- 1Faculty of Forestry and Wood Sciences, Czech University of Life Sciences, Prague, Czechia
- 2Department of Forest and Soil Sciences, BOKU—University of Natural Resources and Applied Life Sciences, Vienna, Austria
Introduction: Climate change has led to rising atmospheric CO2 levels and temperatures, projected to double CO2 concentrations and increase temperatures by 2–5°C by the end of the 21st century. These environmental changes influence plant primary and secondary metabolism, potentially altering plant-insect interactions. Herbivore performance depends on the nutritional quality of host plants, which may decline with elevated CO2 due to an increased carbon-to-nitrogen (C:N) ratio. To explore these effects, the performance of spongy moth larvae (Lymantria dispar) was assessed on oak (Quercus robur) and spruce (Picea abies) seedlings grown under varying climatic conditions. This approach compares a preferred host with a non-preferred one in the case of L. dispar, providing insight into how host plant selection may be influenced under future climate scenarios. In addition, the nun moth (Lymantria monacha), a conifer-feeding species, was also studied on the experimental spruce seedlings to facilitate a comparison with a specialist herbivore.
Methods: Three-year-old oak and spruce seedlings were reared for 1 year under four climate scenarios combining two CO2 levels (ambient: 410 ppm and elevated: 820 ppm) and two temperature regimes (20:15°C and 25:20°C). Seedlings were then processed into leaf powder diets for laboratory bioassays with larvae. Secondary metabolites in the seedlings were analyzed to assess climate-induced changes in tree composition and their effects on herbivores.
Results: Elevated CO2 increased the C:N ratio in both tree species, with spruce showing a higher ratio than oak. Higher temperatures led to increased nitrogen content, particularly in oak seedlings. L. dispar performed better on oak despite higher secondary metabolite concentrations, while L. monacha exhibited minimal variation in performance on spruce across climate treatments.
Conclusion: The combined effects of elevated CO2 levels and increased temperatures impacted plant quality; however, there were nearly no differences in the performance of Lymantria larvae. Despite the higher concentrations of secondary metabolites in the trees, the larvae were able to thrive effectively, demonstrating their resilience to environmental changes.
1 Introduction
1.1 Human activity changes the atmospheric composition
Human activities have resulted in an increase in greenhouse gasses, particularly carbon dioxide (CO2), which contributes significantly to global warming (IPPC, 2021; International Energy Agency [IEA], 2017; Xi-Liu and Gao, 2018; Yu et al., 2008). Despite established agreements and advancements in technology, there has been no meaningful reduction in CO2 emissions and other harmful pollutants (Charfeddine, 2017). At the start of the Industrial Revolution, the concentration of atmospheric CO2 was 280 ppm. Currently, it is approximately 400 ppm (Sodiq et al., 2023) and is projected to double by the end of the 21st century (IPCC, 2014). This increase in CO2 levels is primarily due to the burning of fossil fuels and deforestation (IPCC, 2014; Begum et al., 2020; Uddin et al., 2021; Keeling, 1995). As a result, plants will need to adapt to the current conditions of CO2 and temperature and future scenarios involving elevated CO2 levels, higher temperatures, and potentially more frequent extreme weather events (Slot et al., 2021).
1.2 CO2, temperature and plants
Increased levels of atmospheric CO2 are resulting in higher global temperatures and more severe droughts (Diffenbaugh et al., 2017). Consequently, many trees have died (Hartmann et al., 2018). Forests are responsible for two-thirds of global photosynthesis (Giri, 2017) and cover over a third (43%) of the Earth’s land surface (Bonan, 2008; Karnosky, 2003). While forests are directly exposed to the effects of climate change, they are also impacted by this phenomenon in other ways. Given forests crucial role in mitigating carbon emissions, it is important to understand how elevated CO2 levels affect tree growth and ecosystem functions within forested areas (Psistaki et al., 2024).
Elevated levels of CO2 and temperature can influence ecological processes in several ways: by directly affecting plant or insect herbivore species separately (Murray et al., 2013; Bale et al., 2002; Ellsworth et al., 2004), in combination (Zvereva and Kozlov, 2006), or indirectly through biotic interactions (Blois et al., 2013; Montoya and Raffaelli, 2010; Tylianakis et al., 2008). The complex relationships among abiotic stress factors, host trees, and insect herbivores present significant challenges in predicting the overall impacts of climate change on forest ecosystems (Jactel et al., 2019).
1.2.1 CO2 concentration
Numerous tree-ring studies have indicated that radial growth increases with rising atmospheric CO2 concentration (Guo et al., 2022; Marchand et al., 2020; Zhang et al., 2003), but it seems that only for a short time period (Körner et al., 2005). Elevated levels of CO2 in the atmosphere can change the chemistry of plants. This includes enhancements in photosynthesis and increases in carbohydrates (Zheng et al., 2019; Hartmann et al., 2018; Boullis et al., 2015; Zhang and Dang, 2007; Lindroth et al., 1993; Williams et al., 1998), as well as non-structural carbohydrates and carbon-based allelochemicals (Bae et al., 2019; Rajashekar, 2018). Additionally, the C:N ratio is affected (Sheng et al., 2021; Roy and Mathur, 2021; Watanabe et al., 2021; Lotfiomran et al., 2016; Ziska, 2002). The C:N ratio reflects the plant’s chemical composition, with an increase in carbohydrate content and a decrease in nitrogen concentration (by about 1/10–1/3) (Robinson et al., 2012; Lindroth et al., 2002; Ehleringer et al., 2002), a phenomenon referred to as the nitrogen dilution effect (Strain and Cure, 1985). However, these changes can vary among different tree species (Foss et al., 2013).
1.2.2 Air temperature
It is expected that the rise in atmospheric CO2 levels will result in a global average surface temperature increase of at least 1–3°C within this century (Rapp, 2024; Adak et al., 2023; Ciais et al., 2013; Solomon, 2007). Temperature is the primary factor limiting photosynthesis and plant growth (see Kaiser et al., 2015; Yamori et al., 2014; Kellomäki and Väisänen, 1988). Higher air temperatures are expected to enhance photosynthetic rate, which in turn will boost leaf biomass production and accelerate plant growth, further amplifying the effects of elevated CO2 (Way and Oren, 2010; Farrar and Williams, 1991; Long, 1991). Notably, higher temperatures tend to promote growth in deciduous species more than in coniferous trees (Way and Oren, 2010). Additionally, the nutritional quality of host trees for herbivores is influenced by temperature (Holopainen et al., 2018). The temperature-induced changes in phytochemistry can vary significantly among different traits and tree species (Jactel et al., 2019). While increasing temperatures have a weak effect on nitrogen content or the C:N ratio, they significantly impact other characteristics, such as carbohydrates, phenols, and terpenoid content (Zvereva and Kozlov, 2006).
1.3 Plant-herbivore interaction
Herbivorous insects are closely connected to specific host plant species, which serve as their food source. The presence and success of herbivorous insects depend significantly on the quality of these host plants. The quality, in turn, is influenced by both primary and secondary metabolite composition and content (Schmitt et al., 2020). Organic carbon, bound in a wide range of plant-building compounds is the primary source of energy for insects, while their bodies are predominantly composed of proteins, containing also significant amounts of nitrogen. As a result, insects must consume not only a carbon-rich diet but also must contain substantial amounts of nitrogen to completely meet their nutritional needs (Zhou et al., 2015).
1.3.1 Nitrogen and carbon as important elements of life
Nitrogen is a crucial element for insect growth (Ren et al., 2022), and it plays a key role in plant metabolic processes, cellular structure, and genetic coding (Bala et al., 2018; Mattson, 1980). When nitrogen levels in leaves are low, the development of leaf-chewing insects is prolonged because they need to consume more plant tissue to obtain enough nitrogen-based nutrients (Stiling and Cornelissen, 2007; Johnson and McNicol, 2010; Johnson et al., 2014; Yin et al., 2010; Xie et al., 2015). In high CO2 treatments, insect larvae can consume up to 80% more leaves (Lincoln et al., 1984). Additionally, insects that feed on plants grown in elevated CO2 levels exhibit reduced efficiency in utilizing ingested food (Zhang et al., 2018; Teawkul and Hwang, 2019; Marks and Lincoln, 1996), which correlates with a reduced nitrogen content in their food source (White and Warrington, 1984). An increased feeding rate also leads to a higher intake of defensive compounds from host trees (Richardson et al., 2015).
Carbon provides the energy needed for metabolic processes (Bringaud et al., 2006). When the diet is too high in carbon compared to nitrogen, larvae may have plenty of energy but not enough protein for optimal growth. On the other hand, a diet high in nitrogen relative to carbon can enhance growth but may lack sufficient immediate energy. Although it is known that temperature changes can affect the plant nutritional value for many insect species, there is less understanding of how these temperature variations impact insect development (Stamp and Yang, 1996).
1.3.2 Secondary metabolites
Changes in the chemical composition of plants, particularly the levels of plant carbon-based secondary metabolites such as phenolic glycosides and tannins, can significantly affect the quality of plant foliage as food for insect herbivores (Boeckler et al., 2011; Singh et al., 2021; Moreira et al., 2017; Barbehenn and Constabel, 2011; Lindroth et al., 2001). Although secondary metabolites are not essential for the growth and development of organisms, they often serve other important ecological functions (Salam et al., 2023). These compounds can help plants adapt to environmental stresses and act as chemical defenses against various threats, including insects (Reddy and Guerrero, 2004; Chen et al., 2005). For example, phenolic compounds like tannin, lignin, and flavonoids play a role in defending against herbivores and pathogens. Condensed tannins can hinder the growth and development of herbivores by precipitating proteins (Croteau et al., 2000).
1.4 Hypothesis and goals
Previous studies have demonstrated that the effects of elevated CO2 levels and temperature on plants vary among different tree species (Jactel et al., 2019; Way and Oren, 2010; Foss et al., 2013; Lindroth et al., 1993; Roth and Lindroth, 1994). Numerous studies have focused on the impact of elevated CO2 on plants, trees and forests (Klein et al., 2016; Ceulemans and Mousseau, 1994; Curtis et al., 1996; Curtis and Wang, 1998; Saxe et al., 1998; Norby et al., 1999; Karnosky et al., 2001; Karnosky, 2003). Reviews focused on the combination of these factors report that the anticipated negative impacts of elevated CO2 on herbivores may be mitigated by rising temperatures (Zvereva and Kozlov, 2006; Robinson et al., 2012).
Most studies on insect performance in elevated CO2 atmospheres or varying air temperatures have primarily focused on a single host plant species, with herbivores raised directly on those plants, or considered only one climatic factor at a time (Henn and Schopf, 2001; Milanović et al., 2015; Xiaowei et al., 2006). Our research aim was to test the interactions between two climatic factors, temperature and CO2 levels, on both broadleaf and coniferous trees, using two polyphagous herbivores that are significant forestry pests; one herbivore prefers conifers while the other prefers broadleaves. Furthermore, most of the studies cited in this article didn’t analyze changes in plant chemical composition when evaluating larval performance.
In our study, we planted seedlings in four different combinations of climatic conditions, using two host tree species [Quercus robur (L.) and Picea abies (L.) Karst. and two closely related herbivores] [Lymantria dispar (L., 1758) and Lymantria monacha (L., 1758)]. We also prepared leaf powder diets from these seedlings to ensure precise scoring of herbivore performance. To analyze changes in plant chemistry, we conducted a chemical analysis of the leaves and needles, focusing on alterations in selected secondary metabolites. We expected that seedlings grown under less favorable conditions (higher temperature and CO2 levels) would produce more defensive compounds, potentially leading to slower growth rates in the larvae.
The objectives of this study were: (I) to compare the effects of different combinations of CO2 levels and temperatures on seedlings and the performance of two closely related herbivorous insects (II) to test how the secondary metabolic compounds in these seedlings are affected by varying environmental conditions.
2 Materials and methods
2.1 Study species
Our study examines two species of lepidopterans, the spongy moth (Lymantria dispar) and the nun moth (Lymantria monacha), along with two types of trees: the coniferous Norway spruce (Picea abies) and the broadleaf pedunculate oak (Quercus robur) from the nursery of the Tree Breeding Station in Kostelec nad Černými lesy in the Czech Republic.
The larvae of L. dispar originate from a laboratory rearing (the New Jersey Standard Strain) of USDA- APHIS (Animal and Plant Health Inspection Service, OTIS Methods Development Center, MA 02542). Nun moth larvae were reared from eggs collected around the town of Zgierz in Poland in February 2021.
Both larvae species belong to the family Erebidae. The spongy moth (L. dispar) is a common pest found in temperate forests across Europe. L. dispar typically feeds on broadleaves, especially oaks, rarely feeding on pines in natural environments (Castedo-Dorado et al., 2016). This study compared the response to a preferred broadleaf host with a non-preferred coniferous host. Its larvae are folivores, feeding on the leaves of over 300 tree species, including Betula sp. (birch), Crataegus sp. (hawthorn), Larix sp. (larch), Populus sp. (aspen), Quercus sp. (oak), Salix sp. (willow), and Tilia sp. (basswood) (Elkinton and Liebhold, 1990; Liebhold et al., 1995). The nun moth (L. monacha) primarily feeds on the needles of conifers, especially spruces and pines, as well as larch and fir (Nakládal and Brinkeová, 2015). In spring, the larvae of both species hatch from overwintering eggs and progress through five instars for males or six instars for females (Doane and McManus, 1981; Schwenke, 1978). The larvae feed on leaves from April to June, at which point they pupate. Adult moths have a short lifespan of less than 7 days, and females are unable to fly (Keena et al., 2007). Both species experience regular and cyclical population outbreaks (Hlásny et al., 2016), leading to extensive defoliation that has been documented since the Middle Ages (Komárek, 1931; Schwenke, 1978).
Norway spruce and pedunculate oak are common and economically important tree species in Europe. English oak typically grows well in lowland areas, while Norway spruce is more prevalent at higher elevations. The economic significance of Norway spruce is highlighted by its extensive use in the forestry and timber industries (Jansson et al., 2013). However, its growth and resilience are increasingly threatened by climate change, especially at the trailing edge of its distribution (Honkaniemi et al., 2020).
