti, the vector of dengue and zika virus, in the laboratory. PLoS ONE 11, e0152805. doi:10.1371/journal.pone.0152805
Pallis, S., Alyokhin, A., Manley, B., Rodrigues, T., Barnes, E., and Narva, K. (2023). Effects of low doses of a novel dsRNA-based biopesticide (Calantha) on the Colorado potato beetle. J. Econ. Entomology 116 (2), 456–461. doi:10.1093/jee/toad034
Paramasivam, M., and Banerjee, H. (2012). Degradation dynamics of flubendiamide in different types of soils. Bull. Environ. Contam. Toxicol. 88 (4), 511–514. doi:10.1007/s00128-012-0552-8
Parker, K. M., Barragán Borrero, V., van Leeuwen, D. M., Lever, M. A., Mateescu, B., and Sander, M. (2019). Environmental fate of RNA interference pesticides: adsorption and degradation of double-stranded RNA molecules in agricultural soils. Environ. Sci. Technol. 53, 3027–3036. doi:10.1021/acs.est.8b05576
Pathak, V. M., Verma, V. K., Rawat, B. S., Kaur, B., Babu, N., Sharma, A., et al. (2022). Current status of pesticide effects on environment, human health and it’s eco-friendly management as bioremediation: a comprehensive review. Front. Microbiol. 13, 962619. doi:10.3389/fmicb.2022.962619
Patil, V., Jangra, S., and Ghosh, A. (2024). Advances in antisense oligo technology for sustainable crop protection. Crit. Rev. Plant Sci., 1–23. doi:10.1080/07352689.2024.2394001
Paul, B., Singh, S., Shankarganesh, K., and Khan, M. A. (2018). “Synthetic insecticides: the backbone of termite management,” in Termites and sustainable management. Sustainability in plant and crop protection. Editors M. Khan, and W. Ahmad (Cham: Springer). doi:10.1007/978-3-319-68726-1_11
Peng, Y., Wang, K., Fu, W., Sheng, C., and Han, Z. (2018). Biochemical comparison of dsRNA degrading nucleases in four different insects. Front. Physiol. 9, 624. doi:10.3389/fphys.2018.00624
Pereira Costa, E. S., Soares, M. A., Caldeira, Z. V., Von Dos Santos Veloso, R., da Silva, L. A., da Silva, D. J. H., et al. (2020). Selectivity of deltamethrin doses on palmistichuselaeisis (Hymenoptera: eulophidae) parasitizing Tenebrio molitor (Coleoptera: tenebrionidae). Sci. Rep. 10, 12395. doi:10.1038/s41598-020-69200-x
Powell, M., Pyati, P., Cao, M., Bell, H., Gatehouse, J. A., and Fitches, E. (2017). Insecticidal effects of dsRNA targeting the Diap1 gene in dipteran pests. Sci. Rep., 1–13. doi:10.1038/s41598-017-15534-y
Prusty, A. K., Meena, D. K., Mohapatra, S., Panikkar, P., Das, P., Gupta, S. K., et al. (2015). Synthetic pyrethroids (Type II) and freshwater fish culture: perils and mitigations. Int. Aquat. Res. 7, 163–191. doi:10.1007/s40071-015-0106-x
Puzanova, Y. V., Novikov, I. A., Bilyk, A. I., Sharmagiy, A. K., Plugatar, Y. V., and Oberemok, V. V. (2023). Perfect complementarity mechanism for aphid control: oligonucleotide insecticide macsan-11 selectively causes high mortality rate for Macrosiphoniella sanborni gillette. Int. J. Mol. Sci. 24 (14), 11690. doi:10.3390/ijms241411690
Qiao, Z. H., Zhang, F. W., Yao, X. F., Yu, H. Y., Sun, S. A., Li, X. D., et al. (2019). Growth DNA damage and biochemical toxicity ofcyantraniliprole in earthworms (Eisenia fetida). Chemosphere 236, 124328. doi:10.1016/j.chemosphere.2019.07.059
Rabelo, M. M., Paula-Moraes, S. V., Pereira, E. J. G., and Siegfried, B. D. (2020). Contrasting susceptibility of lepidopteran pests to diamide and pyrethroid insecticides in a region of overwintering and migratory intersection. Pest Manag. Sci. 76 (12), 4240–4247. doi:10.1002/ps.5984
Ranjkesh, M. R., Naghili, B., Goldust, M., and Rezaee, E. (2013). The efficacy of permethrin 5% vs. oral ivermectin for the treatment of scabies. Ann. Parasitol. 59, 189–194.
