- 1Institute of Hydrogeology and Environmental Geology, Chinese Academy of Geological Sciences, Shijiazhuang, China
- 2Key Laboratory of Groundwater Contamination and Remediation, Hebei Province and China Geological Survey, Shijiazhuang, China
- 3Water Environment Monitoring Center, Hebei Geological Environmental Monitoring Institute, Shijiazhuang, China
- 4School of Gemmology and Materials Science, Hebei GEO University, Shijiazhuang, China
Introduction: The Zhengzhou section of the lower Yellow River is an ecologically vulnerable region, where the groundwater chemistry has experienced significant variations due to the combined effects of natural factors and human activities.
Methods: In this study, we employed methods such as the Piper trilinear diagram, Gibbs plot, ion ratio coefficient method, and multivariate statistical techniques to explore the major controlling factors influencing groundwater chemical evolution.
Results: The results indicate that 46% of shallow groundwater in the intensive conversion zone between surface water and groundwater is of the HCO3—Na·Ca·Mg water type. Influenced by lateral infiltration from the Yellow River, groundwater on both sides of the river evolves into Cl·SO4·HCO3 and Cl·HCO3 types. Groundwater near the riverbanks and ponds, directly replenished by Yellow River water or by leakage, is more significantly impacted by human activities compared to other regions. Factor analysis indicates that the chemical evolution of regional shallow groundwater is primarily controlled by factors such as mineral dissolution, human activities, and Yellow River water recharge. More pronounced water-rock interactions in unconfined aquifers, leads to an increase in the concentrations of conventional components like TDS and Mg2+.
Discussion: Due to the long-term unidirectional transformation of surface water into groundwater, SO42- and Na+ in the groundwater are strongly influenced by surface water. NO3− is mainly affected by human activities, including domestic and agricultural activities. The concentrations of Fe and Mn are primarily influenced by the high background values of aquifer sediments and, to a lesser extent, by the significant enhancement of the aquifer’s reducing conditions, leading to higher concentrations of Fe and Mn in groundwater. The findings of this study provide theoretical and data-based support for the scientific formulation of groundwater protection and rational development measures.
1 Introduction
Ecological protection and high-quality development in the Yellow River basin have become major national strategies, and the downstream alluvial plain area is one of the most ecologically fragile zones in the basin. Due to the combined impacts of factors such as climate change (Marganingrum et al., 2023) and human activities (Zhou et al., 2025), the hydrochemical evolution of regional groundwater has undergone fundamental changes, leading to groundwater quality deterioration, water scarcity, and a series of ecological and environmental issues (Cao et al., 2022). Therefore, studying the hydrochemical characteristics and evolution mechanisms of groundwater is of great significance for water resource conservation, rational development and utilization, and ensuring ecological security (Zaryab et al., 2023; Chen et al., 2021).
The hydrochemical evolution of groundwater is typically studied using hydrochemical diagrams and multivariate statistical analysis (Zhai et al., 2024; Su et al., 2024). This evolution is mainly influenced by the combined effects of natural and anthropogenic factors. In the lower Yellow River, groundwater is affected by geological conditions, rock weathering, evaporation concentration, and water-rock interactions, leading to increased concentrations of total dissolved solids (TDS) and arsenic (As), among other elements (Chen et al., 2023; Ren et al., 2024). Human activities, such as industrial and agricultural practices, contribute to elevated concentrations of sodium (Na+) and nitrate (NO3−) (Meng et al., 2022). Lateral recharge from the Yellow River and irrigation from the river have caused increases in fluoride concentrations in groundwater (Wang et al., 2024; Wangs et al., 2025). Previous studies have mainly focused on qualitatively assessing the effects of natural and anthropogenic factors on groundwater chemistry. Consequently, increasing attention has been given to distinguishing the primary controlling factors of groundwater chemical composition and quantitatively assessing the contribution of each factor (Tu et al., 2023). The identification of groundwater chemical composition sources primarily involves methods such as chemical mass balance models and multivariate statistical models. The chemical mass balance method requires real-time monitoring of pollution sources in the study area and continuous updates of the source composition spectrum (Zhao et al., 2021), making it costly and highly susceptible to human influence. Traditional multivariate statistical methods, such as Principal Component Analysis (PCA) (Lv et al., 2021) and Cluster Analysis (CA) (Xia et al., 2021), cannot accurately quantify the contribution of pollution sources. The Absolute Principal Component Score-Multiple Linear Regression (APCS-MLR) method first applies PCA for dimensionality reduction to extract the main influencing factors of hydrochemical components, then calculates the absolute scores of each factor, and finally applies linear regression to calculate the contribution of each factor to water quality (Muangthong and Shrestha, 2015; Zhang et al., 2020). This approach, which relies on extensive water quality data, enables both qualitative identification and quantitative analysis of factors influencing hydrochemical evolution, making it increasingly applicable in groundwater and soil pollution studies (Li et al., 2021; Xu et al., 2022).
In the Zhengzhou section of the lower Yellow River, the riverbank floodplain is extensive with numerous villages scattered throughout, experiencing significant pollution from production and daily life activities. This floodplain serves as both a flood discharge zone for the Yellow River and essential land for residents’ production and daily life. Yellow River water infiltrates into the aquifer, carrying its hydrochemical components as well. Urban wastewater and infiltration from precipitation or surface water further complicate the hydrochemical evolution process of groundwater (Yuan et al., 2024; Chen et al., 2023). This study investigates the hydrochemical characteristics of groundwater in the lower Yellow River region near Zhengzhou, utilizing methods such as Piper trilinear diagrams, Gibbs plot, ion ratio analysis, and multivariate statistical techniques to further explain the main controlling factors of groundwater chemical evolution in the region. The results of this study provide theoretical and data-based support for the formulation of groundwater conservation and rational development measures, which are of great significance for the ecological preservation of the Yellow River Basin.
2 Materials and methods
2.1 Study area overview
This study focuses on the Yellow River floodplain in Zhengzhou (Figure 1). The study area has a temperate continental monsoon climate with distinct seasons. The average annual temperature is 15.4°C, and the average annual precipitation is 631.3 mm. Water resources are widely distributed in the region, with the Yellow River being the largest river and primary water source. Additionally, surface water bodies such as ponds and irrigation channels crisscross the area.
