- 1Department of Ecology and Forest Management, Forest Research Institute, University of Sopron, Sopron, Hungary
- 2Department of Botany, Faculty of Science and Technology, University of Debrecen, Debrecen, Hungary
- 3Institute of Forest Ecology, University of Natural Resources and Life Sciences BOKU, Vienna, Austria
- 4Space Research Group, Department of Geophysics and Space Sciences, Institute of Geography and Earth Sciences, ELTE Eötvös Loránd University, Budapest, Hungary
- 5Faculty of Forestry and Wood Sciences, Czech University of Life Sciences, Prague, Czechia
- 6Department of Forest Protection, Forest Research Institute, University of Sopron, Sopron, Hungary
- 7Unaffiliated Researcher, Sopron, Hungary
- 8Department of Tree Breeding, Forest Research Institute, University of Sopron, Sopron, Hungary
- 9Department of Crop Production, Centre for Agricultural Research, Agricultural Institute, Martonvásár, Hungary
The growth and vitality of forest ecosystems in Central Europe are expected to be affected by climate change, posing a challenge for forest management. In this study, we examined and projected the relationship between growth and climate for dominant sessile oak (Quercus petraea (Matt.) Liebl.) and Turkey oak (Quercus cerris L.) trees in two sites in Hungary, each with different water availability. We developed a single linear mixed model based on tree age, climatic water balance and insect outbreak for the study period (1971–2021) to estimate the impact of projected climate change in the 21st century on basal area increments until 2100 using two climate scenarios (RCP4.5 and RCP8.5). Our study suggests that the growth response of oak species to different seasonal water balances is site and species-specific. We found that the water balance of the previous winter had a significantly greater influence on the growth in the stands of the dry site than in the stands of the wet site. Q. cerris reacted more sensitively to the water balance conditions of the previous summer than Q. petraea. Predictive models forecast a general growth decline of up to 10.8% for both oak species during the 21st century compared to past growth with a significant change according to the RCP8.5 scenario. Additionally, the models predict a significantly greater decrease in growth in the more humid site compared to the xeric site which could be attributed to the site-specific response of growth to the winter water balance. At the same time, we did not detect significant differences in the future growth of the two oak species. These findings provide insights into the climate-related factors influencing growth in Hungarian oak forests.
1 Introduction
Forests in Hungary face a significant threat due to climate change. Climate models predict a 20% decrease in summer precipitation and a 30% increase in winter precipitation during this century (Kis et al., 2020), along with a rise in the frequency of long-lasting droughts and heat waves (Pongrácz et al., 2014; Perkins-Kirkpatrick and Lewis, 2020). This will have an unfavorable effect on the water balance during the growing season, depending on site conditions and the characteristics of the forest stand.
To adapt to this changing climate, Hungary’s forestry policy currently supports the plantation and maintenance of forest-forming tree species that are more resistant to drought. Numerous dendroecological studies have shown that oaks generally have a high climate tolerance and could play a significant role in the forests of Central Europe in the future (Nardini et al., 1999; Härdtle et al., 2013; Zimmermann et al., 2015; Ciceu et al., 2020; Kasper et al., 2022). However, oaks can become vulnerable to climate change in some conditions, such as spring drought in historically mesic sites (Bose et al., 2021). In addition, the higher drought tolerance of oak is usually linked to lower productivity, which can result in lower growth rates and smaller heights of the oak species under unfavorable water availability conditions (Kasper et al., 2022). Pests may also become more problematic due to the indirect effects of climate change, which could further impede growth (Csóka et al., 2018; Kern et al., 2021). In Hungary, sessile oak (Quercus petraea (Matt.) Liebl.) and Turkey oak (Quercus cerris L.) are the two most significant tree species found in over 20% of the total forest area (National Forestry Database, 2021).1
Species distribution models typically predict the expansion of Q. cerris and the declining distribution of Q. petraea in Hungary (Czúcz et al., 2011; Führer et al., 2011; Móricz et al., 2013; Thurm et al., 2018; Illés and Móricz, 2022). The bioclimatic niche-based distribution models estimate the future areas suitable for various tree species by analyzing the climatic niche of their current distribution range alongside future climate scenarios. However, these models usually do not consider how climatic conditions might affect the growth of these species within their potential distribution areas. Furthermore, forest stands at the margins of their distribution may be particularly sensitive to climatic changes (Dorado-Liñán et al., 2019; Camarero et al., 2021). To predict how these species will respond to future climate change, it is crucial to understand their local growth responses to past climatic changes (Mina et al., 2016).
Recent studies examining the relationship between climate and radial growth in oak species show that the growth of both Q. cerris and Q. petraea is mainly dependent on summer water availability without sustained water availability from groundwater. These included dendrochronological studies on the drought tolerance and growth-climate relationship in Central Europe (Cufar et al., 2014; Dobrovolný et al., 2016; Kolář et al., 2017; Árvai et al., 2018; Kunz et al., 2018; Ciceu et al., 2020; Móricz et al., 2021; Kasper et al., 2022). However, few studies have found that winter water balance is also highly important for the growth of oak species which may be related to physiological activities for the earlywood formation in spring (Bose et al., 2021; Mészáros et al., 2022). Recent research has shown that the growth response to drought differs between Q. cerris and Q. petraea (Ciceu et al., 2020; Móricz et al., 2021; Mészáros et al., 2022). The growth response of Q. petraea is less sensitive to water scarcity caused by drought than the reaction of Q. cerris, indicating differences in their physiological responses to water availability (Ciceu et al., 2020; Móricz et al., 2021; Mészáros et al., 2022).
A limited number of studies have been conducted on the future growth of oak trees in Central and Western Europe (Friedrichs et al., 2009; Bauwe et al., 2015; Stojanović et al., 2017; Vallet and Perot, 2018). These studies were conducted in the cooler and moister part of Central Europe, so no representative growth projection is available for the two main oak tree species (sessile oak and Turkey oak) in the more drought-endangered regions of Europe, such as Hungary. This study aims to examine the influence of climatic water balance on the radial growth of dominant Q. cerris and Q. petraea trees in Southwestern Hungary and answer two main research questions: (1) Does the growth-climate relationship of the examined species differ between sites with contrasting water availability? (2) How will the radial growth of the oak species be affected by the anticipated change in climate and water availability in the 21st century?
2 Materials and methods
2.1 Study sites and forest stands
We selected two study sites in south-western Hungary that differ considerably in terms of water availability, close to the municipalities Iharos (WET, 46.32 N/17.06E) and Ságvár (DRY, 46.82 N/18.06E), situated at an elevation range of 220 and 265 m a.s.l., respectively (Figure 1). At each site, we have selected two seedling forest stands close to each other (<1,000 m) with ages between 90 and 109 years old (Table 1). One of the stands is dominated by sessile oak (Quercus petraea; hereafter QP), while the other is prevailed by Turkey oak (Quercus cerris; hereafter QC). In the selected stands, other tree species were also present, such as European hornbeam (Carpinus betulus L.), silver lime (Tilia tomentosa Moench.), and pedunculate oak (Quercus robur L.) (Supplementary Table S1).
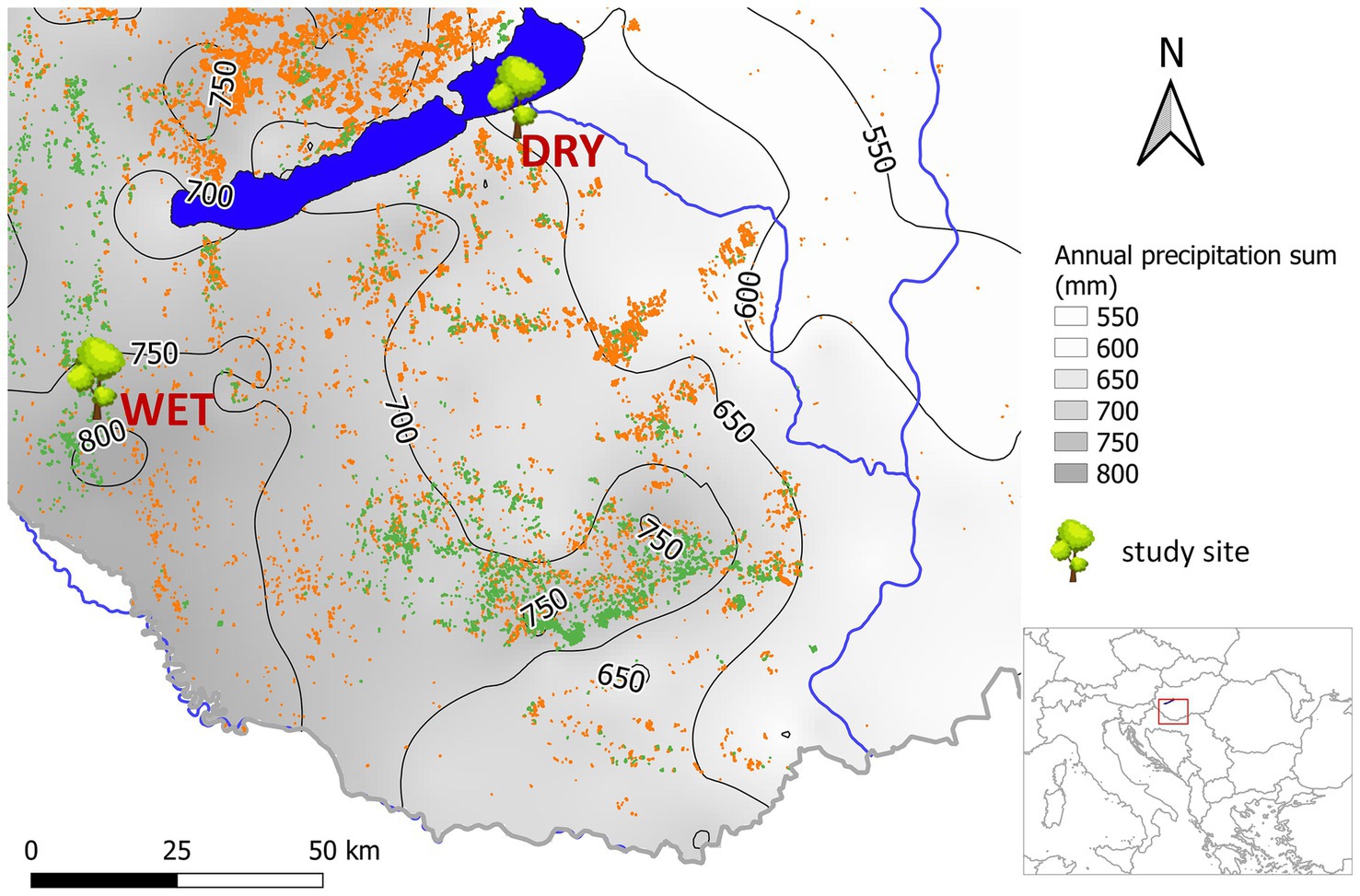
Figure 1. Location of the two study sites (WET, DRY). The shaded color map and contour lines represent the mean annual precipitation (mm) for the period 1971–2021; the colored polygons mark forest sub-compartments where sessile oak—Quercus petraea (green) and Turkey oak—Quercus cerris (orange) are the dominant tree species in the forest canopy (Source: National Forestry Database, 2021, https://erdoleltar.nfk.gov.hu).
To ensure comparability across the study areas, we selected the different tree populations with similar topography and soil conditions as much as possible (Table 1). The genetic soil type was Clayic Luvisol (lessivated brown forest soil) at the WET site with a depth of 90 cm, while the Cambiosol (brown earth) at the DRY site had a depth of 75 cm. However, both soils had the same loamy texture without rock fraction content and similar water capacity, resulting in the lack of surplus water provided.