Rank, A. P., and Koch, A. (2021). Lab-to-Field transition of RNA spray applications – how far are we? Front. Plant Sci. 12, 755203. doi:10.3389/fpls.2021.755203
Reese, D. (2018). Hawaii becomes first state to ban popular pesticide. Available at: https://www.courthousenews.com/hawaii-becomes-first-state-to-ban-popular-pesticide/.
Remmington, A., and Turner, C. E. (2018). The DNases of pathogenic lancefield streptococci. Microbiology 164 (3), 242–250. doi:10.1099/mic.0.000612
Richardson, E. B., Troczka, B. J., Gutbrod, O., Davies, T. G. E., and Nauen, R. (2020). Diamide resistance: 10 years of lessons from lepidopteran pests. J. Pest Sci. 93, 911–928. doi:10.1007/s10340-020-01220-y
Rizwan, M., Afzal, M., Arshad, M., Rizwan, M., Mubeen, N., Muhammad Raza, A. B., et al. (2022). Susceptibility of six insecticides in brown planthopper, Nilaparvata lugens (Hemiptera: Delphacidae) in Kallar tract of Punjab, Pakistan; 2015-2019. Enfoque UTE 13 (4). doi:10.29019/enfoqueute.867
Rodrigues, T. B., Mishra, S. K., Sridharan, K., Barnes, E. R., Alyokhin, A., Tuttle, R., et al. (2021). First sprayable double-stranded RNA-based biopesticide product targets proteasome subunit beta type-5 in Colorado potato beetle (Leptinotarsa decemlineata). Front. Plant Sci. 12, 728652. doi:10.3389/fpls.2021.728652
Rodríguez, A., Castrejón-Godínez, M. L., Salazar-Bustamante, E., Gama-Martínez, Y., Sánchez-Salinas, E., Mussali-Galante, P., et al. (2020). Omics approaches to pesticide biodegradation. Curr. Microbiol. 77 (4), 545–563. doi:10.1007/s00284-020-01916-5
Rowland, M., Boko, P., Odjo, A., Asidi, A., Akogbeto, M., and N’Guessan, R. (2013). A new long-lasting indoor residual formulation of the organophosphate insecticide pirimiphos methyl for prolonged control of pyrethroid-resistant mosquitoes: an experimental hut trial in Benin. PLoS ONE 8 (7), e69516. doi:10.1371/journal.pone.0069516
Sabourmoghaddam, N., Zakaria, M. P., and Omar, D. (2015). Evidence for the microbial degradation of imidacloprid in soils of cameron highlands. J. Saudi Soc. Agr. Sci. 14, 182–188. doi:10.1016/j.jssas.2014.03.002
Sánchez-Bayo, F. (2021). Indirect effect of pesticides on insects and other arthropods. Toxics 9 (8), 177. doi:10.3390/toxics9080177
Sanhueza-Guevara, S., Neira-Osses, K., Rojas, C., Genevière, A., and Fernandez, C. (2018). Effects of three pesticides used to control sea lice on the early development of Choromytilus chorus, Sphaerechinus granularis, and Paracentrotus lividus. Lat. Am. J. Aquat. Res. 46 (5), 969–980. doi:10.3856/vol46-issue5-fulltext-10
Santamaría, E., and Marceló, C. (2019). Toxic activity of pyrethroids in Lutzomyia longipalpis (Diptera: psychodidae) from magdalena river basin, Colombia. Acta Biol. Colomb. 24 (2), 391–396. doi:10.15446/abc.v24n2.74570
Seo, A., Tohnishi, M., Nakao, H., Furuya, T., Kodama, H., Tsubata, K., et al. (2008). Flubendiamide, a new insecticide characterized by its novel chemistry and biology, in Pesticide chemistry. Crop protection, public health, environmental safety, ed. by H. Ohkawa, H. Miyagawa, and P. W. Lee Wiley VCH, Weinheim, Germany, pp. 127–135.