2.2 Plant growing conditions
The experiment was conducted in four growth chambers (Step-In Fytoscope FS-SI 3400), which were set either to ambient CO2 levels (410 ppm) or elevated CO2 levels (820 ppm) at day and night temperatures to 20:15°C and 25:20°C under a light-dark cycle of 16:8 h. Relative humidity remained consistent at 70% in all chambers.
Oak and spruce seedlings were planted in trays (20 cm × 20 cm × 30 cm) filled with AGRO substrate (Agro CS a.s., Czech Republic) in the year 2016 in the nursery. On April 1st, 2019, nine spruce and nine oak seedlings were placed in each growth chamber. From November 10th to December 6th, 2019, the air temperature was gradually lowered from 20 and 25°C by 5 and 3°C per week until the temperature reached 5°C. On December 7th, 2019, the seedlings were placed outside for dormancy until February 17th, 2020, when they were returned to the chambers, and both the photoperiod and temperature were gradually increased again by 5 and 3°C each week until they finally reached 20 and 25°C. The collection of leaves was conducted in May 2020. The collected leaves were weighed, placed in paper bags, and frozen in liquid nitrogen. A freeze dryer (Alpha 2-4 LSC basic, Christ) was used for lyophilization, and the samples were then ground and homogenized using a mixer mill (MM 400, Retsch) and stored in a deep freezer at -80°C until diet preparation.
2.3 Chemical analysis
2.3.1 Elementary N and C analysis
For the analysis, 10–30 mg of the pure sample of the leaf powder was weighed into tin capsules designed for the instrument used. The capsules were then introduced into the combustion chamber of the Thermo Scientific Flash 2000 Elemental analyzer (Thermo Scientific, United States). The samples were combusted in a stream of pure oxygen at a temperature of 1,000°C. The resulting carbon and nitrogen oxides were passed through a copper reduction column and directed to a separation column, where moisture was removed. Helium was used as the carrier gas. The content of the separated oxides was determined using a thermal conductivity detector, and signal processing was performed with Eager Xperience software (Thermo Scientific).
2.3.2 Metabolite analysis
2.3.2.1 Extraction of carbohydrates
A total of 40 mg of freeze-dried samples (72 spruce and 36 oak samples) was extracted by 500 μL of methanol:chloroform: water in the ratio 12:5:3 and kept in the water bath at 60°C for 30 min. After 1,000 μL of water was added to the test tube. The solution was vortexed and then centrifugated for 3 min at 13,000 rpm. The supernatant was filtered using a 0.22 μm PVDF filter prior to LC-MS-qTOF analysis.
2.3.2.2 Determination of total phenolic content
TCT was performed according to Makkar et al. (1993). Briefly, 20 μL of the extract was placed in the test tube and made up the volume to 0.5 mL of water. Then, 250 μL of the Folin Ciocalteu regent was added. After 3 min, 1 mL of 20% sodium carbonate was added. The test tube was well mixed and kept in a dark place for 40 min. The absorbance was recorded at 725 nm using a spectrophotometer. The concentration of TPC was expressed in tannin acid equivalent.
2.3.2.3 Determination of total flavonoid content
The determination of TFC was carried out according to Zengin et al. (2016). Briefly, 150 μL of sample extract was placed into the 2 mL test tube and filled up to 1 mL with 70% of acetone. Then, a 2% solution of aluminum trichloride prepared in methanol was added. After 15 min, the absorbance was recorded at 415 nm, against blank. The concentration of flavonoid content was expressed in rutin equivalent (mg g–1).
2.3.2.4 Determination of total condensed tannin content
The total flavonoid content was determined following the method described by Porter et al. (1985). 150 μL of sample and 150 μL of 70% acetone were placed in 2-mL test tube. Then, 1.5 mL of butal-HCL reagent was added [butanol-HCL reagent was prepared by mixing 95 ml of butanol and 5 mL of concentrated HCL (37%)]. After, 50 μL of ferric reagent was added (ferric reagent was prepared as 2% ferric ammonium sulfate in 2N HCl). The solution was well mixed and placed in the thermoshaker for 1 h at 95°C and 1,000 rpm. When the solution was cooled, the absorbance was recorded at 550 nm. The concentration of TCT was expressed in cyanidin equivalent (mg g-1).
2.3.2.5 LC-MS-qTOF analysis of carbohydrates
LC-qTOF-MS/MS analysis was performed using Agilent 1290 Infinity II coupled with Agilent 6546 LC/MS QTOF system (Agilent, United States). Chromatographic separation was performed on Asahipak NH2P40 3E column 3.0 × 250 mm, 4 μm (Shodex, Japan) operated at 30°C. The mobile phase was composed of acetonitrile (A) and water (B). The isocratic elution was achieved with the started condition of mobile phase 70:30 (A:B) and increased up to 75:25 (A:B) for 30 min with a flow rate of 0.3 mL min-1. The injection volume was set to 1 μL. The system was operated in negative ionization mode. The QTOF parameters were as follows: scan range 100–760 m/z; drying gas temperature, 280°C; sheath gas flow rate, 12.0 L/min; sheath gas temperature, 275°C; capillary voltage, 3.0 kV; fragmentor, 160 V; collision energy at 10, 20 and 40 eV. MS/MS data were acquired at a scan range was 50–760 m/z. During the analysis, two reference masses: 112.9855 m/z and 966.0007 m/z were continuously measured for mass correction. The data collection was carried out using Agilent Mass Hunter Acquisition software. The data analysis was performed using Agilent Mass Hunter Qualitative Analysis 10.0 and Q-TOF Quantitative analysis. Identification of monosaccharides and oligosaccharides was determined according to precursor ions, the fragment ions, and retention time compared with the standards of sucrose, glucose, fructose, and raffinose.
2.4 Leaf powder diet preparation
The larvae were fed a wheat control diet after Bell (1981) from emergence until the end of the second instar (the diet recipe can be found in Supplementary Table S1). This diet (later called “wheat control diet”) was also provided to control group larvae, which were fed this diet for the entire experimental duration.
We prepared leaf powder diets from leaves of spruce and oak experimental seedlings to feed the fourth instar larvae. The preparation process is similar to that of the wheat diet and includes the following steps: (1) Boil water and dissolve agar in it. (2) Allow the mixture to cool down to 50°C. (3) Add leaf powder, Sorbic acid, and Methyl Parabenzene to the mixture. (4) Mix all the ingredients thoroughly. (5) Store the prepared mixture in Petri dishes or syringes in the refrigerator. All the ingredients can be found in Supplementary Table S2.
We used plastic syringes to maintain the freshness of the leaf powder diets, which made it easy to measure the amount of food served to the larvae. When opening the syringes, we cut off the tops and then covered them with parafilm before storing them in the refrigerator.
2.5 Experimental design
The eggs of both species (L. dispar and L. monacha) were placed separately in 250 mL plastic box containing a wheat control diet. They were kept at 20°C under a light:dark cycle of 16:8 h. Once the larvae emerged, they were allowed to feed on the wheat control diet until they reached the end of the second instar. Beginning in the third instar, the larvae were reared in groups of 15 within Petri dishes (ϕ6 cm) and provided with leaf powder diets. L. dispar larvae were fed both spruce and oak diets, while L. monacha larvae were fed only the spruce diet. We monitored the larvae hourly (besides night time (7:00 a.m.–10:00 p.m.), to identify the premolt stage, which is indicated by the larvae being stuck with their heads moved forward. When in the premolt stage, the larvae were transferred to individual Petri dishes containing a small piece of leaf powder diet (2 g). Immediately after molting into the fourth instar, we weighed both the larva and the piece of diet. During the fourth instar, the larvae were left to feed ad libitum for 5 days (the duration of the fourth instar at 20°C), with additional food provided if necessary. Once the larvae reached the premolt stage to the fifth instar, they were weighed, placed in small Eppendorf tubes, and frozen along with any remaining food and frass. The collected material was then dried in an incubator with blue desiccant and stored refrigerated at -20°C.
To ensure accurate measurements of evaporation-induced weight loss in artificial larval diets, we stored 10 samples of each diet type at 20°C for 5 days and determined the water loss thereafter. This information was then used to adjust our data analysis accordingly.
2.6 Evaluating larval performance
According to the gravimetric method of Waldbauer (1968), four nutritional indices were used to evaluate larval performance on the different diets (Table 1). These indices include AD (approximate digestibility), ECD (efficiency of conversion of digested food), ECI (conversion of ingested food), and RGR (relative growth rate) (Figure 1). All indices are calculated based on dry weights. Approximately 25 larvae were weighed immediately after molting from the third to the fourth instar (FW—Fresh weight). They were then frozen and dried (DW—Dry weight) to determine the FW/DW ratio. This ratio was used to estimate the dry weight of the larvae at the beginning of the fourth instar using the formula WC = [(FW-DW) × 100]/FW. To determine the dry weight of the food at the start of the observed period, we applied this formula to 10 pieces (2 g) of each type of diet (as described in the previous chapter), and the average of these results was used as the initial diet weight.
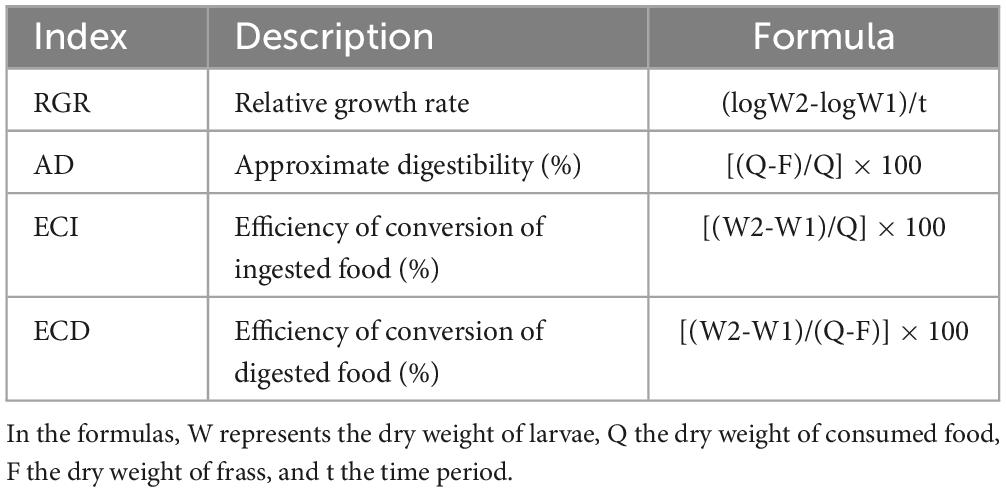
Table 1. Formulas of all nutritional indices used for evaluating larval performance, including their abbreviations.
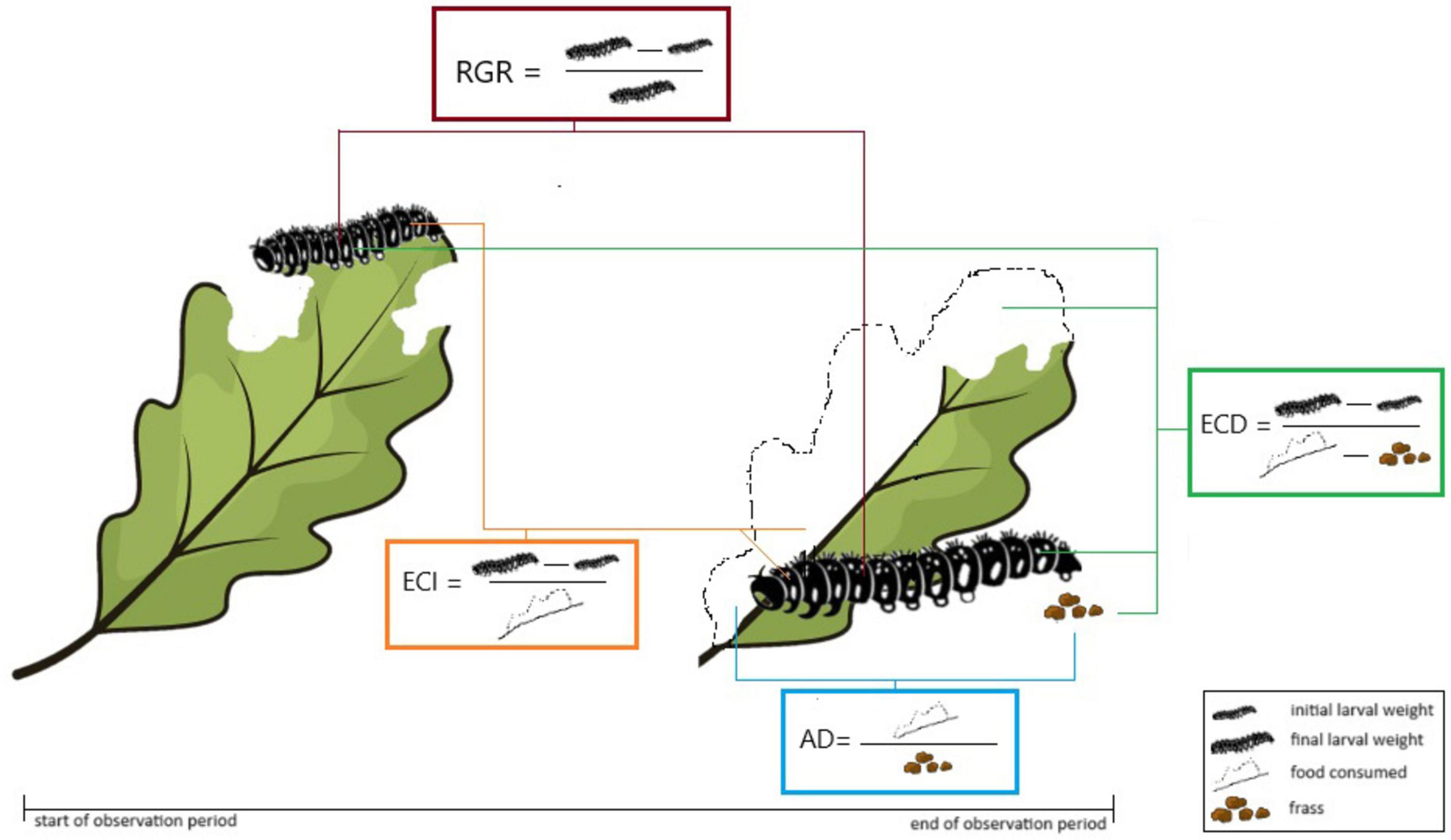
Figure 1. Four nutritional indices (ECI, RGR, ECD, AD) used to evaluate the performance of larvae on different types of leaf powder diet [inspired by Castagneyrol et al. (2018)]. The abbreviations and formulas for calculating these indices are presented in Table 1.