We calculated the available water capacity of soils based on the particle composition and baserock fraction content taken from four depths according to soil horizons in each site using a soil sampling corer. Field capacity and wilting point water content of the soil samples were estimated using pedotransfer functions and the Rosetta3 model in the soilDB package of the R software (Zhang and Schaap, 2017). We then summed up the water content differences between field capacity and wilting point over the entire rooting depth, which was more than 1 m at all stands, as estimated by visual observation of fine roots in the soil samples.
To minimize the anthropogenic (forest) management impact on growth-climate relationships, we selected forest stands with the lowest management intensity in a wider area of the study sites during the last decades (personal communication with forest managers Tamás Papp and Péter Farkas).
In each stand, we selected large (~1 ha) distinct unmixed patches of the analyzed species to avoid competition and effects of mixing species. We sampled individuals that are currently dominant, as they express the growth potential of a site that the climatic conditions allow and are generally less sensitive to thinning. However, there is some uncertainty because a tree’s current social status does not necessarily reflect its past condition. An even more comprehensive sampling of the entire forests stands was also limited by cost-efficiency considerations.
Tree height and diameter at breast height were measured for each selected tree of all stands using a TruPulse 200 laser instrument (Laser Technology, Inc., Colorado 80112, United States) and Haglöf Mantax Blue forestry caliper (Haglöf Sweden AB, Långsele, Sweden), respectively and subsequently averaged.
The study was carried out at two locations situated on a (sub)regional hydroclimatic gradient. The WET site received approximately 20% more rainfall than the DRY site, resulting in an excess of 150 mm of mean annual precipitation during the period 1971–2021 (Figure 2; Supplementary Figure S1). During the same period, both locations had almost the same mean annual temperature, which was derived from the database of the Hungarian Meteorological Service.2
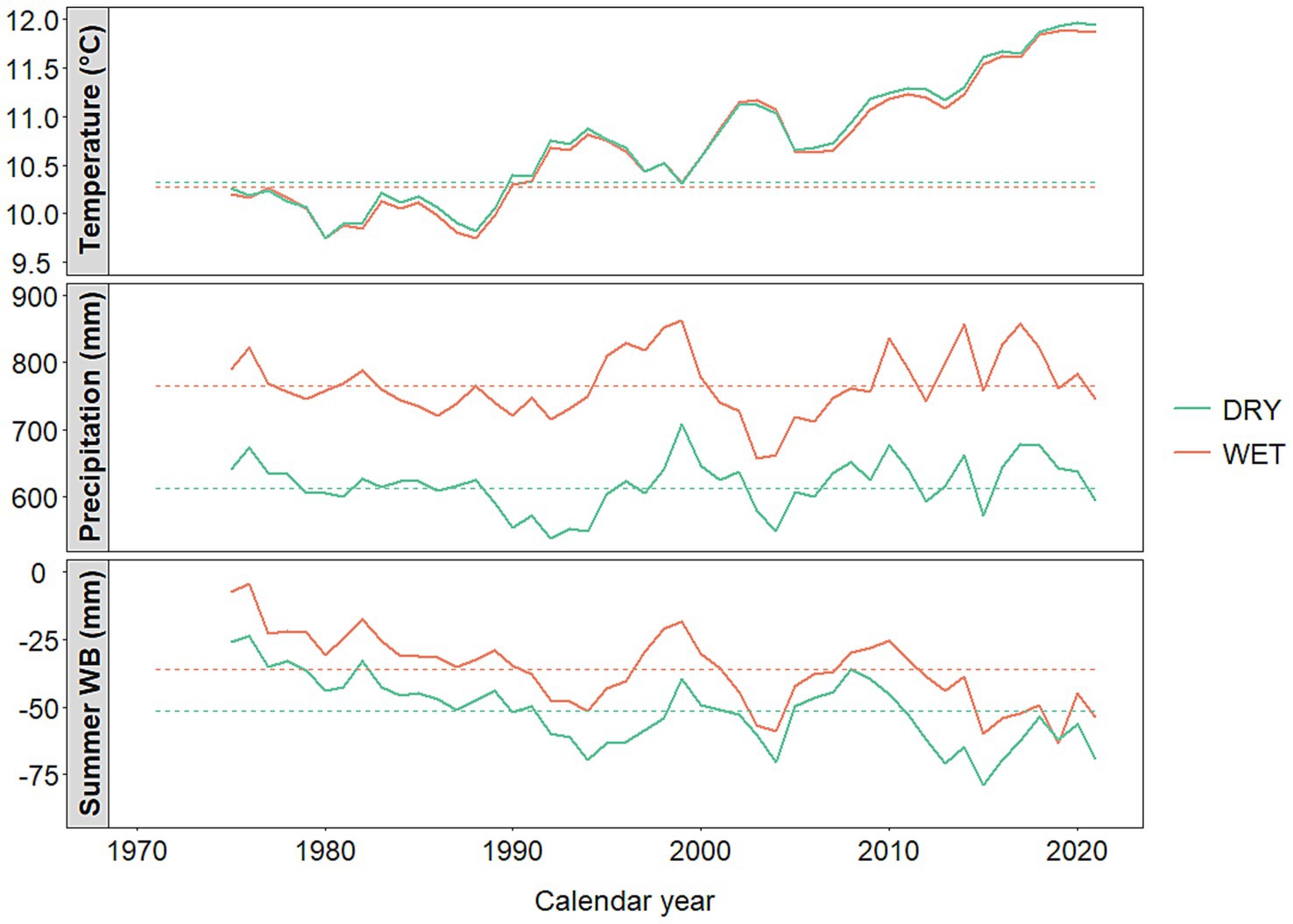
Figure 2. Trends of annual precipitation sum and annual mean temperature at the study sites during the period 1971–2021. The dashed horizontal lines represent the average values of the reference period 1971–2000; the curves are smoothed using a 5-year moving average for better visualization of climatic trends (HMS, 2022).
2.2 Tree-ring data
At each stand, 40 dominant specimens (a total of 160 trees) were selected and one increment core was taken from each sample tree at breast height (1.3 m) using a Pressler increment borer (Haglöf, Långsele, Sweden). Tree cores were extracted in the direction that would be least affected by tension wood. The samples were air-dried and then glued to grooved wooden mounting board, sanded following dendrochronological protocol, and scanned at 1,200 dpi resolution. We measured tree-ring widths (TRW) on the digital images to 0.01 mm using the WinDENDRO software ver. 2014a (Regent Instruments Inc., Canada). We visually checked the samples to detect characteristic rings, and cross-dated the measured TRW series for each population using the software COFECHA (Holmes, 1983). Series flagged with potential problems via the COFECHA cross-dating process were interpreted as indications of trees with potential individual growth bias compared to the population mean and omitted from further analysis (4–7 cores per stand). Tree age was estimated by adding the measured number of tree rings to the estimated number of missing rings to the pith. The number of missing rings was approximated using the diameter and core length of each tree with the radius-length method (Norton et al., 1987). Raw ring-width series (Supplementary Figure S2) were detrended by smoothing splines with a 50% frequency cut-off at 25 years to calculate common dendrochronological statistics like the inter-series correlation (Rbar) and the expressed population signal (EPS) of the inter-series and inter-annual signal in the measurements. The chronologies were pre-whitened using autoregressive models to eliminate temporal autocorrelation, resulting in residual chronologies for each stand (Table 2). However, opting for using the basal area increments (BAI) for our analysis (Biondi and Qeadan, 2008), the detrended series were exclusively used to derive these statistics. The mean inter-series correlation (Rbar) and the expressed population signal (EPS) of the detrended chronologies indicated a high growth coherency among trees in all stands, confirming the adequacy of the number of sampled trees in our analysis. The BAI was calculated for the study period of 1971–2021 from the tree-ring widths as the difference between consecutive basal areas using the Equation (1):
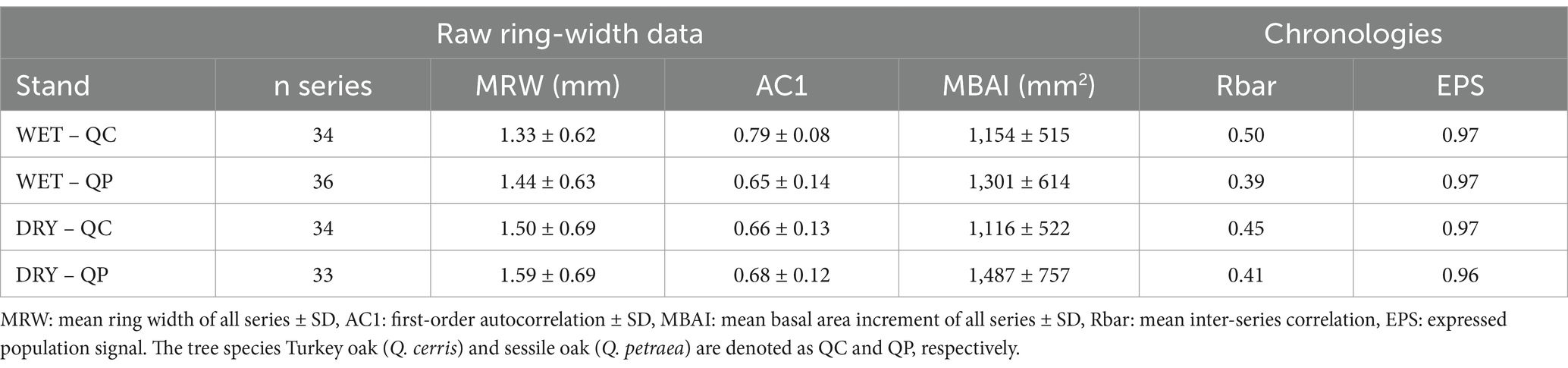
Table 2. Dendrochronological statistics for the ring-width series (raw data) and index-based chronologies (all truncated to the period 1971–2021) of the four stands.
where r is the tree radius and t is the year of the ring formation. We did not find any significant growth release caused by substantial thinning that took place during the study period in either of the studied stands but a moderate event in the early 1990s at the stands of the DRY site that was linked to a sanitary cut following a severe drought event (Nowacki and Abrams, 1997; Supplementary Figure S3). Given the large-scale pest outbreaks in the region (Hirka, 2006), we assessed any biotic effect on radial growth (Varga, 1964; Muzika and Liebhold, 1999; McManus and Csóka, 2007) by using Cook’s distance to the linear regression between the BAI and the annual mean values of the WB of each stand (Cook and Weisberg, 1982). We checked for any outlier years in the datasets that coincided with biotic damage observations reported by local forest managers covering a larger forest landscape (Hirka, 2006). Our analysis identified that the year 2005 was significantly affected by the outbreak of the spongy moth (Lymantria dispar L.) in the stands of the DRY site. This had a remarkable impact on radial growth, affecting 92–93% of the selected trees in both species in the stands of the DRY site. The effect of this outbreak was so great that it masked the effect of the climate on growth during this year. Therefore, we included the 2005 outbreak event in our modeling approach for the stands at the DRY site.
2.3 Meteorological data
Meteorological data were obtained from the HUCLIM daily gridded climate dataset of the Hungarian Meteorological Service (HMS, 2022) with 0.1° spatial resolution (appr. 10 km) for the period 1971–2021 by assigning the closest grid points to the study sites. Daily mean temperature (averaged from daily maximum and minimum temperatures) and precipitation sum were aggregated into monthly mean temperatures and precipitation sums.
The monthly water balance (WB) was calculated as the difference between precipitation and potential evapotranspiration, following McCabe and Markstrom (2007). The WB is an ecologically sensible proxy of water availability for tree growth and is strongly correlated with the radial growth of various tree species (Stojanović et al., 2017; Vitasse et al., 2019). WB captures the negative impact of increased temperatures on water availability and unlike the standardized precipitation-evaporation index based on it (Vicente-Serrano et al., 2010), it is more suitable for comparing sites with different water availability (Vitasse et al., 2019). To reduce the number of variables used for modeling, seasonal WB for the previous summer (JJAp), autumn (SONp) and winter (DJFp), as well as for the spring (MAM) and summer (JJA) of the current year of ring formation were considered.