Shafer, T. J., Meyer, D. A., and Crofton, K. M. (2005). Developmental neurotoxicity of pyrethroid insecticides: critical review and future research needs. Environ. Health Perspect. 113, 123–136. doi:10.1289/ehp.7254
Sharma, S., Singh, B., and Gupta, V. (2014). Biodegradation of imidacloprid by consortium of two soil isolated Bacillus sp. Bull. Environ. Contam. Toxicol. 93, 637–642. doi:10.1007/s00128-014-1386-3
Shaw, G. M., Yang, W., Roberts, E., Kegley, S. E., Padula, A., English, P. B., et al. (2014). Early pregnancy agricultural pesticide exposures and risk of gastroschisis among offspring in the San Joaquin Valley of California. Birth Defects Res. A Clin. Mol. Teratol. 100 (9), 686–694. doi:10.1002/bdra.23263
Shi, X., Bi, H., Fu, H., Li, L., Liu, D., and Li, J. (2011). Effect of low-dose fenvalerate on semen quality capacitation in adult mice. Chin. Med. J. 124 (10), 1529–1533.
Singh, B. (2009). Organophosphorus-degrading bacteria: ecology and industrial applications. Nat. Rev. Microbiol. 7, 156–164. doi:10.1038/nrmicro2050
Singh, B. K., and Walker, A. (2006). Microbial degradation of organophosphorus compounds. FEMS Microbiol. Rev. 30 (3), 428–471. doi:10.1111/j.1574-6976.2006.00018.x
Siripattanakul-Ratpukdi, S., Vangnai, A. S., Sangthean, P., and Singkibut, S. (2015). Profenofos insecticide degradation by novel microbial consortium and isolates enriched from contaminated chili farm soil. Environ. Sci. Pollut. Res. Int. 22, 320–328. doi:10.1007/s11356-014-3354-1
Siuda, W., and Chróst, R. J. (2001). Utilization of selected dissolved organic phosphorus compounds by bacteria in lake water under non-limiting orthophosphate conditions. Pol. J. Environ. Stud. 10 (6), 475–483.
Smagghe, G., Deknopper, J., Meeus, I., and Mommaerts, V. (2013). Dietary chlorantraniliprole suppresses reproduction in worker bumblebees. Pest Manag. Sci. 69, 787–791. doi:10.1002/ps.3504
Sogorb, M. A., and Vilanova, E. (2002). Enzymes involved in the detoxification of organophosphorus, carbamate and pyrethroid insecticides through hydrolysis. Toxicol. Lett. 128, 215–228. doi:10.1016/S0378-4274(01)00543-4
Stará, J., and Kocourek, F. (2019). Cabbage stem flea beetle’s (Psylliodes chrysocephala L.) susceptibility to pyrethroids and tolerance to thiacloprid in the Czech Republic. PLOS ONE 14 (9), e0214702. doi:10.1371/journal.pone.0214702
Strouhova, A., Velisek, J., and Stara, A. (2023). Selected neonicotinoids and associated risk for aquatic organisms. Vet. Med. (Praha) 68 (8), 313–336. doi:10.17221/78/2023-VETMED
Sun, S., Fan, Z., Zhao, Y., Guo, L., and Dai, Y. (2018). A novel nutrient deprivation-induced neonicotinoid insecticide acetamiprid degradation by Ensifer adhaerens CGMCC 6315. J. Agric. Food Chem. 67 (1), 63–71. doi:10.1021/acs.jafc.8b06154
Svoboda, P. (2020). Key mechanistic principles and considerations concerning RNA interference. Front. Plant Sci. 11, 1237. doi:10.3389/fpls.2020.01237
Tang, J., Liu, B., Chen, T. T., Yao, K., Zeng, L., Zeng, C. Y., et al. (2018a). Screening of a beta-cypermethrin-degrading bacterial strain Brevibacillus parabrevis BCP-09 and its biochemical degradation pathway. Biodegradation 29 (6), 525–541. doi:10.1007/s10532-018-9850-0
Tang, J., Liu, B., Shi, Y., Zeng, C., Chen, T., Zeng, L., et al. (2018b). Isolation, identification, and fenvalerate-degrading potential of Bacillus licheniformis CY-012. Biotechnol. and Biotechnol. Equip. 32 (3), 574–582. doi:10.1080/13102818.2018.1438210