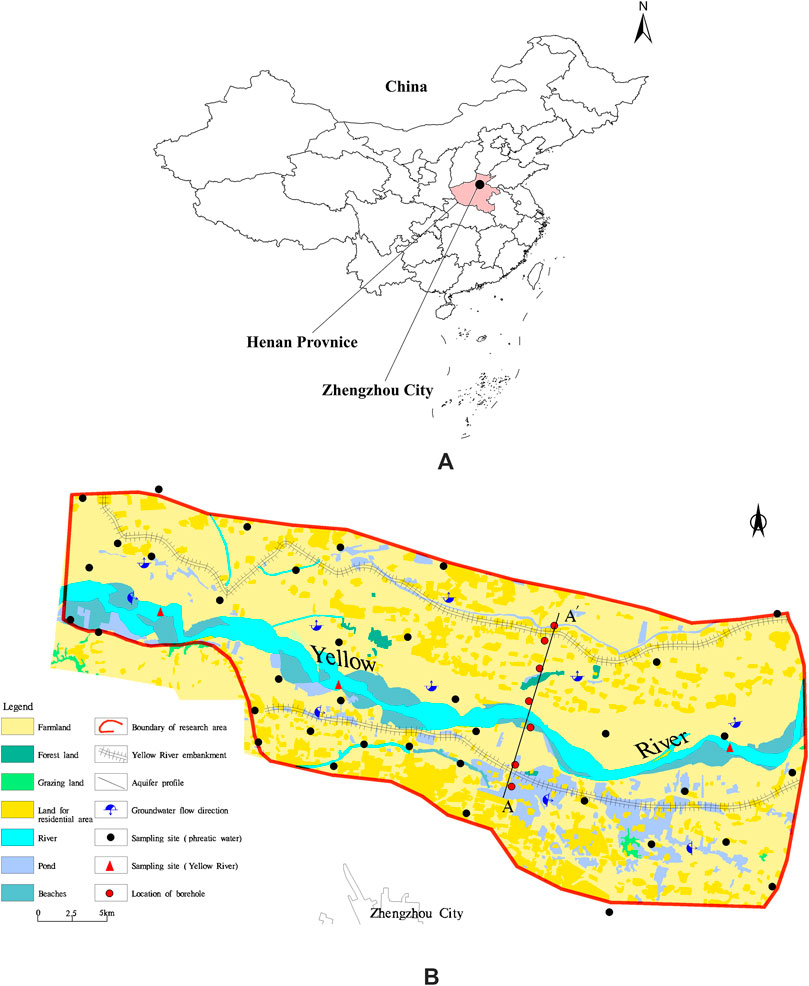
Figure 1. Distribution of sampling sites in the study area. (A): Geographical location of study area; (B): Spatial distribution of sampling sites in the study area.
The study area is part of the Yellow River alluvial plain, characterized by vast and flat terrain. The land inside the levee consists mainly of depositional landforms, with relatively flat floodplains. Due to artificial modifications and natural river evolution, the boundary between the high and low floodplains has become indistinct, with the terrain gently sloping towards the riverbed. The recent Yellow River alluvial deposits inside the levee consist of floodplain sediments, with the upper layer being composed of loose grayish-yellow silty clay interbedded with gray-black silt layers, while the lower layer consists of brownish yellow to light gray fine to medium-coarse sand interspersed with small gravel. These sediments are loose, water-saturated, and exhibit stable distribution patterns (Figure 2).
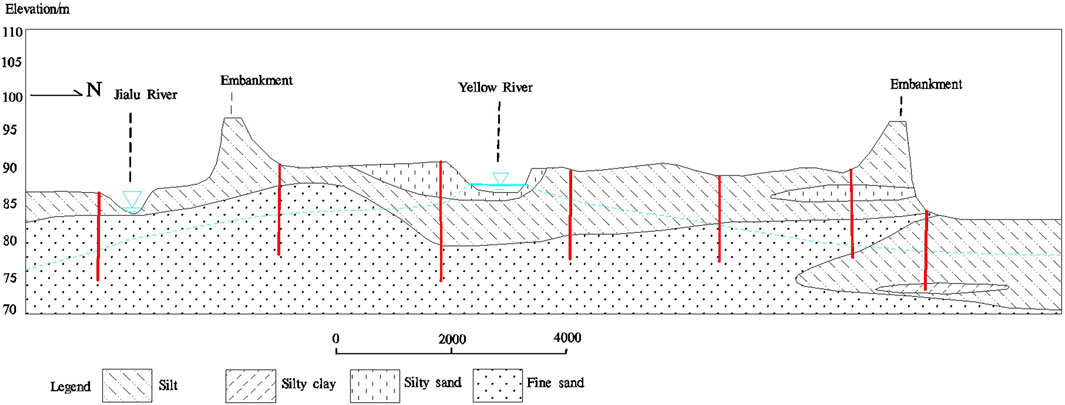
Figure 2. Hydrogeological profile (along line A-A′ in Figure 1) of the study area.
Groundwater in the study area is mainly unconfined pore water within loose rock formations, characterized by widely distributed aquifers with large thicknesses and abundant water resources. The primary recharge sources of shallow groundwater include atmospheric precipitation, lateral infiltration from the Yellow River, seepage from irrigation channels, and return flow from irrigation. Evaporation and extraction are the main discharge processes for groundwater. The flow of shallow groundwater is controlled by topography and recharge sources. As the Yellow River serves as a natural divide between surface water and groundwater, the groundwater flow direction south of the river gradually transitions from west to east, then southeastward.
2.2 Sample collection and testing
In June 2023, 30 shallow groundwater and 3 Yellow River water samples were collected from the Zhengzhou floodplain (Figure 1). The groundwater samples were primarily obtained from domestic wells and agricultural irrigation wells, with sampling depths ranging from 5 to 50 m. Before sampling, wells were pumped for approximately 30 min, and the sampling bottles were rinsed three times with the raw water sample. After collection, the samples were immediately preserved in a portable refrigerated storage box. The hydrochemical samples were sent to the Groundwater and Mineral Water Monitoring Center at the Institute of Hydrogeology and Environmental Geology, Chinese Academy of Geological Sciences, for testing. The test results underwent charge balance verification, with all relative error absolute values remaining within 5%.
