- 1Unit of Occupational Medicine, Interdisciplinary Department of Medicine (DIM), School of Medicine, University Hospital “Policlinico”, University of Bari “A. Moro”, Bari, Italy
- 2Marine Environment and Pollution Prevention, Department of Prevention, ASL TA Health Company, Taranto, Italy
- 3Institute for the Coastal Marine Environment of the Italian National Research Council (IAMC-CNR), Taranto, Italy
The presence of products containing nanoparticles or nanofibers is rapidly growing. Nanotechnology involves a wide spectrum of industrial fields. There is a lack of information regarding the toxicity of these nanoparticles in aqueous media. The potential acute toxicity of ZnO NPs using two marine crustacean species: the copepod Tigriopus fulvus and the amphypod Corophium insidiosum was evaluated. Acute tests were conducted on adults of T. Fulvus nauplii and C. insidiosum. Both test species were exposed for 96 h to 5 increasing concentrations of ZnO NPs and ZnSO4H2O, and the endpoint was mortality. Statistical analysis revealed that the mean LC50 values of both ZnO NPs and ZnSO4H2O (ZnO NPs: F = 59.42; P < 0.0015; ZnSO4H2O: F = 25.57; P < 0.0015) were significantly lower for Tigriopus fulvus than for Corophium insidiosum. This result confirms that the toxic effect could be mainly attributed to the Zn ions, confirming that the dissolution processes play a crucial role in the toxicity of the ZnO NPs.
Introduction
The number of products containing nanoparticles (NPs) or nanofibers has grown considerably. Nanotechnology involves a wide spectrum of industrial fields and offers the potential for enormous improvements in economic growth, health, technological production and environmental rehabilitation. The increasing use of nanomaterials (NMs) in consumer products has raised concerns about their potential risks for the workers, consumers and environment (1). Understanding the effects of NPs in exposed subjects is becoming a public and occupational health priority since according to some authors by 2020, the number of workers involved in the nanotechnology sector worldwide will be approximately six million (2–6). From a health perspective, animal investigations are important to extrapolate possible biomarkers of exposure and effects as well as to link the biomarkers in experimental animals to biomarkers in humans. Considering the extremely high doses frequently employed in experimental settings and the extremely low NPs-retrieved fractions in biological fluids, potential biomarkers should be validated under low doses and longer periods of treatment and related to long-term effects, such as those potentially experienced by the general and occupationally exposed population (7–10).
Given their diffusion, there is a lack of information in the consulted literature regarding the toxicity of NPs in aqueous media, and the need to implement this information has emerged. Crustaceans are important biological indicators that play a fundamental role as primary and sometimes even secondary consumers. As predators of bacteria, plants, algae, and other invertebrates, or as feeders on substrates, crustaceans have become preferential prey of large organisms, which in turn represent a large part of the human diet (11).
Extrapolating the animal data to humans by estimating an equivalent dose is necessary to lower the risk levels (8). This deficiency in information regarding NP toxicity can affect not only risk assessment but also the formulation of NPs regulations. While there are doubts and uncertainties, legislation will not be able to support the sustainable development of nanotechnologies, and the entire productive sector risks being blocked (3). In fact, competent authorities applying the principle of “no data, no market” (12) could prevent the use and sale of materials that are presumed to be dangerous for which there is no toxicological and ecological information.
General Overview of the Health Implications of NPs for Workers, Consumers, General Population, and Environment
The term “nanomaterial” is defined in the European Commission (EC) recommendation [EC 2011-(696/2011)] (13) NMs are defined by the International Organization for Standardization (ISO) (ISO TS 27687) (14, 15). According to the ISO and European Union (EU) (16), NPs (single, free) can be defined as a subgroup of nano-objects, and their agglomerates (weakly bonded, embedded) and aggregates (strongly bonded, fixed) can be divided into nanofibers, nanorods, and nanoplates. The most commonly used NMs are titanium, silver, silicon, zinc, iron, and calcium NPs. NMs produced annually and used worldwide are reported in Table 1 (17), these may be present singly or in combination with other inorganic or organic molecules, such as lipids, proteins and polysaccharides.
Debia et al. (18) reports that “the 2014 Nanowerk Nanomaterials Database contains more than 3,000 commercially available NMs from over 200 suppliers worldwide” (19).
The nanotechnology-based global market is projected to achieve $3 trillion (3–5). Other authors report that over 1,800 NMs based products are currently available on the market (7, 17, 20). Available data have estimated that the total annual amount of engineered NM produced worldwide is ~11 million tons (21–23).
About NPs of anthropogenic origin, a non-exhaustive list of the main industrial sectors and products where these, especially metal oxide based, are used includes the following: construction, health care, energy, automobile and aerospace industry, chemical industry, electronics and communication, textile, biomedicine, pharmaceutical and cosmetics industries, agriculture, food industry, processing, and packaging, as well as for water treatment and environmental remediation (1, 6, 11, 24–28). In particular, NPs enter the food chain through nanofertilizers, nanopesticides, environmental pollutants or through processed foods where nanotechnology is used to modify taste and the length of time for which a product remains usable and fit for consumption (11, 29). Obviously this entails a possible risk both for workers, in farm and in the food industry, and for consumers.
NPs may be a new challenge for public health, so many national and international agencies [i.e., World Health Organization (WHO), Food and Drug Administration (FDA), ISO, EC etc.], are involved to define an acceptable risk level following their marketing and therefore define their risk assessment, management and governance (16, 30).
EU member states, including France, Belgium, Denmark, and Sweden, have established the obligation to register for the manufacturing, importation and distribution of NMs (31). In the EU, labeling the presence of NPs in consumer products is just required for food, biocides and cosmetics (32).
Moreover, in the EU Program Horizon 2020, Project NPs Pro Safe will be implemented for a “safe by design” concept that is to say that NMs can maintain their physicochemical properties simultaneously with an absent or low level of toxicity (30, 33).
The properties considered for evaluating the effects of NPs on health include both intrinsic (system-independent) as particles size and shape, specific surface area, porosity, hydrophobicity, water solubility/dispersion agglomeration/aggregation, chemical composition, redox potential, photocatalytic activity, and extrinsic (system-dependent) properties as density, dustiness, zeta potential, agglomeration rate and surface affinity, dissolution rate and solubility, and reactive oxygen species generation (23, 34–37). The interaction of NPs with environmental or biological matrices may determines the formation of the so-called “protein corona” (8, 38). This “contamination” of their surfaces is due to adhesion of reactive chemicals and biological compounds, so in this way may lead to NPs alterations such as dissolution or degradation, complexation, aggregation, or agglomeration (16).
NPs can be much more reactive than their corresponding bulk form due to the large active surface area per mass unit (24, 36, 39, 40). All these aforementioned NPs properties are important when we study the human health risk i.e., exposure (deposition and agglomeration), absorption and distribution (transport across biological barriers such as the gut epithelium, blood-brain barrier, or skin), accumulation, and toxic potency (dose-response relationships) (41).
To facilitate hazard and risk assessment, various criteria for grouping manufactured NMs have been proposed (42, 43). Overall, there is a broad consensus on these namely in terms of physical and chemical properties, toxicity and modes of action, biokinetics, interaction with the biological fluids and formation of protein corona, genotoxicity, and the bioaccumulation. This last one is very important because NPs elimination from tissues can be very slow (i.e., years) thus being a chronic irritant stimulus for the organism (10).
Various mechanisms are involved in NP-mediated toxicity, such as oxidative stress with reactive oxygen species (ROS) generation and genotoxicity through both direct and indirect action to genetic material (11, 44, 45).
By virtue of the mechanism just described, it should also be emphasized that certain nanotubes, such as asbestos fibers, show carcinogenic effects. The IARC (International Agency for Research on Cancer) has classified these as possibly carcinogenic to humans—Group 2B (46). This classification was made because of the interactions between NMs and the immune system that may result in sterile inflammatory responses like that induced by asbestos fibers (33).
Human exposure to NPs may alter heart rate and blood pressure (16, 47). NPs toxicity has also been reported in the digestive system, nervous system, kidney, liver, reproductive system, and skin and has been reported to alter body immune responses in exposed subjects (11).
To define the impact of NPs on both the ecosystem and human health, some authors suggest to study the human health impact of low-dose by long-term exposure by means of applying the hormesis concept: “biphasic dose–response relationship characterized by a low-dose stimulation and high-dose inhibition” to NPs exposure, of workers and the general population (48). The conditions of hypersusceptibility and inflammation in response to NPs should be ascertained to predict and eventually control variable adverse outcomes/pathological responses following NM exposure and to prevent a possible flare-up of chronic diseases, such as those induced by mixtures of NMs (49).
On the other hand environmental pollution of NPs can lead to interferences at food chain, and ingested NPs can possibly be translocated from the intestine into the lymphatic system and other organs (26, 41, 50). Inhalation of NPs by patients with asthma or chronic obstructive pulmonary disease, has been demonstrated to induced chronic inflammation, in the same way this type of exposure may increase allergic reactions in atopic patients (16). NPs can be transported in the blood and lymphatic system and bypass the hematoencephalic barrier via olfactory bulb (39, 51, 52). Through blood circulation, NPs can then reach other organs, i.e., liver, kidney, spleen, and hematopoietic and nervous systems (11, 51, 53).
Oral exposure may be accidental due to presence of NPs or contaminants in food, water and consumer products (54). This kind of exposure may include uptake of residues from cosmetics or dishwashing products or can occur as a consequence of the clearance of lung-transporting materials out of the lung with the mucus, which is ultimately ingested. So ingestion of MNs via food or its contact materials is the most relevant source of oral exposure (32). The European Food Safety Authority (55) distinguishes two different mode of exposure for NPs in food, namely if there is no exposure i.e., no persistence in marketed preparations or no NM migration from food to contact materials or, on the contrary, if there is complete NM transformation in food before ingestion or during digestion. However, to date, is not know the actual determinant of consumer exposure, i.e., the transfer factor, which is the fraction of the substance transferred from the product to the air, mouth or skin and represents the estimated dose of exposure (41).
The general human population environmental exposure to NPs may be due to their release by atmospheric factors from NM waste or from NMs in catalytic paints (32, 56). NPs environmental pollution may also derivate from sewage treatment plants, abrasion from tires, disposal and incineration of waste, and direct application of NPs in agriculture (32, 56).
Lastly workers could be exposed during production and processing of products containing engineered NPs (57).
Obviously, workers may be exposed for long periods and to high levels in respect to those of consumers of NM-containing products (42). In addition to inhalation and cutaneous routes workers gastrointestinal exposure may result from the mucociliary clearance of inhaled NMs, or due to lack of personal and industrial hygiene standards (58, 59).
There have been reports that among workers exposed to NPs, the risk of developing respiratory, cardiovascular and neurological disorders is increased (57). NPs may also aggravate existing diseases, such as asthma, chronic obstructive pulmonary disease and bronchitis as well as may increase inflammation and change lung function even at low engineered NPs exposure (11, 52).
Some examples of occupational exposures to NPs reported in the literature are in the dental laboratory with the use of plaster for fillings containing zinc phosphate cements with ZnO or MgO particles in the powder form (26). While upper-airway inflammation, with possible anosmia and hyposmia, it was reported in photocopier workers exposed to NPs, while in the past these pathologies were attributed to volatile organic compounds (VOCs) (51).
Nowadays, a new challenge to protect workers and the environment is represented by the study, by multidisciplinary cross fertilization, of the adverse effects of the substances at the nanoscale because of the wide and uncontrolled diffusion of NPs in the general environment, the same substances historically studied at the macroscale.
A lot of examples may be considered, regarding the adverse effects on the environment and on men of various substances studied both at macro and NPs level, so regarding xenobiotics exposure in the general population (60–62) reported stress responses in plants exposed to heavy metals or metal-containing NPs, while Gifford et al. (63) showed that novel nano enabled sorbents can reduce multiple contaminants of health concern, resulting in groundwater that was treated to drinking water standards.
Concerning air pollution and allergic diseases (64, 65) studied the prenatal exposure to urban freeway nanoparticulate matter (n PM), vehicular aerosols, that may alter neuronal differentiation, with a toxicological model on mouse. Regarding exposure to PAHs, in workers and the general population (66, 67) recently reported the combined use of ozone, carbon nano-onions with subsequent biological degradation as a means of removing PAHs from an urban runoff or a commercial waste stream. Topuz and Uyar (68) described a new method for PAH removal from aqueous solutions using silica NPs, while Celebioglu et al. (69) using nanofibers poly-cyclodextrin membranes. Zambrano-Zaragoza et al. (70) reported that nanosystems may be candidates for efficient edible coatings for food preservation, thus allowing the incorporation of antimicrobial and antioxidant ingredients. However, this implies risks of both inhalation and oral exposure for workers and for consumers. Regarding the exposure by inhalation of traffic air pollution to pathogenic agents and other ubiquitary toxic agents, as formaldehyde, for the respiratory system (71–74) studied the concentrations of charged NPs near busy roads where traffic consisted of heavy-duty diesel vehicles that typically had high particle and charge emission rates. The presence of charge on inhaled particles can influence their effect on human health (75). Regarding the respiratory health of waste collection and disposal workers (76, 77) showed that in waste incineration plants, depending on the particle sizes, the NPs present in the fly ash may produce environmental pollution.
