- IUF - Leibniz Research Institute for Environmental Medicine, Düsseldorf, Germany
As with toxicology in general, major challenges have emerged in its subfield neurotoxicology regarding the testing of engineered nanomaterials (ENM). This is on the one hand due to their complex physicochemical properties, like size, specific surface area, chemical composition as well as agglomeration and dissolution behavior in biological environments. On the other hand, toxicological risk assessment has faced an increasing demand for the development and implementation of non-animal alternative approaches. Regarding the investigation and interpretation of the potential adverse effects of ENM on the brain, toxicokinetic data are relatively scarce and thus hampers dose selection for in vitro neurotoxicity testing. Moreover, recent in vivo studies indicate that ENM can induce neurotoxic and behavioral effects in an indirect manner, depending on their physicochemical properties and route of exposure. Such indirect effects on the brain may proceed through the activation and spill-over of inflammatory mediators by ENM in the respiratory tract and other peripheral organs as well via ENM induced disturbance of the gut microbiome and intestinal mucus barrier. These ENM specific aspects should be incorporated into the ongoing developments of advanced in vitro neurotoxicity testing methods and strategies.
Introduction
The steady development and production of engineered nanomaterials (ENM) and their expanding range of uses in various fields requires an appropriate assessment of their potential health effects in humans. Initial concern about the harmful effects of ENM emerged from: (i) early inhalation toxicology studies with specific types of ultrafine particles, showing increased local pulmonary toxicity compared to larger particles of the same chemical composition, and (ii) studies that revealed evidence of increased uptake and translocation of nanoscale particles to tissues and organs beyond the initial deposition site in the respiratory tract (1, 2). The importance of toxicology research on ENM has been substantiated by studies that support a role of ambient ultrafine particles (UFP) in air pollution-associated diseases (3). Similarly, increased attention has been given to the potential neurotoxicity of ENM (4–6). A pioneering inhalation study by Oberdoerster and co-workers (7), demonstrated that insoluble carbon particles in the nano size range can rapidly translocate to the brain upon deposition in the nasal mucosa in rats. This observation formed a major trigger for further research to determine potential adverse health effects of inhaled UFP and ENM on the brain.
Adverse effects on the brain have been shown nowadays in various studies. Investigations with diesel engine exhaust (DEE), collected diesel exhaust particles (DEP) or ambient particulate matter (PM) (6, 8) as well as on-site-exposure studies with concentrated ambient PM (CAPs) have provided important support for the growing number of epidemiological studies that link air pollution to neurological diseases including dementia (9, 10). Neurotoxicological studies with ENM in rodents have been performed with the most widely produced and used materials like Ag, SiO2, TiO2, CeO2, ZnO and carbon black. However, also less common materials have been studied like gold, quantum dots or iridium nanoparticles (11–13).
In the context of regulatory testing, neurotoxicity of ENM can be defined as a direct or indirect adverse effect caused by such particulate materials on the structure or functioning of the nervous system (14, 15). The scope of this mini-review is not to provide an in-depth overview of all studies that have been performed with various types of ENM and to provide a state of the art on their identified underlying mechanisms of neurotoxicity. For this we refer to various reviews by others [e.g. (5, 16, 17)]. In this paper, we highlight some of the unique physicochemical properties and associated effects of ENM that should be considered during testing and interpretation of their potential neurotoxicity.
In general, as with other effects and associated disease outcomes (e.g., in the respiratory or cardiovascular system), oxidative stress and inflammation are also considered key processes of potential neurotoxic and neurological consequences following ENM exposure (5, 6). Oxidative stress in ENM exposed cells, can cause activation of redox-sensitive signaling cascades involved in activation of pro-inflammatory cytokines and chemokines, proliferation, apoptosis and DNA damage induction (18), representing mediators or processes that all have been implicated in neurological and neurodegenerative diseases (5, 19). When taken together, current available neurotoxicological studies with ENM suggest substantial differences in hazards, strongly depending on their physicochemical properties, including the chemical composition. However, as we will discuss later, such an interpretation must take into account specific differences in dosimetry, (i.e., dose and route of administration) as well as the species selected for investigation (e.g., rat vs. mouse) used in the various studies, in perspective to realistic human exposures.