The relative growth rate (RGR) explains the amount of larval gain within a specific unit of time. Approximate digestibility (AD) measures the efficiency of food conversion. The efficiency of conversion of ingested food (ECI) is an overall index of the insect’s ability to utilize foliage for growth. The conversion efficiency of digested food (ECD) is an index of the insect’s ability to assimilate and convert food into body substances (Waldbauer, 1968).
2.7 Statistical analysis
Statistical analyses were performed using R statistical software (R Core Team, 2021). Principal Component Analysis (PCA) was conducted using the prcomp function from base R.
To assess the effects of different diets on larval growth, estimated by four nutritional indices, an analysis of variance (ANOVA) was first performed using the aov function. This compared the means of the selected indices across diet groups for each moth species separately. Tukey’s Honest Significant Difference (HSD) test was then applied to the ANOVA models to conduct pairwise comparisons between food groups, with the results summarized in plots using letter groupings (above each tasted treatment).
For further analysis of diet effects on nutritional indices, linear mixed-effects models were fitted for each growth index and moth species using the lmer function from the lme4 package (Bates et al., 2015). The initial models included tree species, cultivation temperature, and CO2 regimes as fixed effects, with individual food batches treated as random effects. Since only the tree species factor showed statistically significant differences, diets were categorized into three diet groups: control, oak-based and spruce-based. Subsequently, a simplified linear mixed-effects model was fitted with these diet categories as fixed effects and individual food batches as random effects. Than emmeans function from the emmeans package (Russell et al., 2021) was used to estimate marginal means and perform pairwise comparisons between diet categories. Results were visualized using letter groupings in summary plots (above each diet group).
To compare carbon (C), nitrogen (N), the carbon-to-nitrogen ratio (C:N), sugar compounds, and phenolic compounds among food batches, ANOVA models were employed. These included tree species, cultivation temperature, CO2 regimes, and interactions of tree species with sampling date, cultivation temperature and CO2 regimes as explanatory variables.
Effect sizes (η2 values) were calculated using the etaSquared function from the lsr package (Navarro and Navarro, 2022) to evaluate the contribution of individual factors and interactions to variation in the response variables. The η2 statistic quantifies the proportion of the total variance in a response variable that is explained by each explanatory variable or interaction in the model. This provides a standardized measure of effect size, allowing for the comparison of the relative importance of different factors.
For all models, the normality of residuals was checked. Several outlying values, likely due to measurement errors, were omitted, and the data were reanalyzed to ensure robustness.
3 Results
3.1 Nitrogen, carbon, and C:N ratio in spruce and oak
The nitrogen concentration varied significantly among tree species, with lower levels observed in spruce. This difference was logically reflected in the C:N ratio, which was significantly higher in spruce. Seedlings growing in doubled CO2 concentration had a higher C:N ratio. Seedlings growing at higher temperatures contained higher nitrogen content and didn’t affect the C:N ratio (Table 2 and Figure 2).
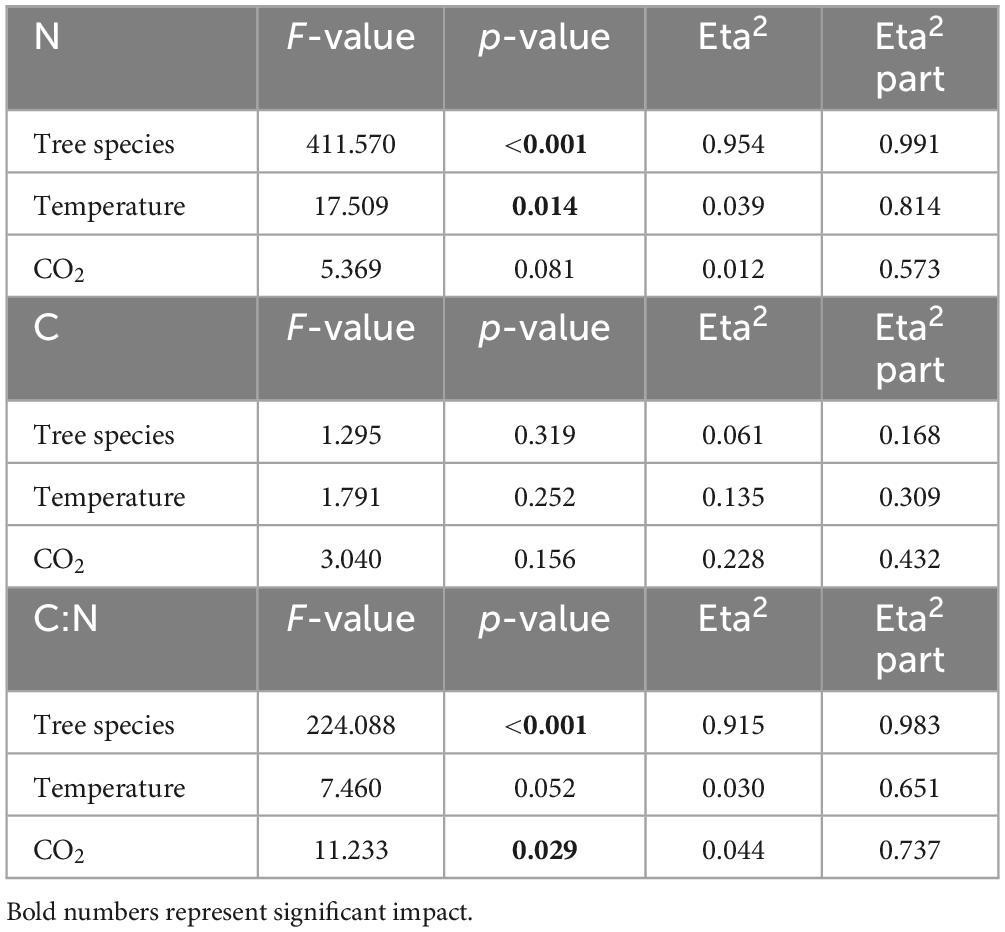
Table 2. Influence of tree species (spruce and oak), elevated temperature (20°C vs. 25°C), and doubled air CO2 concentration (410 ppm vs. 820 ppm) on nitrogen, carbon, and the C:N ratio in leaves.
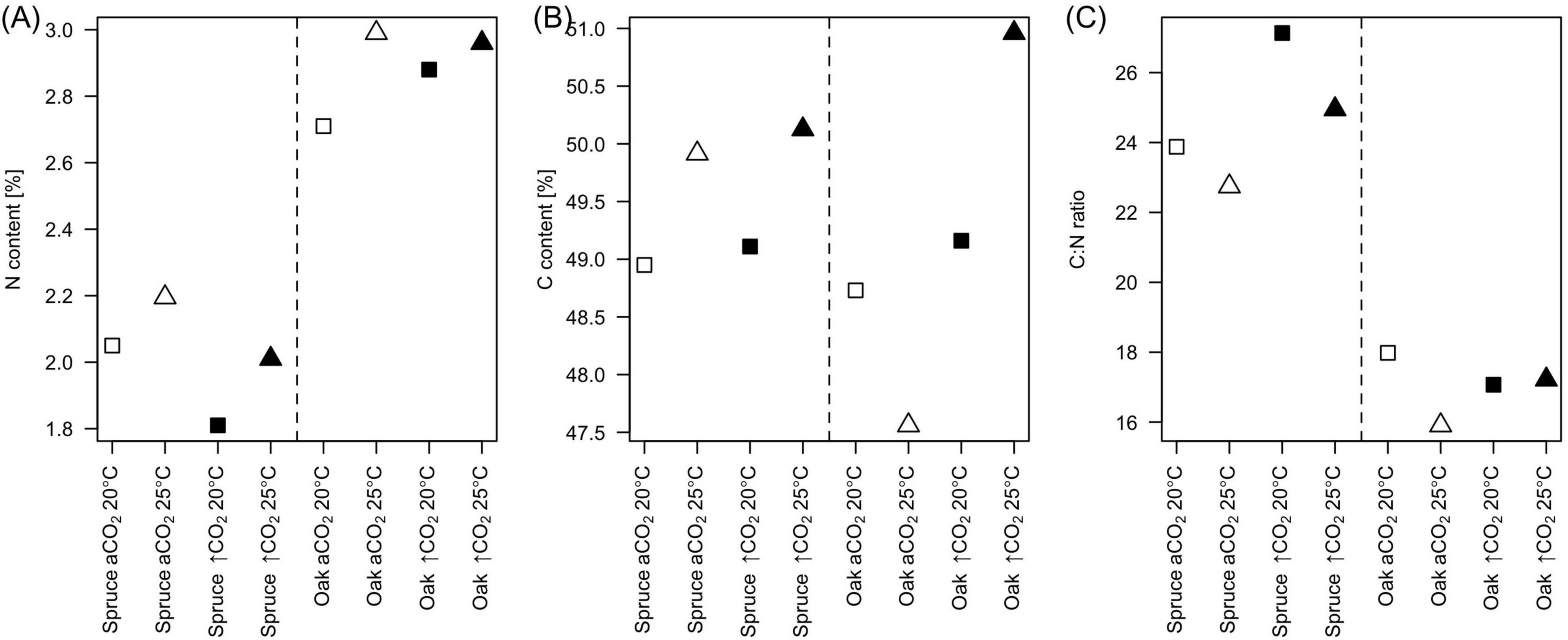
Figure 2. Influence of CO2 concentrations and temperature on the nitrogen (A), carbon (B) content and the C:N ratio (C) in leaf powder diets prepared from spruce and oak seedlings. In all cases, 36 samples were analyzed.
3.2 Chemical analysis of oak leaves and spruce needles
The leaves of oak seedlings contained higher levels of phenolics and condensed tannins compared to spruce needles. Spruce had higher flavonoid content. However, there was no significant difference in non-tannins between oak and spruce seedlings (Tables 3, 4).
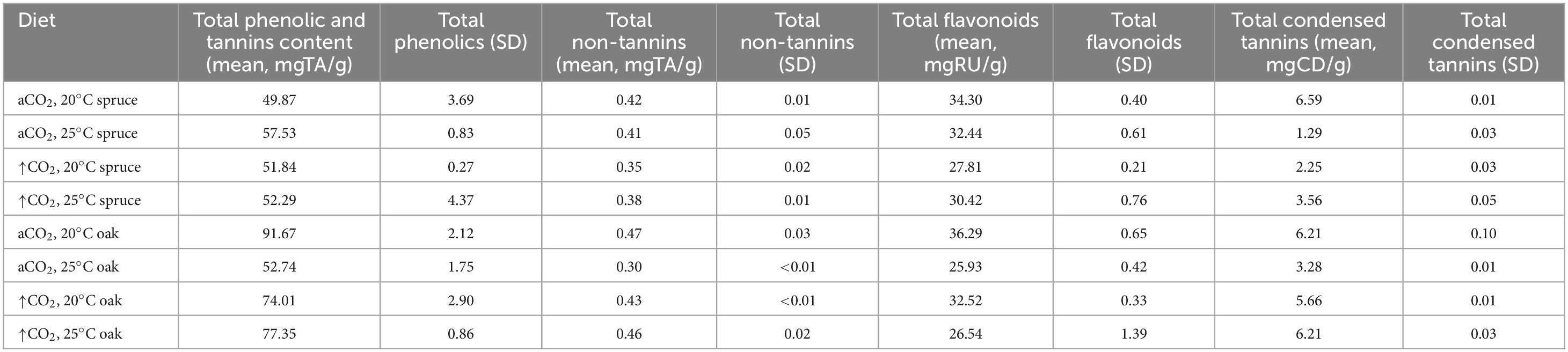
Table 3. Contents of secondary metabolites in leaves of oak (n = 36) and spruce (n = 72) seedlings grown under lower (20°C) and higher (25°C) temperatures, as well as ambient (410 ppm) and doubled (↑, 820 ppm) CO2 concentrations.