The overall effects of occupational exposure to engineered NPs on humans and environment have not yet been well-identified (25, 30, 32, 43, 52, 78). For many NPs, to date, data on dose-response, exposure assessment and risk characterization, are insufficient to define risk management (1, 6, 7, 11, 49, 75). In a recent systematic review, only 56 occupational exposure limits (OELs) developed for engineered NMs have been reported (36, 75). As previous referred due to the interaction of NPs with environment or biologic fluids consumers, workers and environment are exposed to transformed NMs, that may have acquired different toxicity profiles (11, 79).
ECHA has released a guidance document (80) on specific methods for calculating the inhalation, oral and dermal exposures for workers and consumers (32).
There is a general consensus on the fact that “nano” size means that the exposure limits determined for substances at macro level are not necessarily valid with respect NPs in regard to occupational, environmental and public health (28).
Until health based OELs are developed and released by official regulatory agencies for biomonitoring and environmental monitoring, to define and establish regulations for the safe use of NPs and to further develop universal standardizing methods, any possible exposure for workers, consumers, and general public needs to be minimized with a conservative approach according to precautionary principles (6, 7, 10, 15, 17, 25, 32, 54, 59, 81). Understanding the effects of NPs at exposed working contexts is becoming a public and occupational health priority due to the widespread application of nano-enabled products and the increased likelihood for consumer and workplace exposures (8, 32). NPs are an environmental health problem for the general population and for the whole biota (8, 32). The study of human and environmental toxicology is required to understand the relationships between the environmental biophysical and chemical characteristics as the biological reactivity of NPs during their lifecycle, including their disposal/recycling (48, 49).
Risk assessment and regulatory programs of NPs for occupational safety, consumer safety, and safety of the general public and environment are necessary for correct risk management, i.e., risk prevention, risk mitigation, and risk communication (25). NPs will be the next challenges for the protection of occupational and environmental health. Answers should be given to the questions on “What they are—Characterization; Where they go—Destiny and their persistence; What do they do—(Re) activities” (34, 82).
ZnO NPs
Engineered NMs are Part of NPs and Represent the Last Frontier of the Industry. The NMs Produced in This Way Can Be Made Up of a Single Type or Combination of Engineered NPs.
According to the National Science Foundation, in the next decade, the miniaturization industry will be worth $ 1 trillion (83). Among the nano-metal oxides, the highest global production is estimated for TiO2, SiO2 and ZnO (84).
Zinc oxide is an essential ingredient of many enzymes, sunscreen in which zinc oxide (ZnO) particles are added as ultraviolet (UV) light filters, cosmetics.
ZnO NPs, are used in food products as additives, supplements, containers and packaging; in the energy sector as fuels and catalysts; in consumer electronics, semiconductors, and air filter; in the pharmaceutical industry; in biomedical engineering and also in drinking water (6, 26). These NPs have UV emission capacity, conductivity and piezoelectricity, making ZnO NPs particularly interesting for applications of electronic sensors, solar photovoltaics and transducers (85).
ZnO NPs are also a very effective photographic catalyst material with excellent UV absorption and reflection properties (86).
Impact of zinc oxide on biological functions depends on its morphology, particle size, exposure time, concentration, pH, and biocompatibility. ZnO NPs, are effective against microorganisms, their action mechanism has been ascribed to their activation by light, and their ability to disintegrate the cell membrane and accumulate in the cytoplasm where causing cell death. ZnO NPs are used in biomedicine, due to their excellent biocompatibility and low toxicity, especially as anticancer and antibacterial, because of their potent ability to trigger excess reactive oxygen species (ROS) production, release zinc ions, and induce cell apoptosis. Moreover, zinc may keep the structural integrity of insulin and is used as antidiabetic treatment (87). ZnO NPs show excellent luminescent properties and have turned them into one of the main candidates for bioimaging (88).
Generally, exposure to ZnO has been linked to adverse health and environmental effects (89–92) reported oxidative DNA damage in workers exposed to metal oxide Nms. ROS may cause ZnO NPs-induced cytotoxicity and genotoxicity. ZnO NPs are more toxic than other metallic oxide NPs because of their ion-shedding ability. ZnO NPs are not very soluble in neutral solutions on the contrary in acid environments. ZnO NPs induced significant cytotoxicity in a size dependent manner. The ZnO NPs may cause neurotoxicity and probably reached the brain by olfactory system (7, 8).
Zinc oxide NPs have also been shown to induce apoptosis in colon carcinoma cells by oxidative stress leading to cytotoxicity by inflammatory responses, mitochondrial membrane alterations, and IL-8 release in cancerous cells (11, 93). Hematological alterations were reported in animals after oral or intragastric exposure to ZnO NPs (94). Chuang et al. (95) demonstrated that ZnO NPs impair cardiopulmonary functions.
Overall, the effects observed in healthy human volunteers suggest that systemic inflammation follows ZnO-Engineered NM exposure, which may be explained by either primary local inflammation of the respiratory tract/lung and secondary resorption of inflammatory markers or primary systemic inflammation due to resorbed zinc ions.
About worker's exposure (96) has shown the possible inhalation of ZnO NPs during the spray application and power sanding of common wood sealant. Inhaled ZnO NPs fumes produced by thermal cutting, welding, and other occupational activities are able to produce, after a latency period of 4–12 h, the characteristic “zinc fever” (throat irritation, coughing, metallic taste, flu-like symptoms) (97). Other studies in the past described the development of zinc fever after exposure to 5 mg/m3 and a slight increase in body temperature after exposure to 2.5 mg/m3 ZnO NPs (98, 99). Exposure to ZnO NPs confirmed the higher incidence of cardiovascular disease found in welders (7, 8). Increases in the number of blood leukocytes and the amount of highly sensitive C-reactive protein (hsCRP) due to the exposure to 1.5 mg/m3 ZnO NPs from the welding fumes of zinc coated steel were also reported in different studies (11, 100–102).
Other results indicated a no-observed-effect Level (NOEL) for ZnO NPs with concentrations of 1.1–1.5 mg/m3 contained in welding fumes with concentrations of 1.5–2.0 mg/m3 (PM10) (103). These findings highlight the occupational health effects for ZnO NPs-exposed workers.
The influences of ZnO nanostructures, such as nano-plate, nano-rod, and nano-flower, on various human cancer cells were studied by examining reactive oxygen species (ROS) and genotoxicity (104). They can also be cytotoxic and genotoxic to multiple types of human cells [i.e., neuronal or epithelial cells; (105)].
About the dimension of these NPs the toxicity is generally related to their chemistry not only to their size (106, 107). The small size, ease of transport within tissues/organs, ability to cross plasma membranes, are used in biomedical applications. At the same time the small size don't have increased adverse effects on skin respect to the larger ones (108, 109).
Experimental toxicological studies have shown that numerous phosphorylcholine-containing lipid (PC-CL) species were altered when exposed to high and moderate concentrations of fine ZnO NPs. The toxicity of these NM is commonly attributed to oxidative stress, inflammation and cell membrane damage caused by lipid peroxidation (108, 109). Studies on mice indicated that the liver, kidney, lung, and pancreas were target organs for the cumulative oral exposure of 50-nm nano-ZnO and might be target organs for subchronic and chronic toxicity of orally administered 50-nm ZnO (110). The mutants of yeast (Saccharomyces cerevisiae) seems to be more sensitive to the NPs' nanospecific toxicity. These effects were attributable to dissolved zinc ions from the ZnO (nano or bulk) particles. Oxidative damage and mechanical damage contributed to the toxic effects of the ZnO particles (104).
ZnO NPs, a wide-band-gap ntype semiconductor, can interact with lipopolysaccharide molecules present in the outer membrane of Escherichia coli (87), as well as produce reactive oxygen species (ROS) under UV illumination.
Inhaled ZnO NPs cause sustained renal periglomerular and interstitial inflammation through lymphocytic infiltration in Sprague-Dawley rats (111). Hou et al. (91) summarized the toxic effects of ZnO NPs in different exposure conditions on different species. Minetto et al. (112) in his review on saltwater ecotoxicology of ZnO NPs underlined criticisms and limits referred in the current studies of their toxicity. Khosravi-Katuli et al. (113) suggested that the size of ZnO NPs can influence their toxic potential as well as the release of these NPs in the aquatic environment in presence of other contaminants. Effects of ZnO NPs on marine crustaceans have been studied using saltwater microcrustacean (114, 115), linking time variable exposure (116), or different trophic level in multi NM system (117).
The aim of this study was to provide a general overview of the health implications of NPs for workers, consumers and the general population. In this perspective, because the data on the toxicity of ZnO NPs toward some marine crustaceans remain unexplored with the risks for humans related to the food chain, the purpose of the research was to evaluate the potential acute toxicity of these using two marine crustacean species, the copepod Tigriopus fulvus and the amphipod Corophium insidiosum, and to assess their mortality upon exposure to different concentrations of ZnO NPs and the effect of the “dissolution processes” on the toxicity of the ZnO NPs.
Materials and Methods for the Experimental Study
Test Condition
The study evaluated the toxicity of zinc oxide nanoparticles (ZnO NPs) through the execution of acute tests with two species of native crustaceans, characterized by high representativeness and diffusion in the Mediterranean area: the copepod Tigriopus fulvus and the amphypod Corophium insidiosum. Crustaceans are placed at a key trophic level in the food chain because they connect energy flows between primary producers (algae and phanerogams) and higher-level consumers (e.g., fish) and therefore also represent a powerful vehicle for the recycling of deposited pollutants in sediments.
For these reasons, crustaceans are among the most widespread model organisms in acute biotests for the evaluation of toxicity (118).
The species selected for this study belong to distinct taxonomic groups, occupy different ecological niches, and were chosen according to their ecological relevance (key species in the food chain):
• Ease of retrieval and ease of management (available and/or livestock)
• Sensitivity to different toxic compounds
• Reproducibility of the results
• Availability of standardized methods for carrying out the tests.
Acute tests were conducted on young T. fulvus nauplii and C. insidiosum adults.
The tests were repeated three times, and the concentration that resulted in the death of 50% of individuals after 96 h of exposure (LC50) was chosen as an endpoint The results were expressed as % mortality (±sd) with a 95% confidence limit. The reference method used for conducting the toxicity tests was the ISO protocol (2005) (119). The test was considered valid when the survival of the negative control was >90% [Organization for Economic Co-operation and Development (OECD) guideline 202, 2004] (120). The detailed conditions of the assay are shown in Table 2. The algal cultivation procedures are similar to those reported in previous experiences (121–126).
Test Conditions With Tigriopus fulvus
The algal cultures were prepared starting from algal strains belonging to the phytography of the IRSA Institute of Taranto and were placed in soil F2 with continuous oxygenation.
The tests were carried out on synchronized stage I-II nauplii, from a culture of homogeneous organisms, hatched within 24–48 h. This choice was made because the results of previous experiments showed a great sensitivity of nauplii compared to that of adults (122, 127, 128) and a reduced variability of the results (123, 124). The algal cultures of Tetraselmis suecica and Isochrysis galbana, at a ratio of 1:2, corresponding to 1.5 × 108 and 3.0 × 108 cell/L, were added to the net/support; the plate was placed in a thermostatic environment under the same breeding conditions. Figure 1 shows the preparation of the assay (selection of females with an egg-shaped bag).
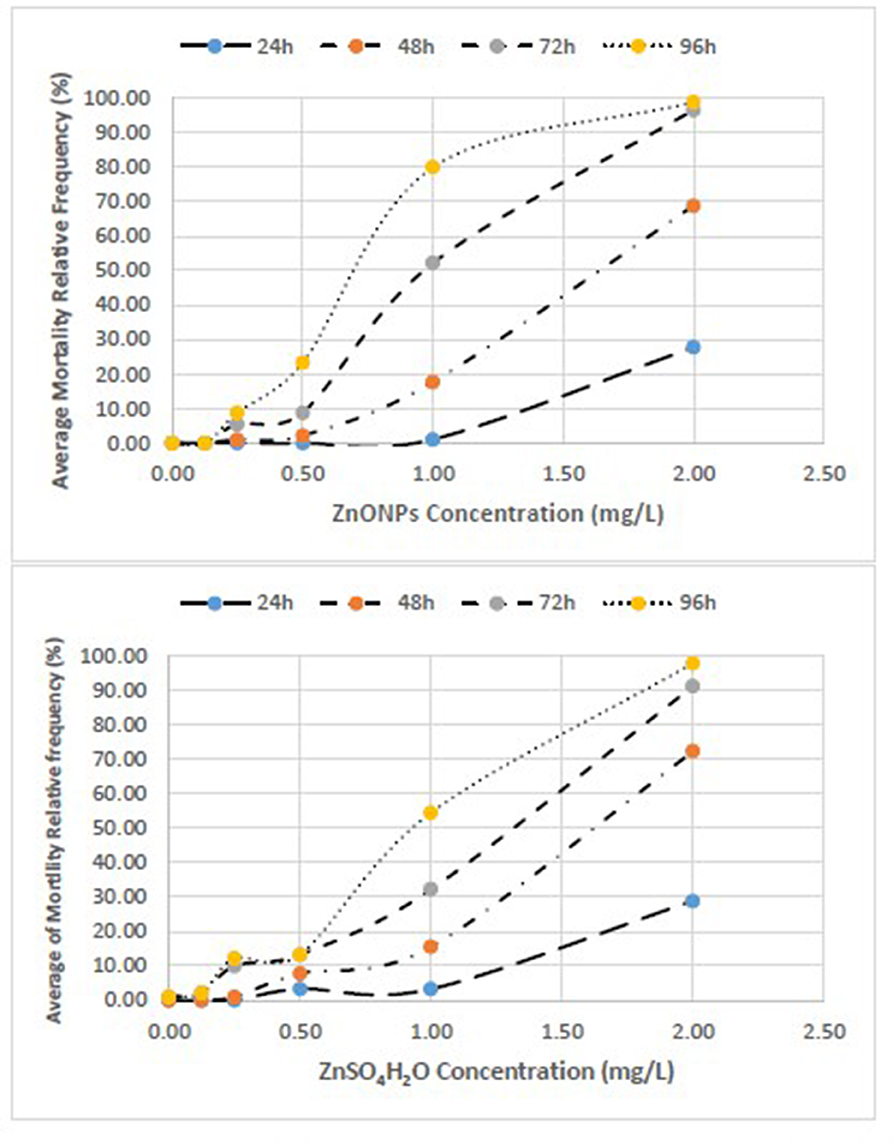
Figure 1. Concentration-effect relationship between Average Mortality Relative Frequency (%) of T. fulvus and concentration of ZnONPs and ZnSO4H2O.