Neurotoxicity-Relevant Exposure Routes of Enm
Originally, nanotoxicology research has strongly focused on the evaluation of the potential adverse health effects that involve the inhalation route of exposure and pulmonary health risks. This obviously relates to the principal relevance of occupational exposures during manufacturing and handling of ENM. The emerging consensus that short- and long-term exposure to ambient PM is a risk factor for cardiovascular disease (20) has been a main drive to initiate studies that explored cardiovascular effects and underlying mechanisms of action for ambient UFP as well as for various types of ENM via inhalation exposure (20–22). Regarding this exposure route, for neurological evaluations, the importance of translocation of nasally deposited particles to the brain is now widely recognized. Herein, it is proposed that ENM can reach the olfactory bulbs of the brain following deposition on the nasal olfactory epithelium and subsequent translocation along the olfactory nerve (7). However, various additional routes of translocation into the brain should be acknowledged. In the upper respiratory tract, this may for instance also include translocation via the trigeminal nerve, while alveolar deposited ENM may enter the brain upon their translocation into the circulation (6). In contrast to the neuronal routes, this latter pathway also requires a subsequent passage of the blood-brain barrier (BBB).
The relative importance of translocation from the upper vs. lower respiratory tract has been elegantly explored in inhalation studies using radiolabelled iridium nanoparticles in rats and revealed the significance for both deposition sites (23). However, it should be emphasized that the significance of these various translocation routes may largely differ between various types of ENM depending on their composition and physicochemical properties and the associated impact of differently absorbed proteins and lipids (corona) on their translocation kinetics (6). Obviously, regarding the deposition and subsequent rate of translocation of nano-sized particles from the respiratory tract system, the distinct anatomical and physiological differences between rodents and humans must be taken into account as well (19).
While inhalation is the primary exposure route of interest for most bulk-manufactured ENM in occupational settings, other routes should also be considered regarding potential neurotoxicity. Systemic availability via dermal exposure, either occupationally or, for example, through its presence in cosmetics, is unlikely to be a major concern for neurological disease risks. In terms of dosimetry and accumulation into the brain, dermal uptake has been generally considered as negligible (2) although this may differ for instance in relation to impaired skin conditions during occupational exposure (24). Exposure to ENM via the oral route can be considered much more relevant and has therefore also become a subject of increasing interest in neurotoxicology research. Concerns about the adverse health effects of ingested ENM have increased with their growing number of applications in nanomedicine and particularly in the food sector, where they are used, for example, as food additives or incorporated in food packaging (25, 26). Accumulation of ENM in the brain following oral uptake is envisaged by their successive translocation from the intestine into the systemic circulation and BBB passage. This therefore also represents a potential translocation route for the fractions of inhaled UFP and ENM that are swallowed following mucociliary clearance (1, 27). Translocation from the intestine has indeed been demonstrated for specific ENM of poor solubility in rodents. However, quantitative findings by and large indicate that the amount of accumulation into the brain following oral exposure may be minimal to absent as well [e.g., (28–30)]. A schematic outline of the various exposure and translocation routes that may result in accumulation of UFP and ENM in the central nervous system is shown in Figure 1.