Climate change projections for the period 2022–2100 were downloaded from the FORESEE-HUN v1.0 database with a resolution of 0.1° (Kern et al., 2024). This database contains bias-corrected daily minimum and maximum temperatures and precipitation sums for 14 EURO-CORDEX regional climate models under RCP4.5 and RCP8.5 scenarios (for the full list of the models see Kern et al., 2024). The daily data was aggregated into monthly mean temperature and precipitation sums for each climate model and seasonal WB values were calculated until the end of the 21st century. The ensemble mean ± SE seasonal WB values of the 14 climate models were also derived.
2.4 Statistical analysis
We utilized the linear mixed-effects modeling approach to develop a single growth-climate model covering the period from 1971 to 2021 for all stands, allowing growth predictions over the 21st-century scenarios from 2026 to 2100. As the BAI followed a skewed gamma distribution in all populations, we logarithmically transformed them (BAIlog) (Zell, 2018). During the model selection process, we employed a top-down approach. First, we set up a base model with all potential predictor variables that could contribute to the final optimal model. The base model included the effect of five seasonal WB values (JJAp, SONp, DJFp, MAM, JJA), the tree age (AGE), the insect outbreak (INS), the location (SITE), the tree species (SP) and their relevant interactions. We used interaction plots to show how the relationship between each seasonal WB variable (as well as the AGE) and the continuous response (BAIlog) depended on the value of the location (SITE) or the tree species (SP) using the ggeffects and sjPlot packages in R software (Lüdecke, 2018; Lüdecke, 2024). We visually selected the nonparallel relationships and added each interaction one at a time to the base model. We then compared the models through a chi-squared test and retained only those that significantly improved the base model. This process gave us five relevant interactions (SITE:DJFp, SP:JJA, SP:AGE, SP:JJAp and SP:INS). In the next step, we applied the dredge function of the Mumln package in R software with the maximum likelihood method to select the final model by comparing the Akaike Information Criteria for small samples (AICc). The final model was selected as the one with the lowest AICc (Burnham and Anderson, 2002). Tree identity was also included in the model as a random factor, as the basal area index represented repeated measures over the same individuals. To ensure error independence, we used the auto-correlation function to extract the normalized residuals. The function revealed a first-order autocorrelation of 0.45. To account for this, we included an auto-correlation structure in the final model, using years as the time covariate and treeid as the grouping factor using Equation (2):
After transforming the BAIlog values to BAI, we corrected them using the smearing estimate method to compensate for the underestimation of BAI caused by its changing distribution (Duan, 1983).
The final growth-climate model was used to calculate the annual basal area index (BAI) of each forest stand during the calibration period (1972–2021). The model-simulated annual BAI values were then compared to the observed BAI. As the observed annual BAI values were not normally distributed, we used the median value for comparison. We did not include the year 1971 in the calculations because the previous year’s climate data was not available. We then made growth forecasts for two future periods: 2026–2075 and 2051–2100, which correspond to the projected future growth of oak populations with the same initial ages as those used in the calibration period. To predict the future growth of each stand, we used 14 regional climate model projections with two climate scenarios (RCP4.5 and RCP8.5) (Kern et al., 2024). During the model simulation, we included a hypothetical biotic event in the stands of the DRY site for one random year in both modeling periods. This was done to ensure that the future conditions were similar to those during the calibration period (specifically, the 2005 outbreak event was included at the DRY site).
We compared the annual median values of the observed BAI from 1972 to 2021 (a span of 50 years) with the modeled ensemble BAI of 14 models (consisting of 50 years of annual median values) for each tree species, site, climate scenario, and modeling period using a one-way ANOVA. We checked for homoscedasticity and normality assumptions with Bartlett and Shapiro–Wilk tests, and then applied a post-hoc Tukey test for multiple comparisons. We used two approaches for comparison: (1) the absolute BAI values when examining a specific tree species at a particular site, and (2) the BAI values standardized for the observation period, to compare BAI changes between sites and tree species. The absolute and relative differences among the sum of the annual medians of BAI for the observation (1972–2021) and the model simulation periods (2026–2075 and 2051–2100) were calculated. We examined whether there were any similar models (with delta AICc < 4) to our final model. We then checked if the basal area indexes of these similar models differed from the final model using a one-way ANOVA with 16 replicates corresponding to the tree species, sites, climate scenarios, and modeling periods. For assessing forecast accuracy during the calibration period, we used the root mean square percentage error (RMSPE) as recommended by Swanson et al. (2011). The model fit was evaluated for various assumptions including linearity, normality of residuals, homogeneity of variance and multicollinearity. To determine whether the models forecasted beyond the range of the observational climate data, we compared the seasonal WB values between the observation and model simulation periods using one-way ANOVA and post-hoc Tukey test for multiple comparisons. The LME model was built using the R software (R Core Team, 2022) package lme4 (Bates et al., 2015), and the significance of the model predictors was assessed using the lmerTest package (Kuznetsova et al., 2017). In all cases, we used the 95% confidence interval as a threshold for significance.
3 Results
3.1 Tree growth models
Model diagnostics for the model calibration period showed that the model assumptions were met (Supplementary Figure S4). We found a linear relationship between the outcome variable annual log (BAI) and the seasonal WB values (Supplementary Figure S4A). The residuals of the fitted model showed normal distribution, equal variance (homoscedasticity) and no multicollinearity (Supplementary Figures S4B,C; Supplementary Table S2).
The final model included all seasonal WB variables, tree age, the insect outbreak, tree species, location and three interactions (SITE:DJFp, SP:AGE, SP:JJAp). All model variables had a significant effect on predicting the basal area index (Figures 3, 4; Supplementary Table S3). We observed significant differences in the effect of seasonal WB between sites and tree species (Figure 3). Specifically, we found that the previous winter had a significantly greater influence on the growth in the stands of the DRY site than in the stands of the WET site (Supplementary Table S3). When examining the difference between the tree species, we found that Q. cerris was significantly more sensitive to WB conditions of the previous summer than Q. petraea (Figure 3; Supplementary Table S3).
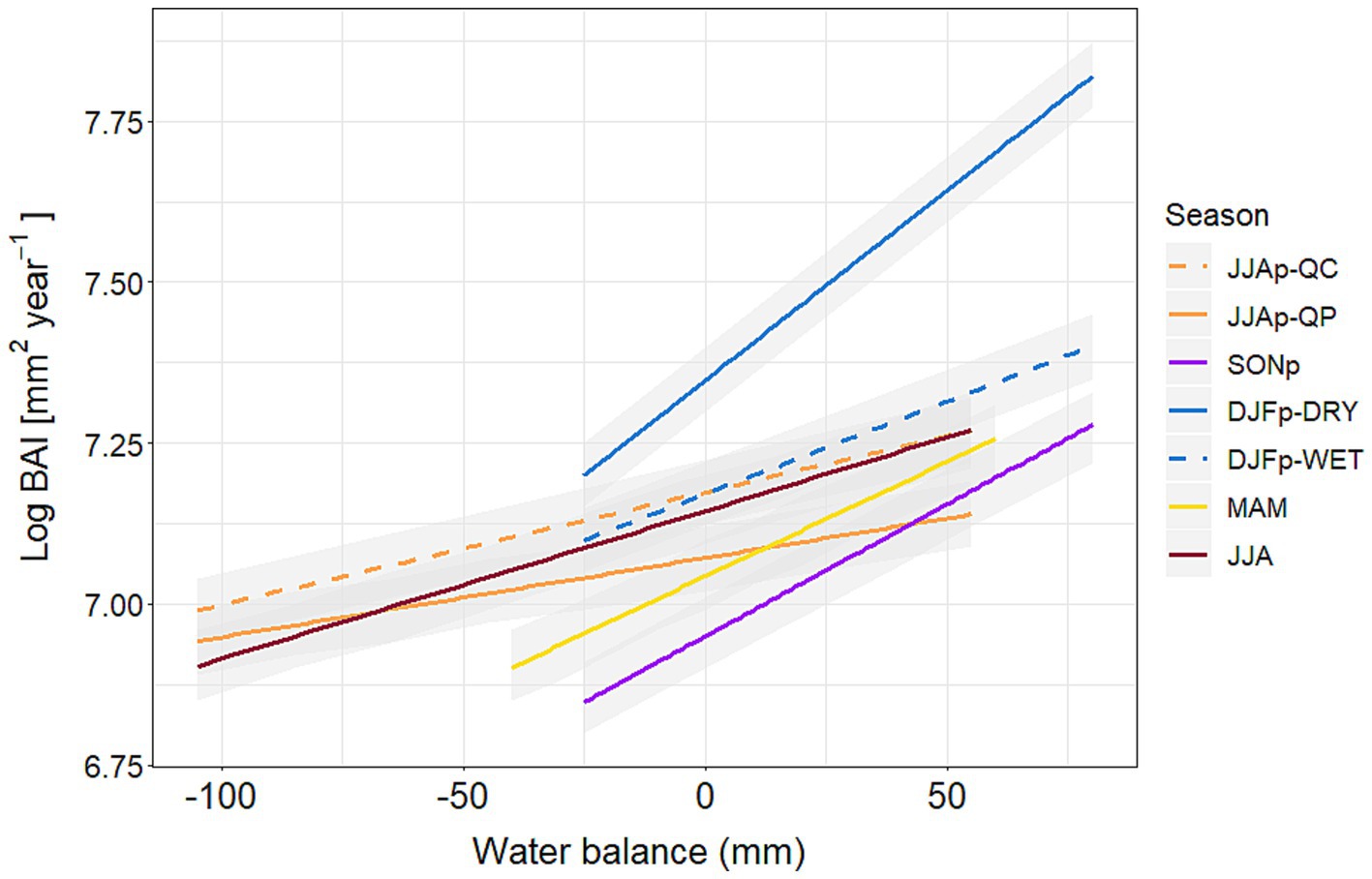
Figure 3. Marginal effects of the regression models (with 95% confidence intervals) among the seasonal water balance values (DJFp and JJAp in interaction with site and species, respectively) and the basal area increment (Log BAI [mm2year−1]) derived from the final mixed-effects model for the study period (1972–2021). JJAp = previous summer, SONp = previous autumn, DJFp = previous winter, MAM = current spring, JJA = current summer, QC = Turkey oak (Quercus cerris), QP = sessile oak (Quercus petraea).