Each test was performed in a controlled environment under the same breeding conditions and repeated three times. The nauplii were exposed to the suspensions/test solutions for 96 h. The suspensions/solutions of ZnO NPs and ZnSO4H2O were prepared at scaling concentrations of 0.125–0.25–0.5–1.0–2.0 (mg/L). For each treatment and for the control, three replicates were made, each containing 10 nauplii in 3 mL of the test solution. At the end of the exposure period, the dead organisms were counted on the stereoscope. The criterion for defining mortality was the absence of movement of the appendages for an observation period of more than 20 s under light and mechanical stimulation.
T. fulvus, originally from the Tyrrhenian Sea (locality Calafuria, Livorno), has been cultivated for several months in the laboratory of ecotoxicology of CNR IRSA of Taranto, in seawater filtered with a 0.45 μm filter, inside 150 cm2 polystyrene culture flasks (0.5 L) with a ventilated cap fitted with a 0.22 mm membrane under the following conditions (121, 127, 128):
• Salinity at 38 PSU;
• Temperature of 20 ± 2°C in a thermostatic chamber;
• Photoperiod of 16L/8B at a brightness of 500–1,200 lux;
• Weekly feeding with Tetramarin®, Tetraselmis suecica, and Isochrysis galbana.
Test Conditions With the Amphypod Corophium insidiosum
The tests were carried out with organisms ~10–14 days old with a size between 2 and 4 mm, i.e., young, and not yet sexually mature. This selection criterion allows the discarding of very small newborn individuals and the most resistant adults, including the ovigerous females who could release the babies during the test.
The juveniles used for the tests were collected and separated from the adults by sifting the upper 2 cm of sediment through a 500 μm mesh sieve.
Toxicity tests were performed by exposing the organisms to increasing concentrations of the two toxic agents over a period of 96 h in the absence of sediment. Ten individuals of C. insidiosum were randomly selected from the breeding tanks and placed in a 250 mL beaker containing 200 mL of the suspension/test solution; three replicas were performed for each concentration. For both toxicants, 5 dilutions were used with a factor of 2 starting from 0.4 (mg/L) up to 6.4 (mg/L) [0.4–0.8–1.6–3.2–6.4 (mg/L)]. The experimental conditions of temperature, salinity and photoperiod during the test are the same as those adopted during acclimatization. The details of the test are shown in Table 2. The animals used for the tests, C. insidiosum, were collected from an area located at Mar Piccolo in Taranto (Ionian Sea, southern Italy), which was used as a reference site due to the low levels of anthropogenic pollution (125).
The aquariums were maintained under the following conditions until the tests were carried out:
• Salinity at 36 PSU;
• Temperature of 16 ± 2°C in a thermostatic chamber;
• 12L/12B photoperiod at a brightness of 500–1,200 lux;
• Weekly feeding with 2 drops of aquarium feed (Liquefy Marine, Interpret Ltd., Dorking, UK) per liter and with the benthic microalga Phaeodactylum tricornutum. The animals were acclimatized to the experimental conditions for at least 7–10 days after removal from the field before the tests were performed. The sea water was changed weekly.
Preparation of the Test Solutions
The ZnO NPs were purchased from US Research Nanomaterials, Inc., as an aqueous dispersion (20% by weight, 99.5% purity) with a nominal particle size in the range of 30–40 nm. The stock suspension of ZnO NPs (1,000 mg/L) was prepared in Milli-Q water filtered with a 0.22 μm filter from a 20% dispersion and ultrasonicated for 15' in a sonicator bath (305 W, 50–60 Hz; Soltec Ultrasonic Baths); the ZnO NPs were then stored in the dark at 4°C until the tests were performed. An intermediate suspension of 100 mg/L was subsequently prepared from a 1,000 mg/L stock suspension. The final test suspensions were prepared from the stock suspensions and sonicated immediately before each preparation in natural sea water (NSW) filtered with GFC Whatman filters (0.22–0.45 μm); the suspensions were vortexed for 5 s before the preparation of the toxicity tests without sonication. To evaluate the accuracy and reproducibility of the data under standard conditions, as well as the sensitivity of the populations employed, the ecotoxicity of the Zn ion was evaluated as a positive and solubility control. Zinc (Zn, purity ≥ 99.9%) was purchased from Sigma-Aldrich. The zinc solution was prepared by dissolving zinc sulfate (II) monohydrate (ZnSO4H2O) in Milli-Q water to obtain a metal concentration of 1,000 mg/L. Another intermediate solution of 100 mg Zn/L was subsequently prepared from the stock solution of 1,000 mg/L. Finally, the intermediate solution was further diluted in sea water filtered in calibrated flasks until the final test concentrations of the range to be tested were obtained. The identity of the compounds under examination, the CAS number, the supplier and purity are reported in Table 3.
The aggregation/agglomeration, of ZnO particles across the concentrations, applied in the medium (NSW) and the effective size of ZnO NPs was evaluated by means a Dynamic Light Scattering instruments (NICOMP™ 380 DLS Particle Size Analyzer, PSS, FL, USA) and by Dispersion Analyser LUMISizer (LUM GmbH, Berlin), at time 0 and after 48 h. Moreover, for DLS, size distributions were calculated by NICOMP™ cumulants analysis, that selects the best fitting distribution and calculate the intensity-weighted diameters (± standard deviation). Samples were read twice after 5 min for each sample. For LUM the particle size distributions were calculated by using SEPView 6.3 (LUMiSizer software). Zeta (ζ-) potential of the ZnO NPs dispersed in NSW was also measured by DLS; measurements were carried out in triplicate, each consisting in 5 runs.
The dissolved Zn concentrations of ZnO NPs and ZnSO4 suspensions test were measured by Inductively Coupled Plasma Mass Spectrometry (ICP-MS) (126).
The semistatic tests were conducted in the absence of a power supply; the suspensions/test solutions were renewed every 48 h. The water quality parameters (temperature, pH, dissolved oxygen, and salinity) were measured at the beginning and end of each test to ensure the acceptance criteria of toxicity tests (ASTM 1993, ISO 2005) (119, 129). The results are expressed as a percentage of the effect with respect to that of the control. The significance of the data obtained was evaluated on the basis of the difference between the sample and the control using Student's t-test for paired data. The tested matrix was judged to be toxic when the difference between the sample and control was statistically significant (p < 0.05). The Microsoft EXCEL program was used for recording the data and graphing the outcomes.
Each experiment was repeated three times with three replicates (n ≥ 9). The tests were considered acceptable when the control mortality was 0%. A mortality value <10% among the negative controls, conducted on water only, was included in the acceptability parameters. At first they were evaluated absolute and relative mortality frequencies of both the T. fulvus and C. insidiosum at 24, 48, 72, and 96 h for both ZnONPs and ZnSO4H2O. Were also built concentration-effect curves.
Analysis of variance (ANOVA) was used to perform a statistical comparison between the samples to evaluate if the toxicant used (zinc) had a significant effect on the responses of the test organisms compared to those in the control group; two species were used, and two toxicants were compared. When significant differences were found (p < 0.05), the post-hoc Tukey test was used for multiple comparisons between different concentrations and control. The assumptions of normality and homogeneity of the variances were evaluated by means of the Kolmogorov-Smirnov test and Levene's test, respectively. Statistical elaborations were performed with Statgraphics Version 5 Plus software (Statistical Graphic Corporation, ManugisticsTM, Rockville, MD, USA) and Stata 15 software was also used for analysis (StataCorp. Stata: Release 15. Statistical Software. College Station, TX, USA: StataCorp LP; 2017).
The values of LC50 with 95% confidence limits were determined using the Litchfield-Wilcoxon method (130). Finally, reproducibility, acceptability and stability of the estimates of the three tests in both the groups of T. fulvus and C. insidiosum were based on the evaluation of the dimensions of CL95% and Variation Coefficient (VC).
Results
We clearly reported the absolute frequencies of deaths (n) for the three replicates of each test and the estimates of mortality based on the three test averages of the relative frequencies (%) (Tables 4–6). We also represented the concentration-effect curves, showing their trends, by time of exposure (24–96 h) (Figures 1, 2) and by different compounds (Figures 3, 4).
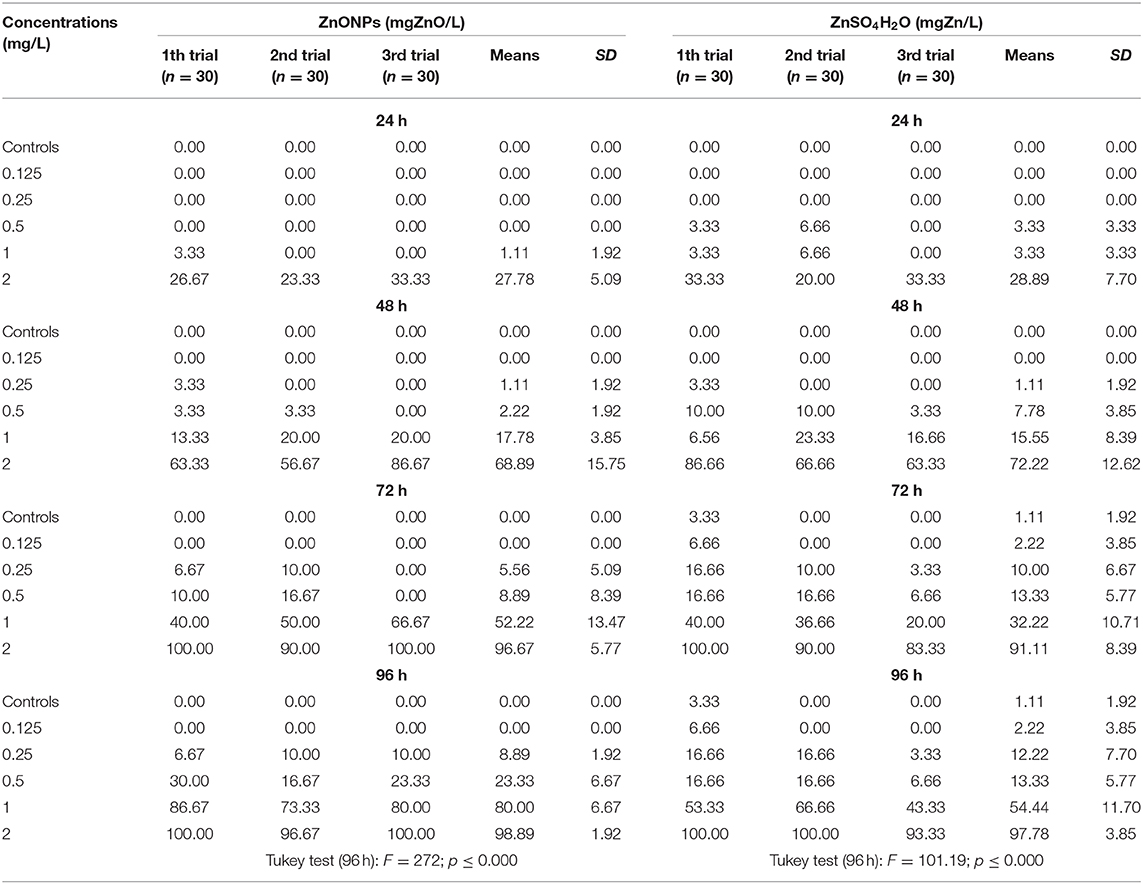
Table 4. Mortality (relative frequencies of deaths [%]) of T. fulvus by time and different concentrations.