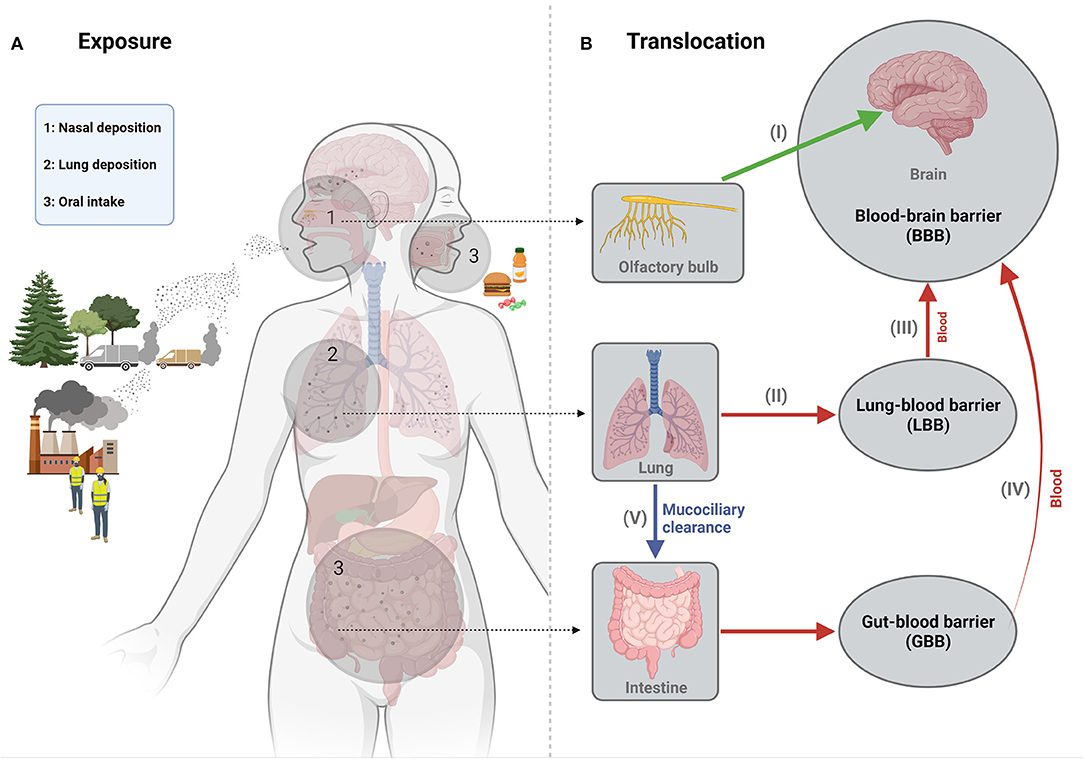
Figure 1. Schematic representation of the main exposure (A) and translocation (B) routes of engineered nanomaterials in relation to their potential neurotoxicity. Concerns about the neurotoxicity of nanoparticles via inhalation exposure exist in particular for UFP originating from combustion processes at transport-dominated locations. Inhalation represents the most important occupational exposure route for ENM, during their manufacturing or further processing. The most relevant pathway of translocation to the brain for such inhaled particles involves their uptake and retrograde transport along the olfactory nerve upon deposition in the nasal cavity (I). UFP and ENM deposited in the lower respiratory tract can also translocate from this region into the bloodstream upon crossing the “lung-blood barrier (LBB)” (II) and a subset of these particles may thus enter the brain parenchyma across the blood-brain barrier (III). In non-occupational settings, the oral exposure route is particularly relevant for ENM, which may be present in food as additives or contaminants. Uptake across the intestinal mucosal barrier into the bloodstream (IV) of these ENM may thus also result in translocation into the brain. This pathway should also be considered for the fraction of inhaled small particles that is swallowed upon lung mucociliary clearance (V). Depending on exposure levels and their physicochemical properties, accumulation of ENM in the brain may thus directly affect brain structures and cells. However, neurotoxicity and neurological disturbances may also proceed in an indirect manner, for instance, driven by inflammatory effects of inhaled or ingested ENM at the organ of entrance or by ENM induced gut microbiome dyshomeostasis. Figure created with Biorender.com.
Neurotoxicity Evaluation of ENM
Neurotoxicity of ENM has been explored in rodent studies as well as in a rapidly growing number of in vitro studies [e.g., see reviews (5, 16, 17)]. While neurotoxicology research involves the investigation of effects of toxicants on both the central and peripheral nervous system (14, 15), current ENM research has strongly focused on the brain. Taken together, current available literature indicates that various ENM may have neurotoxic potential. However, studies cannot always be interpreted unambiguously, not least because of the unique physicochemical properties and behavior of the investigated nanomaterial in biological environments. Since the importance of research into the potential toxicity of ENM was recognized, large-scale research initiatives have been launched to dedicate research to support and improve their risk assessment. For instance, the extensive OECD Testing Programme of Manufactured Nanomaterials was launched, among others, to explore to what extent existing strategies and methods for the safety testing of chemicals are also directly applicable to ENM (31). Over the years, a vast array of studies, have been devoted to the investigation of the toxicity of various ENM, including large-scale interdisciplinary research projects. In this context, several assay artifacts could be identified when testing ENM that could lead to false negative or positive data. As a result, specific assay modifications were developed and further testing recommendations were introduced to minimize potential misclassification [e.g., (32)]. It is crucial that such modifications also be deployed in current available in vitro neurotoxicity assays as well as in early stage development of novel test strategies in this research field (33, 34).