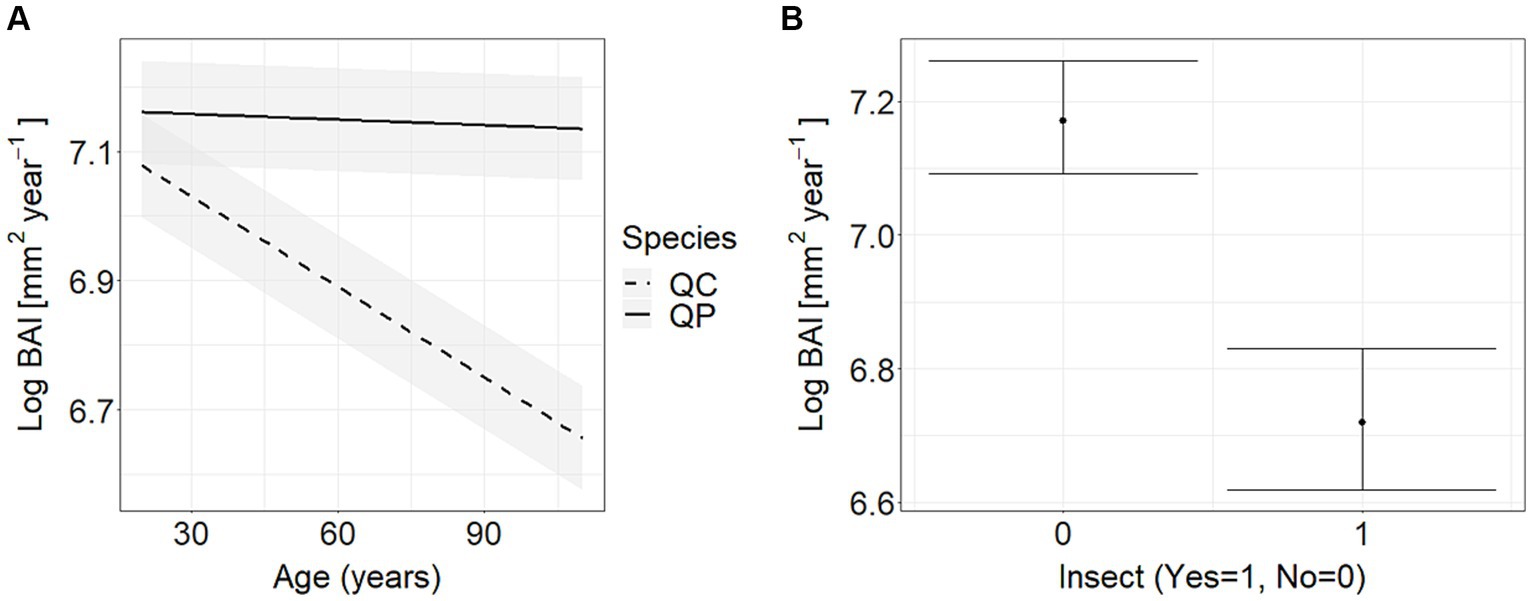
Figure 4. Marginal effects of the regression models (with 95% confidence intervals) between the basal area increment (Log BAI [mm2year−1]) and (A) the tree age (years) as well as (B) the biotic event (insect outbreak). QC = Turkey oak (Quercus cerris), QP = sessile oak (Quercus petraea).
It has been found that the effect of age on the BAI differed significantly for the two different tree species (Figure 4A; Supplementary Table S3). Additionally, both species were significantly affected by an insect outbreak at the DRY site in 2005 (Figure 4B; Supplementary Table S3). The model accurately predicted BAI values throughout the calibration period (1972–2021) (Figure 5). The model also successfully reproduced extreme values. The root mean square percentage errors, which describe the growth prediction performance, ranged from 13.5 to 16.2%. We identified three models that were similar to the final model (delta AICc < 4) (Supplementary Table S4). These models included interactions between species and insect outbreak (SP:INS) and the species and summer WB (SP:JJA). Comparing the simulated BAI of these models with the final model showed no significant differences at any of the examined cases.
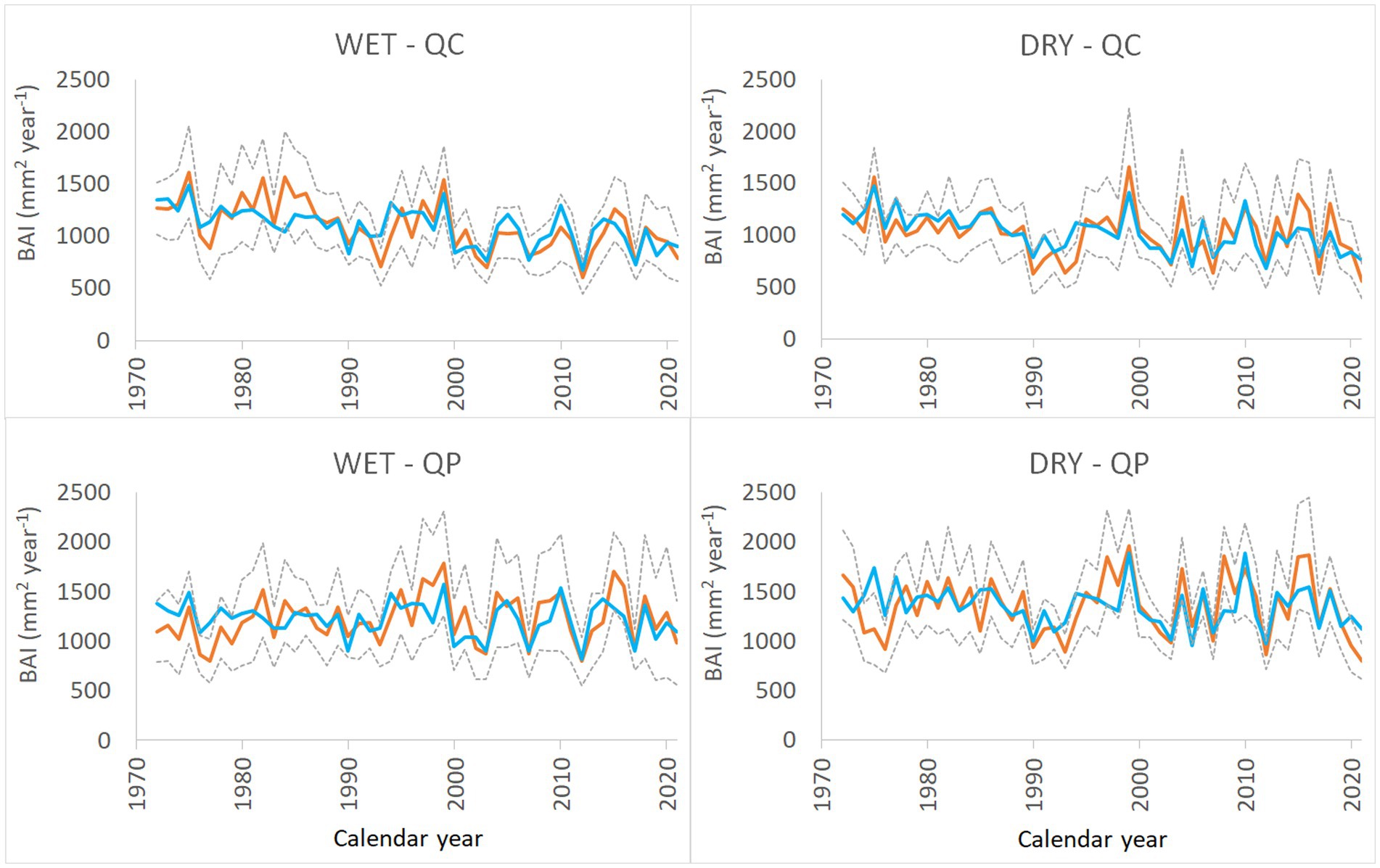
Figure 5. Medians (orange lines) and median absolute deviations (gray dashed lines) of the observed basal area increments of two oak species, QC (Q. cerris) and QP (Q. petraea), growing at the WET and DRY sites from 1972 to 2021. The blue line represents the modeled basal area increments which was predicted by the final model.
3.2 Future growth projections
According to climate scenarios, the WB is anticipated to worsen substantially during summers, especially under the RCP8.5 climate scenario and the second modeled period (Figure 6; Supplementary Table S5). However, the winter WB is expected to be more favorable during this century compared to the period between 1971 and 2021 (Figure 6; Supplementary Table S5). Spring WB conditions may also deteriorate in the future, while autumn conditions may not show noteworthy changes (Figure 6; Supplementary Table S5).
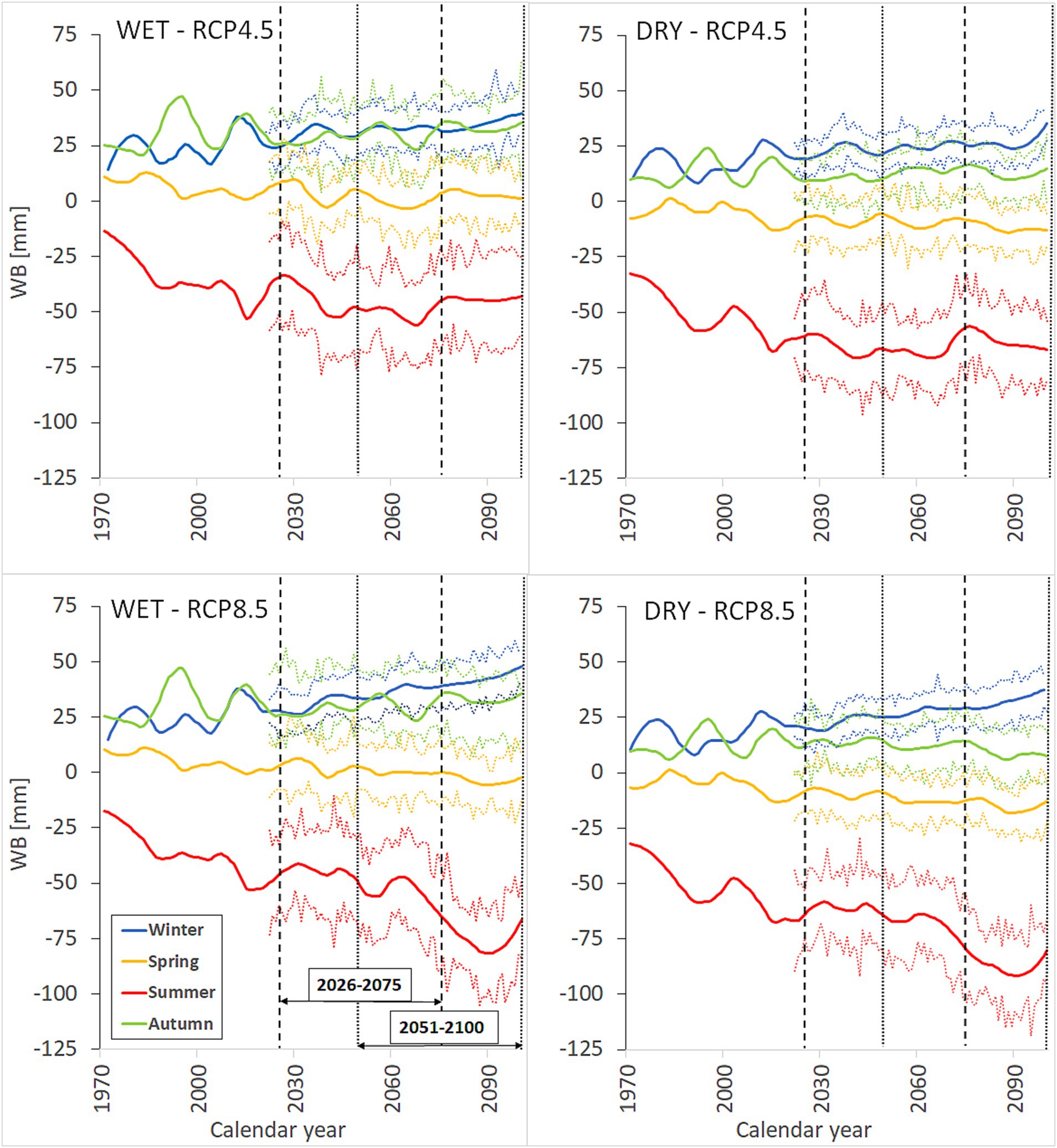
Figure 6. The change of seasonal water balance (mean WB [mm] of the corresponding months) for the study area from 1971 to 2100, based on the RCP4.5 and RCP8.5 climate scenarios. The data has been smoothed using loess smoothing with a span value of 0.2, and the 97.5% confidence interval of standard errors of the climate models is represented (dashed color lines). The observed WB values are until 2021 and the ensemble mean of the 14 regional climate models over the period 2022–2100. The vertical dashed lines separate the two overlapping future modeling periods 2026–2075 and 2051–2100.