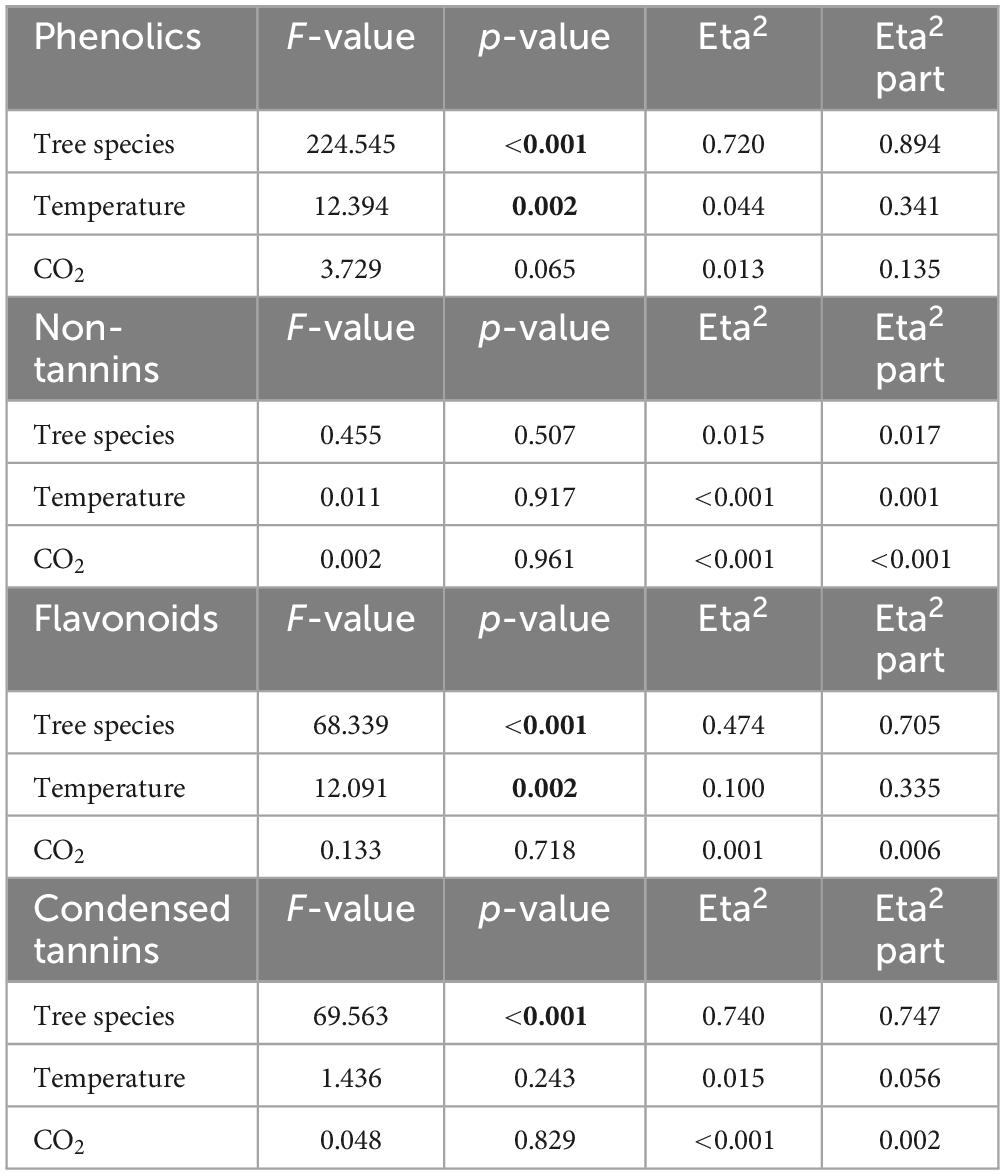
Table 4. Comparison of the contents of individual groups of secondary metabolites in leaves of oak (n = 36) and spruce (n = 72) seedlings grown under lower (20°C) and higher (25°C) temperatures, as well as ambient (410 ppm) and doubled (820 ppm) CO2 concentrations.
Higher temperatures affected phenolic and flavonoid concentrations. Phenolic concentrations increased at higher temperatures, except in oak under ambient CO2 concentration. Flavonoid concentrations lowered at higher temperatures except in spruce under doubled CO2 concentration (Tables 3, 4). The CO2 treatments didn’t affect any secondary metabolites we studied (Table 4).
Sucrose and raffinose concentrations were significantly higher in oak compared to spruce. The levels of all sugar types in diets from seedlings grown under both temperature regimes and in both ambient and doubled CO2 concentrations were similar (Tables 5, 6).
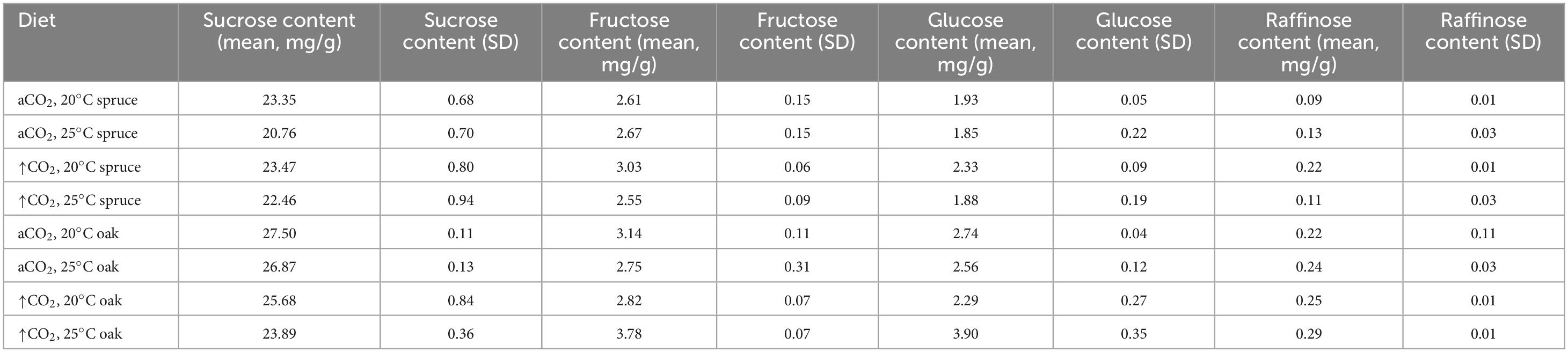
Table 5. Sugar contents in oak (n = 36) and spruce (n = 72) seedlings grown under lower and higher temperatures (20°C and 25°C), as well as ambient (410 ppm) and doubled (↑, 820 ppm) CO2 concentrations.
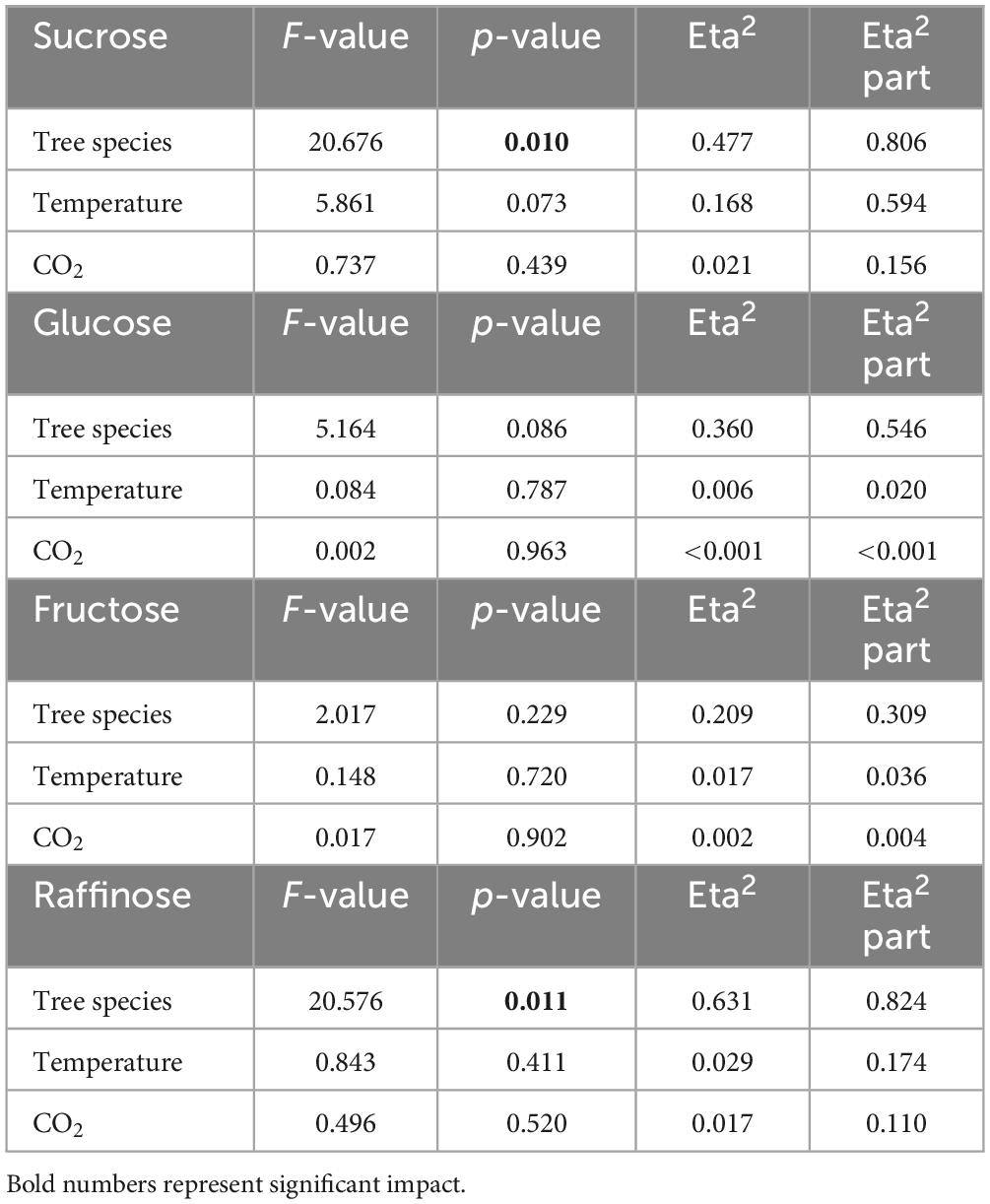
Table 6. Comparison of the sugar contents in oak (n = 36) and spruce (n = 72) seedlings grown under lower and higher temperatures (20°C and 25°C), as well as ambient (410 ppm) and doubled (820 ppm) CO2 concentrations.
3.3 The performance Lymantria species on different leaf powder diets prepared from oak (L. dispar) and spruce (L. dispar and L. monacha)
For L. dispar, the nutritional indices, except for the ECD, were significantly higher in larvae reared on oak diets compared to those reared on spruce diets. Except for the ECD index, the oak diet showed a significant difference when compared to the wheat control diet. Except for the ECD index, larvae reared on spruce diets performed worst.
Only a small influence of climatic conditions on L. dispar larval performance was found, but significant differences were found between different climatic conditions in three cases. AD index was higher for larvae reared on spruce needles grown at 20°C than at 25°C when growing in doubled CO2 concentration. ECI index was higher for larvae reared on spruce needles grown at 20°C and doubled CO2 concentration than other spruce variants. ECD index was lower for larvae reared on spruce needles grown at ambient CO2 and 20°C compared to all other spruce variants (Figure 3).
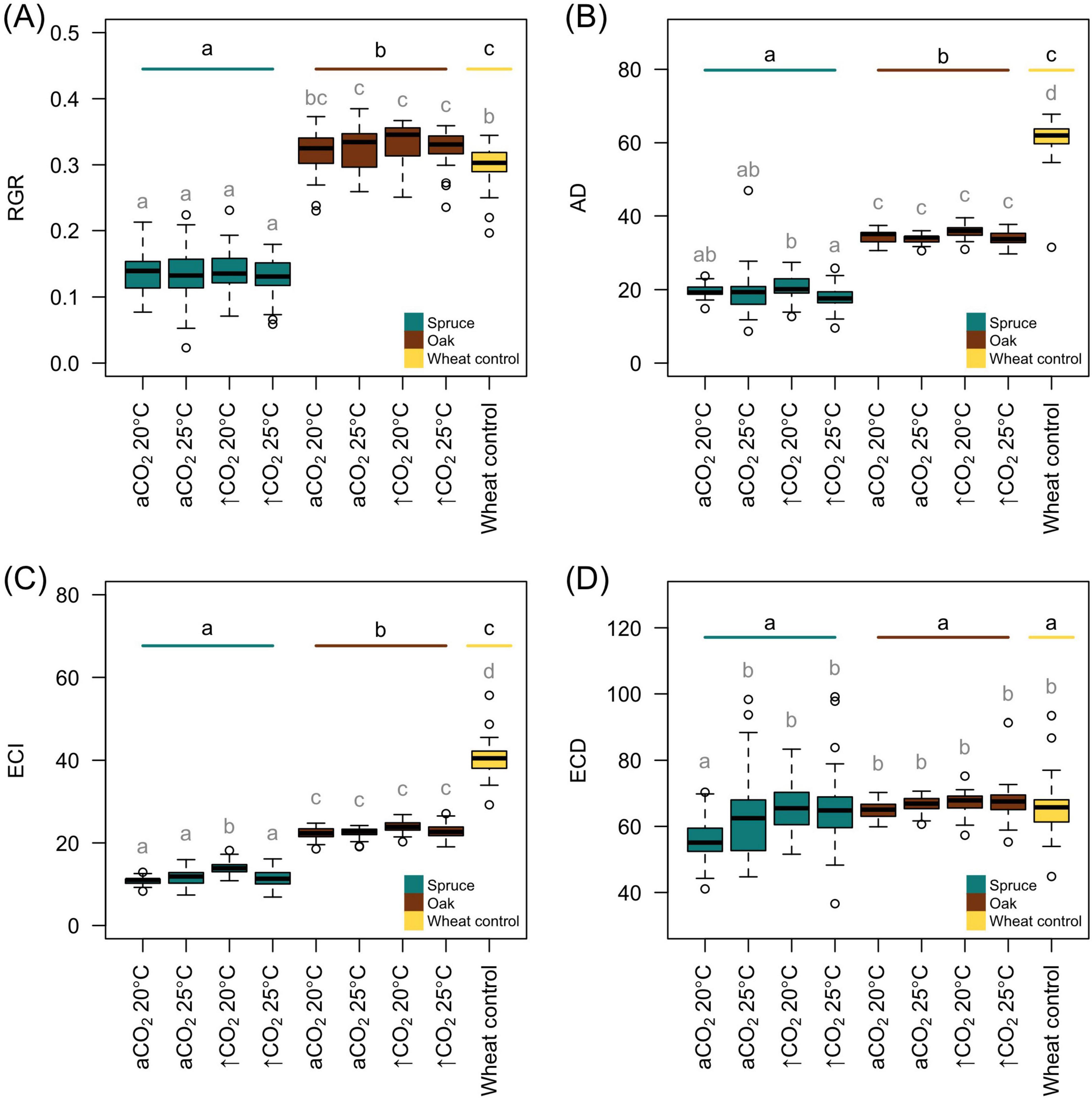
Figure 3. The influence of spruce and oak leaf powder diet from trees grown under two temperatures (20°C and 25°C) and two CO2 concentrations (410 ppm and 820 ppm) on the growth rate (RGR) (A), approximate digestibility (AD) (B), efficiency of conversion of ingested food to body substances (ECI) (C), and efficiency of conversion of digested food to body substances (ECD) (D) of L. dispar compared to the growth on wheat germ diet (controls) (after Bell, 1981). Colorful lines with different letters in the upper part of graphs summarize the significant differences between spruce, oak, and wheat control diet groups. Different gray letters above boxplots indicate significant differences between the tested treatments. For further details on the indices, refer to Table 1 and Figure 1.
For Lymantria monacha, the RGR, AD, and ECI indices were significantly higher in the wheat control diet than in the spruce needle powder diet.