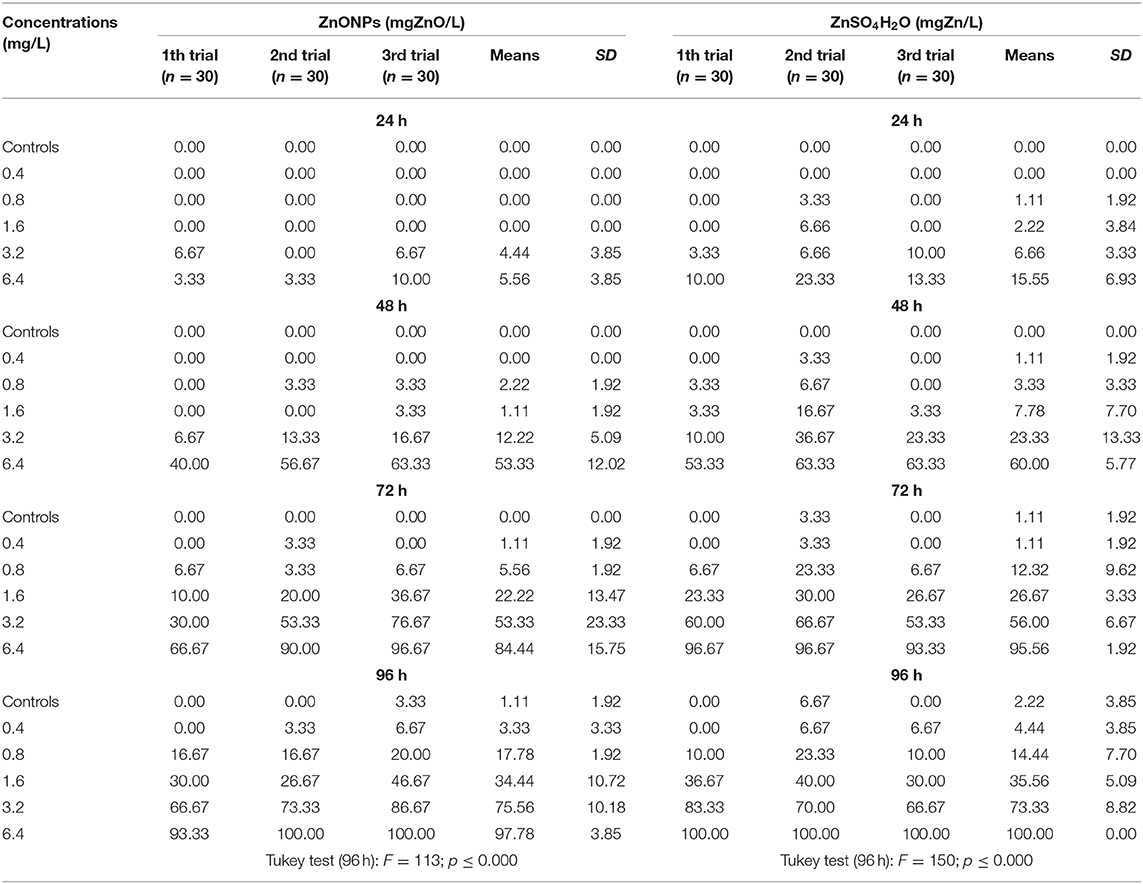
Table 5. Mortality (relative frequencies of deaths [%]) of C. insidiosum by time and different concentrations.
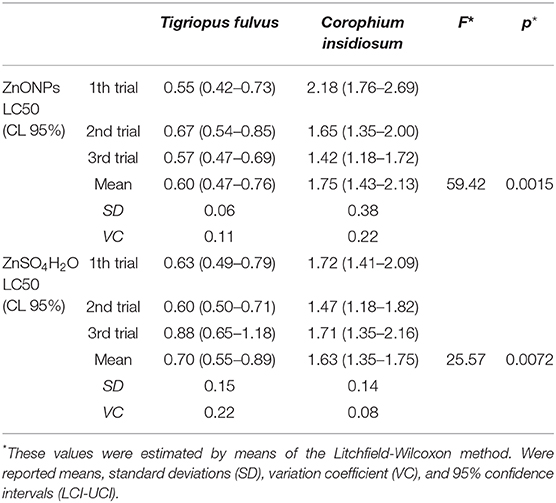
Table 6. Comparison of Lethal Concentration 50 (LC50) (mg/L) at 96 h of the two species of the studied crustaceans by different compounds.
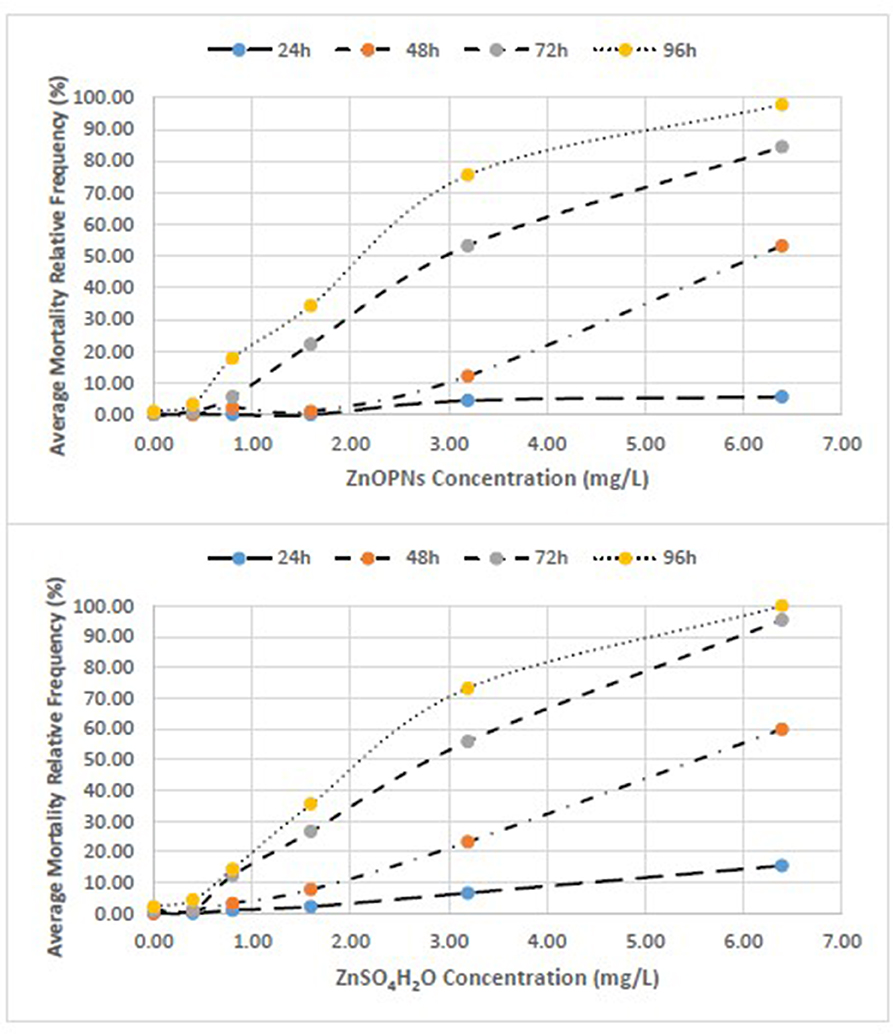
Figure 2. Concentration-effect relationship between Average Mortality Relative Frequency (%) of C. insidiosum and concentration of ZnONPs and ZnSO4H2O.
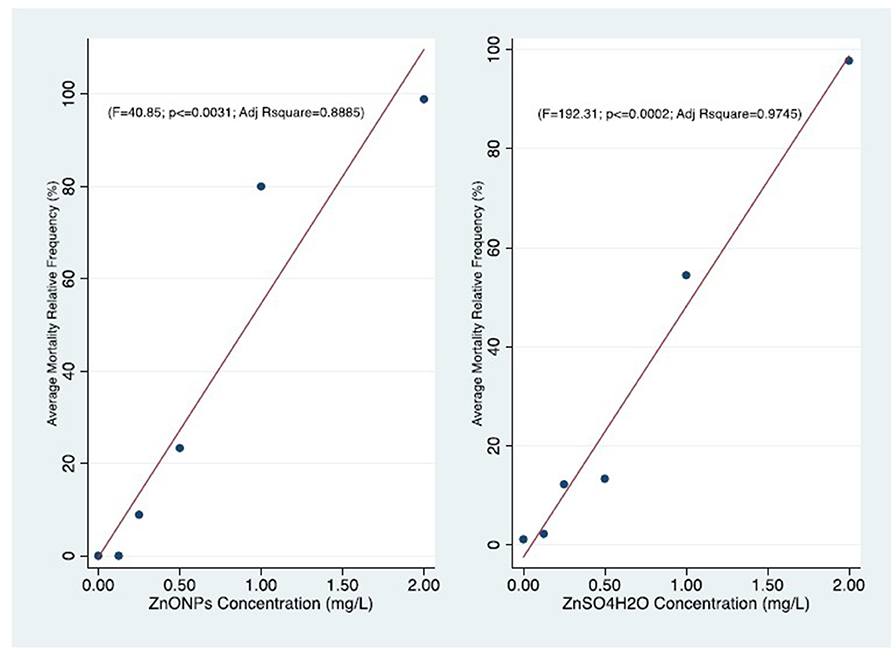
Figure 3. Comparison of concentration-effect curves of T. fulvus after 96 h of exposure to ZnONPs (r = 0.95; P ≤ 0.031) and ZnSO4H2O (r = 0.98; P ≤ 0.002).
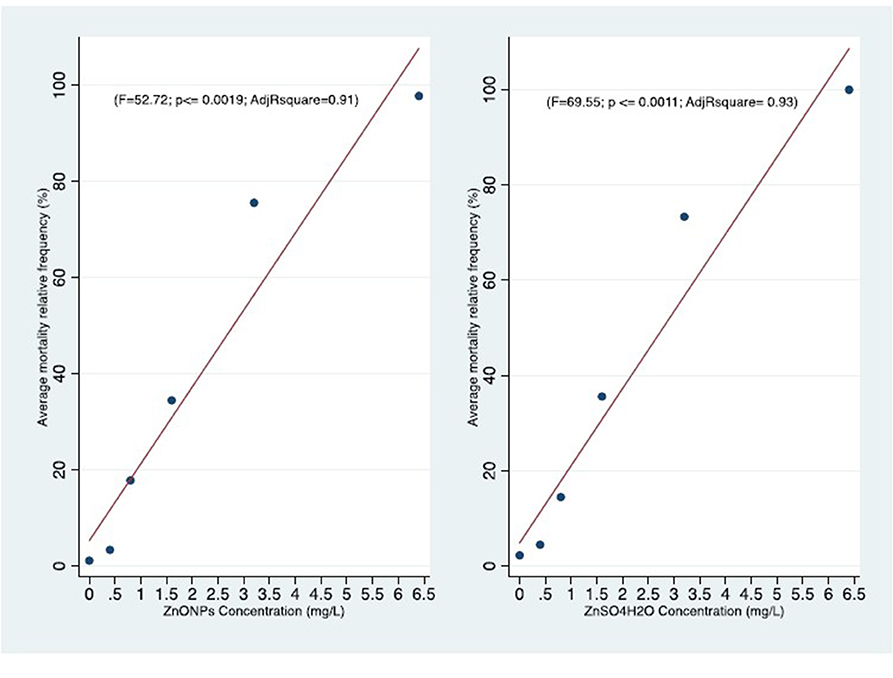
Figure 4. Comparison of concentration-effect curves of C. insidiosum after 96 h of exposure to ZnONPs (r = 0.96; P ≤ 0.019) and ZnSO4H2O (r = 0.97; P ≤ 0.0011).
The observed similar LC50 levels of soluble salts and the particles, may be due to the ZnO NPs partial dissolution so the concentrations of free Zn2+ in the ZnO NPs solutions need to be measured to understand the effects of ions dissolution at different concentrations of the particles, which is important in deciding the toxic impact (131). Zinc is an essential trace element for biological organisms, but it is also known that can cause cellular damage at high concentrations. Regarding the toxic effects of ZnO NPs, some studies attributed the toxicity to the Zn ions (Zn2+) released from the NPs into the solution. On the other hand, the findings of other studies imply that Zn2+ cannot account entirely for the toxicity of ZnO NPs. It is important to understand dissolution rate of the NP in order to quantify potential for toxicological effects following exposure. The extent of this dissolution is based on a number of factors including particle size, coating, and medium. In seawater, for example, the solubility of ZnO NPs can be more than twice that of micron-size ZnO (132). Therefore, they are unlikely to remain of nano-sized in exposures to marine organisms. They are likely to aggregate with increasing salinity, thereby reducing surface area for dissolution. In this study, the dissolved Zn concentrations of ZnO NPs and ZnSO4 suspensions test were measured according to Prato et al. (126). The nominal ZnO NPs e Zn SO4 concentrations in all treatments used did not differ significantly from that measured. It is important to understand dissolution rate of the NP in order to quantify potential for toxicological effects following exposure. The analysis of the samples highlighted that ZnO NPs showed a rapid tendency to aggregate, already in the first hours of the measurement, at the lowest test concentrations (no NPs detected at both T0 and T48h). After 48 h, aggregates slight increased their size, compared to zero time, passing from 110 ± 20 nm up to 140 ± 20 nm. The aggregates showed different sizes suggesting that solution/suspension contained nano-(micro-)sized fractions potentially able to interact with biota.
The low values of the standard deviations and the coefficient of variation of the means of all the tested species and compounds and the small value of 95% CL (Table 6) confirmed the reproducibility and therefore the acceptability of the three tests.
Acute Toxicity Test (96 h) With T. fulvus
The average relative mortality (%) of the nauplii after 24 h of exposure to the ZnO NPs ranged from 0.0 ± 0.0 at the lowest concentration of 0.125 mg/L to 27.8 ± 5.09 at the highest concentration of 2.00 mg/L. After 96 h of exposure, the mortality estimates increased, reaching a value of 98.9 ± 1.92 at 2.00 mg/L. The mortality estimates observed among the nauplii exposed to Zn sulfate (ZnSO4H2O) for 24 h were 0.0 ± 0.0 at the lowest concentration of 0.125 mg/L and 28.89 ± 7.70 at the concentration of 2.00 mg/L. After 96 h of exposure, the mortality estimates increased, reaching a value of 97.78 ± 3.85 at 2.00 mg/L (Table 4).
The concentration-effect relationship between the average of mortality relative frequency (%) of T. fulvus by different experimental times and different exposure are reported in Figure 1.
With respect to the negative control, significant effects were observed as early as 24 h at the highest concentration of 2.00 mg/L for both toxicants. At 96 h, the mortality estimates were significant, starting from 0.25 mg/L with ZnO NPs and from 0.50 mg/L with ZnSO4H2O (Supplementary Tables 1, 2).