In Table 1, we have summarized intrinsic characteristics and properties of ENM in biological systems that may affect neurotoxicity assay outcomes as well as approaches that can be used to avoid such assay artifacts and potential misclassification. Above all, the reliability of in vitro testing approaches for neurotoxicity relies heavily on the use of appropriate ENM dispersion protocols which have been developed over many years for in vitro testing in general [e.g., (35, 36)]. Neurotoxicity testing should also incorporate the necessary control experiments to ensure that assay readouts are not disturbed due to interference of ENM with assay components such as, for instance, by adsorption of dyes, inactivation of reagents or scattering or quenching of (fluorescence) light. Approaches for such control experiments have been proposed and described by various investigators [e.g., (36–38)] and can be used as a basis when developing novel neurotoxicity assays. A particularly great progress in improving in vitro assays for ENM has been made following the recognition of the role of the protein/lipid corona in the toxicity of ENMs. In addition to the aforementioned establishment of the importance of the corona in the toxicokinetics of ENMs, its demonstrated influence on cellular uptake and toxicity has been used for further assay adaptations. Specific in vitro protocols nowadays incorporate a pre-coating step of the pristine ENM with e.g., (lung) surfactants or serum, or include digestion simulation protocols with gastrointestinal model fluids, to better reflect “bio-nano” interactions that are considered to take place in vivo depending on the route of exposure (39, 40).
Interpretation of neurotoxicity findings with ENM from in vitro studies will also benefit from improved dosimetry considerations. The strength of outcomes of in vitro studies increases with the selection of relevant test concentration ranges that are guided by outcomes from toxicokinetic investigations in rodents (25, 41), and support human health risks estimations under realistic exposure scenarios. Indeed, concentrations of ENM applied in neurotoxicity tests are often in the same order of magnitude as those used for in vitro investigations of effects at entrance organs (i.e., lung, intestine), while available toxicokinetic studies indicate large differences in gradients of locally achieved doses. Unfortunately, in-depth quantitative toxicokinetic studies and physiologically based pharmacokinetic (PBPK) modeling studies with ENM in rodents are currently still scarce [e.g., (30, 46–48)]. Moreover, such investigations are typically limited to one or few specific (model) compounds that may not be representative for other types of ENM, in view of each material's unique physicochemical properties. Common quantitative analytical methods used in toxicokinetic studies include AAS and ICP-MS. However, these methods cannot distinguish particulate from non-particulate (e.g., dissolved) compounds. Approaches that do allow for appropriate morphological and size-resolved analyses like electron microscopy are merely qualitative and thus may have limited relevance in toxicokinetic studies. However, these methods can provide important information to complement in vitro data. For instance, if a toxicokinetic investigation with a metal-based ENM would reveal exclusive accumulation of dissolved metal in the brain, in vitro neurotoxicity studies with the pristine particulate material would be irrelevant and redundant.
In vitro neurotoxicity studies can contribute to hazard identification and are nowadays increasingly used and further developed with high-content and high-throughput adaptations to further reduce animal studies (5, 49). Yet, rodent studies currently still remain a major component of neurotoxicity risk assessment. They can simultaneously tackle multiple aspects of neurotoxicity via evaluation of neurophysiological, neurochemical, neuroanatomical and behavioral endpoints (8). In vivo neurotoxicity of ENM can, for instance, be investigated along the design recommendations of the OECD Test Guideline TG 424 (“Neurotoxicity Study in Rodents”) or embedded in subacute/subchronic oral toxicity studies (e.g., TG 407, 408) or inhalation toxicity studies (e.g., TG 412, 413) by inclusion of specific biochemical and molecular markers of neurotoxicity or neurobehavior tests. Apart from the selection of dose range and exposure duration, also the method of administration of ENM is important for the interpretation of the in vivo neurotoxicity findings. For instance, instead of inhalation exposures, studies have used intranasal administration with ENM at very high doses that do not reflect realistic deposition kinetics during inhalation exposure (6, 23). Such intranasal application in terms of dosimetry can exaggerate the neurological impact of ENM via the olfactory route, e.g., as a result of local damage and tissue impairment. Conversely, pharyngeal aspiration and intratracheal instillation studies bypass the nasal compartment. While these respective approaches can be very useful for specific mechanistic evaluations including toxicokinetic studies, they are obviously of lower relevance for quantitative risk assessment compared to inhalation studies.