Due to climate change, the radial growth of oak trees is expected to decrease slightly over the century in the study area (Figure 7). The simulation results indicated that the growth in the future, under both the RCP4.5 and the RCP8.5 climate scenarios will be significantly lower than in the past (Supplementary Table S6). According to the modeling periods, a significant decline was observed for both modeling periods under the RCP4.5 and for the second modeling period under the RCP8.5 scenarios (Supplementary Table S6). When comparing the basal area changes between sites and species, we found that the decline in growth at the WET site was significantly greater than at the DRY site (Supplementary Table S6). Nonetheless, no significant differences were found between the tree species in terms of how the growth decreases in the future compared to the past. Upon examining a specific site, we observed a significant decline in the growth of both tree species at the WET site during the second modeling period (2051–2100) under the RCP8.5 scenario (Table 3; Supplementary Table S6).
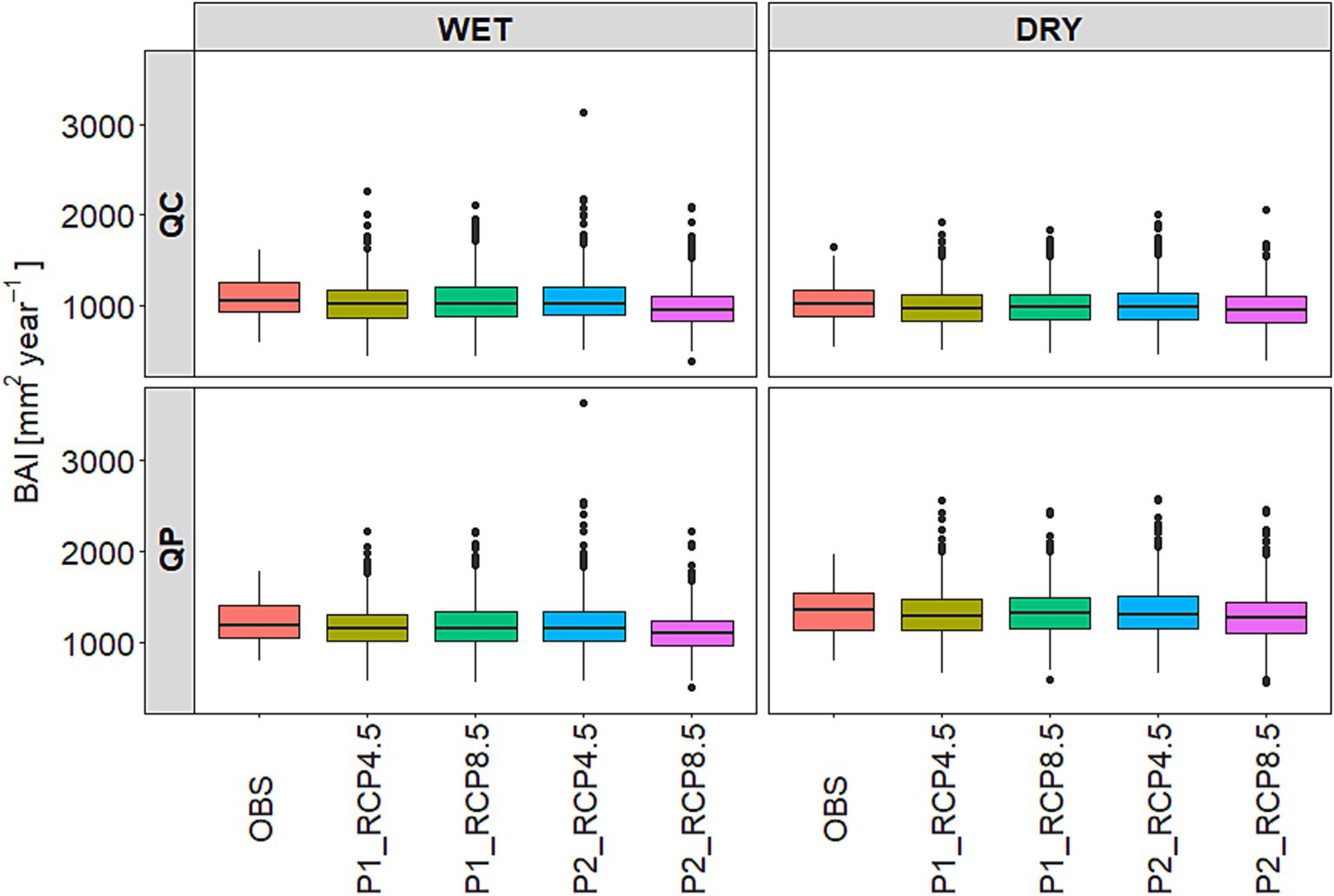
Figure 7. Future basal area index (BAI [mm2year−1]) of the two tree species (QC: Q. cerris and QP: Q. petraea) for the future modeling periods (P1: 2026–2075 and P2: 2051–2100) under the climate scenarios RCP4.5 and RCP8.5. Future BAI values included the annual predictions of the 14 climate models (14 × 50 years) for each tree species, site, climate scenarios and modeling periods. The observed basal area index included the annual median values of the trees for the model calibration period (OBS: 1972–2021, 50 years) for each forest stand. The black lines are the medians, the upper and lower hinges represent the first and the third quartiles (the 25th and 75th percentiles), and the whiskers extend from the hinge to the largest and smallest value that is no more than 1.5 times the interquartile range from the top (bottom) of the box and the black circles denote the outlying values.
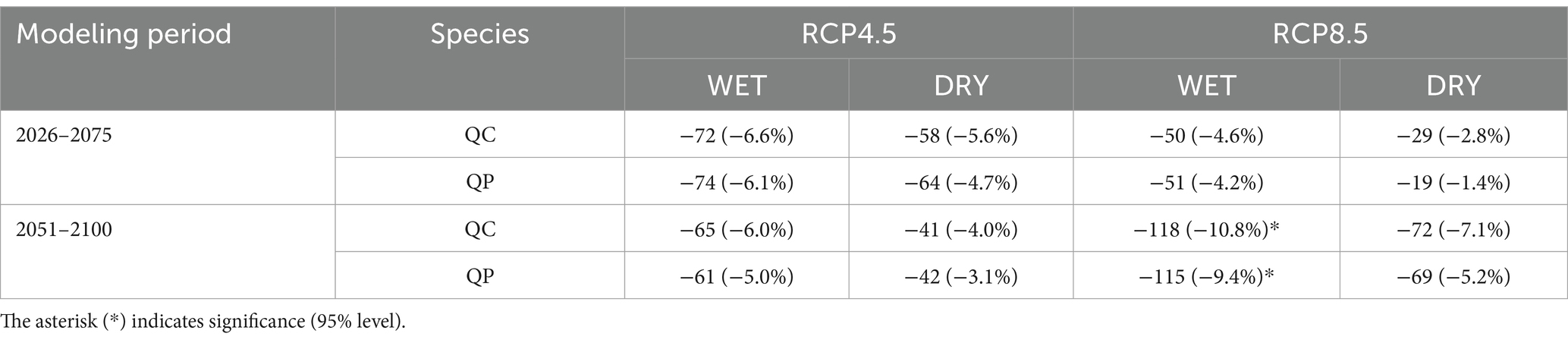
Table 3. The predicted changes (absolute [mm2year−1] and relative [%]) between the sum of the annual medians of BAI for the observation period (1972–2021) and the model simulation periods (2026–2075 and 2051–2100) for the WET and DRY sites and for the tree species QC (Q. cerris) and QP (Q. petraea) using RCP4.5 and RCP8.5 climatic scenarios.
4 Discussion
4.1 Growth-climate relationships
Our growth-climate models revealed that the growth of the selected oak species is dependent on the summer WB. This finding is consistent with other studies that suggest summer precipitation and temperature are critical to the growth of oaks in temperate regions (Cufar et al., 2014 in the Carpathian Basin; Friedrichs et al., 2009 in Central-West Germany; Mérian et al., 2011 in western France; Scharnweber et al., 2011 in northern Germany; Petráš and Mecko, 2011 in Slovakia; Popa et al., 2013 in Romania; Rybníček et al., 2015; Dobrovolný et al., 2016; Stojanović et al., 2017 in Czech Republic; Stojanović et al., 2018 in Serbia; Roibu et al., 2020 in Moldova; Móricz et al., 2021; Mészáros et al., 2022 in Hungary). Some studies have found that the effect of the summer WB becomes less important in more xeric areas (e.g., Bose et al., 2021). However, we have not found such differences between our study sites. Our research has shown that the growth of Q. cerris is more sensitive to the previous year’s summer WB when compared to Q. petraea. This finding is consistent with other studies that have proclaimed the higher growth sensitivity of Q. cerris to summer WB, such as those conducted by Ciceu et al. (2020), Móricz et al. (2021), and Mészáros et al. (2022). The WB of spring was an essential climatic factor that affected tree growth, as noted by several studies in Central Europe (Gárcia-González and Eckstein, 2003; Cufar et al., 2014; Stojanović et al., 2017). This indicates that the WB can affect tree-ring width by influencing the onset of wood formation or the growth rate (Gárcia-González and Eckstein, 2003). The winter WB substantially influences the growth of oak species and this effect varies significantly between DRY and WET sites. During winter, soil water storage is refilled, and if insufficient, the winter WB can become a limiting factor during the growing season, affecting the physiology of earlywood formation (Matisons and Brūmelis, 2012; Martin et al., 2018). Deciduous oak trees rely more on winter than summer precipitation during the growing season, according to a study by Allen et al. (2019), based on the analysis of isotopic signatures from 49 oak sites across Switzerland. Similarly, Bose et al. (2021) and Mészáros et al. (2022) reported the great importance of the previous winter WB for the analyzed oak species. The previous autumn’s WB had a large impact on the following year’s growth of oak species. Numerous studies have also reported a positive effect of precipitation and higher temperature at the end of the previous growing season on radial growth (Mérian et al., 2011; Petráš and Mecko, 2011; Móricz et al., 2021; Mészáros et al., 2022). Oaks may benefit from higher temperatures and sufficient precipitation in the previous year’s September and October, as later leaf senescence and higher final non-structural carbohydrate concentration in sapwood might advance earlywood formation in the following year (Barbaroux and Bréda, 2002; Rybníček et al., 2016; Stojanović et al., 2017; Mészáros et al., 2022). Although Q. cerris and Q. petraea exhibit notable differences in terms of wood anatomy and water relations (e.g., Mészáros et al., 2011; Deaconu et al., 2023), our study could only identify a greater dependence on the previous year’s summer WB for the growth of Q. cerris when compared to Q. petraea.
4.2 Predicted growth for the 21st century
The consensus prediction of 14 regional climate models (Kern et al., 2024) forecasts a rise in mean annual temperature for the study area to 2071–2100, compared to the reference period of 1971–2000 (2.5°C and 4.1°C at the WET site, and 2.3°C and 4.0°C at the DRY site for RCP4.5 and RCP8.5 climate scenarios, respectively). Additionally, the models predict an increase in annual precipitation sum between 9.6 and 10.7% at the WET site and between 9.4 and 11.3% at the DRY site for the RCP4.5 and RCP8.5 climate scenarios, respectively. The comparison of seasonal WB values between the observation and model simulation periods indicated some significant differences (Supplementary Figure S5; Supplementary Table S7). Specifically, the WB values of spring, summer, and autumn are predicted to drop well below the observed range at the DRY site. Meanwhile, at the WET site, only the WB of the summer is projected to fall largely outside of the past observations (Supplementary Figure S5; Supplementary Table S7). However, the WB of the winter is expected to be more positive in the future than it is now (Supplementary Figure S5; Supplementary Table S7).