Only a small influence of climatic conditions on L. monacha larval performance was found. Still, significant differences were found between different climatic conditions in three cases. RGR index was higher for larvae reared on spruce grown at ambient CO2 and 20°C than fed on spruce grown in doubled CO2 concentrations and both temperatures. AD index was higher for larvae reared on spruce grown in doubled CO2 when comparing both CO2 treatments at 20°C. ECD index was higher for larvae reared on spruce grown at ambient CO2 when comparing both CO2 treatments at 25°C (Figure 4).
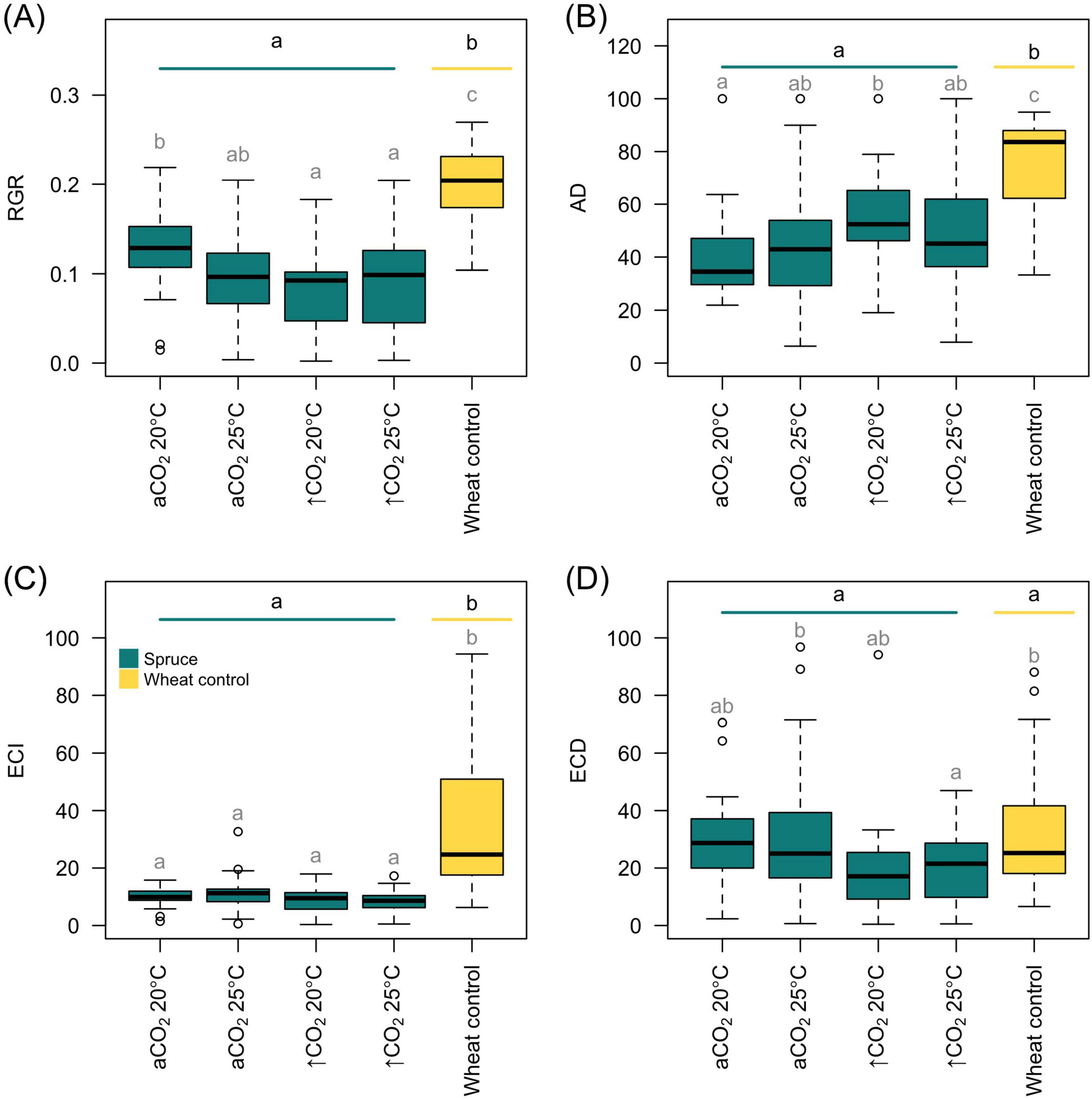
Figure 4. Influence of spruce leaf powder diet from trees grown under two temperatures (20°C and 25°C) and two CO2 concentrations (410 ppm and 820 ppm) on the growth rate (RGR) (A), approximate digestibility (AD) (B), efficiency of conversion of ingested food to body substances (ECI) (C), and efficiency of conversion of digested food to body substances (ECD) (D) of L. monacha compared to the growth on wheat germ diet (controls) (after Bell, 1981). Colorful lines with different letters in the upper part of graphs summarize the significant differences between spruce, oak, and wheat control diet groups. Different gray letters above boxplots indicate significant differences between the tested treatments. For further details on the indices, refer to Table 1 and Figure 1.
4 Discussion
In our experiment, seedlings of two tree species, spruce and oak, were reared under four different climatic conditions for 1 year. The study aimed to examine how these factors interact and their potential effects on the food quality for herbivorous insects. We compared a preferred host tree (oak) with a non-preferred host tree (spruce) and the response of Spongy moth (L. dispar) larval performance and conifer-preferring nun moth (L. monacha) on spruce, providing insight into how host plant selection may be influenced under future climate scenarios.
4.1 Effects of doubled CO2 concentration and higher temperature on C:N ratio and water soluble carbohydrates in seedlings
Our findings support the hypothesis that the plant C:N ratio in plants increases with elevated air CO2 concentrations, as observed in previous studies (Vencl et al., 2024; Zheng et al., 2019; Lotfiomran et al., 2016; Du et al., 2019; Hikosaka et al., 2006; Luo et al., 2006). The extent of this impact can vary based on plant species (Kubiske et al., 2015), growth conditions, and other environmental factors (Gifford et al., 2000).
Understanding how climate warming affects ecosystem function and stability is crucial for predicting the impacts of global climate change (Liu et al., 2021). Our results showed that seedlings grown at higher temperatures had increased nitrogen concentrations in their leaves and needles. This finding aligns with research by Yuan et al. (2021), which reported an almost 8% decrease in the C:N ratio at elevated temperatures in Quercus mongolica Fisch. ex Turcz. and Fraxinus mandschurica Rupr. Similarly, Zhang et al. (2011) indicated that increased nitrogen concentrations in leaves at higher temperatures are linked to enhanced photosynthesis and a greater capacity for nitrogen uptake. This mechanism likely explains our findings, where doubled CO2 levels did not result in a significant increase in carbon content in the seedlings.
The response of the C:N ratio varied between tree species. Spruce seedlings exhibited a higher C:N ratio compared to oak trees, which had correspondingly higher nitrogen concentrations in their leaves. The low C:N ratio in oak indicates a greater investment in proteins essential for photosynthesis (Zhang et al., 2020). Additionally, oak leaves contained a higher content of certain sugars, particularly sucrose and raffinose. These sugars are critical products of photosynthesis, providing carbon and energy necessary for synthesizing secondary metabolites (Rolland et al., 2006). Compounds such as phenols, flavonoids, and condensed tannins are synthesized via the shikimate pathway, which relies on intermediates derived from sugar metabolism, including phosphoenolpyruvate and erythrose-4-phosphate as primary substrates (Marchiosi et al., 2020). This relationship may explain why oak, with its higher sugar content, also exhibited elevated levels of these carbon-based secondary metabolites.
4.2 Effects of doubled CO2 concentration and higher temperature on plant secondary metabolites
4.2.1 Phenolics
Our research shows that higher temperatures in both tree species, along with doubled CO2 in oak, lead to an increase in phenolic concentrations. Only one opposite finding was in oak at ambient CO2 with rising temperature, which aligns with a meta-analysis by Zvereva and Kozlov (2006) and a review by Julkunen-Tiitto et al. (2015), which stated that phenolic concentrations tend to decrease as temperatures rise under ambient CO2 conditions. In contrast, spruce needles exhibited a different response under ambient conditions, with no significant differences observed in seedlings exposed to elevated CO2 levels, as reported in previous studies (Koricheva et al., 1998; Zvereva and Kozlov, 2006; Lindroth, 2012; Julkunen-Tiitto et al., 2015; Robinson et al., 2012). Moreover, our findings indicate that the interaction between CO2 and temperature can interfere with each other’s effects, suggesting that the impact of these factors varies depending on the specific context (Julkunen-Tiitto et al., 2015; Zvereva and Kozlov, 2006; Veteli et al., 2007).
4.2.2 Flavonoids
We observed that flavonoid levels significantly decreased at higher temperatures in oak leaves under both ambient and elevated CO2 conditions. This finding is consistent with the research conducted by Julkunen-Tiitto et al. (2015). In contrast, we found an opposing result in spruce needles grown under doubled CO2 concentration, where the flavonoid content was higher at 25°C compared to 20°C. This trend was also reported in studies by Li et al. (2011) and Shamloo et al. (2017). Additionally, Holopainen et al. (2018) mentioned that elevated CO2 levels should increase flavonoid concentrations, although this effect is weaker than that of temperature changes. This discrepancy may help explain the differences we observed, but a similar trend was not found in oak trees. Some studies, including those by Holopainen et al. (2018) and Julkunen-Tiitto et al. (2015), indicated that the combination of temperature and CO2 had no significant impact on flavonoid levels.
In summary, our findings indicate that the effects of climate change factors, such as elevated temperatures and increased CO2 concentrations, on secondary metabolites are highly variable and depend on the specific context. The responses of phenolics and flavonoids differ, suggesting that the interaction between CO2 levels and temperature can either enhance or reduce the impacts on plant metabolites. Further studies with a longer exposure period of the trees under the corresponding temperature and CO2 conditions could possibly provide a clearer picture of the factors influencing the changes in leaf constituents and contribute to a better understanding of the complex interactions between climate factors and their effects on plant chemistry.
4.3 Effects of doubled CO2 concentration and higher temperature on larval performance
Both Lymantria species examined are polyphagous insects, with L. dispar preferring deciduous trees and L. monacha preferring coniferous species as their host trees. The larvae of L. dispar have been reared in a laboratory for decades on the wheat germ diet, which was used as a control diet in our study, while the nun moth larvae were collected from the field. Therefore, it was not surprising that in the gravimetric analyses, the spongy moth larvae showed the highest AD and ECI values on the artificial wheat control diet, which lacked the secondary metabolites found in their natural food sources. Interestingly, development on the high wheat germ diet showed similar RGR values to those on the oak powder diet; however, both diets produced RGR values that were more than twice as high as those on the spruce powder diet. The effects of different temperatures and CO2 exposure on the trees were only evident with the less suitable spruce needle diet.
The reason why the effects were evident only in spruce can be explained by Stowe and Osborn (1980), as they reported that the toxicity of phenolic compounds is closely linked to nutrient concentrations. Specifically, phenolic acids have been shown to inhibit growth only at low nutrient levels significantly. This helps clarify why L. dispar performs better on oak, despite its higher levels of phenolics, and condensed tannins (see Barbehenn et al., 2013; Milanović et al., 2015). Additionally, in another study, L. dispar demonstrated significantly better performance on white alder (Alnus rhombifolia Nutt.) compared to Douglas-fir [Pseudotsuga menziesii (Mirb.) Franco] (Joseph et al., 1991). Interestingly, the alder had higher levels of phenolic compounds and greater nitrogen content, contributing to its overall nutritional value (Joseph et al., 1991; also see Hättenschwiler and Schafellner, 1999).
For L. dispar, the effects of increased CO2 on ECI and ECD were more pronounced at the lower temperature (20°C) compared to the higher temperature (25°C). This finding is supported by higher flavonoid levels in spruce trees at higher temperatures with doubled CO2 levels. Conversely, flavonoid concentrations decreased at lower temperatures, which allowed the larvae to perform better when exposed to doubled CO2 concentration in spruce. Additionally, for L. monacha, the lower temperature (20°C) seems to positively impact larval performance at ambient CO2 concentrations, resulting in higher RGR and ECD. This effect can be attributed to the higher phenol concentrations in the needles from trees at higher temperatures, thereby underscoring the role of secondary metabolites in influencing larval performance.
The diet of larvae from oak trees also contained higher levels of sugars, particularly sucrose and raffinose. Glucose seemed to be higher in oak in most cases, especially when temperatures were higher. Still, the result is not significant, probably because the numbers are similar to those of spruce, and the amount of oak is much higher in ambient CO2 and lower temperature treatment. This likely explains why L. dispar larvae performed better on oak than on spruce. Sugars serve as a primary energy source for insects (see Skowronek et al., 2021). Simple sugars like glucose and fructose are quickly metabolized, providing immediate energy necessary for essential activities such as growth, movement, and reproduction (Sheng et al., 2019; Mayack et al., 2020).
The overall quality of a plant as a food source is influenced by several factors, including the balance of nutrients, the presence of secondary metabolites, and the plant’s digestibility (Scogings et al., 2013; Wink, 2018). The artificial diet was prepared by powdering lyophilized leaves, a standardized method for the chemical analysis of leaves (Šulc et al., 2021). However, this method overlooks physiological factors that are also crucial in plant-herbivore interactions. For instance, leaf hardness, which can be increased by higher levels of structural carbohydrates resulting from elevated CO2 concentrations (Prior et al., 2004; Gely et al., 2020; Münzbergová and Skuhrovec, 2020), cannot be taken into account when using dried leaves.
Escalating temperatures lead to the combined effects of drought and heat waves (Sato et al., 2024; Cook et al., 2018). These factors have become significant direct consequences that severely impact ecosystems (Bailey-Serres et al., 2019). Including drought as an additional variable in studies like ours would provide more complex insights into the effects of climate change on forest ecosystems (Clark et al., 2016). Our study focused on elevated CO2 concentrations in the atmosphere and their direct consequence: higher air temperatures. The results indicate that trees respond to changes in climate conditions, which in turn affects their chemical composition. However, herbivorous insects can still thrive on these trees despite variations in nutritional quality, suggesting that climate change does not significantly impact their performance via this indirect effect.