The mortality values obtained for Zn sulfate (ZnSO4H2O) were similar to those obtained for the ZnO NPs.
With respect to the negative controls at 96 h of exposure, the observed variance of the mortality estimates of the highly exposed groups, evaluated by means of the Tukey test, was significant for ZnO NPs (F = 272.00; p ≤ 0.000) and ZnSO4H2O (F = 101.19; p ≤ 0.000). The exposed to the first compound showed a higher significant variability between groups compared with the same variability between the groups exposed to the second compound as certified by the Fisher values [F = 272; p < 0.000 vs. F = 101.19; p < 0.000; Table 4].
We observed that the mortality curves of the two different compounds had a similar trend according to the concentrations tested (Figure 3). The mean LC50 values obtained for the two forms of zinc were similar [ZnO NPs: 0.60 ± 0.06 mg/L; ZnSO4H2O: 0.70 ± 0.15 mg/L] and were not significantly different from each other (Table 6). The low values of standard deviation and coefficient of variation and the small confidence interval of LD50 for the ZnO NPs (S.D. = 0.06; CV = 0.11; and C.I. = 0.27) and ZnSO4H2O (S.D. = 0.15; CV = 0.22; and C.I. = 0.34) confirmed the reproducibility, acceptability and stability of the estimates of the three tests in the group of T. fulvus.
The Pearson r is highly significant in both the T. fulvus exposure (ZnONPs, ZnSO4H2) effects relationships. This indicate very strong correlations. The strength of those result is supported also by the linear regression significance of the Adjusted determination coefficient (R2) for same variables (Figure 3).
Acute Toxicity Test (96 h) With C. insidiosum
The average relative mortality (%) (χ ± δ) of the C. insidiosum obtained with Zn sulfate (ZnSO4H2O) was slightly higher than that obtained with the ZnO NPs. In fact, the mortality estimates of the animals exposed to the ZnO NPs for 24 h varied from 0.00 ± 0.00 at the lowest concentration of 0.4 mg/L to 5.5 ± 3.85 at the highest concentration of 6.4 mg/L; after 96 h of exposure, mortality reached 97.8 ± 3.85 at 6.4 mg/L. Among the animals exposed to Zn sulfate (ZnSO4H2O), the estimates ranged from 0 ± 0 at the lowest concentration of 0.4 mg/L to 15.55 ± 6.93 at the highest concentration of 6.4 mg/L; after 96 h of exposure, the mortality estimates increased, reaching a value of 100.00 ± 0.00 at 6.4 mg/L. At 96 h, with respect to that of the negative controls, the observed variance of the mortality estimates of the highly exposed groups, evaluated by means of the Tukey test, is significant for the ZnO NPs (F = 113; p ≤ 0.000) and ZnSO4H2O (F = 150; p ≤ 0.000; Table 5). The concentration-effect curves reported an increase in the mortality estimates with increasing zinc concentrations and increasing exposure time. The observed trends are very similar for both the ZnO NPs and ZnSO4H2O and the concentration-effect relationship between Average Mortality Relative Frequency (%) of C. insidiosum and concentrations of ZnONPs and ZnSO4H2O was reported in Figure 2.
The mortality values obtained for ZnSO4H2O were similar to those obtained for the ZnO NPs. With respect to those observed for the negative control, significant effects were observed after 24 h of exposure starting from the 3.2 mg/L concentration with both toxic substances; and after 96 h, the differences were significant starting from 0.8 mg/L (Table 5).
Statistical analysis of the mortality rates for each concentration tested, after 96 h of exposure, did not show significant differences between the two forms of zinc. In fact, we observed that the two mortality curves have a similar trend according to the tested concentrations (Figure 4). The mean LC50 values obtained for the two forms of zinc were similar [ZnO NPs: 1.75 ± 0.39 mg/L; ZnSO4H2O: 1.63 ± 0.14 mg/L] and were not significantly different from each other (Table 6). The low values of standard deviation and coefficient of variation and the small confidence interval of LC50 for the ZnO NPs (S.D. = 0.38; CV = 0.22; and C.I. = 0.70) and ZnSO4H2O (S.D. = 0.14; CV = 0.08; and C.I. = 0.40) confirmed the reproducibility, acceptability and stability of the estimates of the three tests in the group of C. insidiosum.
The Pearson r is highly significant in both the C. insidiosum exposure (ZnONPs, ZnSO4H2) effects relationships. This indicate very strong correlations. The strength of those result is supported also by the linear regression significance of the Adjusted determination coefficient (R2) for same variables (Figure 4).
Comparison Between the Two Test Species
Statistical analysis revealed that the mean LC50 for Tigriopus fulvus was significantly lower than that for Corophium insidiosum for both the ZnO NPs and ZnSO4H2O (ZnO NPs: F = 59.42; p < 0.0015; ZnSO4H2O: F = 25.57; p < 0.0015; Table 6).
Discussion: the Study on Marine Crustaceans
Nanomaterials and nanotechnologies are a thriving industrial sector destined to grow due to the increasing amount of investments it is able to attract. The increase in the production and application of NPs means that more of them are released into the marine environment, which represents the final pollutant receptor.
Despite the increased use of NMs in recent years, the volumes produced and used in different applications are not known, and the resulting emissions in the environment and their impact, fate and consequences on human health are not known. These gaps do not allow an exact assessment of the risk.
The progress made in the last decade by research in the development and application of nanomaterials has helped to define a framework of policies able to support the safe and responsible development of nanotechnologies, which has emerged as an increasingly pressing issue.
That is, the need to support the growth of the nanotechnology sector was introduced in order to maximize the benefits both in economic terms and in improving the quality of life while limiting the potential risks to health and safety, and a link between these emerging technologies and their continuous evolution was established.
For the safe and responsible development of nanotechnologies, the protection of the health and safety of the environment and of the workers involved in such processes assumes a specific relevance due to the particular conditions (levels, modalities, and times) of exposure.
The results of this study were intended to be used as ecotoxicological information, showing the toxicity of ZnO NPs that enter the food chain, on two model organisms characterized by high representativeness and diffusion in the Mediterranean area, the copepod Tigriopus fulvus and the amphypod Corophium insidiosum.
Experimentation with the two selected test species has shown that to test the toxic effects of ZnO NPs, exposure times longer than 24 h are more suitable than shorter exposure times. In fact, both tested zinc forms exhibited moderate 24 h toxicity, while the effects increased considerably with increasing exposure. The two species showed different sensitivities: Corophium insidiosum was less sensitive than Tigriopus fulvus toward both forms of zinc.
These results confirm that since the sensitivity of animal and plant communities to pollutants varies significantly from one species to another, a single experimental organism or model is not able to represent the complex variety of responses to stressors (133, 134) and that the use of a single test produces results with a high level of uncertainty.
Moreover, the results obtained have shown the existence of positive relationship between the effects of toxicity found and the concentration of NPs and between the effects of toxicity and observation times. Both species showed, when exposed to the two forms of zinc (ZnO NPs and ZnSO4), an absence of significant differences between the LC50 values, showing the same trend with respect to time and dose. This result confirms that the toxic effect could be mainly attributed to the Zn ions, confirming that the dissolution processes would a crucial role in the toxicity of the ZnO NPs (113, 126, 135, 136). Once introduced into the aquatic environment, the NPs undergo a series of processes (aggregation, sedimentation, biological degradation, and dissolution) that modify their destiny and lead to the formation of both potentially toxic aggregates and metal ions. These processes are influenced not only by multiple environmental factors, such as pH, salinity, and the presence of organic substances, but also by the structural characteristics of the particle, which include shape, size, morphological substructure of the substance (crystallinity, porosity, and surface roughness), chemical properties of the coarse material, solubility, dispersion state, area, and surface charge (115, 137–139).
Moreover, the differences in experimental procedures, particularly regarding the preparation of the NP suspension (presence/absence of solubilization vehicles, filtering, centrifugation, sonication), can produce results that are sometimes not very comparable, making the comparison complicated (132).
The results of the acute tests on T. fulvus reported in this study are comparable to those found in the literature. Wong et al. (140) reported that the LC50 value at 96 h for Tigriopus japonicus nauplii was 0.85 mg/L for the ZnO NPs and 1.14 mg/L for ZnSO4 x H2O. Park et al. (94) indicated that the LC50 value at 96 h for adult T. japonicus organisms was 2.44 mg/L.
There are no acute toxicity data on Corophium insidiosum in the literature; the only existing data on the species refer to a chronic toxicity assessment for Corophium volutator, which shows that exposure to sublethal concentrations of ZnO NPs [0.2–1.0 mg/L] delays growth and influences the reproductive outcome of the exposed populations.
Previous work related to the acute toxicity of ZnO NPs to other marine amphypod crustaceans. Wong et al. (140) reported LC50 values of 1.19 mg/L for Elasmopus rapax exposed to ZnO NPs and 0.80 mg/L for those exposed to ZnSO4H2O. Poynton (115) reported that the LC50 values at 96 h for Hyalella azteca were lower than those obtained by us (0.08 mg/L for the ZnO NPs and 0.154 mg/L for ZnSO4), placing this species as one of the most sensitive to ZnO NPs.
Similar results to those obtained in the present study were found in the acute toxicity tests of ZnO NPs for the microalgae Phaeodactylum tricornutum [EC50 = 1.09 mg/L] and Dunaliella tertiolecta [EC50 = 1.94 mg/L] and the freshwater cladocerus crustacean Daphnia magna [LC50 = 3.2 mg/L] (135, 141, 142). Several studies on the ecotoxicity of ZnO NPs suggest that different mechanisms/modalities of action may be involved. However, most of these studies reported that the dissolution of the ionic zinc NPs contributes mainly to the toxicity of the observed ZnO NPs, and this deduction is generally based on the comparable toxicity results obtained with ZnO NPs and zinc salts (85). However, the extent to which dissolved Zn2+ contributes to the toxicity of ZnO NPs and the mechanisms involved are still not clear (85). The effects highlighted could be of mechanical origin: the adhesion of NP aggregates to the exoskeleton of crustaceans can in fact have physical effects, such as the obstruction of the respiratory tract and/or the loss of mobility (143, 144). Damage could also be linked to the ingestion of NPs; in organisms that ingest particles, the accumulation of these particles can be observed in the digestive tract, as we have seen for Daphnia magna and Artemia salina (116, 143, 144). The production of ROS by visible light and the generation of oxidative stress are the main mechanisms proposed to explain the negative effects of NP toxicity (145). ROS and oxidative stress damage lipids, carbohydrates, proteins and DNA, resulting in cell death (146).
Some limits of this study concern the possible aggregation/agglomeration of ZnO particles across the concentrations applied in the medium, the effective size of the agglomerate may control biological reactivity in the system, and the size distribution of the particles will be a function of concentration, and time of exposure in the medium.
Other aspects unknown or unsolved here referee to oxidative stress generation—ROS mediated mechanism—effects on enzymatic and non-enzymatic controls on ROS, internalization and distribution of NPs such as compartmentalization, bioaccumulation and histopathological alterations (91, 113–115, 131).
Conclusion
The aim of the present work was to evaluate the acute toxicity of ZnO NPs on marine invertebrates, since at nowadays few studies on marine organisms are available. For this reason, became important to identify the Effect/Lethal Concentrations values to calculate mortality thresholds and assess potential environmental hazard. In order to allows to establish preliminary the concentrations range that can be considered toxics. These information are the fundamental importance. However, in this preliminary study, that aim only to evaluate the acute toxicity of ZnO NPs, the authors did not consider studying any mechanistic aspects of the toxic effects. It is well-known that the oxidative stress is a common pathway of toxicity induced by pollutants with the induction ROS generation and subsequent oxidative stress, which leads to damaged DNA, lipids, and proteins and potentially to cell death as reported for several organisms. Accordingly, for a full comprehension on ecotoxicity of ZnO NPs toward these marine invertebrate (still unexplored) these focus represent aims of a future study and all the limits highlighted in these preliminary results are being resolved in the study still in progress with special reference to physicochemical analyses.
More investigation, with standard experimental conditions is needed to better understand ZnO NPs toxicity to the cellular and organisms level as these NPs may enter the food chain. Environmental and human exposure due to nanomaterial residues in air, soil and crops is expected to increase with exposure routes, including possible bioaccumulation in the environment and food chain.
The possible risks, together with other unforeseen events for human health and environmental degradation, cannot be set aside or underestimated. As a consequence, effectively evaluating the specific or generalized risks associated with the various types of NPs using a precautionary approach (“no data, no market”) is necessary.
In particular, much effort should be made to develop innovative, green, and sustainable solutions (nano), which have eco-sustainability features such as limited environmental diffusion and no toxic effects on man and nature. To this end, the following measures are necessary: to support research and innovation for the identification of nanoeco-sustainable solutions; and to define a standardized methodology to evaluate the effectiveness, eco-safety and economic sustainability of NMs.
Data Availability Statement
The datasets generated for this study are available on request to the corresponding author.
Author Contributions
GF, EP, LV, and DC: conceptualization. EP and MT: investigation. EP, DC, MT, and AC: data curation. GF and EP: formal analysis and methodology. LV: supervision. GF, DC, and LV: writing—original draft. GF, DC, LV, AC, and LD: writing—review and editing.
Conflict of Interest
The authors declare that the research was conducted in the absence of any commercial or financial relationships that could be construed as a potential conflict of interest.