Teixeira, L. A., and Andaloro, J. T. (2013). Diamide insecticides: global efforts to address insect resistance stewardship challenges. Pesticide Biochem. Physiology 106 (3), 76–78. doi:10.1016/j.pestbp.2013.01.010
Thorp, H. H. (2000). The importance of being r: greater oxidative stability of RNA compared with DNA. Chem. Biol. 7 (2), 33–36. doi:10.1016/s1074-5521(00)00080-6
Tian, H. G., Peng, H., Yao, Q., Chen, H. X., Xie, Q., Tang, B., et al. (2009). Developmental control of a Lepidopteran pest Spodoptera exigua by ingestion of bacteria expressing dsRNA of a non-midgut gene. PloS One 4 (7), e6225. doi:10.1371/journal.pone.0006225
Tian, J. W., Long, X. F., Zhang, S., Qin, Q. M., Gan, L. Z., and Tian, Y. Q. (2018). Screening cyhalothrin degradation strains from locust epiphytic bacteria and studying Paracoccus acridae SCU-M53 cyhalothrin degradation process. Environ. Sci. Pollut. R. 25, 11505–11515. doi:10.1007/s11356-018-1410-y
Tien, C. J., Huang, H. J., and Chen, C. S. (2017). Accessing the carbofuran degradation ability of CulturesFrom natural river biofilms in different environments. CLEAN–Soil, Air, Water 45 (5), 1–10. doi:10.1002/clen.201600380
Tomoyasu, Y., Miller, S. C., Tomita, S., Schoppmeier, M., Grossmann, D., and Bucher, G. (2008). Exploring systemic RNA interference in insects: a genome-wide survey for RNAi genes in Tribolium. Genome Biol. 9 (1), R10. doi:10.1186/gb-2008-9-1-r10
Townshend, R. J. L., Eismann, S., Watkins, A. M., Rangan, R., Karelina, M., Das, R., et al. (2021). Geometric deep learning of RNA structure. Science 373, 1047–1051. doi:10.1126/science.abe5650
TriLink, B. T. (2024). Feasibility of antisense oligonucleotides as DNA insecticides. Available at: https://www.trilinkbiotech.com/blog/feasibility-of-antisense-oligonucleotides-as-dna-insecticides/.
Tyagi, H., and Prashar, P. (2015). Isolation and identification of cypermethrin degrading Serratia nematodiphila from cauliflower rhizosphere. Int. J. Pharm. Tech. Res. 7, 64–71.
Ufarté, L., Laville, E., Duquesne, S., Morgavi, D., Robe, P., Klopp, C., et al. (2017). Discovery of carbamate degrading enzymes by functional metagenomics. PLoS One 12 (12), e0189201. doi:10.1371/journal.pone.0189201
Useinov, R. Z., Gal'chinsky, N. V., Yatskova, E. V., Novikov, I. A., Puzanova, Y. V., Trikoz, N. N., et al. (2020). To bee or not to bee: creating DNA insecticides to replace non-selective organophosphate insecticides for use against the soft scale insect Ceroplastes japonicus Green. J. Plant Prot. Res. doi:10.24425/JPPR.2020.133956
Van Laerhoven, S. L. (2008). Blind validation of postmortem interval estimates using developmental rates of blow flies. Forensic. Sci. Int. 180 (2-3), 76–80. doi:10.1016/j.forsciint.2008.07.002
Vuong, A. M., Zhang, C., and Chen, A. (2022). Associations of neonicotinoids with insulin and glucose homeostasis parameters in US adults: NHANES 2015–2016. Chemosphere 286, 131642. doi:10.1016/j.chemosphere.2021.131642
Wang, X. W., and Blanc, S. (2021). Insect transmission of plant single-stranded DNA viruses. Annu. Rev. Entomol. 66, 389–405. doi:10.1146/annurev-ento-060920-094531
Wu, W., Shan, H. W., Li, J. M., Zhang, C. X., Chen, J. P., and Mao, Q. (2022). Roles of bacterial symbionts in transmission of plant virus by hemipteran vectors. Front. Microbiol. 13, 805352. doi:10.3389/fmicb.2022.805352