Data availability statement
The raw data supporting the conclusions of this article will be made available by the authors, without undue reservation.
Ethics statement
Ethical approval was not required for the studies involving animals in accordance with the local legislation and institutional requirements because the partner who sent us the eggs of larvae breeds them especially for this kind of experiments. Written informed consent was obtained from the owners for the participation of their animals in this study.
Author contributions
BD: Conceptualization, Funding acquisition, Investigation, Methodology, Validation, Visualization, Writing – original draft, Writing – review & editing. JHo: Conceptualization, Investigation, Methodology, Supervision, Validation, Visualization, Writing – original draft, Writing – review & editing. DM: Investigation, Methodology, Writing – review & editing. AK: Formal Analysis, Validation, Writing – review & editing. JHr: Formal Analysis, Validation, Writing – review & editing. JČ: Data curation, Formal Analysis, Visualization, Writing – review & editing. AS: Conceptualization, Methodology, Resources, Supervision, Validation, Writing – review & editing.
Funding
The author(s) declare that financial support was received for the research and/or publication of this article. This research was supported by the European Regional Development Fund, project EVA4.0, No. CZ.02.1.01/0.0/0.0/16_019/0000803 and by the Czech University of Life Sciences Prague project No. IGA A_01_23.
Acknowledgments
We are grateful to Soňa Knířová for her work on the methodology.
Conflict of interest
The authors declare that the research was conducted in the absence of any commercial or financial relationships that could be construed as a potential conflict of interest.
Generative AI statement
The authors declare that no Generative AI was used in the creation of this manuscript.
Publisher’s note
All claims expressed in this article are solely those of the authors and do not necessarily represent those of their affiliated organizations, or those of the publisher, the editors and the reviewers. Any product that may be evaluated in this article, or claim that may be made by its manufacturer, is not guaranteed or endorsed by the publisher.
Supplementary material
The Supplementary Material for this article can be found online at: https://www.frontiersin.org/articles/10.3389/ffgc.2025.1564011/full#supplementary-material
References
Adak, S., Mandal, N., Mukhopadhyay, A., Maity, P. P., and Sen, S. (2023). “Current state and prediction of future global climate change and variability in terms of CO2 levels and temperature,” in Enhancing Resilience of Dryland Agriculture Under Changing Climate: Interdisciplinary and Convergence Approaches, eds A. Naorem and D. Machiwalpp (Singapore: Springer Nature Singapore), 15–43.
Bae, J., Byun, C., Ahn, Y. G., Choi, J. H., Lee, D., and Kang, H. (2019). Effect of elevated atmospheric carbon dioxide on the allelopathic potential of common ragweed. J. Ecol. Environ. 43:21. doi: 10.1186/s41610-019-0116-5
Bailey-Serres, J., Parker, J., Ainsworth, E., Oldroyd, G., and Schroeder, J. (2019). Genetic strategies for improving crop yields. Nature 575, 109–118. doi: 10.1038/s41586-019-1679-0
Bala, K., Sood, A. K., Pathania, V. S., and Thakur, S. (2018). Effect of plant nutrition in insect pest management: A review. J. Pharmacognosy Phytochem. 7, 2737–2742.
Bale, J. S., Masters, G. J., Hodkinson, I. D., Awmack, C., Bezemer, T. M., Brown, V. K., et al. (2002). Herbivory in global climate change research: Direct effects of rising temperature on insect herbivores. Global Change Biol. 8, 1–16. doi: 10.1046/j.1365-2486.2002.00451.x
Barbehenn, R. V., Niewiadomski, J., Pecci, C., and Salminen, J. P. (2013). Physiological benefits of feeding in the spring by Lymantria dispar caterpillars on red oak and sugar maple leaves: Nutrition versus oxidative stress. Chemoecology 23, 59–70. doi: 10.1007/s00049-012-0119-5
Barbehenn, R., and Constabel, C. (2011). Tannins in plant-herbivore interactions. Phytochemistry 72, 1551–1565. doi: 10.1016/j.phytochem.2011.01.040
Bates, D., Maechler, M., Bolker, B., Walker, S., Christensen, R. H. B., Singmann, H., et al. (2015). Package ‘lme4’. Convergence 12:2. doi: 10.18637/jss.v067.i01
Begum, R. A., Raihan, A., and Said, M. N. M. (2020). Dynamic impacts of economic growth and forested area on carbon dioxide emissions in Malaysia. Sustainability 12:9375. doi: 10.3390/su12229375
Bell, R. A. (1981). “Development of mass-rearing technology,” in The gypsy moth: Research toward integrated pest management, eds C. C. Doane and M. L. McManus (Washington, DC: U.S. Department of Agriculture, Forest Service and Education Agency), 599–655.
Blois, J., Zarnetske, P., Fitzpatrick, M., and Finnegan, S. (2013). Climate change and the past, present, and future of biotic interactions. Science 341, 499–504. doi: 10.1126/science.1237184
Boeckler, G., Gershenzon, J., and Unsicker, S. (2011). Phenolic glycosides of the Salicaceae and their role as anti-herbivore defenses. Phytochemistry 72, 1497–1509. doi: 10.1016/j.phytochem.2011.01.038
Bonan, G. (2008). Forests and climate change: Forcings, feedbacks, and the climate benefits of forests. Science 320, 1444–1449. doi: 10.1126/science.1155121
Boullis, A., Francis, F., and Verheggen, F. (2015). Climate change and tritrophic interactions: Will modifications to greenhouse gas emissions increase the vulnerability of herbivorous insects to natural enemies? Environ. Entomol. 44, 277–286. doi: 10.1093/ee/nvu019
Bringaud, F., Rivière, L., and Coustou, V. (2006). Energy metabolism of trypanosomatids: Adaptation to available carbon sources. Mol Biochem Parasitol. 149, 1–9. doi: 10.1016/j.molbiopara.2006.03.017
Castagneyrol, B., Moreira, X., and Jactel, H. (2018). Drought and plant neighbourhood interactively determine herbivore consumption and performance. Sci. Rep. 8:5930. doi: 10.1038/s41598-018-24299-x
Castedo-Dorado, F., Lago-Parra, G., Lombardero, M. J., Liebhold, A. M., and Álvarez-Taboada, M. F. (2016). European gypsy moth (Lymantria dispar) completes development and defoliates exotic radiata pine plantations in Spain. N. Z. J. For. Sci. 46, 1–6. doi: 10.1186/s40490-016-0074-y
Ceulemans, R., and Mousseau, M. (1994). Tansley review No. 71: Effects of elevated atmospheric CO2 on woody plants. New Phytol. 127, 425–446. doi: 10.1111/j.1469-8137.1994.tb03961.x
Charfeddine, L. (2017). The impact of energy consumption and economic development on ecological footprint and CO2 emissions: Evidence from a Markov switching equilibrium correction model. Energy Econ. 65, 355–374. doi: 10.1016/j.eneco.2017.05.009
Chen, F., Wu, G., Ge, F., Parajulee, M. N., and Shrestha, R. B. (2005). Effects of elevated CO2 and transgenic Bt cotton on plant chemistry, performance, and feeding of an insect herbivore, the cotton bollworm. Entomol. Exp. Appl. 115, 341–350. doi: 10.1111/j.1744-7909.2007.00472_1.x
Ciais, P., Sabine, C., Bala, G., Bopp, L., Brovkin, V., Canadell, J., et al. (2013). “Carbon and other biogeochemical cycles,” in Climate Change 2013: The Physical Science Basis. Contribution of Working Group I to the Fifth Assessment Report of the Intergovernmental Panel on Climate Change, eds M. Tignor, S. K. Allen, and J. Boschung (Cambridge: Cambridge University Press), 465–570.
Clark, J., Iverson, L., Woodall, C., Allen, C., Bell, D., Bragg, D., et al. (2016). The impacts of increasing drought on forest dynamics, structure, and biodiversity in the United States. Glob. Chang. Biol. 22, 2329–2352. doi: 10.1111/gcb.13160
Cook, B. I., Mankin, J. S., and Anchukaitis, K. J. (2018). Climate change and drought: From past to future. Curr. Climate Change Rep. 4, 164–179. doi: 10.1007/s40641-018-0093-2
Croteau, R., Kutchan, T. M., and Lewis, N. G. (2000). “Natural products (secondary metabolites),” in Biochemistry and molecular biology of plants, eds B. Buchanan, W. Gruissem, and R. Jones 1250–1318.
Curtis, P. S., Zak, D. R., Pregitzer, K. S., Lussenhop, J., and Teeri, J. A. (1996). “Linking above- and belowground responses to rising CO2 in northern deciduous forest species,” in Carbon dioxide and terrestrial ecosystems, eds G. W. Koch and H. A. Mooney (San Diego, CA: Academic Press), 41–51.
Curtis, P., and Wang, X. A. (1998). meta-analysis of elevated CO2 effects on woody plant mass, form, and physiology. Oecologia 113, 299–313. doi: 10.1007/s004420050381
Diffenbaugh, N., Singh, D., Mankin, J., Horton, D., Swain, D., Touma, D., et al. (2017). Quantifying the influence of global warming on unprecedented extreme climate events. Proc. Natl. Acad. Sci. U S A. 114, 4881–4886. doi: 10.1073/pnas.1618082114
Doane, C. C., and McManus, M. L. (1981). The Gypsy Moth: Research Toward Integrated Pest Management. No. 1584. Washington, DC: U.S. Department of Agriculture.
Du, C., Wang, X., Zhang, M., Jing, J., and Gao, Y. (2019). Effects of elevated CO2 on plant C-N-P stoichiometry in terrestrial ecosystems: A meta-analysis. Sci. Total Environ. 650, 697–708. doi: 10.1016/j.scitotenv.2018.09.051
Ehleringer, J., Cerling, T., and Dearing, M. (2002). Atmospheric CO2 as a global change driver influencing plant-animal interactions. Integr. Comp. Biol. 42, 424–430. doi: 10.1093/icb/42.3.424
Elkinton, J. S., and Liebhold, A. M. (1990). Population dynamics of gypsy moth in North America. Annu. Rev. Entomol. 35, 571–596. doi: 10.1146/annurev.en.35.010190.003035
Ellsworth, D. S., Reich, P. B., Naumburg, E. S., Koch, G. W., Kubiske, M. E., and Smith, S. D. (2004). Photosynthesis, carboxylation and leaf nitrogen responses of 16 species to elevated CO2 across four free-air CO2 enrichment experiments in forest, grassland and desert. Glob. Change Biol. 10, 2121–2138. doi: 10.1111/j.1365-2486.2004.00867.x
Farrar, J. F., and Williams, M. L. (1991). The effects of increased atmospheric carbon dioxide and temperature on carbon partitioning, source-sink relations and respiration. Plant Cell Environ. 14, 819–830. doi: 10.1111/j.1365-3040.1991.tb01445.x
Foss, A., Mattson, W., and Trier, T. (2013). Effects of elevated CO2 leaf diets on gypsy moth (Lepidoptera: Lymantriidae) respiration rates. Environ. Entomol. 42, 503–514. doi: 10.1603/EN12074
Gely, C., Laurance, S., and Stork, N. (2020). How do herbivorous insects respond to drought stress in trees? Biol. Rev. Camb. Philos. Soc. 95, 434–448. doi: 10.1111/brv.12571
Gifford, R. M., Barrett, D. J., and Lutze, J. L. (2000). The effects of elevated [CO2] on the C:N and C:P mass ratios of plant tissues. Plant and Soil 224, 1–14.
Giri, C. C. (2017). “Biodiversity and climate change: Tree the saviour of future,” in Insights on global challenges and opportunities for the century ahead, eds V. Dashavantha, K Reddy, and Venkateswara Rao K. Rama Krishna (Hyderabad: BSP Books Pvt. Limited), 303.
Guo, Y., Zhang, L., Yang, L., Shen, W., Pan, Y., Wright, I., et al. (2022). Enhanced leaf turnover and nitrogen recycling sustain CO2 fertilization effect on tree-ring growth. Nat. Ecol. Evol. 6, 1271–1278. doi: 10.1038/s41559-022-01811-1
Hartmann, H., Moura, C., Anderegg, W., Ruehr, N., Salmon, Y., Allen, C., et al. (2018). Research frontiers for improving our understanding of drought-induced tree and forest mortality. New Phytol. 218, 15–28. doi: 10.1111/nph.15048
Hättenschwiler, S., and Schafellner, C. (1999). Opposing effects of elevated CO2 and N deposition on Lymantria monacha larvae feeding on spruce trees. Oecologia 118, 210–217. doi: 10.1007/s004420050720
Henn, M. W., and Schopf, R. (2001). Response of beech (Fagus sylvatica) to elevated CO2 and N: Influence on larval performance of the gypsy moth Lymantria dispar (Lepidoptera, Lymantriidae). J. Appl. Entomol. 125, 501–505. doi: 10.1046/j.1439-0418.2001.00592.x
Hikosaka, K., Ishikawa, K., Borjigidai, A., Muller, O., and Onoda, Y. (2006). Temperature acclimation of photosynthesis: mechanisms involved in the changes in temperature dependence of photosynthetic rate. J. Exp. Bot. 57, 291–302. doi: 10.1093/jxb/erj049
Hlásny, T., Trombik, J., Holuša, J., Lukášová, K., Grendár, M., Turčáni, M., et al. (2016). Multi-decade patterns of gypsy moth fluctuations in the Carpathian Mountains and options for outbreak forecasting. J. Pest Sci. 89, 13–25. doi: 10.1007/s10340-015-0694-7
Holopainen, J., Virjamo, V., Ghimire, R., Blande, J., Julkunen-Tiitto, R., and Kivimäenpää, M. (2018). Climate change effects on secondary compounds of forest trees in the northern hemisphere. Front. Plant Sci. 9:1445. doi: 10.3389/fpls.2018.01445
Honkaniemi, J., Rammer, W., and Seidl, R. (2020). Norway spruce at the trailing edge: the effect of landscape configuration and composition on climate resilience. Landsc. Ecol. 35, 591–606. doi: 10.1007/s10980-019-00964-y
International Energy Agency [IEA]. (2017). CO2 emissions from fuel combustion 2017 - highlights. Int. Energy Agency 1, 1–162. doi: 10.1787/co2_fuel-2017-en
IPCC. (2014). “Impacts, adaptation and vulnerability,” in Working Group II Contribution to the Fifth Assessment Report of the Intergovernmental Panel on Climate Change, eds C. B. Field, V. R. Barros, D. J. Dokken, K. J. Mach, M. D. Mastrandrea, T. E. Bilir, et al. (Cambridge: Cambridge University Press), 1132.