2.3 Research methods
2.3.1 Contrast Coefficient variance
Variance is used to quantify the degree of dispersion of a random variable from its expected value (mean). Since the absolute values of different groundwater chemical components may vary significantly, direct variance comparison is not feasible. Therefore, the contrast coefficient (V) is defined as the ratio of each sample’s variable (Xi) to the average value of that variable (
The formula for calculating the contrast coefficient variance is as follows:
Where: Vi is the contrast coefficient for the ith chemical component of groundwater;
When
2.3.2 APCS-MLR analysis method
The APCS-MLR model is based on factor analysis (PCA) to derive the absolute principal component score (APCS) and uses multiple linear regression (MLR) to calculate the contribution of each factor to each sample. After standardizing the factor data, PCA is conducted to calculate factor scores. A zero-concentration sample is introduced and standardized for PCA to compute factor scores. The APCS value for each factor is obtained by subtracting the factor score of the zero-concentration sample from that of each actual sample. Using APCS as the independent variable, and the observed values for each factor as dependent variables, multiple linear regression analysis is performed. The regression coefficients allow conversion of APCS into the contribution of each factor to the sample’s chemical composition,as shown in Equations 2, 3.
The contribution formula for known sources to water quality is:
The contribution formula for unknown sources to water quality is:
Where: aim is the multiple regression coefficient for source m on indicator i; bi is the constant term in the multiple linear regression for the ith factor; APCSk is the absolute principal component score for factor k; aim × APCSim represents the contribution of source m to factor k.
The limitations of APCS-MLR mainly include the following aspects: 1. Subjectivity of parameter presetting: The APCS-MLR method requires presetting the number of principal components, which is subjective and may affect the accuracy of the results.2. Limitations of assumptions: This method assumes that pollution sources are independent, but there may be mutual influences between pollution sources, which may lead to inaccurate results.
3 Results
3.1 Main ion statistical characteristics
The statistical parameters of hydrochemistry for groundwater and surface water samples are presented in Table 1.
The pH values of groundwater range from 7.17 to 7.75, indicating weak alkalinity. The variation range of TDS and total hardness exhibit significant variation, ranging from 337 to 2,546 mg/L and 213 to 1,509 mg/L, respectively. The mean values are 716 mg/L and 453 mg/L, respectively, classifying the water as high-TDS and hard water. The mean TDS value is significantly higher than the global average of 100 mg/L, reflecting the intense water-rock interaction in the region. The major cations in groundwater are Ca2+, with concentrations ranging from 42.1 to 313 mg/L (mean: 100 mg/L), followed by Na+, with concentrations ranging from 28.3 to 306 mg/L (mean: 88.4 mg/L). The predominant anions are HCO3−, with concentrations ranging from 237 to 684 mg/L (mean: 449 mg/L), followed by SO42-, ranging from 6.88 to 312 mg/L (mean: 110 mg/L).
For surface water, the main cations are Na+, with concentrations ranging from 91.7 to 94.6 mg/L (mean: 93.1 mg/L), followed by Ca2+, ranging from 43.9 to 51.0 mg/L (mean: 48.3 mg/L). The main anions are HCO3−, with concentrations ranging from 216 to 230 mg/L (mean: 225 mg/L), followed by SO42-, ranging from 205 to 218 mg/L (mean: 211 mg/L).
The coefficient of variation (Cv) provides insight into the complexity of the factors influencing the formation and evolution of groundwater chemical components. Typically, Cv ≥ 1 indicates strong variability, 0.1 < Cv < 1 indicates moderate variability, and Cv ≤ 0.1 indicates weak variability. For the statistical parameters of groundwater, except for pH, which exhibits weak variability, all the other ion components display moderate variability. For surface water, in contrast, all statistical parameters exhibit weak variability.
3.2 Groundwater hydrochemical types
The Piper tri-linear diagram visually presents the major ion composition of groundwater and plays an important role in revealing the evolution of groundwater chemical components. According to the Shukarev classification method, the shallow groundwater in the study area is classified into nine types (Figure 3; Table 2). The HCO3—Na·Ca·Mg type accounts for the highest proportion (45.95%), followed by HCO3—Ca·Mg (13.51%) and Cl·SO4·HCO3—Na·Ca·Mg (13.51%). The Yellow River water is classified as Cl·SO4·HCO3—Na·Ca·Mg type.
3.3 Spatial characteristics of groundwater chemistry
The groundwater types in the study area are relatively stable (Figure 4). Among the anion types, groundwater is predominantly HCO3—type, which is widely distributed on both the northern and southern banks of the Yellow River in the area. The Cl·SO4 type is distributed on both sides of the north levee. The Cl·SO4·HCO3 type has a localized distribution, mainly occurring in the sections of the river adjacent to the central part of the Yellow River. The Cl·HCO3 and SO4·HCO3 types are sporadically distributed. In terms of cation composition, groundwater is primarily Na·Ca·Mg type. On both sides of the northern and southern levees of the Yellow River, Ca·Mg type water is present. The Na·Ca type is present on the wider side of the northern levees and the sections of the river adjacent to the central part of the Yellow River. Overall, the dominant groundwater type in the area is HCO3—Na·Ca·Mg.
4 Discussion
4.1 Analysis of groundwater hydrochemical characteristics
4.1.1 Rock weathering and leaching processes
The Gibbs diagram illustrates the intrinsic relationship between TDS, the cation ratio Na+/(Na++Ca2+), and the anion ratio Cl−/(Cl− + HCO3−), classifying the controlling factors of natural water chemistry into three categories: precipitation dominance, water-rock interaction dominance, and evaporation concentration dominance (Gibbs 1970).
According to the Gibbs diagram of karst groundwater in the study area (Figure 5), most samples are distributed between the rock weathering and evaporation zones. However, the Yellow River water in this area has been supplying groundwater for a long time, and the impact of Yellow River water on the chemical composition of groundwater cannot be ignored. Due to the replenishment from the Yellow River, the water table in this area is relatively shallow (3.4m–13.2m), evaporation serves as one of the main discharge mechanisms for shallow groundwater. Six shallow groundwater samples in the area have TDS values ranging from 1 to 3 g/L, categorized as slightly brackish water. These samples are mostly distributed near surface water bodies such as rivers and ponds, in low-lying areas where groundwater levels are shallow and strongly affected by evaporation and concentration.