Supplementary Material
The Supplementary Material for this article can be found online at: https://www.frontiersin.org/articles/10.3389/fpubh.2020.00192/full#supplementary-material
References
1. Leso V, Iavicoli I. Palladium nanoparticles: toxicological effects and potential implications for occupational risk assessment. Int J Mol Sci. (2018) 19:503. doi: 10.3390/ijms19020503
2. Dang Y, Zhang Y, Fan L, Chen H, Roco MC. Trends in worldwide nanotechnology patent applications: 1991 to 2008. J Nanopart Res. (2010) 12:687–706. doi: 10.1007/s11051-009-9831-7
3. Roco MC, Mirkin CA, Hersam MC. WTEC Panel Report on Nanotechnology Research Directions for Societal Needs in 2020: Retrospective and Outlook. Baltimore, MD: World Technology Evaluation Center, Inc. (2010). doi: 10.1007/978-94-007-1168-6
4. Roco MC. The long view of nanotechnology development: the national nanotechnology initiative at 10 years. J Nanopart Res. (2011) 13:427-45 doi: 10.1007/s11051-010-0192-z
5. Boccuni F, Gagliardi D, Ferrante R, Rondinone BM, Iavicoli S. Measurement techniques of exposure to nanomaterials in the workplace for low- and medium-income countries: a systematic review. Int J Hyg Environ Health. (2017) 220:1089–97. doi: 10.1016/j.ijheh.2017.06.003
6. Iavicoli I, Leso V, Beezhold DH, Shvedova AA. Nanotechnology in agriculture: opportunities, toxicological implications, and occupational risks. Toxicol Appl Pharmacol. (2017) 329:96–111. doi: 10.1016/j.taap.2017.05.025
7. Schulte PA, Kuempel ED, Drew NM. Characterizing risk assessments for the development of occupational exposure limits for engineered nanomaterials. Regul Toxicol Pharmacol. (2018) 95:207–19. doi: 10.1016/j.yrtph.2018.03.018
8. Schulte P, Leso V, Niang M, Iavicoli I. Biological monitoring of workers exposed to engineered nanomaterials. Toxicol Lett. (2018) 298:112–24. doi: 10.1016/j.toxlet.2018.06.003
9. Leso V, Fontana L, Iavicoli I. The occupational health and safety dimension of Industry 4.0. Med Lav. (2018) 110:327–38. doi: 10.23749/mdl.v110i5.7282
10. Oomen AG, Steinhäuser KG, Bleeker EAJ, van Broekhuizen F, Sips A, Dekkers S, et al. Risk assessment frameworks for nanomaterials: scope, link to regulations, applicability, and outline for future directions in view of needed increase in efficiency. NanoImpact. (2018) 9:1–13. doi: 10.1016/j.impact.2017.09.001
11. Jain A, Ranjan S, Dasgupta N, Ramalingam C. Nanomaterials in food and agriculture: an overview on their safety concerns and regulatory issues. Crit Rev Food Sci Nutr. (2018) 58:297–317. doi: 10.1080/10408398.2016.1160363
12. Savolainen K, Alenius H, Norppa H, Pylkkänen L, Tuomi T, Kasper G. Risk assessment of engineered nanomaterials and nanotechnologies: a review. Toxicology. (2010) 269:92–104. doi: 10.1016/j.tox.2010.01.013
13. European Commission. (2011/696/EU) Commission Recommendation on the Definition of Nanomaterial. OJ L 275/38 (2011).
14. ISO/TS 27687 (2008). Nanotechnologies- Terminology and Definitions for Nano-Objects-Nanoparticle, Nanofbre and Nanoplate. International Organization for Standardization (2008). Available online at: http://www.iso.org/iso/catalogue_detail?csnumber=44278 (accessed March 26, 2020).
15. Sayre PG, Steinhauser KG, van Teunenbroek T. Methods and data for regulatory risk assessment of nanomaterials: questions for an expert consultation. Nanoimpact. (2017) 8:20–7. doi: 10.1016/j.impact.2017.07.001
16. Schmalz G, Hickel R, van Landuyt KL, Reichl FX. Nanoparticles in dentistry. Dent Mater. (2017) 33:1298–314. doi: 10.1016/j.dental.2017.08.193
17. Schulte PA, Leso V, Niang M, Iavicoli I. Current state of knowledge on the health effects of engineered nanomaterials in workers: a systematic review of human studies and epidemiological investigations. Scand J Work Environ Health. (2019) 45:217–38. doi: 10.5271/sjweh.3800
18. Debia M, Bakhiyi B, Ostiguy C, Verbeek JH, Brouwer DH, Murashov V. A systematic review of reported exposure to engineered nanomaterials. Ann Occup Hyg. (2016) 60:916–35. doi: 10.1093/annhyg/mew041
19. Nanowerk. Nanomaterials Database. (2015). Available online at: http://www.nanowerk.com/nanomaterial-database.php (accessed March 26, 2020).
20. Vance ME, Kuiken T, Vejerano EP, McGinnis SP, Hochella MF Jr, Rejeski D, et al. Nanotechnology in the real world: redeveloping the nanomaterial consumer products inventory. Beilstein J Nanotechnol. (2015) 6:1769–80. doi: 10.3762/bjnano.6.181
21. EEC (European Economic Community). Types and uses of nanomaterials, including safety aspects. Commission Staff Working Paper accompanying the Communication from the Commission to the European Parliament, the Council and the European Economic and Social Committee on the Second Regulatory Review on Nanomaterials. The European Commission (2012). Available online at: https://ec.europa.eu/health//sites/health/files/nanotechnology/docs/swd_2012_288_en.pdf (accessed March 26, 2020).
22. WHO (World Health Organization). WHO Guidelines on Protecting Workers from Potential Risks of Manufactured Nanomaterials. Geneva: World Health Organization (2017).
23. Iavicoli I, Fontana L, Pingue P, Todea AM, Asbach C. Assessment of occupational exposure to engineered nanomaterials in research laboratories using personal monitors. Sci Total Environ. (2018) 627:689–702. doi: 10.1016/j.scitotenv.2018.01.260
24. Pietroiusti A, Magrini A. Engineered nanoparticles at the workplace: current knowledge about workers' risk. Occup Med. (2014) 64:319–30. doi: 10.1093/occmed/kqu051
25. Hristozov D, Gottardo S, Semenzin E, Oomen A, Bos P, Peijnenburg W, et al. Frameworks and tools for risk assessment of manufactured nanomaterials. Environ Int. (2016) 95:36–53. doi: 10.1016/j.envint.2016.07.016
26. Schmalz G, Hickel R, van Landuyt KL, Reichl FX. Scientific update on nanoparticles in dentistry. Int Dent J. (2018) 68:299–305. doi: 10.1111/idj.12394
27. Asbach C, Alexander C, Clavaguera S, Dahmann D, Dozol H, Faure B, et al. Review of measurement techniques and methods for assessing personal exposure to airborne nanomaterials in workplaces. Sci Total Environ. (2017) 603-604:793–806. doi: 10.1016/j.scitotenv.2017.03.049
28. Runa S, Hussey M, Payne CK. Nanoparticle-cell interactions: relevance for public health. J Phys Chem B. (2018) 122:1009–16. doi: 10.1021/acs.jpcb.7b08650
29. Rico CM, Majumdar S, Duarte-Gardea M, Peralta-Videa JR, Gardea-Torresdey JL. Interaction of nanoparticles with edible plants and their possible implications in the food chain. J Agric Food Chem. (2011) 59:3485–98. doi: 10.1021/jf104517j
30. Steinhauser KG, Sayre PG. Reliability of methods and data for regulatory assessment of nanomaterial risks. Nanoimpact. (2017) 7:66–74. doi: 10.1016/j.impact.2017.06.001
31. Schimpel C, Resch S, Flament G, Carlander D, Vaquero C, Bustero I, et al. A methodology on how to create a real-life relevant risk profile for a given nanomaterial. J Chem Health Saf. (2018) 25:12–23. doi: 10.1016/j.jchas.2017.06.002
32. Kuhlbusch TAJ, Wijnhoven SWP, Haase A. Nanomaterials exposure for workers, consumer and the general public. Nanoimpact. (2018) 19:11–25. doi: 10.1016/j.impact.2017.11.003
33. Leso V, Fontana L, Iavicoli I. Nanomaterial exposure and sterile inflammatory reactions. Toxicol Appl Pharmacol. (2018) 355:80–92. doi: 10.1016/j.taap.2018.06.021
34. Rasmussen K, González M, Kearns P, Sintes JR, Rossi F, Sayre P. Review of achievements of the OECD working party on manufactured nanomaterials' testing and assessment programme. From exploratory testing to test guidelines. Regul Toxicol Pharmacol. (2016) 74:147–60. doi: 10.1016/j.yrtph.2015.11.004
35. Liu J, Kang Y, Yin S, Song B, Wei L, Chen L, et al. Zinc oxide nanoparticles induce toxic responses in human neuroblastoma SHSY5Y cells in a size-dependent manner. Int J Nanomed. (2017) 12:8085–99. doi: 10.2147/IJN.S149070
36. Boccuni F, Ferrante R, Tombolini F, Lega D, Antonini A, Alvino A, et al. Workers' exposure to nano-objects with different dimensionalities in R&D laboratories: measurement strategy and field studies. Int J Mol Sci. (2018) 19:E349. doi: 10.3390/ijms19020349
37. Gao X, Lowry GV. Progress towards standardized and validated characterizations for measuring physicochemical properties of manufactured nanomaterials relevant to nano health and safety risks. Nanoimpact. (2018) 9:14–30. doi: 10.1016/j.impact.2017.09.002
38. Monopoli MP, Aberg C, Salvati A, Dawson KA. Biomolecular coronas provide the biological identity of nanosized materials. Nat Nanotechnol. (2012) 7:779–86. doi: 10.1038/nnano.2012.207
39. Oberdörster G, Maynard A, Donaldson K, Castranova V, Fitzpatrick J, Ausman K, et al. Principles for characterizing the potential human health effects from exposure to nanomaterials: elements of a screening strategy. Part Fibre Toxicol. (2015) 2:8. doi: 10.1186/1743-8977-2-8
40. Hubbs AF, Sargent LM, Porter DW, Sager TM, Chen BT, Frazer DG, et al. Nanotechnology: toxicologic pathology. Toxicol Pathol. (2013) 41:395–409. doi: 10.1177/0192623312467403
41. Dekkers S, Oomen AG, Bleeker EA, Vandebriel RJ, Micheletti C, Cabellos J, et al. Towards a nanospecific approach for risk assessment. Regul Toxicol Pharmacol. (2016) 80:46–59. doi: 10.1016/j.yrtph.2016.05.037
42. Landvik NE, Skaug V, Mohr B, Verbeek J, Zienolddiny S. Criteria for grouping of manufactured nanomaterials to facilitate hazard and risk assessment, a systematic review of expert opinions. Regul Toxicol Pharmacol. (2018) 95:270–9. doi: 10.1016/j.yrtph.2018.03.027
43. Rycroft T, Larkin S, Ganin A, Thomas T, Matheson J, van Grack T, et al. A framework and pilot tool for the risk-based prioritization and grouping of nano-enabled consumer products. Environ Sci Nano. (2018) 6:356–65. doi: 10.1039/C8EN00848E
44. Kisin ER, Murray AR, Keane MJ, Shi XC, Schwegler-Berry D, Gorelik O, et al. Single-walled carbon nanotubes: geno- and cytotoxic effects in lung fibroblast V79 cells. J Toxicol Environ Health A. (2007) 70:2071–9. doi: 10.1080/15287390701601251
45. Ursini CL, Cavallo D, Fresegna AM, Ciervo A, Maiello R, Buresti G, et al. Comparative cyto-genotoxicity assessment of functionalized and pristine multiwalled carbon nanotubes on human lung epithelial cells. Toxicol In Vitro. (2012) 26:831–40. doi: 10.1016/j.tiv.2012.05.001
46. IARC. Monographs on the Evaluation of Carcinogenic Risks to Humans, 2017, Some Nanomaterials and Some Fibres. Vol. 111. World Health Organization (2017). Available online at: https://monographs.iarc.fr/wp-content/uploads/2018/06/mono111.pdf (accessed March 26, 2020).