IPPC. (2021). Strategic Framework for the International Plant Protection Convention (IPPC) 2020–2030. Italy: FAO.
Jactel, H., Koricheva, J., and Castagneyrol, B. (2019). Responses of forest insect pests to climate change: not so simple. Curr. Opin. Insect. Sci. 35, 103–108. doi: 10.1016/j.cois.2019.07.010
Jansson, G., Danusevièius, D., Grotehusman, H., Kowalczyk, J., Krajmerova, D., Skrøppa, T., et al. (2013). Forest Tree Breeding in Europe: Current State-of-the-Art and Perspectives, ed. H. Karst (Berlin: Springer), 123–176.
Johnson, S., and McNicol, J. (2010). Elevated CO2 and aboveground-belowground herbivory by the clover root weevil. Oecologia 162, 209–216. doi: 10.1007/s00442-009-1428-4
Johnson, S., Lopaticki, G., and Hartley, S. (2014). Elevated atmospheric CO2 triggers compensatory feeding by root herbivores on a C3 but not a C4 grass. PLoS One 9:e90251. doi: 10.1371/journal.pone.0090251
Joseph, G., Miller, J., Berry, R., Wernz, J., Moldenke, A., and Kelsey, R. (1991). White alder and Douglas-fir foliage quality and interegg-mass influences on larval development of gypsy moth Lymantria dispar. J. Chem. Ecol. 17, 1783–1799. doi: 10.1007/BF00993728
Julkunen-Tiitto, R., Nenadis, N., Neugart, S., Robson, M., Agati, G., Vepsäläinen, J., et al. (2015). Assessing the response of plant flavonoids to UV radiation: An overview of appropriate techniques. Phytochem. Rev. 14, 273–297. doi: 10.1007/s11101-014-9362-4
Kaiser, E., Morales, A., Harbinson, J., Kromdijk, J., Heuvelink, E., and Marcelis, L. (2015). Dynamic photosynthesis in different environmental conditions. J. Exp. Bot. 66, 2415–2426. doi: 10.1093/jxb/eru406
Karnosky, D. (2003). Impacts of elevated atmospheric CO(2) on forest trees and forest ecosystems: Knowledge gaps. Environ. Int. 29, 161–169. doi: 10.1016/S0160-4120(02)00159-9
Karnosky, D. F., Gielen, B., Ceulemans, R., Schlesinger, W. H., Norby, R. J., Oksanen, E., et al. (2001). “FACE systems for studying the impacts of greenhouse gases on forest ecosystems,” in The impact of carbon dioxide and other greenhouse gases on forest ecosystems, eds D. F. Karnosky, R. Ceulemans, G. E. Scarascia-Mugnozza, and J. L. Innes (Wallingford: CABI Publishing), 297–324.
Keeling, R. F. (1995). The atmospheric oxygen cycle: The oxygen isotopes of atmospheric CO2 and O2 and the O2/N2 ratio. Rev. Geophys. 33, 1253–1262. doi: 10.1029/95RG00438
Keena, M., Grinberg, P., and Wallner, W. (2007). Inheritance of female flight in Lymantria dispar (Lepidoptera: Lymantriidae). Environ. Entomol. 36, 484–494. doi: 10.1603/0046-225x200736[484:ioffil]2.0.co;2
Kellomäki, S., and Väisänen, H. (1988). Dynamics of branch population in the canopy of young Scots pine stands. For. Ecol. Manag. 24, 67–83. doi: 10.1016/0378-1127(88)90025-4
Klein, T., Bader, M. K. F., Leuzinger, S., Mildner, M., Schleppi, P., Siegwolf, R. T., et al. (2016). Growth and carbon relations of mature Picea abies trees under 5 years of free-air CO2 enrichment. J. Ecol. 104, 1720–1733. doi: 10.1111/1365-2745.12621
Komárek, J. (1931). Mnišková kalamita v létech 1917–1927 [The nun moth calamity during 1917–1927]. Sborník Výzkumných ústavů zemědělských ČSR 78:256.
Koricheva, J., Larsson, S., and Haukioja, E. (1998). Insect performance on experimentally stressed woody plants: A meta-analysis. Annu. Rev. Entomol. 43, 195–216. doi: 10.1146/annurev.ento.43.1.195
Körner, C., Asshoff, R., Bignucolo, O., Hättenschwiler, S., Keel, S., Peláez-Riedl, S., et al. (2005). Carbon flux and growth in mature deciduous forest trees exposed to elevated CO2. Science 309, 1360–1362. doi: 10.1126/science.1113977
Kubiske, M. E., Foss, A. R., Burton, A. J., Jones, W. S., Lewin, K. F., Nagy, J., et al. (2015). Supporting 13 years of global change research: the history, technology, and methods of the Aspen FACE experiment. Gen. Tech. Rep. NRS-153. Newtown Square, PA: US Department of Agriculture, Forest Service, Northern Research Station, 50.
Li, C. M., Wang, Y., and Yu, W. X. (2011). Dynamic changes of phenolic compound contents in leaf and bark of poplar during autumn temperature drop. J. For. Res. 22, 481–485. doi: 10.1007/s11676-011-0191-7
Liebhold, A. M., Elkinton, J. S., Zhou, C., Hohn, M. E., Rossi, R. E., Boettner, G. H., et al. (1995). Regional correlation of gypsy moth (Lepidoptera: Lymantriidae) defoliation with counts of egg masses, pupae, and male moths. Environ. Entomol. 24, 193–203. doi: 10.1093/ee/24.2.193
Lincoln, D. E., Sionit, N., and Strain, B. R. (1984). Growth and feeding response of Pseudoplusia includens (Lepidoptera: Noctuidae) to host plants grown in controlled carbon dioxide atmospheres. Environ. Entomol. 13, 1527–1530. doi: 10.1093/ee/13.6.1527
Lindroth, R. L. (2012). “Atmospheric change, plant secondary metabolites, and ecological interactions,” in The ecology of plant secondary metabolites: From genes to global processes, eds G. R. Iason, M. Dicke, and S. E. Hartley (Cambridge: Cambridge University Press), 120–153.
Lindroth, R. L., Kinney, K. K., and Platz, C. L. (1993). Responses of deciduous trees to elevated atmospheric CO2: Productivity, phytochemistry, and insect performance. Ecology 74, 763–777. doi: 10.2307/1940804
Lindroth, R. L., Wood, S. A., and Kopper, B. J. (2002). Response of quaking aspen genotypes to enriched CO2: Foliar chemistry and tussock moth performance. Agric. For. Entomol. 4, 315–323. doi: 10.1046/j.1461-9563.2002.00156.x
Lindroth, R., Roth, S., and Nordheim, E. (2001). Genotypic variation in response of quaking aspen (Populus tremuloides) to atmospheric CO2 enrichment. Oecologia 126, 371–379. doi: 10.1007/s004420000521
Liu, X., Ma, Q., Yu, H., Li, Y., Li, L., Qi, M., et al. (2021). Climate warming-induced drought constrains vegetation productivity by weakening the temporal stability of the plant community in an arid grassland ecosystem. Agric. For. Meteorol. 307:108526. doi: 10.1016/j.agrformet.2021.108526
Long, S. P. (1991). Modification of the response of photosynthetic productivity to rising temperature by atmospheric CO2 concentrations: Has its importance been underestimated? Plant Cell Environ. 14, 729–739. doi: 10.1111/j.1365-3040.1991.tb01439.x
Lotfiomran, N., Köhl, M., and Fromm, J. (2016). Interaction effect between elevated CO2 and fertilization on biomass, gas exchange and C/N ratio of european beech (Fagus sylvatica L.). Plants (Basel) 5:38. doi: 10.3390/plants5030038
Luo, G., Lai, X., Jiang, H., and Zhang, K. (2006). Size variation of the end permian conodont neogondolella at meishan section, Changxing, Zhejiang and its significance. Sci. China Ser. D 49, 337–347. doi: 10.1007/s11430-008-0125-1
Makkar, H. P. S., Blümmel, M., Borowy, N. K., and Becker, K. (1993). Gravimetric determination of tannins and their correlations with chemical and protein precipitation methods. J. Sci. Food Agric. 61, 161–165. doi: 10.1002/jsfa.2740610205
Marchand, W., Girardin, M., Hartmann, H., Depardieu, C., Isabel, N., Gauthier, S., et al. (2020). Strong overestimation of water-use efficiency responses to rising CO2 in tree-ring studies. Glob. Chang Biol. 26, 4538–4558. doi: 10.1111/gcb.15166
Marchiosi, R., dos Santos, W. D., Constantin, R. P., de Lima, R. B., Soares, A. R., Finger-Teixeira, A., et al. (2020). Biosynthesis and metabolic actions of simple phenolic acids in plants. Phytochem. Rev. 19, 865–906. doi: 10.1007/s11101-020-09689-2
Marks, S., and Lincoln, D. E. (1996). Antiherbivore defense mutualism under elevated carbon dioxide levels: A fungal endophyte and grass. Environ. Entomol. 25, 618–623. doi: 10.1093/ee/25.3.618
Mattson, W. J. (1980). Herbivory in relation to plant nitrogen content. Annu. Rev. Ecol. Syst. 11, 119–161. doi: 10.1146/annurev.es.11.110180.001003
Mayack, C., Carmichael, K., Phalen, N., Khan, Z., Hirche, F., Stangl, G., et al. (2020). Gas chromatography - Mass spectrometry as a preferred method for quantification of insect hemolymph sugars. J. Insect. Physiol. 127:104115. doi: 10.1016/j.jinsphys.2020.104115
Milanović, S., Lazarević, J., Karadžić, D., Milenković, I., Jankovskı, L., Vuleta, A., et al. (2015). Belowground infections of the invasive Phytophthora plurivora pathogen enhance the suitability of red oak leaves to the generalist herbivore Lymantria dispar. Ecol. Entomol. 40, 479–482. doi: 10.1111/een.12193
Montoya, J., and Raffaelli, D. (2010). Climate change, biotic interactions and ecosystem services. Philos. Trans. R. Soc. Lond. B Biol. Sci. 365, 2013–2018. doi: 10.1098/rstb.2010.0114
Moreira, X., Glauser, G., and Abdala-Roberts, L. (2017). Interactive effects of plant neighbourhood and ontogeny on insect herbivory and plant defensive traits. Sci. Rep. 7:4047. doi: 10.1038/s41598-017-04314-3
Münzbergová, Z., and Skuhrovec, J. (2020). Data on herbivore performance and plant herbivore damage identify the same plant traits as the key drivers of plant-herbivore interaction. Insects 11:865. doi: 10.3390/insects11120865
Murray, T., Ellsworth, D., Tissue, D., and Riegler, M. (2013). Interactive direct and plant-mediated effects of elevated atmospheric [CO2] and temperature on a eucalypt-feeding insect herbivore. Glob. Chang. Biol. 19, 1407–1416. doi: 10.1111/gcb.12142
Nakládal, O., and Brinkeová, H. (2015). Review of historical outbreaks of the nun moth (Lymantria monacha) with respect to host tree species. J. For. Sci. 61, 18–26. doi: 10.17221/94/2014-JFS
Navarro, D. J., and Navarro, M. D. (2022). lsr: Companion to “learning statistics with R”. R package version 0.5.2. Available online at: https://cran.r-project.org/package=lsr
Norby, R. J., Wullschleger, S. D., Gunderson, C. A., Johnson, D. W., and Ceulemans, R. (1999). Tree responses to rising CO2 in field experiments: Implications for the future forest. Plant Cell Environ. 22, 683–714. doi: 10.1046/j.1365-3040.1999.00391.x
Porter, L. J., Hrstich, L. N., and Chan, B. G. (1985). The conversion of procyanidins and prodelphinidins to cyanidin and delphinidin. Phytochemistry 25, 223–230. doi: 10.1016/S0031-9422(00)94533-3
Prior, S. A., Pritchard, S. G., and Runion, G. B. (2004). Leaves and the effects of elevated carbon dioxide levels. Dekker Encycl. Plant Crop Sci. 648–650. doi: 10.1081/E-EPCS120010613
Psistaki, K., Tsantopoulos, G., and Paschalidou, A. K. (2024). An overview of the role of forests in climate change mitigation. Sustainability 16:6089. doi: 10.3390/su16146089
Rajashekar, C. B. (2018). Elevated CO2 levels affect phytochemicals and nutritional quality of food crops. Am. J. Plant Sci. 9, 150–162. doi: 10.4236/ajps.2018.92013
Rapp, D. (2024). Estimate of temperature rise in the 21st century for various scenarios. IgMin Res. 2, 564–569. doi: 10.61927/igmin218
Reddy, G., and Guerrero, A. (2004). Interactions of insect pheromones and plant semiochemicals. Trends Plant Sci. 9, 253–261. doi: 10.1016/j.tplants.2004.03.009
Ren, X., Guo, R., Akami, M., and Niu, C. (2022). Nitrogen acquisition strategies mediated by insect symbionts: A review of their mechanisms, methodologies, and case studies. Insects 13:84. doi: 10.3390/insects13010084
Richardson, L., Adler, L., Leonard, A., Andicoechea, J., Regan, K., Anthony, W., et al. (2015). Secondary metabolites in floral nectar reduce parasite infections in bumblebees. Proc. Biol. Sci. 282:20142471. doi: 10.1098/rspb.2014.2471
Robinson, E., Ryan, G., and Newman, J. A. A. (2012). meta-analytical review of the effects of elevated CO2 on plant-arthropod interactions highlights the importance of interacting environmental and biological variables. New Phytol. 194, 321–336. doi: 10.1111/j.1469-8137.2012.04074.x
Rolland, F., Baena-Gonzalez, E., and Sheen, J. (2006). Sugar sensing and signaling in plants: Conserved and novel mechanisms. Annu. Rev. Plant Biol. 57, 675–709. doi: 10.1146/annurev.arplant.57.032905.105441
Roth, S., and Lindroth, R. (1994). Effects of CO2-mediated changes in paper birch and white pine chemistry on gypsy moth performance. Oecologia 98, 133–138. doi: 10.1007/BF00341464
Roy, S., and Mathur, P. (2021). Delineating the mechanisms of elevated CO2 mediated growth, stress tolerance and phytohormonal regulation in plants. Plant Cell Rep. 40, 1345–1365. doi: 10.1007/s00299-021-02738-w
Russell, A., Lenth, V., Buerkner, P., Herve, M., Love, J., Singmann, H., et al. (2021). EMMEANS: Estimated Marginal Means, Aka Least-Squares Means. Available online at: https://doi.org/10.32614/CRAN.package.emmeans (accessed January 15, 2025).