Along the same lines, oral exposure studies with ENM also can involve various methods of administration that may have a large impact on toxicokinetics and toxicodynamics and thus study outcome interpretation. The most commonly applied method of oral administration is gavage. However, like the methods of intranasal instillation, intratracheal instillation and pharyngeal aspiration for the inhalation route, this represents a bolus dose delivery of ENM. Application of ENM in drinking water or in food thus represent more realistic administration methods. Marked differences in accumulations of (elemental) silver in brain were for instance observed following single oral gavage versus application in food pellets (42). However, also studies that address neurotoxicity upon administration in drinking water or food as “vehicles” require additional interpretation. Application in drinking water may affect dosing as well as the physicochemical properties of ENM as a result of agglomeration, sedimentation or dissolution. Introduction in food unavoidably results in complex ENM food matrix interactions that can strongly impact on various physicochemical properties as well including surface-reactivity. Drinking water vs. food applications will also result in different stomach and intestine passage times as well as digestive processes. Accordingly, the selection of the method and regime of application may have major potential impact on neurotoxic effects in animal studies. Various in vitro methods have been developed in recent years to simulate how digestion processes can affect the physicochemical properties of ENM (43).
Indirect Effects of ENM on Neurotoxicity
A final important aspect in neurotoxicity risk assessment of ENM is the consideration of direct vs. indirect effects. On the one hand, it is recognized that in vivo neurotoxicity studies may detect indirect effects that are not neurotoxicity-specific. For instance, adverse behavioral responses in exposed rodents may be the consequence of the animals' responses to impairments in other organs and thus a mere reflection of general sickness (8). Inclusion of neurotoxicity-independent indicators of systemic toxicity in the study design, for instance by histology and clinical biochemistry, thus benefits insight on underlying mechanisms of identified neurobehavioural responses in the rodent models and improve judgement of their relevance for neurotoxicity risk in humans. In this context, it is pointed out to be particularly careful when interpreting single-dose animal studies performed at very high dose levels (8). On the other hand, studies may also identify neurotoxicity effects that, albeit indirect, may have a strong mechanistic basis and relevance for humans. The importance of indirect effects of inhaled particles, including ENM, in mutagenesis in the respiratory tract is nowadays well recognized, and considered to be driven by oxidative and proliferative mediators released from recruited inflammatory phagocytes (50, 51). Sustained pulmonary inflammation by ENM has also been proposed as a mechanism for systemic responses e.g., in the cardiovascular system (52). In turn, it is nowadays also increasingly recognized that systemic peripheral inflammation can contribute to neurotoxicity and neurological disease, e.g., via activation of microglia by inflammatory cytokines and other mediators or as a result of infiltration of the brain by peripheral immune cells [reviewed by (44)]. Interestingly, these indirect effects can be amplified in conditions of BBB impairments, and effects on the integrity of this barrier and associated neurotoxic responses have indeed been demonstrated for inhaled ENM (53, 54).
Finally, indirect mechanisms of neurotoxicity should also be recognized for ENM in relation to oral exposure. It has emerged that oral exposure to ENM can lead to changes in the intestinal microbiome (45, 55). Intestinal dyshomeostasis resulting from alterations in microbiota composition and their products (e.g., short-chain fatty acids and associated bidirectional gut-brain-axis signaling process that influence mucosal immune responses and the integrity of the intestinal barrier (56, 57). As such, one can even hypothesize that potential effects of inhaled ENM on the brain could involve microbiome-gut-brain axis effects following their mucociliary clearance (27).