Wang, B., Ma, Y., Zhou, W., Zheng, J., Zhu, J., He, J., et al. (2011). Biodegradation of synthetic pyrethroids by Ochrobactrum tritici strain pyd-1. World J. Microbiol. Biotechnol. 27, 2315–2324. doi:10.1007/s11274-011-0698-2
Wang, G., Chen, X., Yue, W., Zhang, H., Li, F., and Xiong, M. (2013). Microbial degradation of acetamiprid by Ochrobactrum sp. D-12 isolated from contaminated soil. PLoS One 8 (12), e82603. doi:10.1371/journal.pone.0082603
Wang, T., Hu, C., Zhang, R., Sun, A., Li, D., and Shi, X. (2019). Mechanism study of cyfluthrin biodegradation by Photobacterium ganghwense with comparative metabolomics. Appl. Microbiol. Biotechnol. 103, 473–488. doi:10.1007/s00253-018-9458-7
Wei, J., Wang, X., Tu, C., Long, T., Bu, Y., Wang, H., et al. (2023). Remediation technologies for neonicotinoids in contaminated environments: current state and future prospects. Environ. Int. 178, 108044. doi:10.1016/j.envint.2023.108044
Wen, Y., Wang, Z., Gao, Y., Zhao, X., Gao, B., Zhang, Z., et al. (2019). Novel liquid chromatography–tandem mass spectrometry method for enantioseparation of tefluthrin via a box–behnken design and its stereoselective degradation in soil. J. Agric. Food Chem. 67, 11591–11597. doi:10.1021/acs.jafc.9b04888
WHO (2014). 2,2-Dimethylcyclopropane-Carboxylate and (R)-α-Cyano-3-Phenoxybenzyl-(1S,3S)-3-(2,2-Dichlorovinyl)2,2-Dimethylcyclopropane-Carboxylate. Geneva, Switzerland: World Health Organization.
WHO (2015). Permethrin (25:75 Cis:trans isomer ratio, nonracemic) 3-phenoxybenzyl (1rs,3rs;1rs,3sr)-3-(2,2dichlorovinyl) 2,2-dimethyl-cyclopropane carboxylate. Geneva, Switzerland: World Health Organization.
WHO (2016). Vector control operations framework for zika virus. Geneva, Switzerland: World Health Organization.
WHO (2017). α-Cyano-3-Phenoxybenzyl (1r,3r)-3-(2,2dibromovinyl)-2,2-dimethylcyclopropane carboxylate. Geneva, Switzerland: World Health Organization.
Wylie, B. J., Hauptman, M., Woolf, A. D., and Goldman, R. H. (2016). Insect repellants during pregnancy in the era of the zika virus. Obstet. Gynecol. 128, 1111–1115. doi:10.1097/AOG.0000000000001685
Xiao, W., Wang, H., Li, T., Zhu, Z., Zhang, J., He, Z., et al. (2013). Bioremediation of Cd and carbendazim co-contaminated soil by Cd-hyperaccumulator Sedum alfredii associated with carbendazim-degrading bacterial strains. Environ. Sci. Pollut. Res. 20, 380–389. doi:10.1007/s11356-012-0902-4
Yan, X., Jin, W., Wu, G., Jiang, W., Yang, Z., Ji, J., et al. (2018). Hydrolase CehA and monooxygenase CfdC are responsible for carbofuran degradation in sphingomonas sp. strain CDS-1. Appl. Environ. Microbiol. 84. doi:10.1128/aem.00805-18
Yang, W. (2011). Nucleases: diversity of structure, function and mechanism. Q. Rev. Biophys. 44 (1), 1–93. doi:10.1017/S0033583510000181
Yang, W., Carmichael, S. L., Roberts, E. M., Kegley, S. E., Padula, A. M., English, P. B., et al. (2014). Residential agricultural pesticide exposures and risk of neural tube defects and orofacial clefts among offspring in the San Joaquin Valley of California. Am. J. Epidemiol. 179, 740–748. doi:10.1093/aje/kwt324
Yoshida, M. (2014). “Cyantraniliprole,” in Pesticide residues in food—2013 part 2: toxicological evaluations (Geneva: World Health Organization).
Zaitsev, A., Omel'chenko, O., Nyadar, P., and Oberemok, V. (2015). Influence of DNA oligonucleotides used as insecticides on biochemical parameters of Quercus robur and Malus domestica. Bull. Transilvania Univ. Brasov. 8, 37–46.