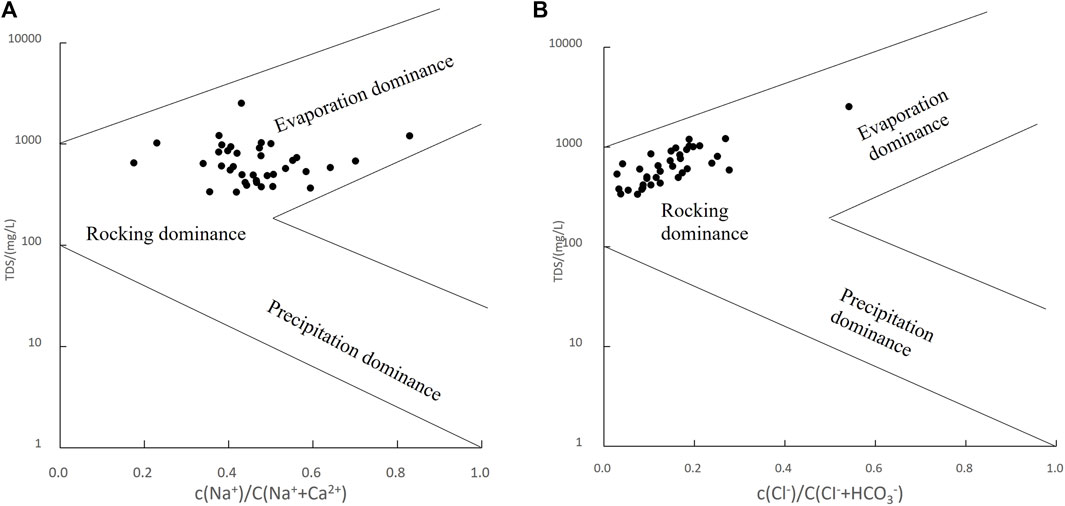
Figure 5. Gibbs plot of groundwater in the study area. (A): TDS VS c(Na+ )/c(Na+ +Ca2+); (B): TDS VS c(Cl−)/c(Cl−+HCO3−).
For most water sample points, the Cl−/(Cl− + HCO3−) and c (Na+)/c (Na++Ca2+) ratios are less than 0.5, indicating that, overall, the concentration of c (HCO3−) is higher than that of c (Cl−), and the concentration of c (Ca2+) is higher than that of c (Na+). As the Na+/(Na++Ca2+) ratio increases, TDS content does not show significant variation, which may be attributed to cation exchange processes.
4.1.2 Cation exchange and Adsorption processes
Many scholars have analyzed cation exchange processes in groundwater using the relationship γ [(Ca2+ + Mg2+) − (HCO3− + SO42-)] and γ (Na+ − Cl−) (Carol et al., 2013; Liu et al., 2015). Figure 6 illustrates the correlation between the residual Ca2+ and Mg2+ generated by the dissolution or precipitation of calcite, dolomite, and gypsum, and the residual Na+ generated by the dissolution or precipitation of halite.
When sample points are in the fourth quadrant, Na+ and K+ adsorbed on the surfaces of aquifer rocks and soils undergo exchange with Ca2+ and Mg2+ in the groundwater. Conversely, when sample points are in the second quadrant, the reverse occurs, a phenomenon known as reverse cation exchange. Many sampling points in the area fall within the cation exchange zone, indicating that during groundwater flow, Ca2+ and Mg2+ exchange with Na+ and K+ adsorbed on rock surfaces. This exchange process results in the precipitation of Ca2+ and Mg2+, leading to a reduction in their residual concentrations. A small number of sampling points fall in the second quadrant, indicating that a reverse cation exchange has occurred between Na+ and K+ in the groundwater and Ca2+ and Mg2+ adsorbed on rock surfaces.
4.1.3 Mineral dissolution processes
Ion ratio diagrams help further identify the sources of major ions in groundwater (Reddy and Kumar, 2010; Zhou et al., 2021). The relationship diagram γ (Na+ + K+) vs γ (Cl−) can be used to determine the sources of Na+, K+, and Cl− in groundwater (Fu et al., 2018; Sami 1992). Most shallow groundwater samples in the study area are positioned above the y = x line (halite dissolution line) in Figure 7A, where the molar concentration of Na+ is significantly higher than that of Cl−. This suggests that, in addition to rock salt mineral dissolution, other sources also contribute a considerable amount of Na+. The Na+/Cl− concentration ratio in seawater and atmospheric precipitation is 0.86, while the ratio resulting from the weathering dissolution of silicate rocks is greater than 1, indicating that Na+ in groundwater is influenced by the dissolution of silicate minerals.
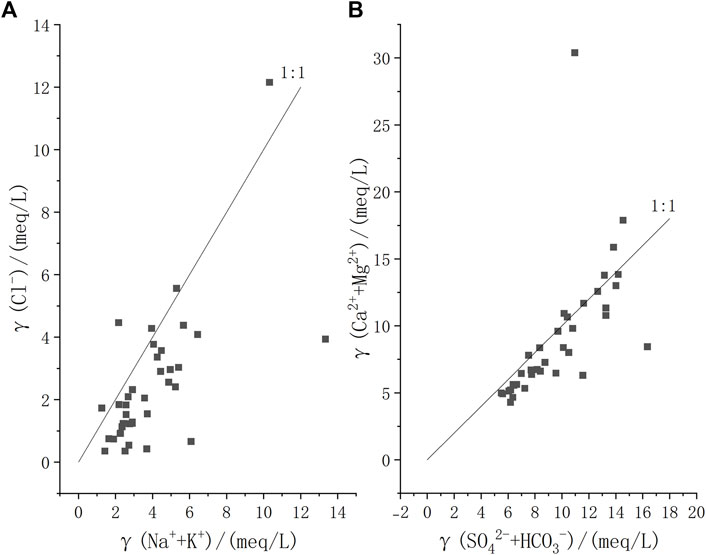
Figure 7. Ion ratio diagram of groundwater in the study area. (A): γ(Cl−) VS γ(Na+ + K+); (B): γ(Cl−) VS γ(SO42-+ HCO3−).