Due to the remarkable future warming trends, forest ecosystems are expected to face higher constraints on evapotranspiration, intensified soil drought and direct effect of heat stress (Hammond et al., 2022). Without a notable increase in precipitation, the WB will become more negative, especially during the summer season. Consequently, this will lead to hotter droughts, threatening the vitality of forests and causing tree mortality (Allen et al., 2015). However, the projected positive changes in winter WB offset the effect of the deteriorating conditions in summer to some extent. This was particularly true for the DRY site, where the decline of growth is projected to be significantly less severe than at the WET site. Notably, this difference can be attributed to the greater reliance of growth on the winter WB at the DRY site (Figure 3). As a result of the WB changes, the growth of the analyzed oak species is predicted to decrease significantly under the RCP8.5 climate scenario.
Several previous studies have shown that the growth of Q. cerris exhibits a more dynamic growth response to changes in WB than Q. petraea, indicating it is likely better adapted to withstand drought stress (Ciceu et al., 2020; Móricz et al., 2021; Mészáros et al., 2022). However, in our modeling study, we found that while the growth of Q. cerris is projected to decline at a higher rate than that of Q. petraea in the future, the difference between the species was not significant. This is likely because we only identified the previous year’s summer WB as the sole significant difference between the two tree species.
Previous studies have presented conflicting predictions for Quercus species in Central Europe for the 21st century (e.g., Friedrichs et al., 2009; Hlásny et al., 2011; Bauwe et al., 2015; Stojanović et al., 2017). Besides the variability in hydro-climatic conditions of the study areas, other environmental factors may affect tree growth such as the duration of the growing season, CO2 concentration or nitrogen deposition and fertilization (Becker et al., 1994; Bergès et al., 2000; Kint et al., 2012; Doležal et al., 2016; Charru et al., 2017; de Wergifosse et al., 2022). While future warming may lead to more favorable growth conditions for oaks in more humid and cooler areas of Europe, even without a notable change in precipitation (Friedrichs et al., 2009; Härdtle et al., 2013; Stojanović et al., 2017), the same may not be true for areas that are currently warmer and drier. In these areas, the growth of oaks may decline as the available moisture in the soil decreases during periods of drought due to higher evapotranspiration and intensified soil drought (Bauwe et al., 2015; Charru et al., 2017; Mátyás et al., 2018; Petritan et al., 2021). Our study result, which predict slightly declining growth, support this latter scenario, as our study sites are located in the warmer and drier temperate climatic zone of Europe.
4.3 Limitations
Our modeling results related to climate scenarios should be interpreted with caution. We only modeled the effect of changes in seasonal WB on radial growth while considering all other influencing factors to remain constant at limited number of sites. It is important to keep in mind that future growth trends were modeled on a limited number of individuals growing under less intensive silvicultural regimes, which means that the legacy effects of past management might affect long-term growth trends and climatic sensitivity of the tree species in different ways (Petritan et al., 2021). Major silvicultural interventions can make it difficult to distinguish between climate and competition as drivers of tree growth. For instance, trees might show rapid recoveries following drought due to competitive release from declining neighbors. We aimed to select forest stands that were managed similarly and exhibited the lowest management intensity in the broader study area. However, it was apparent that for the sessile oak stands, the stand density was lower at the DRY site compared to the WET site, while the age, BAI, and diameter at breast height (DBH) were similar (Tables 1, 2). According to the yield table for sessile oak (III class) in Hungary, this suggests that the sessile oak at the DRY site has been thinned more substantially during the analyzed period than the stand at the WET site. Although we did not observe significant growth releases during the study period in either stand, there was a moderate event in the early 1990s at the DRY site that was linked to a sanitary cut following a severe drought event (Supplementary Figure S3). This observation was also confirmed by local forest managers.
There is some uncertainty regarding our decision to take only one core sample per tree, as the common practice is to take two cores. However, the trees we sampled had a normal stem shape, so we did not anticipate significant differences among the core samples. Tree cores were extracted in the direction that would be least affected by tension wood. Additionally, Fritts (1976) suggested that one sample per tree would be sufficient for climate studies if the number of sampled trees in a stand exceeds 14. It is important to note that our modeling approach does not account for certain sources of uncertainty, such as extreme climatic events, global radiation trends, heat stress, atmospheric ozone and carbon dioxide levels, nitrogen deposition, biotic effects, reproductive trends, species composition, intra- or inter-specific competition, site productivity, the effect of tree age on physiological processes and different phenotypic plasticity of the species (Magnani et al., 2000; Leuzinger et al., 2005; Bruschi, 2010; Chen et al., 2010; Knapp and Soule, 2011; Grossiord et al., 2014; Martínez-Sancho et al., 2018; Bello et al., 2019; Mátyás, 2021). Forest microclimate differs significantly from the surrounding macroclimate and provides a protective effect for forest tree species against climatic extremes (De Frenne et al., 2019). Canopy structure is a key factor of forest microclimate. Generally, greater canopy closure increases the forest’s ability to buffer against extreme weather through enhanced shading, reduced soil evaporation and air mixing (Kovács et al., 2017; De Frenne et al., 2021). As a result, these conditions lead to a more stable microclimate within the forest, which may significantly impact the water balance and future growth of the trees (De Lombaerde et al., 2022).
We assumed stationarity, i.e., the tree growth responds to climate drivers in the same way (linearly) beyond the observed range of climatic variables. However, recent studies suggest that growth responses to climatic drivers may not always be linear but temporally unstable (Peltier and Ogle, 2020). Abrupt variability in growth responses is commonly observed in reaction to diverse events, so increased frequency and severity of drought events may trigger physiological tipping points leading to a sudden and prolonged decline in growth and subsequent tree mortality (Hammond et al., 2022). As a result, our conclusions regarding the gradual decline of growth over the 21st-century climate change might be influenced by such tipping points and can further impede confidence in our predictions for growth.
5 Conclusion
Our research provides a novel prediction about the future growth of dominant oak trees of the species, Q. cerris and Q. petraea, in Southwest Hungary under different water availability conditions. According to the RCP8.5 climate change scenario, the radial growth of the oak species is likely to decrease significantly during the 21st century. We found that the growth of these oak species in drier conditions depended significantly more on the winter water balance compared to the wetter conditions. As a result, our projection showed a significantly greater decrease in growth in the more humid site compared to the drier site. Although Q. cerris is more sensitive to the water balance conditions of the previous year’s summer, we could not detect significant differences in the future growth of the two oak species.
Our methodology highlights the importance of having reliable historical growth data to assess responses to past climate changes and predict future population dynamics under climate change. The projected growth dynamics are primarily applicable to similar landscapes in Southwestern Hungary with comparable site conditions, and subject to drier conditions in the forthcoming decades. Despite the limitations of our approach, our results can assist in developing forest management plans and species selection strategies in the broader study area. Additionally, it could serve as a methodological recommendation for similar oak sites elsewhere in Central Europe that rely on the climatic water balance.
Data availability statement
The raw data supporting the conclusions of this article will be made available by the authors, without undue reservation.
Author contributions
NM: Conceptualization, Data curation, Formal analysis, Investigation, Methodology, Supervision, Writing – original draft, Writing – review & editing. IM: Conceptualization, Methodology, Supervision, Writing – original draft, Writing – review & editing. GI: Conceptualization, Supervision, Writing – original draft, Writing – review & editing. BG: Investigation, Supervision, Writing – original draft, Writing – review & editing. CE: Data curation, Investigation, Writing – original draft, Writing – review & editing. AK: Data curation, Methodology, Supervision, Writing – original draft, Writing – review & editing. AH: Data curation, Writing – original draft, Writing – review & editing. IB: Investigation, Methodology, Writing – original draft, Writing – review & editing. AB: Funding acquisition, Supervision, Writing – original draft, Writing – review & editing. RH: Data curation, Methodology, Writing – original draft, Writing – review & editing. TN: Conceptualization, Data curation, Investigation, Methodology, Supervision, Writing – original draft, Writing – review & editing.
Funding
The author(s) declare that financial support was received for the research and/or publication of this article. This article was made in the frame of the project TKP2021-NKTA-43 which has been implemented with the support provided by the Ministry of Culture and Innovation of Hungary from the National Research, Development and Innovation Fund, financed under the TKP2021-NKTA funding scheme. The research has been supported by the János Bolyai Research Scholarship of the Hungarian Academy of Sciences (grant no. BO/00291/22/4).
Acknowledgments
The authors express their gratitude to the local state forest company (SEFAG Ltd., branch office Iharos and Szántód) for their valuable support in the field. The laboratory of the Forest Research Institute is also acknowledged for the soil analysis. In addition, our colleagues are thanked for their help with the fieldwork and assistance. Lastly, we would like to express our appreciation to the reviewers for their valuable and constructive criticism.
Conflict of interest
The authors declare that the research was conducted in the absence of any commercial or financial relationships that could be construed as a potential conflict of interest.
The author(s) declared that they were an editorial board member of Frontiers, at the time of submission. This had no impact on the peer review process and the final decision.
Generative AI statement
The author(s) declare that no Gen AI was used in the creation of this manuscript.
Publisher’s note
All claims expressed in this article are solely those of the authors and do not necessarily represent those of their affiliated organizations, or those of the publisher, the editors and the reviewers. Any product that may be evaluated in this article, or claim that may be made by its manufacturer, is not guaranteed or endorsed by the publisher.
Supplementary material
The Supplementary material for this article can be found online at: https://www.frontiersin.org/articles/10.3389/ffgc.2025.1581222/full#supplementary-material
Footnotes
References
Allen, C. D., Breshears, D. D., and McDowell, N. G. (2015). On underestimation of global vulnerability to tree mortality and forest die-off from hotter drought in the Anthropocene. Ecosphere 6, 1–55. doi: 10.1890/ES15-00203.1
Allen, S. T., Kirchner, J. W., Braun, S., Siegwolf, R. T. W., and Goldsmith, G. R. (2019). Seasonal origins of soil water used by trees. Hydrol. Earth Syst. Sci. 23, 1199–1210. doi: 10.5194/hess-23-1199-2019
Árvai, M., Morgós, A., and Kern, Z. (2018). Growth-climate relations and the enhancement of drought signals in Pedunculate oak (Quercus robur L.) tree-ring chronology in eastern Hungary. iForest 11, 267–274. doi: 10.3832/ifor2348-011
Barbaroux, C., and Bréda, N. (2002). Contrasting distribution and seasonal dynamics of carbohydrate reserves in stem wood of adult ring porous sessile oak and diffuse porous beech trees. Tree Physiol. 22, 1201–1210. doi: 10.1093/treephys/22.17.1201
Bates, D., Mächler, M., Bolker, B., and Walker, S. (2015). Fitting linear mixed-effects models using lme4. J. Stat. Softw. 67, 1–48. doi: 10.18637/jss.v067.i01
Bauwe, A., Jurasinski, G., Scharnweber, T., Schröder, C., and Lennartz, B. (2015). Impact of climate change on tree-ring growth of scots pine, common beech and pedunculate oak in northeastern Germany. iForest 9, 1–11. doi: 10.3832/ifor1421-008
Becker, M., Nieminen, T. M., and Gérémia, F. (1994). Short-term variations and long-term changes in oak productivity in North-Eastern France: the role of climate and atmospheric CO2. Ann. For. Sci. 51, 477–492. doi: 10.1051/forest:19940504
Bello, J., Hasselquist, N. J., Vallet, P., Kahmen, A., Perot, T., and Korboulewsky, N. (2019). Complementary water uptake depth of Quercus petraea and Pinus sylvestris in mixed stands during an extreme drought. Plant Soil 437, 93–115. doi: 10.1007/s11104-019-03951-z
Bergès, L., Dupouey, J. L., and Franc, A. (2000). Long-term changes in wood density and radial growth of Quercus petraea Liebl. In northern France since the middle of the nineteenth century. Trees 14, 398–408. doi: 10.1007/s004680000055
Biondi, F., and Qeadan, F. (2008). A theory-driven approach to tree-ring standardization: defining the biological trend from expected basal area increment. Tree-Ring Res. 64, 81–96. doi: 10.3959/2008-6.1
Bose, A., Scherrer, D., Camarero, J., Ziche, D., Babst, F., Bigler, C., et al. (2021). Climate sensitivity and drought seasonality determine post-drought growth recovery of Quercus petraea and Quercus robur in Europe. Sci. Total Environ. 784:147222. doi: 10.1016/j.scitotenv.2021.147222
Bruschi, P. (2010). Geographical variation in morphology of Quercus petraea (Matt.) Liebl. As related to drought stress. Plant Biosyst. 144, 298–307. doi: 10.1080/11263501003672462
Burnham, K. P., and Anderson, D. R. (2002). Model selection and multimodel inference: A practical information-theoretic approach. 2nd Edn. Heidelberg: Springer.