47. Ferreira AJ, Cemlyn-Jones J, Robalo Cordeiro C. Nanoparticles, nanotechnology and pulmonary nanotoxicology. Rev Port Pneumol. (2013) 19:28–37. doi: 10.1016/j.rppnen.2013.01.004
48. Iavicoli I, Leso V, Fontana L, Calabrese EJ. Nanoparticle exposure and hormetic dose-responses: an update. Int J Mol Sci. (2018) 19:E805. doi: 10.3390/ijms19030805
49. Gordon SC, Butala JH, Carter JM, Elder A, Gordon T, Gray G, et al. Workshop report: strategies for setting occupational exposure limits for engineered nanomaterials. Regul Toxicol Pharmacol. (2014) 68:305–11. doi: 10.1016/j.yrtph.2014.01.005
50. Pietroiusti A, Campagnolo L, Fadeel B. Interactions of engineered nanoparticles with organs protected by internal biological barriers. Small. (2013) 9:1557–72. doi: 10.1002/smll.201201463
51. Khatri M, Bello D, Martin J, Bello An, Gore R, Demokritou P., et al. Chronic upper airway inflammation and systemic oxidative stress from nanoparticles in photocopier operators: mechanistic insights. NanoImpact. (2017) 5:133–45. doi: 10.1016/j.impact.2017.01.007
52. Glass DC, Mazhar M, Xiang S, Dean P, Simpson P, Priestly B, et al. Immunological effects among workers who handle engineered nanoparticles. Occup Environ Med. (2017) 74:868–76. doi: 10.1136/oemed-2016-104111
53. Dhawan A, Shanker R, Das M, Gupta KC. Guidance for safe handling of nanomaterials. J Biomed Nanotechnol. (2011) 7:218–24. doi: 10.1166/jbn.2011.1276
54. Arts JH, Hadi M, Irfan MA, Keene AM, Kreiling R, Lyon D, et al. A decision-making framework for the grouping and testing of nanomaterials (DF4nanoGrouping). Regul Toxicol Pharmacol. (2015) 71(Suppl. 2):S1–27. doi: 10.1016/j.yrtph.2015.03.007
55. EFSA Scientific Committee. (2011). Scientific opinion on guidance on the risk assessment of the application of nanoscience and nanotechnologies in the food and feed chain. EFSA J. (2011) 9:2140. doi: 10.2903/j.efsa.2011.2140
56. Nowack B, Bucheli TD. Occurrence, behavior and effects of nanoparticles in the environment. Environ Pollut. (2007) 150:5–22. doi: 10.1016/j.envpol.2007.06.006
57. Tian L, Shang Y, Chen R, Bai R, Chen C, Inthavong K, et al. A combined experimental and numerical study on upper airway dosimetry of inhaled nanoparticles from an electrical discharge machine shop. Part Fibre Toxicol. (2017) 14:24. doi: 10.1186/s12989-017-0203-7
58. Martirosyan A, Schneider YJ. Engineered nanomaterials in food: implications for food safety and consumer health. Int J Environ Res Public Health. (2014) 11:5720–50. doi: 10.3390/ijerph110605720
59. Pietroiusti A, Bergamaschi E, Campagna M, Campagnolo L, de Palma G, Iavicoli S, et al. The unrecognized occupational relevance of the interaction between engineered nanomaterials and the gastro-intestinal tract: a consensus paper from a multidisciplinary working group. Part Fibre Toxicol. (2017) 14:47. doi: 10.1186/s12989-017-0226-0
60. Vimercati L, Baldassarre A, Gatti MF, Gagliardi T, Serinelli M, De Maria L, et al. Non-occupational exposure to heavy metals of the residents of an industrial area and biomonitoring. Environ Monit Assess. (2016) 188:673. doi: 10.1007/s10661-016-5693-5
61. Vimercati L, Cavone D, Lovreglio P, de Maria L, Caputi A, Ferri GM, et al. Environmental asbestos exposure and mesothelioma cases in Bari Apulia region southern Italy a national interest site for land reclamation. Environ Sci Pollut Res. (2018) 25:15692–701. doi: 10.1007/s11356-018-1618-x
62. Mustafa G, Komatsu S. Toxicity of heavy metals and metal-containing nanoparticles on plants. Biochim Biophys Acta. (2016) 1864:932–44. doi: 10.1016/j.bbapap.2016.02.020
63. Gifford M, Hristovski K, Westerhoff P. Ranking traditional and nano-enabled sorbents for simultaneous removal of arsenic and chromium from simulated groundwater. Sci Total Environ. (2017) 601–602:1008–14. doi: 10.1016/j.scitotenv.2017.05.126
64. Vimercati L, Gatti MF, Baldassarre A, Nettis E, Favia N, Palma M, et al. Occupational exposure to Urban air pollution and allergic diseases. Int J Environ Res Public Health. (2015) 12:12977–87. doi: 10.3390/ijerph121012977
65. Davis DA, Bortolato M, Godar SC, Sander TK, Iwata N, Pakbin P, et al. Prenatal exposure to Urban air nanoparticles in mice causes altered neuronal differentiation and depression-like responses. PLoS ONE. (2013) 8:e64128. doi: 10.1371/journal.pone.0064128
66. Campo L, Vimercati L, Carrus A, Bisceglia L, Pesatori AC, Bertazzi PA, et al. Environmental and biological monitoring of PAHs exposure in coke-oven workers at the Taranto plant compared to two groups from the general population of Apulia, Italy. Med Lav. (2012) 103:347–60.
67. Sakulthaew C, Comfort SD, Chokejaroenrat C, Li X, Harris CE. Removing PAHs from urban runoff water by combining ozonation and carbon nano-onions. Chemosphere. (2015) 141:265–73. doi: 10.1016/j.chemosphere.2015.08.002
68. Topuz F, Uyar T. Cyclodextrin-functionalized mesostructured silica nanoparticles for removal of polycyclic aromatic hydrocarbons. J Colloid Interf Sci. (2017) 497:233–41. doi: 10.1016/j.jcis.2017.03.015
69. Celebioglu A, Topuz F, Yildiz ZI, Uyar T. Efficient removal of polycyclic aromatic hydrocarbons and heavy metals from water by electrospun nanofibrous polycyclodextrin membranes. ACS Omega. (2019) 4:7850–60. doi: 10.1021/acsomega.9b00279
70. Zambrano-Zaragoza ML, González-Reza R, Mendoza-Muñoz N, Miranda-Linares V, Bernal-Couoh TF, Mendoza-Elvira S, et al. Nanosystems in edible coatings: a novel strategy for food preservation. Int J Mol Sci. (2018) 19:E705. doi: 10.3390/ijms19030705
71. Vimercati L, Carrus A, Bisceglia L, Tatò I, Bellotta MR, Russo A, et al. Biological monitoring and allergic sensitization in traffic police officers exposed to urban air pollution. Int J Immunopathol Pharmacol. (2006) 19(Suppl. 4):57–60.
72. Vimercati L, Carrus A, Martino T, Galise I, Minunni V, Caputo F, et al. Formaldehyde exposure and irritative effects on medical examiners, pathologic anatomy post-graduate students and technicians. Iran J Public Health. (2010) 39:26–34.
73. Vimercati L. Traffic related air pollution and respiratory morbidity. Lung India. (2011) 28:238. doi: 10.4103/0970-2113.85682
74. Jayaratne ER, Ling X, Morawska L. Comparison of charged nanoparticle concentrations near busy roads and overhead high-voltage power lines. Sci Total Environ. (2015) 526:14–8. doi: 10.1016/j.scitotenv.2015.04.074
75. Mihalache R, Verbeek J, Graczyk H, Murashov V, van Broekhuizen P. Occupational exposure limits for manufactured nanomaterials, a systematic review. Nanotoxicology. (2017) 11:7–19. doi: 10.1080/17435390.2016.1262920
76. Quarato M, De Maria L, Gatti MF, Caputi A, Mansi F, Lorusso P, et al. Air pollution and public health: a PRISMA-compliant systematic review. Atmosphere. (2017) 8:183. doi: 10.3390/atmos8100183
77. Förster H, Thajudeen T, Funk C, Peukert W. Separation of nanoparticles: filtration and scavenging from waste incineration plants. Waste Manag. (2016) 52:346–52. doi: 10.1016/j.wasman.2016.03.050
78. Fransman W, Buist H, Kuijpers E, Walser T, Meyer D, Zondervan-van den Beuken E, et al. Comparative human health impact assessment of engineered nanomaterials in the framework of life cycle assessment. Risk Anal. (2017) 37:1358–74. doi: 10.1111/risa.12703
79. Bakand S, Hayes A, Dechsakulthorn F. Nanoparticles: a review of particle toxicology following inhalation exposure. Inhal Toxicol. (2012) 24:125–35. doi: 10.3109/08958378.2010.642021
80. ECHA. Guidance on Information Requirements and Chemical Safety Assessment, Chapter R.15: Consumer Exposure Assessment. (2016). Available online at: https://echa.europa.eu/documents/10162/13632/information_requirements_r15_en.pdf (accessed March 26, 2020).
81. Liguori B, Foss Hansen S, Baun A, Alstrup Jensen K. Control banding tooòs for occupational exposure assessment of nanomaterials- ready for use in a regulatory context? Nanoimpact. (2016) 2:1–17. doi: 10.1016/j.impact.2016.04.002
82. Rasmussen K, Rauscher H, Mech A, Riego Sintes J, Gilliland D, González M, et al. Physico-chemical properties of manufactured nanomaterials - characterisation and relevant methods. An outlook based on the OECD testing programme. Regul Toxicol Pharmacol. (2018) 92:8–28. doi: 10.1016/j.yrtph.2017.10.019
83. Wiesenthal A, Hunter L, Wang S, Wickliffe J, Wilkerson M. Nanoparticles: small and mighty. Int J Dermatol. (2011) 50:247–54. doi: 10.1111/j.1365-4632.2010.04815.x
84. Piccinno F, Gottschalk F, Seeger S, Nowack B. Industrial production quantities and uses of ten engineered nanomaterials for Europe and the world. J Nanopart Res. (2012) 14:1109e20. doi: 10.1007/s11051-012-1109-9
85. Ma H, Williams PL, Diamond SA. Ecotoxicity of manufactured ZnO nanoparticles: a review. Environ Pollut. (2013) 172:76–85. doi: 10.1016/j.envpol.2012.08.011
86. Hoffmann MR, Martin ST, Choi W, Bahnemann DW. Environmental applications of semiconductor photocatalysis. Chem Rev. (1995) 95:69–96. doi: 10.1021/cr00033a004
87. Siddiqi KS, Ur Rahman A, Tajuddin Husen A. Properties of zinc oxide nanoparticles and their activity against microbes. Nanoscale Res Lett. (2018) 13:141. doi: 10.1186/s11671-018-2532-3
88. Jiang J, Pi J, Cai J. The advancing of zinc oxide nanoparticles for biomedical applications. Bioinorg Chem Appl. (2018) 2018:1062562. doi: 10.1155/2018/1062562
89. Coll C, Notter D, Gottschalk F, Sun T, Som C, Nowack B. Probabilistic environmental risk assessment of five nanomaterials (nano-TiO2, nano-Ag, nano-ZnO, CNT, and fullerenes). Nanotoxicology. (2016) 10:436–44. doi: 10.3109/17435390.2015.1073812
90. Kim KB, Kim YW, Lim SK, Roh TH, Bang DY, Choi SM, et al. Risk assessment of zinc oxide, a cosmetic ingredient used as a UV filter of sunscreens. J Toxicol Environ Health B Crit Rev. (2017) 20:155–82. doi: 10.1080/10937404.2017.1290516
91. Hou J, Wu Y, Li X, Wei B, Li S, Wang X. Toxic effects of different types of zinc oxide nanoparticles on algae, plants, invertebrates, vertebrates and microorganisms. Chemosphere. (2018) 193:852–60. doi: 10.1016/j.chemosphere.2017.11.077
92. Liou SH, Wu WT, Liao HY, Chen CY, Tsai CY, Jung WT, et al. Global DNA methylation and oxidative stress biomarkers in workers exposed to metal oxide nanoparticles. J Hazard Mater. (2017) 331:329–35. doi: 10.1016/j.jhazmat.2017.02.042
93. de Berardis B, Civitelli G, Condello M, Lista P, Pozzi R, Arancia G, et al. Exposure to ZnO nanoparticles induces oxidative stress and cytotoxicity in human colon carcinoma cells. Toxicol Appl Pharmacol. (2010) 246:116–27. doi: 10.1016/j.taap.2010.04.012
94. Park J, Kim S, Yoo J, Lee JS, Park JW, Jung J. Effect of salinity on acute copper and zinc toxicity to Tigriopus japonicus: the difference between metal ions and nanoparticles. Mar Pollut Bull. (2014) 85:526–31. doi: 10.1016/j.marpolbul.2014.04.038
95. Chuang HC, Juan HT, Chang CN, Yan YH, Yuan TH, Wang JS, et al. Cardiopulmonary toxicity of pulmonary exposure to occupationally relevant zinc oxide nanoparticles. Nanotoxicology. (2014) 8:593–604. doi: 10.3109/17435390.2013.809809
96. Cooper MR, West GH, Burrelli LG, Dresser D, Griffin KN, Segrave AM, et al. Inhalation exposure during spray application and subsequent sanding of a wood sealant containing zinc oxide nanoparticles. J Occup Environ Hyg. (2017) 14:510–22. doi: 10.1080/15459624.2017.1296237
97. Monsé C, Hagemeyer O, Raulf M, Jettkant B, van Kampen V, Kendzia B, et al. Concentration-dependent systemic response after inhalation of nano-sized zinc oxide particles in human volunteers. Part Fibre Toxicol. (2018) 15:8. doi: 10.1186/s12989-018-0246-4
98. Gordon T, Chen LC, Fine JM, Schlesinger RB, Su WY, Kimmel TA, et al. Pulmonary effects of inhaled zinc oxide in human subjects, guinea pigs, rats, and rabbits. Am Ind Hyg Assoc J. (1992) 53:503–9. doi: 10.1080/15298669291360030