The dissolution of carbonate rocks, silicate rocks, or evaporite salts is the main source of Ca2+ and Mg2+ in groundwater (Fang et al., 2021). The molar ratios of γ(Ca2+)/γ(HCO3−) produced by the dissolution of calcite (carbonate rock), dolomite (carbonate rock), and gypsum (evaporite) are 1:2, 1:4, and 1:3, respectively. Γ (Ca2+ + Mg2+) – γ (SO42- + HCO3−) indicates whether there is an increase or decrease of Ca2+ and Mg2+ relative to the dissolution of carbonate rocks and evaporite salts. If the dissolved solutes in the water originate solely from the weathering dissolution of carbonate rocks and gypsum, the ion ratio (SO42- + HCO3−): (Ca2+ + Mg2+) would be 1:1, according to their dissolution equation. When water sample points lie above the 1:1 line, it indicates a dominant contribution from carbonate dissolution; points below the 1:1 line suggest that other mineral dissolutions also contribute to an excess of γ (SO42- + HCO3−) relative to γ (Ca2+ + Mg2+). Figure 7B shows that the concentration relationship of some water samples aligns closely with the 1:1 line, indicating that the primary sources of Ca2+, Mg2+, HCO3−, and SO42- components in groundwater are the dissolution of carbonate rocks and gypsum minerals. Some water samples fall below the 1:1 line, indicating that silicate rock dissolution also plays a role in the study area.
4.2 Impact of human activities
The variance of the contrast coefficient can be used to distinguish whether the formation of groundwater chemical components is mainly driven by natural geochemical evolution or significantly influenced by human activities. A larger contrast coefficient variance for a groundwater chemical component indicates a stronger influence of human activities (Zhang et al., 2018). The study area has numerous surface water bodies, including the Yellow River, as well as floodplain wetlands inside the levee and depressions on the outer side of the levee. The floodplains are inundated during floods and directly recharged by Yellow River water. Since the riverbed is elevated above the outer levee areas, the river water seeps laterally under high hydraulic pressure, recharging the surrounding areas, and forming depressions on the back side of the levee, which characterizes the unique hydrological features in the lower reaches of the Yellow River.
As shown in Table 3, groundwater near floodplain wetlands and depressions (P1) is more strongly affected by human activities, while groundwater in other areas (P2) is less impacted. The contrast coefficient variance values for Mg2+, Cl−, and NO3− in the P1 area are all greater than 1, indicating that these three components are strongly influenced by human activities. Na+, Ca2+, and SO42- are moderately affected, while other ions are less affected by human activities. In the P2 area, human activities have a moderate effect on NO3− but little effect on the other ions.
Previous studies (Sun et al., 2021) and this research both indicate that the concentrations of NO3−, SO42-, and Cl− in the Yellow River are higher than in groundwater, suggesting that Yellow River water has been more influenced by human activities than groundwater. Although no agricultural or aquaculture activities are conducted in the floodplain wetlands, calculations show that groundwater near surface water is more affected by human activities than groundwater in other human-impacted areas. This may be due to the direct recharge and lateral seepage of Yellow River water into the groundwater.
4.3 Influence of lateral infiltration of the Yellow River
Regardless of the season—whether flood or dry—Yellow River water in the study area primarily infiltrates into groundwater through lateral seepage or irrigation-induced infiltration. Since the operation of the Xiaolangdi Reservoir, the lateral infiltration influence zone of the Yellow River in the lower Yellow River plain has generally expanded. The Yellow River’s influence in the Zhengzhou section is the largest, with the lateral infiltration impact on the northern bank reaching 20–25 km, and on the southern side within 15 km (Su et al., 2021). The increased infiltration capacity of the Yellow River has also enhanced its impact on the water quality of the adjacent aquifers.
D and 18O isotope data reveals a close hydraulic connection between the Yellow River water and coastal groundwater, with a significant positive correlation between the groundwater level and the Yellow River water level (Zhang et al., 2021). The proportion of shallow groundwater recharge from the Yellow River ranges from 21% to 97%, and the recharge intensity of shallow groundwater on the north bank is significantly higher than that on the south bank (Yang, 2018).
In the 1990s, groundwater along the Yellow River was primarily classified as HCO3—Ca·Mg·Na type water. However, this study reveals an increase in groundwater types, including Cl ·SO4·HCO3—Ca·Mg (Na) and SO4·HCO3—Ca·Mg types. Yellow River water contains higher sulfate (SO42-) concentrations, with SO42- measured values of 108.48 mg/L in 2012, 139.4 mg/L in 2015, and 210.9 mg/L in 2023 along the lower reaches of Yellow River (Zhang et al., 2020; Yu et al., 2017). The transformation in groundwater chemical types in the Yellow River influence zone indicates the significant recharge of groundwater from the river. Moreover, along the flow direction of the Yellow River, ions like TDS, total hardness, HCO3−, Cl−, SO42- are increasing, indicating potential contamination of groundwater by the Yellow River water.
The lateral infiltration of Yellow River water and irrigation-induced infiltration using Yellow River water are unique groundwater recharge mechanisms in the Yellow River influence zone. If the water quality of the Yellow River is not effectively managed and improved, the direct recharge of its water into aquifers will negatively impact groundwater quality. Therefore, improving and regulating the water quality of the Yellow River is an urgent and key issue that requires immediate attention.
4.4 Factor analysis
Principal component analysis (PCA) was performed on 11 hydrochemical indicators of 30 shallow groundwater samples in the study area to explore the main controlling factors of groundwater chemical evolution. The Bartlett’s test for sphericity yielded a chi-square value of 1,284.5, with 78 degrees of freedom and a significance probability of 0, indicating a significance level of P < 0.001. This confirms that the hydrochemical indicators in the study area are not independent of each other but exhibit intercorrelations (normal distribution). Therefore, the selected water quality data is reasonable and suitable for principal component analysis. A total of three principal factors were extracted, with a cumulative contribution rate of 78.2% (Table 4).