Camarero, J. J., Gazol, A., Sangüesa-Barreda, G., Vergarechea, M., Alfaro-Sánchez, R., Cattaneo, N., et al. (2021). Tree growth is more limited by drought in rear-edge forests most of the times. For. Ecosyst. 8:25. doi: 10.1186/s40663-021-00303-1
Charru, M., Seynave, I., Hervé, J. C., Bertrand, R., and Bontemps, J. D. (2017). Recent growth changes in Western European forests are driven by climate warming and structured across tree species climatic habitats. Ann. For. Sci. 74:33. doi: 10.1007/s13595-017-0626-1
Chen, P.-Y., Welsh, C., and Hamann, A. (2010). Geographic variation in growth response of Douglas-fir to inter-annual variability and projected climate change. Glob. Change Biol. 16, 3374–3385. doi: 10.1111/j.1365-2486.2010.02166.x
Ciceu, A., Popa, I., Leca, S., Pitar, D., Chivulescu, S., and Badea, O. (2020). Climate change effects on tree growth from Romanian forest monitoring level II plots. Sci. Total Environ. 698:134129. doi: 10.1016/j.scitotenv.2019.134129
Cook, R. D., and Weisberg, S. (1982). Residuals and influence in regression. first Edn. New York: Chapman and Hall.
Csóka, G., Hirka, A., Szőcs, L., Móricz, N., Rasztovits, E., and Pödör, Z. (2018). Weather-dependent fluctuations in the abundance of the oak processionary moth, Thaumetopoea processionea (Lepidoptera: Notodontidae). Eur. J. Entomol. 115, 249–255. doi: 10.14411/eje.2018.024
Cufar, K., Grabner, M., Morgós, A., del Castillo, E. M., Merela, M., and de Luis, M. (2014). Common climatic signals affecting oak tree-ring growth in SE Central Europe. Trees 28, 1267–1277. doi: 10.1007/s00468-013-0972-z
Czúcz, B., Gálhidy, L., and Mátyás, C. (2011). Present and forecasted xeric climatic limits of beech and sessile oak distribution at low altitudes in Central Europe. Ann. For. Sci. 68, 99–108. doi: 10.1007/s13595-011-0011-4
De Frenne, P., Lenoir, J., Luoto, M., Scheffers, B. R., Zellweger, F., Aalto, J., et al. (2021). Forest microclimates and climate change: importance, drivers and future research agenda. Glob. Change Biol. 27, 2279–2297. doi: 10.1111/gcb.15569
De Frenne, P., Zellweger, F., Rodríguez-Sánchez, F., Scheffers, B. R., Hylander, K., Luoto, M., et al. (2019). Global buffering of temperatures under forest canopies. Nat. Ecol. Evol. 3, 744–749. doi: 10.1038/s41559-019-0842-1
De Lombaerde, E., Vangansbeke, P., Lenoir, J., Van Meerbeek, K., Lembrechts, J., Rodríguez-Sánchez, F., et al. (2022). Maintaining forest cover to enhance temperature buffering under future climate change. Sci. Total Environ. 810:151338. doi: 10.1016/j.scitotenv.2021.151338
de Wergifosse, L., André, F., Goosse, H., Boczon, A., Cecchini, S., Ciceu, A., et al. (2022). Simulating tree growth response to climate change in structurally diverse oak and beech forests. Sci. Total Environ. 806:150422. doi: 10.1016/j.scitotenv.2021.150422
Deaconu, I., Porojan, M., Timar, M. C., Bedelean, B., and Campean, M. (2023). Comparative research on the structure, chemistry, and physical properties of Turkey oak and sessile oak wood. Bio Resources 18, 5724–5749. doi: 10.15376/biores.18.3.5724-5749
Dobrovolný, P., Rybníček, M., Büntgen, U., Trnka, M., Brázdil, R., Stachǒn, Z., et al. (2016). Recent growth coherence in long-term oak (Quercus spp.) ring width chronologies in the Czech Republic. Clim. Res. 70, 133–141. doi: 10.3354/cr01402
Doležal, J., Lehečková, E., Sohar, K., and Altman, J. (2016). Oak decline induced by mistletoe, competition and climate change: a case study from Central Europe. Preslia 88, 323–346.
Dorado-Liñán, I., Piovesan, G., Martínez-Sancho, E., Gea-Izquierdo, G., Zang, C., Cañellas, I., et al. (2019). Geographical adaptation prevails over species-specific determinism in trees’ vulnerability to climate change at Mediterranean rear-edge forests. Glob. Change Biol. 25, 1296–1314. doi: 10.1111/gcb.14544
Duan, N. (1983). Smearing estimate: a nonparametric retransformation method. J. Am. Stat. Assoc. 78, 605–610. doi: 10.2307/2288126
Friedrichs, D. A., Büntgen, U., Frank, D. C., Esper, J., Neuwirth, B., and Löffler, J. (2009). Complex climate controls on 20th century oak growth in central-West Germany. Tree Physiol. 29, 39–51. doi: 10.1093/treephys/tpn003
Führer, E., Horváth, L., Jagodics, A., Machon, A., and Szabados, I. (2011). Application of a new aridity index in Hungarian forestry practice. Időjárás 115, 103–118.
Gárcia-González, I., and Eckstein, D. (2003). Climatic signal of earlywood vessels of oak on a maritime site. Tree Physiol. 23, 497–504. doi: 10.1093/treephys/23.7.497
Grossiord, C., Gessler, A., Granier, A., Pollastrini, M., Bussotti, F., and Bonal, D. (2014). Interspecific competition influences the response of oak transpiration to increasing drought stress in a mixed Mediterranean forest. For. Ecol. Manag. 318, 54–61. doi: 10.1016/j.foreco.2014.01.004
Hammond, W. M., Williams, A. P., Abatzoglou, J. T., Adams, H. D., Klein, T., López, R., et al. (2022). Global field observations of tree die-off reveal hotter-drought fingerprint for Earth’s forests. Nat. Commun. 13:1761. doi: 10.1038/s41467-022-29289-2
Härdtle, W., Niemeyer, T., Assmann, T., Aulinger, A., Fichtner, A., Lang, A., et al. (2013). Climatic responses of tree-ring width and δ13C signatures of sessile oak (Quercus petraea Liebl.) on soils with contrasting water supply. Plant Ecol. 214, 1147–1156. doi: 10.1007/s11258-013-0239-1
Hirka, A. (2006). Biotic and abiotic damage in the Hungarian forests in 2005, and the damage forecast for 2006. Budapest: Hungarian Forest Research Institute (in Hungarian).
Hlásny, T., Barcza, Z., Fabrika, M., Balázs, B., Churkina, G., and Pajtík, J. (2011). Climate change impacts on growth and carbon balance of forests in Central Europe. Clim. Res. 47, 219–236. doi: 10.3354/cr01024
HMS (2022). Available online at: https://odp.met.hu/climate/homogenized_data/gridded_data_series/ (Accessed April 12, 2022).
Holmes, R. L. (1983). Computer-assisted quality control in tree-ring dating and measurement. Tree-Ring Bull. 43, 69–78.
Illés, G., and Móricz, N. (2022). Climate envelope analyses suggests significant rearrangements in the distribution ranges of central European tree species. Ann. For. Sci. 79:35. doi: 10.1186/s13595-022-01154-8
Kasper, J., Leuschner, C., Walentowski, H., Petritan, A. M., and Weigel, R. (2022). Winners and losers of climate warming: declining growth in Fagus and Tilia vs. stable growth in three Quercus species in the natural beech–oak forest ecotone (western Romania). For. Ecol. Manag. 506:119892:119892. doi: 10.1016/j.foreco.2021.119892
Kern, A., Dobor, L., Hollós, R., Marjanović, H., Torma, C. Z., Kis, A., et al. (2024). Seamlessly combined historical and projected daily meteorological datasets for impact studies in Central Europe: the FORESEE v4.0 and the FORESEE-HUN v1.0. Climate Services 33:100443:100443. doi: 10.1016/j.cliser.2023.100443
Kern, A., Marjanović, H., Csóka, G., Móricz, N., Pernek, M., Hirka, A., et al. (2021). Detecting the oak lace bug infestation in oak forests using MODIS and meteorological data. Agric. Forest. Meteorol. 306:108436:108436. doi: 10.1016/j.agrformet.2021.108436
Kint, V., Aertsen, W., Campioli, M., Vansteenkiste, D., Delcloo, A., and Muys, B. (2012). Radial growth change of temperate tree species in response to altered regional climate and air quality in the period 1901-2008. Clim. Chang. 115, 343–363. doi: 10.1007/s10584-012-0465-x
Kis, A., Pongrácz, R., Bartholy, J., Gocic, M., Milanovic, M., and Trajkovic, S. (2020). Multi-scenario and multi-model ensemble of regional climate change projections for the plain areas of the Pannonian Basin. Időjárás 124, 157–190. doi: 10.28974/idojaras.2020.2.2
Knapp, P. A., and Soule, P. T. (2011). Increasing water-use efficiency and age-specific growth responses of old-growth ponderosa pine trees in the northern Rockies. Glob. Change Biol. 17, 631–641. doi: 10.1111/j.1365-2486.2010.02209.x
Kolář, T., Cermák, P., Trnka, M., Zid, T., and Rybnícek, M. (2017). Temporal changes in the climate sensitivity of Norway spruce and European beech along an elevation gradient in Central Europe. Agric. For. Meteorol. 239, 24–33. doi: 10.1016/j.agrformet.2017.02.028
Kovács, B., Tinya, F., and Ódor, P. (2017). Stand structural drivers of microclimate in mature temperate mixed forests. Agric. For. Meteorol. 16, 39–47. doi: 10.2980/16-1-3178
Kunz, J., Löffler, G., and Bauhus, J. (2018). Minor European broadleaved tree species are more drought-tolerant than Fagus sylvatica but not more tolerant than Quercus petraea. For. Ecol. Manag. 414, 15–27. doi: 10.1016/j.foreco.2018.02.016
Kuznetsova, A., Brockhoff, P. B., and Christensen, R. H. B. (2017). lmerTest package: tests in linear mixed effects models. J. Stat. Softw. 82, 1–26. doi: 10.18637/jss.v082.i13
Leuzinger, S., Zotz, G., Asshoff, R., and Körner, C. (2005). Responses of deciduous forest trees to severe drought in Central Europe. Tree Physiol. 25, 641–650. doi: 10.1093/treephys/25.6.641
Lüdecke, D. (2018). Ggeffects: tidy data frames of marginal effects from regression models. J. Open Source Softw. 3:772. doi: 10.21105/joss.00772
Lüdecke, D. (2024). sjPlot: data visualization for statistics in social science. R package version 2.8.16. Available online at: https://CRAN.R-project.org/package=sjPlot.