99. Fine JM, Gordon T, Chen LC, Kinney P, Falcone G, Beckett WS. Metal fume fever: characterization of clinical and plasma IL-6 responses in controlled human exposures to zinc oxide fume at and below the threshold limit value. J Occup Environ Med. (1997) 39:722–6. doi: 10.1097/00043764-199708000-00006
100. Blanc P, Wong H, Bernstein MS, Boushey HA. An experimental human model of metal fume fever. Ann Intern Med. (1991) 114:930–6. doi: 10.7326/0003-4819-114-11-930
101. Blanc PD, Boushey HA, Wong H, Wintermeyer SF, Bernstein MS. Cytokines in metal fume fever. Am Rev Respir Dis. (1993) 147:134–8. doi: 10.1164/ajrccm/147.1.134
102. Hartmann L, Bauer M, Bertram J, Gube M, Lenz K, Reisgen U, et al. Assessment of the biological effects of welding fumes emitted from metal inert gas welding processes of aluminium and zinc-plated materials in humans. Int J Hyg Environ Health. (2014) 217:160–8. doi: 10.1016/j.ijheh.2013.04.008
103. Baumann R, Joraslafsky S, Markert A, Rack I, Davatgarbenam S, Kossack V, et al. IL-6, a central acute-phase mediator, as an early biomarker for exposure to zinc-based metal fumes. Toxicology. (2016) 373:63–73. doi: 10.1016/j.tox.2016.11.001
104. Zhang WD, Zhao Y, Zhang HF, Wang SK, Hao ZH, Liu J, et al. Alteration of gene expression by zinc oxide nanoparticles or zinc sulfate in vivo and comparison with in vitro data: a harmonious case. Theriogenology. (2016) 86:850–61.e1. doi: 10.1016/j.theriogenology.2016.03.006
105. Król A, Pomastowski P, Rafinska K, Railean-Plugaru V, Buszewski B. Zinc oxide nanoparticles: synthesis, antiseptic activity and toxicity mechanism. Adv Colloid Interf Sci. (2017) 249:37–52. doi: 10.1016/j.cis.2017.07.033
106. Nohynek GJ, Dufour EK, Roberts MS. Nanotechnology, cosmetics and the skin: is there a health risk? Skin Pharmacol Physiol. (2008) 21:136–49. doi: 10.1159/000131078
107. Nohynek GJ, Dufour EK. Nano-sized cosmetic formulations or solid nanoparticles in sunscreens: a risk to human health? Arch Toxicol. (2012) 86:1063–75. doi: 10.1007/s00204-012-0831-5
108. Leung YH, Xu X, Ma AP, Liu F, Ng AM, Shen Z, et al. Toxicity of ZnO and TiO(2) to Escherichia coli cells. Sci Rep. (2016) 6:35243. doi: 10.1038/srep35243
109. Lee SH, Tang CH, Lin WY, Chen KH, Liang HJ, Cheng TJ, et al. LC-MS-based lipidomics to examine acute rat pulmonary responses after nano- and fine-sized ZnO particle inhalation exposure. Nanotoxicology. (2018) 12:439–52. doi: 10.1080/17435390.2018.1458918
110. Kong T, Zhang SH, Zhang JL, Hao XQ, Yang F, Zhang C, et al. Acute and cumulative effects of unmodified 50-nm nano-ZnO on mice. Biol Trace Elem Res. (2018) 185:124–34. doi: 10.1007/s12011-017-1233-6
111. Chien CC, Yan YH, Juan HT, Cheng TJ, Liao JB, Lee HP, et al. Sustained renal inflammation following 2 weeks of inhalation of occupationally relevant levels of zinc oxide nanoparticles in Sprague Dawley rats. J Toxicol Pathol. (2017) 30:307–14. doi: 10.1293/tox.2017-0025
112. Minetto D, Volpi Ghirardini A, Libralato G. Saltwater ecotoxicology of Ag, Au, CuO, TiO2, ZnO and C60 engineered nanoparticles: an overview. Environ Int. (2016) 92-93:189–201. doi: 10.1016/j.envint.2016.03.041
113. Khosravi-Katuli K, Lofrano G, Pak Nezhad H, Giorgio A, Guida M, Aliberti F, et al. Effects of ZnO nanoparticles in the Caspian roach (Rutilus rutilus caspicus). Sci Total Environ. (2018) 626:30–41. doi: 10.1016/j.scitotenv.2018.01.085
114. Sarkheil M, Johari SA, An HJ, Asghari S, Park HS, Sohn EK, et al. Acute toxicity, uptake, and elimination of zinc oxide nanoparticles (ZnO NPs) using saltwater microcrustacean, Artemia franciscana. Environ Toxicol Pharmacol. (2018) 57:181–8. doi: 10.1016/j.etap.2017.12.018
115. Poynton HC, Chen C, Alexander SL, Majior KM, Blalock BJ, Unrine JM. Enhanced toxicity of environmentally transformed ZnO nanoparticles relative to Zn ions in the epibenthic amphipod Hyalella Azteca. Environ Sci Nano. (2019) 6:325–40. doi: 10.1039/C8EN00755A
116. Schiavo S, Oliviero M, Li J, Manzo S. Testing ZnO nanoparticles ecotoxicity: linking time variable exposure to effects on different marine model organisms. Environ Sci Pollut Res. (2018) 25:4871–80. doi: 10.1007/s11356-017-0815-3
117. Ye N, Wang Z, Wang S, Peijnenburg WJGM. Toxicity of mixtures of zinc oxide and graphene oxide nanoparticles to aquatic organisms of different trophic level: particles outperform dissolved ions. Nanotoxicology. (2018) 12:423–38. doi: 10.1080/17435390.2018.1458342
118. Pane L, Agrone C, Giacco E. Utilization of marine crustaceans as study models: a new approach in marine ecotoxicology for European (REACH) regulation. Ecotoxicology. (2012) 5:91–106. doi: 10.5772/28513
119. Todaro MA, Faraponova O, Onorati F, Pellegrini D, Tongiorgi P. Tigriopus fulvus (Copepoda, Harpacticoida) una possibile specie-target nella valutazione della tossicità dei fanghi portuali: ciclo vitale e prove tossicologiche preliminari. Biol Mar Medit. (2001) 8:869–72.
120. UNICHIM 2396:2014. Water Quality – Determination of the lethal toxicity at 24h, 48h and 96h exposure to the nauplii of Tigriopus fulvus (Fischer, 1860) (Crostacea: Copepoda). Milan (2014).
121. Pane L, Giacco E, Mariottini GL. Utilizzo di Tigriopus fulvus (Copepoda: Harpacticoi- da) in ecotossicologia. Saggi con disperdenti e tensioattivi. Biol Mar Medit. (2006) 13:348–9.
122. Pane L, Mariottini GL, Lodi A, Giacco E. Effects of heavy metals on laboratory reared Tigriopus fulvus Fischer (Copepoda: Harpacticoida). In: Brown SE, Welton WC, editors. Heavy Metal Pollution. Hauppauge, NY: NOVA Science Publ. Inc. (2008) p. 157–65.
123. OECD. Guideline for Testing of Chemicals, No. 202 (13th April 2004) Daphnia sp., Acute Immobilization Test. Paris (2004).
124. ISO. Water Quality— Determination of Acute Toxicity of Marine or Estuarine Sediment to Amphipods. International Organisation for Standardisation, ISO 16712:2005(E). Geneva (2005).
125. Buccolieri A, Buccolieri G, Cardellicchio N, Dell'Atti A, Di Leo A, Maci A. Heavy metals in marine sediments of Taranto Gulf (Ionian Sea, Southern Italy). Mar Chem. (2006) 99:227–35. doi: 10.1016/j.marchem.2005.09.009
126. Prato E, Parlapiano I, Biandolino F, Rotini A, Manfra L, Berducci MT, et al. Chronic sublethal effects of ZnO nanoparticles on Tigriopus fulvus (Copepoda,Harpacticoida). Environ Sci Pollut Res Int. (2019). doi: 10.1007/s11356-019-07006-9. [Epub ahead of print].
127. Faraponova O, Todaro MA, Onorati F, Finoia MG. Sensibilità sesso ed età specifica di Tigriopus fulvus (Copepoda, Harpacticoida) nei confronti di due metalli pesanti (Cadmio e Rame). Biol Mar Medit. (2003) 10:679–81.
128. Faraponova O, de Pascale D, Onorati F, Finoia MG. Tigriopus fulvus (Copepoda, Harpacticoida) as a target species in biological assays. Meiofauna Mar. (2005) 14:91–5.
129. ASTM. Standard guide for conducting 10-d static sediment toxicity tests with marine and estuarine amphipods. In: Annual Book of ASTM Standards, Water and Environmental Technology. Philadelphia, PA: ASTM (1993). p. 1367–92.
130. Litchfield Jt Jr, Wilcoxon F. A simplified method of evaluating dose-effect experiments. J Pharmacol Exp Ther. (1949) 96:99–113.
131. Vale G, Mehennaoui K, Cambier S, Libralato G, Jomini S, Domingos RF. Manufactured nanoparticles in the aquatic environment-biochemical responses on freshwater organisms: a critical overview. Aquat Toxicol. (2016) 170:162–74. doi: 10.1016/j.aquatox.2015.11.019
132. Schrurs F, Lison D. Focusing the research efforts. Nat Nanotechnol. (2012) 7:546–8. doi: 10.1038/nnano.2012.148
133. Baudo R, Faimali M, Onorati F, Pellegrini D. Arrays of ecotossicological assays for salt and brackish waters sediments. Manu Ecotoxicol. (2011) 67:1–144.
134. Pandard P, Devillers J, Charissou AM, Poulsen V, Jourdain MJ, Ferard JF, et al. Selecting a battery of bioassays for ecotoxicological characterization of wastes. Sci Total Environ. (2006) 363:114–25. doi: 10.1016/j.scitotenv.2005.12.016
135. Matranga V, Corsi I. Toxic effects of engineered nanoparticles in the marine environment: model organisms and molecular approaches. Mar Environ Res. (2012) 76:32–40. doi: 10.1016/j.marenvres.2012.01.006
136. Manzo S, Miglietta ML, Rametta G, Buono S, Di Francia G. Toxic effects of ZnO nanoparticles towards marine algae Dunaliella tertiolecta. Sci Total Environ. (2013) 445–446:371–6. doi: 10.1016/j.scitotenv.2012.12.051
137. Handy RD, von der Kammer F, Lead JR, Hassellöv M, Owen R, Crane M. The ecotoxicology and chemistry of manufactured nanoparticles. Ecotoxicology. (2008) 17:287–314. doi: 10.1007/s10646-008-0199-8
138. Handy RD, Henry TB, Scown TM, Johnston BD, Tyler CR. Manufactured nanoparticles: their uptake and effects on fish–a mechanistic analysis. Ecotoxicology. (2008) 17:396–409. doi: 10.1007/s10646-008-0205-1
139. Handy RD, Owen R, Valsami-Jones E. The ecotoxicology of nanoparticles and nanomaterials: current status, knowledge gaps, challenges, and future needs. Ecotoxicology. (2008) 17:315–25. doi: 10.1007/s10646-008-0206-0
140. Wong SWY, Leung PTY, Djurišić AB, Leung KMY. Toxicities of nano zinc oxide to five marine organisms: influences of aggregate size and ion solubility. Anal Bioanal Chem. (2010) 396:609–18. doi: 10.1007/s00216-009-3249-z
141. Heinlaan M, Ivask A, Blinova I, Dubourguier HC, Kahru A. Toxicity of nanosized and bulk ZnO, CuO and TiO2 to bacteria vibrio fischeri and crustaceans daphnia magna and thamnocephalus platyurus. Chemosphere. (2008) 71:1308–16. doi: 10.1016/j.chemosphere.2007.11.047
142. Li J, Schiavo S, Rametta G, Miglietta ML, La Ferrara V, Wu C, et al. Comparative toxicity of nano ZnO and bulk ZnO towards marine algae Tetraselmis suecica and Phaeodactylum tricornutum. Environ Sci Pollut Res. (2017) 26:6543–53. doi: 10.1007/s11356-016-8343-0
143. Baun A, Hartmann NB, Grieger K, Kusk KO. Ecotoxicity of engineered nanoparticles to aquatic invertebrates: a brief review and recommendations for future toxicity testing. Ecotoxicology. (2008) 17:387–95. doi: 10.1007/s10646-008-0208-y
144. Baun A, Sørensen SN, Rasmussen RF, Hartmann NB, Koch CB. Toxicity and bioaccumulation of xenobiotic organic compounds in the presence of aqueous suspensions of aggregates of nano-C(60). Aquat Toxicol. (2008) 86:379–87. doi: 10.1016/j.aquatox.2007.11.019
145. Kohen R, Nyska A. Oxidation of biological systems: oxidative stress phenomena, antioxidants, redox reactions, and methods for their quantification. Toxicol Pathol. (2002) 6:620–50. doi: 10.1080/01926230290166724
Keywords: nanoparticles, Zinc oxide nanoparticles (ZnO NPs), toxicity, marine crustaceans, health risks, workers, consumers, general population
Citation: Vimercati L, Cavone D, Caputi A, De Maria L, Tria M, Prato E and Ferri GM (2020) Nanoparticles: An Experimental Study of Zinc Nanoparticles Toxicity on Marine Crustaceans. General Overview on the Health Implications in Humans. Front. Public Health 8:192. doi: 10.3389/fpubh.2020.00192
Received: 19 September 2019; Accepted: 27 April 2020;
Published: 21 May 2020.
Edited by:
Mohiuddin Md. Taimur Khan, Washington State University Tri-Cities, United StatesReviewed by:
Yue-Wern Huang, Missouri University of Science and Technology, United StatesYlenia Carotenuto, Stazione Zoologica Anton Dohrn, Italy
Copyright © 2020 Vimercati, Cavone, Caputi, De Maria, Tria, Prato and Ferri. This is an open-access article distributed under the terms of the Creative Commons Attribution License (CC BY). The use, distribution or reproduction in other forums is permitted, provided the original author(s) and the copyright owner(s) are credited and that the original publication in this journal is cited, in accordance with accepted academic practice. No use, distribution or reproduction is permitted which does not comply with these terms.
*Correspondence: Luigi Vimercati, luigi.vimercati@uniba.it