First Principal Factor (F1): The contribution rate of F1 is 45.5%, with higher loadings on TDS, Cl−, Ca2+, Mg2+, NO3−, and Na+, all of which are positively correlated with F1. The study area is located in the Yellow River alluvial plain, where the hydraulic gradient is low and groundwater flow is relatively slow, resulting in more extensive water-rock interactions between groundwater and aquifer media. Over long periods, carbonate rocks, gypsum, and silicate minerals within the aquifer undergo dissolution and cation exchange, causing Cl−, Ca2+, Mg2+, and Na+ to become the dominant components in the groundwater. The area experiences significant human activities, and the unsaturated zone is made up of layered sandy soils with high permeability, allowing NO3− from animal manure, pesticides, and fertilizers to infiltrate the groundwater through irrigation and rainfall. As discussed earlier, human activities have a strong and moderate impact on Cl−, Mg2+, and Na+. Therefore, F1 represents the impact of water-rock interactions and human activities on the chemistry of groundwater.
The contribution rate of F2 is 19.2%, with high loadings on HCO3−, Fe, Mn, and K+. Quaternary sediments in the Henan Plain contain many iron-manganese nodules, which continuously dissolve into groundwater through leaching, leading to higher concentrations of iron and manganese in some areas (Sun et al., 2021). Therefore, PC2 can be interpreted as the dissolution of iron-manganese minerals.
The contribution rate of F3 is 13.5%, with SO42- showing a high loading. As discussed earlier, the continuous recharge from the Yellow River has led to an increase in SO4-rich groundwater types within the Yellow River influence zone. In addition to the dissolution of sulfate minerals, the input of SO42- from surface water also contributes significantly to SO42−concentrations. Therefore, F3 represents the influence of Yellow River water on the chemistry of groundwater.
4.5 APCS -MLR analysis
Based on the above analysis, the contribution rates of the influencing factors to the hydrochemical indicators were calculated, as shown in Figure 8. The linear fit coefficients (R2) between the observed and the model predicted values of the water chemistry factors are all greater than 0.75, and the significance level of the regression equations is p < 0.05, indicating a good linear fit.
The average contribution rates of the four sources to the shallow groundwater in the study area are: 30.6%, 27.3%, 21.0%, and 21.2%.
Water-rock interactions and human activities have a strong influence on the conventional ions, with contribution rates exceeding 50% for TDS, Cl−, and Mg2+. The contribution rates for Na+, Ca2+, and NO3− are 44.0%, 36.2%, and 37.8%, respectively.
High iron-manganese groundwater is widely distributed in the Henan Plain, mainly influenced by the high background values of the aquifer sediments. The dissolution of iron-manganese minerals accounts for 72.6% and 64.9% of Fe and Mn contributions, respectively, While the Yellow River water contributes 21.5% to Fe and 25.5% to Mn. Due to sediment deposition from the Yellow River, the aquifer has a characteristic alternating layer of sand and soil, which restricts groundwater flow and enhances reducing conditions (Qiao et al., 2022). Iron-manganese oxides will undergo reduction and dissolution under anaerobic conditions and enter the groundwater.
The contribution rates of Yellow River water to SO42- and Na+ are 43.8% and 37.1%, respectively. Both SO42- and Na+ are dominant ions in Yellow River water. The presence of numerous channels and ponds in the study area that divert Yellow River water allows surface water to recharge the groundwater through infiltration. Additionally, groundwater near the Yellow River is further influenced by lateral recharge, leading to the accumulation of these ions.
5 Conclusion
(1) In the surface water-groundwater exchange zone, 46% of the shallow groundwater is of the HCO3—Na·Ca·Mg type. Due to the lateral infiltration of Yellow River water, groundwater on both sides of the river evolves into Cl·SO4·HCO3 type and SO4·HCO3 type water.
(2) PCA identified water-rock interactions, human activities, and Yellow River water as the primary controlling factors affecting shallow groundwater in the study area, with a cumulative variance explanation rate of 78.2%.
(3) Sufficient water-rock interaction has led to increased concentrations of TDS and Mg2+ as major components. Since surface water is continuously converted into groundwater through lateral infiltration or irrigation-induced infiltration, SO42- and Na+ in the groundwater are strongly influenced by surface water input. NO3− is primarily affected by human activities, including domestic and agricultural practices. Fe and Mn are mainly influenced by the high background values of aquifer sediments, while the significant enhancement of the aquifer’s reducing conditions leads to higher concentrations of Fe and Mn in the groundwater.
(4) For the protection of groundwater resources in areas with strong exchange between surface water and groundwater, factors such as the Yellow River water and geographical location should be taken into account. Continuous monitoring of the Yellow River water quality is essential, as well as strict control over farmland fertilization and urban pollution discharge on both sides of the river.
Data availability statement
The datasets presented in this article are not readily available because The original contributions presented in the study are included in the article, further inquiries can be directed to the corresponding author. Requests to access the datasets should be directed to Cui Xiangxiang, Y3VpeGlhbmcyMDA4QDEyNi5jb20=.
Author contributions
CX: Data curation, Investigation, Methodology, Software, Writing – original draft. LL: Data curation, Methodology, Writing – review and editing. LY: Data curation, Software, Writing – original draft. ZY: Investigation, Methodology, Writing – review and editing. MS: Investigation, Resources, Writing – original draft. LS: Data curation, Investigation, Validation, Writing – original draft.
Funding
The author(s) declare that financial support was received for the research and/or publication of this article. This work was supported by Chinese Academy of Geological Sciences Basal Research Fund (JKYZD202411), Basic Scientific Research Funds of China Geological Survey (SK202318), National Land and Resources Major Survey Project, Ministry of Land and Resources (DD20221773-3).
Conflict of interest
The authors declare that the research was conducted in the absence of any commercial or financial relationships that could be construed as a potential conflict of interest.
Generative AI statement
The author(s) declare that no Generative AI was used in the creation of this manuscript.
Publisher’s note
All claims expressed in this article are solely those of the authors and do not necessarily represent those of their affiliated organizations, or those of the publisher, the editors and the reviewers. Any product that may be evaluated in this article, or claim that may be made by its manufacturer, is not guaranteed or endorsed by the publisher.