Discussion
With regard to the potential neurotoxic effects of ENM, inhalation and ingestion represent the two most relevant exposure routes. Depending on the route and exposure levels as well as on their specific physicochemical characteristics, ENM may reach the brain via various pathways (Figure 1). While available toxicokinetic/PBPK studies generally indicate that translocation to the brain will be low or even absent, this cannot necessarily be extrapolated to all types of ENM. Further research is needed to guide in vitro dosimetry of molecular mechanistic and animal-alternative neurotoxicity testing methods as well as to substantiate assessment of translocation and potential accumulation of ENM for the human brain. In contrast to current in vitro methods, in vivo studies can also identify neurotoxic effects that results from indirect mechanisms of action of ENM, for instance, mediated by pulmonary and systemic inflammatory mediators or altered gut-brain axis signaling (Figure 1).
To avoid misclassification of ENM regarding their potential neurotoxicity, dosimetric and mechanistic aspects discussed in this paper and summarized on Table 1, should be critically considered in the current and future development of alternative testing methods and strategies. In the regulatory context, the assessment of neurotoxicity has traditionally been strongly focused on the evaluation of in vivo data, whereas in vitro assays have been seen merely as complementary (8, 49). The respective advantages and disadvantages of in vivo and in vitro tests have been discussed and weighed over the years with respect to their values in human neurotoxicity risk assessment [see, for instance: (58–60)]. The importance of animal testing reduction and replacement methods in toxicology is widely recognized today, both in basic and applied research. The extent to which currently available and newly developed in vitro neurotoxicity tests are reliable for the assessment of ENM increases with the recognition and active investigation of the potential occurrence of assay artifacts with these complex particulate substances. At the same time, there is a need for further research into the role of ENM-induced peripheral inflammation, gut dyshomeostasis and other possible indirect mechanisms in the causation of neurological and neurodegenerative diseases.
Author Contributions
All authors listed have made a substantial, direct, and intellectual contribution to the work and approved it for publication.
Funding
This project has received funding from the European Union's Horizon 2020 research and innovation programme under grant agreement no. 814978 (TUBE).
Conflict of Interest
The authors declare that the research was conducted in the absence of any commercial or financial relationships that could be construed as a potential conflict of interest.
Publisher's Note
All claims expressed in this article are solely those of the authors and do not necessarily represent those of their affiliated organizations, or those of the publisher, the editors and the reviewers. Any product that may be evaluated in this article, or claim that may be made by its manufacturer, is not guaranteed or endorsed by the publisher.
References
1. Oberdorster G, Oberdorster E, Oberdorster J. Nanotoxicology: an emerging discipline evolving from studies of ultrafine particles. Environ Health Perspect. (2005) 113:823–39. doi: 10.1289/ehp.7339
2. Borm PJ, Robbins D, Haubold S, Kuhlbusch T, Fissan H, Donaldson K, et al. The potential risks of nanomaterials: a review carried out for ECETOC. Part Fibre Toxicol. (2006) 3:11. doi: 10.1186/1743-8977-3-11
3. Stone V, Miller MR, Clift MJD, Elder A, Mills NL, Moller P, et al. Nanomaterials versus ambient ultrafine particles: an opportunity to exchange toxicology knowledge. Environ Health Perspect. (2017) 125:106002. doi: 10.1289/EHP424
4. Block ML, Elder A, Auten RL, Bilbo SD, Chen H, Chen JC, et al. The outdoor air pollution and brain health workshop. NeuroToxicology. (2012) 33:972–84. doi: 10.1016/j.neuro.2012.08.014
5. Boyes WK, van Thriel C. Neurotoxicology of nanomaterials. Chem Res Toxicol. (2020) 33:1121–44. doi: 10.1021/acs.chemrestox.0c00050
6. Oberdörster G, Elder A, Rinderknecht A. Nanoparticles and the Brain: Cause for Concern? J Nanosci Nanotechnol. (2009) 9:4996–5007. doi: 10.1166/jnn.2009.GR02
7. Oberdörster G, Sharp Z, Atudorei V, Elder A, Gelein R, Kreyling W, et al. Translocation of Inhaled Ultrafine Particles to the Brain. Inhal Toxicol. (2004) 16:437–45. doi: 10.1080/08958370490439597
8. OECD Environment Health and Safety Publications. Guidance Document for Neurotoxicity Testing. Series on testing and Assessment No. 20. NNV/JM/MONO 25 (2004).