Zamule, S. M., Dupre, C. E., Mendola, M. L., Widmer, J., Shebert, J. A., Roote, C. E., et al. (2021). Bioremediation potential of select bacterial species for the neonicotinoid insecticides, thiamethoxam and imidacloprid. Ecotoxicol. Environ. Saf. 209, 111814. doi:10.1016/j.ecoenv.2020.111814
Zee Van Der, R., Pisa, L., Andonov, S., Brodschneider, R., Charrière, J. D., Chlebo, R., et al. (2012). Managed honey bee colony losses in Canada, China, Europe, Israel and Turkey, for the winters of 2008 – 9 and 2009 – 10. J. Apic. Res. 51, 100–114. doi:10.3896/ibra.1.51.1.12
Zhang, C., Wang, S., and Yan, Y. (2011). Yan, Y. Isomerization and biodegradation of beta-cypermethrin by Pseudomonas aeruginosa CH7 with biosurfactant production. Bioresour. Technol. 102 (14), 7139–7146. doi:10.1016/j.biortech.2011.03.086
Zhang, H., Zhang, R., Zeng, X., Wang, X., Wang, D., Jia, H., et al. (2022b). Exposure to neonicotinoid insecticides and their characteristic metabolites: association with human liver cancer. Environ. Res. 208, 112703. doi:10.1016/j.envres.2022.112703
Zhang, X. L., Wang, X. G., Liu, Y. L., Fang, K., and Liu, T. (2020). Residue and toxicity of cyantraniliprole and its main metabolite J9Z38 in soil-earthworm microcosms. Chemosphere 249, 126479. doi:10.1016/j.chemosphere.2020.126479
Zhang, Z., Sun, P., Zhao, J., Zhang, H., Wang, X., Li, L., et al. (2022a). Design, synthesis and biological activity of diamide compounds based on 3-substituent of the pyrazole ring. Pest Manag. Sci. 78 (5), 2022–2033. doi:10.1002/ps.6826
Zhao, J., Jia, D., Du, J., Chi, Y., and Yao, K. (2019). Substrate regulation on co-metabolic degradation of beta-cypermethrin by Bacillus licheniformis B-1. Amb. Express 9, 83. doi:10.1186/s13568-019-0808-3
Zhao, J. H., Liu, Q. Y., Xie, Z. M., and Guo, H. S. (2024). Exploring the challenges of RNAi-based strategies for crop protection. Adv. Biotechnol. 2, 23. doi:10.1007/s44307-024-00031-x
Zhao, X., Xu, S., Liu, C., He, J., Li, C., Deng, Y., et al. (2020). Design, synthesis and insecticidal activity of novel analogues of flubendiamide containing alkoxyhexafluoroisopropyl groups. RSC Adv. 10 (57), 34486–34492. doi:10.1039/d0ra07121h
Zhou, Lu., Zhiguang, H., and Hongyu, P. (2022). Degradation of anthranilic diamide insecticide tetrachlorantraniliprole in water: kinetics, degradation pathways, product identification and toxicity assessment. Sci. Total Environ. 836, 155448. doi:10.1016/j.scitotenv.2022.155448
Keywords: chemical insecticides, oligonucleotide insecticides, contact unmodified antisense DNA (CUAD) biotechnology, RNA biocontrols, RNAi, biodegradability of insecticides
Citation: Oberemok VV, Laikova KV, Andreeva OA and Gal’chinsky NV (2024) Biodegradation of insecticides: oligonucleotide insecticides and double-stranded RNA biocontrols paving the way for eco-innovation. Front. Environ. Sci. 12:1430170. doi: 10.3389/fenvs.2024.1430170
Received: 13 June 2024; Accepted: 30 September 2024;
Published: 23 October 2024.
Edited by:
Joginder Singh, Nagaland University, IndiaReviewed by:
Athanasios Dalakouras, Hellenic Agricultural Organisation, GreeceAmit Roy, Czech University of Life Sciences Prague, Czechia
Copyright © 2024 Oberemok, Laikova, Andreeva and Gal’chinsky. This is an open-access article distributed under the terms of the Creative Commons Attribution License (CC BY). The use, distribution or reproduction in other forums is permitted, provided the original author(s) and the copyright owner(s) are credited and that the original publication in this journal is cited, in accordance with accepted academic practice. No use, distribution or reproduction is permitted which does not comply with these terms.
*Correspondence: Vol V. Oberemok, dm9sb2JlcmVtb2tAZ21haWwuY29t; Nikit V. Gal’chinsky, cGNyLnByb2R1Y3RAZ21haWwuY29t