References
Cao, W., Gao, Z., Guo, H., Pan, D., Qiao, W., Wang, S., et al. (2022). Increases in groundwater arsenic concentrations and risk under decadal groundwater withdrawal in the lower reaches of the Yellow River basin, Henan Province, China. Environ. Pollut. 296, 118741. doi:10.1016/j.envpol.2021.118741
Carol, E., Mas-Pla, J., and Kruse, E. (2013). Interaction between continental and estuarine waters in the wetlands of the northern coastal plain of Samborombón Bay, Argentina. Appl. Geochem. 34, 152–163. doi:10.1016/j.apgeochem.2013.03.006
Chen, J., Gao, Y., Qian, H., Ren, W., and Qu, W. (2021). Hydrogeochemical evidence for fluoride behavior in groundwater and the associated risk to human health for a large irrigation plain in the Yellow River Basin. Sci. Total Environ. 800, 149428. doi:10.1016/j.scitotenv.2021.149428
Chen, J., Wang, S., Zhang, S. X., Bai, Y. J., Zhang, X. Y., Chen, D., et al. (2023b). Identifying the hydrochemical features, driving factors, and associated human health risks of high-fluoride groundwater in a typical Yellow River floodplain, North China. Environ. Geochem. Health. 45, 8709–8733. doi:10.1007/s10653-023-01748-9
Chen, J. P., Yan, Y., Feng, Y., Wu, X. H., Liu, H., Tan, Z. R., et al. (2023a). Hydrochemical genesis and ecological environment influence of groundwater in Dezhou city at lower Yellow River Basin. Environ. Chem. 42 (1), 125–137. doi:10.7524/j.issn.0254-6108.2022081103
Fang, L. J., Gao, R. Z., Jia, D. B., Yu, R. H., Liu, X. Y., and Liu, Y. T. (2021). Spatial-temporal characteristics of groundwater quality and its environmental driving factors of Steppe Basin—taken Balaguer river basin of Inner Mongolia for instance. China Environ. Sci. 41 (5), 2161–2169. doi:10.19674/j.cnki.issn1000-6923.20210330.001
Fu, C. C., Li, X. Q., Ma, J. F., Ling, X. L., Ming, G., and Zhan, X. B. (2018). A hydrochemistry and multi-isotopic study of groundwater origin and hydrochemical evolution in the middle reaches of the Kuye River basin. Appl. Geochem. 98, 82–93. doi:10.1016/j.apgeochem.2018.08.030
Gibbs, R. J. (1970). Mechanisms controlling world water chemistry. Sci. 170 (3962), 1088–1090. doi:10.1126/science.170.3962.1088
Li, W., Wu, J., Zhou, C., and Nsabimana, A. (2021). Groundwater pollution source identification and apportionment using PMF and PCA-APCS-MLR receptor models in Tongchuan City, China. Arch. Environ. Contam. Toxicol. 81, 397–413. doi:10.1007/s00244-021-00877-5
Liu, F., Song, X., Yang, L., Han, D., Zhang, Y., Ma, Y., et al. (2015). The role of anthropogenic and natural factors in shaping the geochemical evolution of groundwater in the subei lake basin, ordos energy base, northwestern China. Sci. Total Environ. 538, 327–340. doi:10.1016/j.scitotenv.2015.08.057
Lv, X. L., Liu, J. T., Zhou, B., Zhu, L., and Zhang, Y. X. (2021). Distribution characteristics and enrichment mechanism of fluoride in the shallow aquifer of the Tacheng Basin. Earth Sci. Fron 28 (2), 426–436. doi:10.13745/j.esf.sf.2020.10.29
Marganingrum, D., Santoso, H., Wulan, D. R., Sudrajat, Y., Yulianto, E., Triyono, T., et al. (2023). Climate variability and changes in shallow groundwater quality on Indonesia’s small tropical island. Sustain. Water Resour. Manag. 9, 79. doi:10.1007/s40899-023-00857-8
Meng, S. R., Lu, D. Y., Wang, C. L., and Zhang, J. Y. (2022). Research of groundwater chemical characteristics and controlling factors in Zhongmu County, Zhengzhou City. Environ. Chem. 41 (3), 977–986. doi:10.7524/j.issn.0254-6108.2021010802
Muangthong, S., and Shrestha, S. (2015). Assessment of surface water quality using multivariate statistical techniques: case study of the Nampong River and Songkhram River, Thailand. Environ. Monit. Assess. 187, 548. doi:10.1007/s10661-015-4774-1
Qiao, W., Cao, W., Gao, Z., Pan, D., Ren, Y., Li, Z., et al. (2022). Contrasting behaviors of groundwater arsenic and fluoride in the lower reaches of the Yellow River basin, China: geochemical and modeling evidences. Sci. Total Environ. 851, 158134. doi:10.1016/j.scitotenv.2022.158134
Reddy, A. G. S., and Kumar, K. N. (2010). Identification of the hydrogeochemical processes in groundwater using major ion chemistry: a case study of Penna–Chitravathi river basins in Southern India. Environ. Monit. Assess. 170, 365–382. doi:10.1007/s10661-009-1239-4
Ren, Y., Cao, W., Zhao, L., Wang, S., Pan, D., Zhang, L., et al. (2024). Environmental factors influencing groundwater quality and health risks in northern Henan Plain, China. Expo. Health 17, 507–522. doi:10.1007/s12403-024-00674-0
Sami, K. (1992). Recharge mechanisms and geochemical processes in a semi-arid sedimentary basin, Eastern Cape, South Africa. J. Hydro. 139 (1-4), 27–48. doi:10.1016/0022-1694(92)90193-y
Su, C., Zhang, X. Q., Fei, Y. H., Li, Z., Meng, S. H., Cui, X. X., et al. (2021). Lateral seepage scope of downstream of Yellow River after the operation of Xiaolangdi reservoir and its impact on groundwater environment. Geo. China. 48 (6), 1669–1680. doi:10.12029/gc20210601
Su, H. M., Zhang, F. W., Hu, J. Y., Lei, J. F., Zuo, W., Yang, B., et al. (2024). Identified the hydrochemical and the sulfur cycle process in subsidence area of Pingyu mining area using multi-isotopes combined with hydrochemistry methods. J. Groundw. Sci. Eng. 12 (1), 62–77. doi:10.26599/JGSE.2024.9280006
Sun, L., Wang, L. L., and Cao, W. G. (2021). Evolution of groundwater hydrochemical characteristics in the influence zone of the lower Yellow River in Henan. Yellow Riv. 43 (12), 91–99. doi:10.3969/j.issn.1000-1379.2021.12.018