Salam, U., Ullah, S., Tang, Z., Elateeq, A., Khan, Y., Khan, J., et al. (2023). Plant metabolomics: An overview of the role of primary and secondary metabolites against different environmental stress factors. Life (Basel) 13:706. doi: 10.3390/life13030706
Sato, H., Mizoi, J., Shinozaki, K., and Yamaguchi-Shinozaki, K. (2024). Complex plant responses to drought and heat stress under climate change. Plant J. 117, 1873–1892. doi: 10.1111/tpj.16612
Saxe, H., Ellsworth, D. S., and Heath, J. (1998) Tree and forest functioning in an enriched CO2 atmosphere. New Phytol. 139, 395–436. doi: 10.1046/j.1469-8137.1998.00221.x
Schmitt, M. H., Shuttleworth, A., Shrader, A. M., and Ward, D. (2020). The role of volatile plant secondary metabolites as pre-ingestive cues and potential toxins dictating diet selection by African elephants. Oikos 129, 24–34. doi: 10.1111/oik.06665
Scogings, P. F., Hjältén, J., and Skarpe, C. (2013). Does large herbivore removal affect secondary metabolites, nutrients and shoot length in woody species in semi-arid savannas? J. Arid Environ. 88, 4–8.
Shamloo, M., Babawale, E., Furtado, A., Henry, R., Eck, P., and Jones, P. (2017). Effects of genotype and temperature on accumulation of plant secondary metabolites in Canadian and Australian wheat grown under controlled environments. Sci. Rep. 7:9133. doi: 10.1038/s41598-017-09681-5
Sheng, M., Tang, J., Yang, D., Fisher, J., Wang, H., and Kattge, J. (2021). Long-term leaf C:N ratio change under elevated CO2 and nitrogen deposition in China: Evidence from observations and process-based modeling. Sci. Total Environ. 800:149591. doi: 10.1016/j.scitotenv.2021.149591
Sheng, S., Zhang, X., Zheng, Y., Wang, J., Zhou, Y., Liao, C., et al. (2019). Effect of six sugars on the longevity, oviposition performance and nutrition accumulation in an endoparasitoid, Meteorus pulchricornis (Hymenoptera: Braconidae). J. Asia Pac. Entomol. 22, 263–268. doi: 10.1016/j.aspen.2019.01.010
Singh, S., Kaur, I., and Kariyat, R. (2021). The multifunctional roles of polyphenols in plant-herbivore interactions. Int. J. Mol. Sci. 22:1442. doi: 10.3390/ijms22031442
Skowronek, P., Wójcik, Ł, and Strachecka, A. (2021). Fat body-multifunctional insect tissue. Insects. 12:547. doi: 10.3390/insects12060547
Slot, M., Rifai, S., and Winter, K. (2021). Photosynthetic plasticity of a tropical tree species, Tabebuia rosea, in response to elevated temperature and [CO2]. Plant Cell. Environ. 44, 2347–2364. doi: 10.1111/pce.14049
Sodiq, A., Abdullatif, Y., Aissa, B., Ostovar, A., Nassar, N., El-Naas, M., et al. (2023). A review on progress made in direct air capture of CO2. Environ. Technol. Innov. 29:102991. doi: 10.1016/j.eti.2022.102991
Solomon, S. (2007). Carbon dioxide storage: Geological security and environmental issues – Case study on the Sleipner gas field in Norway. Bellona Rep. 128, 1–107.
Stamp, N. E., and Yang, Y. (1996) Response of insect herbivores to multiple allelochemicals under different thermal regimes. Ecology 77, 1088–1102. doi: 10.2307/2265990
Stiling, P., and Cornelissen, T. (2007). How does elevated carbon dioxide (CO2) affect plant–herbivore interactions? A field experiment and meta-analysis of CO2-mediated changes on plant chemistry and herbivore performance. Glob. Change Biol. 13, 1823–1842. doi: 10.1111/j.1365-2486.2007.01392.x
Stowe, L. G., and Osborn, A. (1980). The influence of nitrogen and phosphorus levels on the phytotoxicity of phenolic compounds. Can. J. Bot. 58, 1149–1153. doi: 10.1016/0038-0717(91)90033-G
Strain, B. R., and Cure, J. D. (1985). Direct effects of Increasing Carbon Dioxide on Vegetation. No. DOE/ER-0238. Durham, NC: Duke University.
Šulc, M., Tomášková, I., Krejzková, A., Samek, M., Diuzheva, A., Hradecký, J., et al. (2021). Trehalose determination in Norway spruce (Picea abies) roots. Analytics matters. MethodsX 8:101280. doi: 10.1016/j.mex.2021.101280
Teawkul, P., and Hwang, S. Y. (2019). Carbon dioxide- and temperature-mediated changes in plant defensive compounds alter food utilization of herbivores. J. Appl. Entomol. 143, 289–298. doi: 10.1111/jen.12593
Tylianakis, J., Didham, R., Bascompte, J., and Wardle, D. (2008). Global change and species interactions in terrestrial ecosystems. Ecol. Lett. 11, 1351–1363. doi: 10.1111/j.1461-0248.2008.01250.x
Uddin, R., Shaikh, A. J., Khan, H. R., Shirazi, M. A., Rashid, A., and Qazi, S. A. (2021). Renewable energy perspectives of Pakistan and Turkey: Current analysis and policy recommendations. Sustainability 13:3349. doi: 10.3390/su13063349
Vencl, F. V., Bartram, S., Winter, K., Boland, W., and Srygley, R. B. (2024). Effects of elevated CO2 and temperature on the performance of a diet specialized neotropical herbivore and its host plant. Biotropica 56:e13371. doi: 10.1111/btp.13371
Veteli, T., Mattson, W., Niemelä, P., Julkunen-Tiitto, R., Kellomäki, S., Kuokkanen, K., et al. (2007). Do elevated temperature and CO2 generally have counteracting effects on phenolic phytochemistry of boreal trees? J. Chem. Ecol. 33, 287–296. doi: 10.1007/s10886-006-9235-4
Waldbauer, G. P. (1968). “The consumption and utilization of food by insects,” in Advances in Insect Physiology, Vol. 5, (Cambridge, MA: Academic Press), 229–288.
Watanabe, Y., Hinata, K., Qu, L., Kitaoka, S., Watanabe, M., Kitao, M., et al. (2021). Effects of elevated CO2 and nitrogen loading on the defensive traits of three successional deciduous broad-leaved tree seedlings. Forests 12:939. doi: 10.3390/f12070939
Way, D., and Oren, R. (2010). Differential responses to changes in growth temperature between trees from different functional groups and biomes: A review and synthesis of data. Tree Physiol. 30, 669–688. doi: 10.1093/treephys/tpq015
White, J. W., and Warrington, I. J. (1984). Growth and development responses of geranium to temperature, light integral, CO2, and chlormequat. J. Am. Soc. Hortic. Sci. 109, 728–735. doi: 10.21273/JASHS.109.5.728
Williams, R. S., Lincoln, D. E., and Norby, R. J. (1998). Leaf age effects of elevated CO2-grown white oak leaves on spring-feeding lepidopterans. Glob. Change Biol. 4, 235–246. doi: 10.1046/j.1365-2486.1998.00127.x
Wink, M. (2018). Plant secondary metabolites modulate insect behavior – steps toward addiction? Front. Physiol. 9:364.
Xiaowei, W., Ji, L., and Liu, Y. (2006). Effects of increased atmospheric CO2 on nutritional contents in poplar (Populus pseudo-simonii [Kitag.]) tissues and larval growth of gypsy moth (Lymantria dispar). Acta Ecol. Sinica 26, 3166–3173. doi: 10.1016/S1872-2032(06)60047-4
Xie, M., Li, Q., Zha, L., Zhu, M., Cheng, Q., Yuan, J., et al. (2015). Effects of low nitrogen stress on the physiological and morphological traits of roots of different low nitrogen tolerance maize varieties at seedling stage. Chin. J. Eco Agric. 23, 946–953. doi: 10.13930/j.cnki.cjea.150194
Xi-Liu, Y. U. E., and Gao, Q. X. (2018). Contributions of natural systems and human activity to greenhouse gas emissions. Adv. Climate Change Res. 9, 243–252. doi: 10.1016/j.accre.2018.12.003
Yamori, W., Hikosaka, K., and Way, D. (2014). Temperature response of photosynthesis in C3, C4, and CAM plants: Temperature acclimation and temperature adaptation. Photosynth. Res. 119, 101–117. doi: 10.1007/s11120-013-9874-6
Yin, J., Sun, Y., Wu, G., and Ge, F. (2010). Effects of elevated CO2 associated with maize on multiple generations of the cotton bollworm, Helicoverpa armigera. Entomol. Exp. Appl. 136, 12–20. doi: 10.1111/j.1570-7458.2010.00998.x
Yu, K., Curcic, I., Gabriel, J., and Tsang, S. (2008). Recent advances in CO2 capture and utilization. ChemSusChem 1, 893–899. doi: 10.1002/cssc.200800169
Yuan, D., Zhu, L., Cherubini, P., Li, Z., Zhang, Y., and Wang, X. (2021). Species-specific indication of 13 tree species growth on climate warming in temperate forest community of Northeast China. Ecol. Indicators 133:108389. doi: 10.1016/j.ecolind.2021.108389
Zengin, G., Nithiyanantham, S., Locatelli, M., Ceylan, R., Uysal, S., Aktumsek, A., et al. (2016). Screening of in vitro antioxidant and enzyme inhibitory activities of different extracts from two uninvestigated wild plants: Centranthus longiflorus subsp. longiflorus and Cerinthe minor subsp. auriculata. Eur. J. Integr. Med. 8, 286–292. doi: 10.1016/j.eujim.2015.12.004
Zhang, J. Z., Wang, W., Sun, G. F., Liu, H. Z., and Li, X. D. (2011). Photosynthesis of Hosta under light and controlled-release nitrogen fertilizer. Russian J. Plant Physiol. 58, 261–270. doi: 10.1134/S1021443711020269
Zhang, J., Xing, G. M., Liao, J. X., Hou, Z. D., Wang, G. X., and Wang, Y. F. (2003). Effects of different atmospheric CO2 concentrations and soil moistures on the populations of bird cherry-oat aphid (Rhopalosiphum padi) feeding on spring wheat. Eur. J. Entomol. 100, 521–530. doi: 10.14411/eje.2003.080
Zhang, S., and Dang, Q. L. (2007). Interactive effects of soil temperature and [CO2] on morphological and biomass traits in seedlings of four boreal tree species. For. Sci. 53, 453–460. doi: 10.1093/forestscience/53.3.453
Zhang, Y. F., Wan, G. J., Liu, B., Zhang, X. G., Xing, G. N., and Chen, F. J. (2018). Elevated CO2 and temperature alter development and food utilization of Spodoptera litura fed on resistant soybean. J. Appl. Entomol. 142, 250–262. doi: 10.1111/jen.12463
Zhang, Y., Moser, B., Li, M., Wohlgemuth, T., Lei, J., and Bachofen, C. (2020). Contrasting leaf trait responses of conifer and broadleaved seedlings to altered resource availability are linked to resource strategies. Plants (Basel) 9:621. doi: 10.3390/plants9050621
Zheng, Y., Li, F., Hao, L., Yu, J., Guo, L., Zhou, H., et al. (2019). Elevated CO2 concentration induces photosynthetic down-regulation with changes in leaf structure, non-structural carbohydrates and nitrogen content of soybean. BMC Plant Biol. 19:255. doi: 10.1186/s12870-019-1788-9
Zhou, S., Lou, Y. R., Tzin, V., and Jander, G. (2015). Alteration of plant primary metabolism in response to insect herbivory. Plant Physiol. 169, 1488–1498.
Ziska, L. (2002). Influence of rising atmospheric CO2 since 1900 on early growth and photosynthetic response of a noxious invasive weed, Canada thistle (Cirsium arvense). Funct. Plant Biol. 29, 1387–1392. doi: 10.1071/FP02052
Keywords: climate change, herbivory, plant chemistry, plant-insect interactions, secondary metabolites
Citation: Dvořáková B, Holuša J, Musiolek D, Kalyniukova A, Hradecký J, Čepl J and Schopf A (2025) Host tree impact on Lymantria species under CO2 and temperature changes. Front. For. Glob. Change 8:1564011. doi: 10.3389/ffgc.2025.1564011
Received: 05 February 2025; Accepted: 24 April 2025;
Published: 20 May 2025.
Edited by:
Jürgen Kreuzwieser, University of Freiburg, GermanyReviewed by:
Manuela Branco, University of Lisbon, PortugalHilke Schroeder, Thünen Institute of Forest Genetics, Germany
Copyright © 2025 Dvořáková, Holuša, Musiolek, Kalyniukova, Hradecký, Čepl and Schopf. This is an open-access article distributed under the terms of the Creative Commons Attribution License (CC BY). The use, distribution or reproduction in other forums is permitted, provided the original author(s) and the copyright owner(s) are credited and that the original publication in this journal is cited, in accordance with accepted academic practice. No use, distribution or reproduction is permitted which does not comply with these terms.
*Correspondence: Barbora Dvořáková, RHZvcmFrb3ZhMUBmbGQuY3p1LmN6
†These authors have contributed equally to this work and share senior authorship