Magnani, F., Mencuccini, M., and Grace, J. (2000). Age-related decline in stand productivity: the role of structural acclimation under hydraulic constraints. Plant Cell Environ. 23, 251–263. doi: 10.1046/j.1365-3040.2000.00537.x
Martin, J., Looker, N., Hoylman, Z., Jencso, K., and Hu, J. (2018). Differential use of winter precipitation by upper and lower elevation Douglas fir in the northern Rockies. Glob. Change Biol. 24, 5607–5621. doi: 10.1111/gcb.14435
Martínez-Sancho, E., Dorado-Liñán, I., Gutiérrez Merino, E., Matiu, M., Helle, G., Heinrich, I., et al. (2018). Increased water-use efficiency translates into contrasting growth patterns of scots pine and sessile oak at their southern distribution limits. Glob. Change Biol. 24, 1012–1028. doi: 10.1111/gcb.13937
Matisons, R., and Brūmelis, G. (2012). Influence of climate on tree-ring and earlywood vessel formation in Quercus robur in Latvia. Trees 26, 1251–1266. doi: 10.1007/s00468-012-0701-z
Mátyás, C. (2021). Adaptive pattern of phenotypic plasticity and inherent growth reveal the potential for assisted transfer in sessile oak (Quercus petraea L.). For. Ecol. Manag. 482:118832. doi: 10.1016/j.foreco.2020.118832
Mátyás, C., Berki, I., Bidló, A., Csóka, G., Czimber, K., Führer, E., et al. (2018). Sustainability of forest cover under climate change on the temperate-continental xeric limits. Forests 9:489. doi: 10.3390/f9080489
McCabe, G. J., and Markstrom, S. L. (2007). A monthly water-balance model driven by a graphical user interface. U.S. Geological Survey, Open-File report 2007-1088.
McManus, M., and Csóka, G. (2007). History and impact of gypsy moth in North America and comparison to the recent outbreaks in Europe. Acta Silv. Lign. Hung. 3, 47–64. doi: 10.37045/aslh-2007-0004
Mérian, P., Bontemps, J.-D., Bergès, L., and Lebourgeois, F. (2011). Spatial variation and temporal instability in climate-growth relationships of sessile oak (Quercus petraea [Matt.] Liebl.) under temperate conditions. Plant Ecol. 212, 1855–1871. doi: 10.1007/s11258-011-9959-2
Mészáros, I., Adorján, B., Nyitrai, B., Kanalas, P., Oláh, V., and Levanič, T. (2022). Long-term radial growth and climate-growth relationships of Quercus petraea (Matt.) Liebl. And Quercus cerris L. in a xeric low elevation site from Hungary. Dendrochronologia 76:126014. doi: 10.1016/j.dendro.2022.126014
Mészáros, I., Kanalas, P., Fenyvesi, A., Kis, J., Nyitrai, B., Szőllősi, E., et al. (2011). Diurnal and seasonal changes in stem radius increment and sap flow density indicate different responses of two co-existing oak species to drought stress. Acta Silv. Lign. Hung. 7, 97–108. doi: 10.37045/aslh-2011-0008
Mina, M., Martin-Benito, D., Bugmann, H., and Cailleret, M. (2016). Forward modeling of treering width improves simulation of forest growth responses to drought. Agric. For. Meteorol. 221, 13–33. doi: 10.1016/j.agrformet.2016.02.005
Móricz, N., Illés, G., Mészáros, I., Garamszegi, B., Berki, I., Bakacsi, Z., et al. (2021). Different drought sensitivity traits of young sessile oak (Quercus petraea (Matt.) Liebl.) and Turkey oak (Quercus cerris L.) stands along a precipitation gradient in Hungary. For. Ecol. Manag. 492:119165. doi: 10.1016/j.foreco.2021.119165
Móricz, N., Rasztovits, E., Gálos, B., Berki, I., Eredics, A., and Loibl, W. (2013). Modelling the potential distribution of three climate zonal tree species for present and future climate in Hungary. Acta Silv. Lign. Hung. 9, 85–96. doi: 10.2478/aslh-2013-0007
Muzika, R. M., and Liebhold, A. M. (1999). Changes in radial increment of host and nonhost tree species with gypsy moth defoliation. Can. J. For. Res. 29, 1365–1373. doi: 10.1139/x99-098
Nardini, A., Lo Gullo, M. A., and Saelleo, S. (1999). Competitive strategies for water availability in two Mediterranean Quercus species. Plant Cell Environ. 22, 109–116. doi: 10.1046/j.1365-3040.1999.00382.x
NASA Shuttle Radar Topography Mission (SRTM) (2013). Shuttle radar topography Mission (SRTM) global. Distributed by Open Topography. doi: 10.5069/G9445JDF. (Accessed January 8, 2023).
National Forestry Database, Stand-based inventory (2021). Unpublished data. Available online at: https://erdoleltar.nfk.gov.hu.
Norton, D., Palmer, J., and Ogden, J. (1987). Dendroecological studies in New Zealand 1. An evaluation of tree age estimates based on increment cores. N. Z. J. Bot. 25, 373–383. doi: 10.1080/0028825X.1987.10413355
Nowacki, G. J., and Abrams, M. D. (1997). Radial-growth averaging criteria for reconstructing disturbance histories from presettlement-origin oaks. Ecol. Monogr. 67, 225–249. doi: 10.1890/0012-9615(1997)067[0225:RGACFR]2.0.CO;2
Peltier, D. M. P., and Ogle, K. (2020). Tree growth sensitivity to climate is temporally variable. Ecol. Lett. 23, 1561–1572. doi: 10.1111/ele.13575
Perkins-Kirkpatrick, S. E., and Lewis, S. C. (2020). Increasing trends in regional heatwaves. Nat. Commun. 11:3357. doi: 10.1038/s41467-020-16970-7
Petráš, R., and Mecko, J. (2011). Effect of climatic factors on the dynamics of radial increments of Norway spruce, European beech and sessile oak. J. For. Sci. 57, 293–302. doi: 10.17221/49/2010-JFS
Petritan, A. M., Petritan, I. C., Hevia, A., Walentowski, H., Bouriaud, O., and Sánchez-Salguero, R. (2021). Climate warming predispose sessile oak forests to drought-induced tree mortality regardless of management legacies. For. Ecol. Manag. 491:119097. doi: 10.1016/j.foreco.2021.119097
Pongrácz, R., Bartholy, J., and Kis, A. (2014). Estimation of future precipitation conditions for Hungary with special focus on dry periods. Időjárás 118, 305–321.
Popa, I., Stefan, L., Crǎciunescu, A., Sidor, C., and Badea, O. (2013). Dendroclimatic response variability of Quercus species in the Romanian intensive Forest monitoring network. Not. Bot. Horti Agrobot. 41, 326–332. doi: 10.15835/nbha4119015
R Core Team (2022). R: A language and environment for statistical computing. R Foundation for Statistical Computing, Vienna. Available online at: https://www.R-project.org/.
Roibu, C. C., Sfeclâ, V., Mursa, A., Ionita, M., Nagavciuc, V., Chiriloaei, F., et al. (2020). The climatic response of tree ring width components of ash (Fraxinus excelsior L.) and common oak (Quercus robur L.) from Eastern Europe. Forests 11:600. doi: 10.3390/f11050600
Rybníček, M., Čermák, P., Prokop, O., Žid, T., Trnka, M., and Kolář, T. (2016). Oak (Quercus spp.) response to climate differs more among sites than among species in Central Czech Republic. Dendrobiology 75, 55–65. doi: 10.12657/denbio.075.006
Rybníček, M., Čermák, P., Žid, T., Kolář, T., Trnka, T., and Büntgen, U. (2015). Exploring growth variability and crown vitality of sessile oak (Quercus petraea) in the Czech Republic. Geochronometria 42, 17–27. doi: 10.1515/geochr-2015-0003
Scharnweber, T., Manthey, M., Criegee, C., Bauwe, A., Schröder, C., and Wilmking, M. (2011). Drought matters–declining precipitation influences growth of Fagus Sylvatica L. and Quercus robur L. in North-Eastern Germany. For. Ecol. Manag. 262, 947–961. doi: 10.1016/j.foreco.2011.05.026
Stojanović, D. B., Levanič, T., Matović, B., Stjepanović, S., and Orlović, S. (2018). Growth response of different tree species (oaks, beech and pine) from SE Europe to precipitation over time. Dendrobiology 79, 97–110. doi: 10.12657/denbio.079.009
Stojanović, M., Sánchez-Salguero, R., Levanič, T., Szatniewska, J., Pokorný, R., and Linares, J. C. (2017). Forecasting tree growth in coppiced and high forests in the Czech Republic. The legacy of management drives the coming Quercus petraea climate responses. For. Ecol. Manag. 405, 56–68. doi: 10.1016/j.foreco.2017.09.021
Swanson, A. D., Tayman, J., and Bryan, T. (2011). MAPE-R: a rescaled measure of accuracy for cross-sectional subnational population forecasts. J. Popul. Res. 28, 225–243. doi: 10.1007/s12546-011-9054-5
Thurm, E. A., Hernandez, L., Baltensweiler, A., Ayan, S., Rasztovits, E., Bielak, K., et al. (2018). Alternative tree species under climate warming in managed European forests. For. Ecol. Manag. 430, 485–497. doi: 10.1016/j.foreco.2018.08.028
Vallet, P., and Perot, T. (2018). Coupling transversal and longitudinal models to better predict Quercus petraea and Pinus sylvestris stand growth under climate change. Agric. For. Meteorol. 263, 258–266. doi: 10.1016/j.agrformet.2018.08.021
Varga, F. (1964). Loss in increment due to damage caused by Lymantria dispar in Turkey oak stand. Sci. Publ. Univ. For. Timber Indust. 2, 217–226. (in Hungarian)
Vicente-Serrano, S. M., Beguería, S., and López-Moreno, J. I. (2010). A multiscalar drought index sensitive to global warming: the standardized precipitation evapotranspiration index. J. Clim. 23, 1696–1718. doi: 10.1175/2009JCLI2909.1
Vitasse, Y., Bottero, A., Cailleret, M., Bigler, C., Fonti, P., Gessler, A., et al. (2019). Contrasting resistance and resilience to extreme drought and late spring frost in five major European tree species. Glob. Change Biol. 25, 3781–3792. doi: 10.1111/gcb.14803
Zell, J. (2018). Climate sensitive tree growth functions and the role of transformations. Forests 9:382. doi: 10.3390/f9070382
Zhang, Y., and Schaap, M. G. (2017). Weighted recalibration of the Rosetta pedotransfer model with improved estimates of hydraulic parameter distributions and summary statistics (Rosetta 3). J. Hydrol. 547, 39–53. doi: 10.1016/j.jhydrol.2017.01.004
Keywords: mixed models, dendroecology, basal area increment, climate change, Quercus cerris, Quercus petraea
Citation: Móricz N, Mészáros I, Illés GZ, Garamszegi B, Eötvös CB, Kern A, Hirka A, Berki I, Borovics A, Hollós R and Németh TM (2025) Radial growth projections reveal site-specific futures of different oak species with contrasting water availability in SW Hungary. Front. For. Glob. Change. 8:1581222. doi: 10.3389/ffgc.2025.1581222
Edited by:
Aziz Ebrahimi, Purdue University, United StatesReviewed by:
Pieter Vangansbeke, Research Institute for Nature and Forest (INBO), BelgiumShebeshe Assefa Haile, Free University of Bozen-Bolzano, Italy
Copyright © 2025 Móricz, Mészáros, Illés, Garamszegi, Eötvös, Kern, Hirka, Berki, Borovics, Hollós and Németh. This is an open-access article distributed under the terms of the Creative Commons Attribution License (CC BY). The use, distribution or reproduction in other forums is permitted, provided the original author(s) and the copyright owner(s) are credited and that the original publication in this journal is cited, in accordance with accepted academic practice. No use, distribution or reproduction is permitted which does not comply with these terms.
*Correspondence: Norbert Móricz, bW9yaWN6Lm5vcmJlcnRAdW5pLXNvcHJvbi5odQ==