Tu, C. L., Yang, R. B., Ma, Y. Q., Linghu, C. W., Zhao, R. G., and He, C. Z. (2023). Characteristics and driving factors of hydrochemical evolution in Tuochangjiang River Basin, western Guizhou province. Environ. Sci. 44 (02), 740–751. doi:10.13227/j.hjkx.202204313
Wang, S., Ren, Y., Guo, H., Cao, W. G., Li, X. Z., and Xiao, X. Y. (2024). Chemical characteristics of shallow groundwater in the Yellow River diversion area of henan province and identification of main control pollution sources. Environ. Sci. 45 (2), 792–801. doi:10.13227/j.hjkx.202303263
Wang, W., Li, Y., Li, G., and Xu, H. (2025). Effects of surface water irrigation on fertility and properties of agricultural soil in the aboveground segment of the Yellow River downstream. Water Air Soil Pollu 236, 77. doi:10.1007/s11270-024-07722-0
Xia, Q. W., Li, B. H., He, J. T., Huang, J. X., and Guo, M. L. (2021). Nitrogen transformation of shallow groundwater in river area of ecological recharge of reclaimed water in Chaobai River. Res. Environ. Sci. 34 (3), 618–628. doi:10.13198/j.issn.1001-6929.2020.07.22
Xu, J., Wang, M., Zhong, T., Zhao, Z., Lu, Y., Zhao, X., et al. (2022). Insights into site-specific influences of emission sources on accumulation of heavy metal(loids) in soils by wheat grains. Environ. Sci. Pollut. Res. 29, 73131–73146. doi:10.1007/s11356-022-21022-2
Yang, W. f. (2018). Research on the transformation between surface water and groundwater based on environmental isotopes in the Yubei plain. Beijing). Beijing: China University of Geosciences. doi:10.27493/d.cnki.gzdzy.2018.000019
Yu, Y. L., Song, X. F., Guo, J., Li, S. N., Wei, W., Song, X. J., et al. (2017). Characteristics and controlling factors of water chemistry in surface waters in the Yellow River delta. J. Arid. Land. Resour. Environ. 31 (10), 58–64. doi:10.13448/j.cnki.jalre.2017.314
Yuan, R., Li, Z., and Guo, S. (2024). Hydrochemical evolution of groundwater in a river corridor: the compounded impacts of various environmental factors. Discov. Water. 4, 32. doi:10.1007/s43832-024-00086-w
Zaryab, A., Farahmand, A., Nassery, H. R., Alijani, F., Ali, S., and Jamal, M. Z. (2023). Hydrogeochemical and isotopic evolution of groundwater in shallow and deep aquifers of the Kabul Plain, Afghanistan. Environ. Geochem Health 45, 8503–8522. doi:10.1007/s10653-023-01734-1
Zhai, T. L., Zhang, Q. Q., Wang, L., and Wang, H. W. (2024). Temporal and spatial variations hydrochemical components and driving factors in Baiyangdian Lake in the Northern Plain of China. J. Groundw. Sci. Eng. 12 (3), 293–308. doi:10.26599/JGSE.2024.9280022
Zhang, D., Zhao, Z. Q., Peng, Y., Fan, B., Zhang, L., Li, J., et al. (2020). Sulfur cycling in the Yellow River and the sulfate flux to the ocean. Chem. Geo 534, 119451. doi:10.1016/j.chemgeo.2019.119451
Zhang, M., Wang, X., Liu, C., Lu, J., Liu, Y., Mo, Y., et al. (2020). Quantitative source identification and apportionment of heavy metals under two different land use types: comparison of two receptor models APCS-MLR and PMF. Environ. Sci. Pollut. Res. 27, 42996–43010. doi:10.1007/s11356-020-10234-z
Zhang, Q. Q., Wang, H. W., Wang, L., and Shang, M. S. (2018). Increasing mechanism of groundwater total hardness (TH) in the Hutuo River alluvial pluvial fan. Environ. Sci. Technol. 41 (S2), 62–68. doi:10.19672/j.cnki.1003-6504.2018.S2.012
Zhang, X. Q., Su, C., Liu, L., Meng, S. H., and Cui, X. X. (2023). Mechanism of groundwater gushing outside the dike of the lower reaches of the Yellow River. Water Res. Power 41 (02), 207–210. doi:10.20040/j.cnki.1000-7709.2023.20220673
Zhao, X. N., Wang, S. B., Yang, J. R., Ma, Q. H., Liu, Y., and Zhang, R. Q. (2021). Chemical components and sources of PM 2. 5 and their evolutive characteristics in Zhengzhou. Environ. Sci. 42 (08), 3633–3643. doi:10.13227/j.hjkx.202012033
Zhou, B., Wang, H., and Zhang, Q. (2021). Assessment of the evolution of groundwater chemistry and its controlling factors in the Huangshui River Basin of Northwestern China, using hydrochemistry and multivariate statistical techniques. Int. J. Environ. Res. Public Health. 18, 7551. doi:10.3390/IJERPH18147551
Keywords: hydrochemical characteristics, unconfined aquifers, Yellow River, water-rock interactions, lateral infiltration, human activities
Citation: Xiangxiang C, Lu L, Yutong L, Xueqing Z, Suhua M and Shan L (2025) Chemical characteristics and controlling factors of groundwater in an intensive surface water-groundwater conversion zone: a case study of the Zhengzhou section of the lower Yellow River. Front. Environ. Sci. 13:1580232. doi: 10.3389/fenvs.2025.1580232
Received: 20 February 2025; Accepted: 25 April 2025;
Published: 12 May 2025.
Edited by:
Venkatramanan Senapathi, National College, Tiruchirappalli, IndiaReviewed by:
Dong-Hun Kim, Korea Institute of Geoscience and Mineral Resources, Republic of KoreaSheming Chen, China Geological Survey, China
Copyright © 2025 Xiangxiang, Lu, Yutong, Xueqing, Suhua and Shan. This is an open-access article distributed under the terms of the Creative Commons Attribution License (CC BY). The use, distribution or reproduction in other forums is permitted, provided the original author(s) and the copyright owner(s) are credited and that the original publication in this journal is cited, in accordance with accepted academic practice. No use, distribution or reproduction is permitted which does not comply with these terms.
*Correspondence: Liu Lu, bGl1bHUwMDExQDE2My5jb20=