9. Power MC, Adar SD, Yanosky JD, Weuve J. Exposure to air pollution as a potential contributor to cognitive function, cognitive decline, brain imaging, and dementia: a systematic review of epidemiologic research. Neurotoxicology. (2016) 56:235–53. doi: 10.1016/j.neuro.2016.06.004
10. Livingston G, Huntley J, Sommerlad A, Ames D, Ballard C, Banerjee S, et al. Dementia prevention, intervention, and care: 2020 report of the lancet commission. Lancet. (2020) 396:413–46. doi: 10.1016/S0140-6736(20)30367-6
11. Chen CY, Liao PL, Tsai CH, Chan YJ, Cheng YW, Hwang LL, et al. Inhaled gold nanoparticles cause cerebral edema and upregulate endothelial aquaporin 1 expression, involving caveolin 1 dependent repression of extracellular regulated protein kinase activity. Part Fibre Toxicol. (2019) 16:37. doi: 10.1186/s12989-019-0324-2
12. Hopkins LE, Patchin ES, Chiu PL, Brandenberger C, Smiley-Jewell S, Pinkerton KE. Nose-to-brain transport of aerosolised quantum dots following acute exposure. Nanotoxicology. (2014) 8:885–93. doi: 10.3109/17435390.2013.842267
13. Buckley A, Warren J, Hodgson A, Marczylo T, Ignatyev K, Guo C, et al. Slow lung clearance and limited translocation of four sizes of inhaled iridium nanoparticles. Part Fibre Toxicol. (2017) 14:5. doi: 10.1186/s12989-017-0185-5
14. Tilson HA. Neurotoxicology in the 1990s. Neurotoxicol Teratol. (1990) 1:293–300. doi: 10.1016/0892-0362(90)90046-F
15. Spencer PS, Lein PJ. Neurotoxicity. In: Encyclopedia of Toxicology, Cambridge, MA: Academic Press. (2014). pp. 489–500.
16. Ge D, Du Q, Ran B, Liu X, Wang X, Ma X, et al. The neurotoxicity induced by engineered nanomaterials. Int J Nanomedicine. (2019) 14:4167–86. doi: 10.2147/IJN.S203352
17. Teleanu DM, Chircov C, Grumezescu AM, Teleanu RI. Neurotoxicity of nanomaterials: An up-to-date overview. Nanomaterials (Basel). (2019) 9:96. doi: 10.3390/nano9010096
18. Unfried K, Albrecht C, Klotz LO, Von Mikecz A, Grether-Beck S, Schins RPF. Cellular responses to nanoparticles: target structures and mechanisms. Nanotoxicology. (2007) 1:52–71. doi: 10.1080/00222930701314932
19. Heusinkveld HJ, Wahle T, Campbell A, Westerink RHS, Tran L, Johnston H, et al. Neurodegenerative and neurological disorders by small inhaled particles. Neurotoxicology. (2016) 56:94–106. doi: 10.1016/j.neuro.2016.07.007
20. Brook RD, Franklin B, Cascio W, Hong Y, Howard G, Lipsett M, et al. Prevention Science of the American Heart, Air pollution and cardiovascular disease: a statement for healthcare professionals from the Expert Panel on Population and Prevention Science of the American Heart Association. Circulation. (2004) 109:2655–71. doi: 10.1161/01.CIR.0000128587.30041.C8
21. Miller MR, McLean SG, Duffin R, Lawal AO, Araujo JA, Shaw CA, et al. Diesel exhaust particulate increases the size and complexity of lesions in atherosclerotic mice. Part Fibre Toxicolo. (2013) 10:61. doi: 10.1186/1743-8977-10-61
22. Møller P, Mikkelsen L, Vesterdal LK, Folkmann JK, Forchhammer L, Roursgaard M, et al. Hazard identification of particulate matter on vasomotor dysfunction and progression of atherosclerosis. Crit Rev Toxicol. (2011) 41:339–68. doi: 10.3109/10408444.2010.533152
23. Kreyling WG. Discovery of unique and ENM- specific pathophysiologic pathways: Comparison of the translocation of inhaled iridium nanoparticles from nasal epithelium versus alveolar epithelium towards the brain of rats. Toxicol Appl Pharmacol. (2016) 299:41–6. doi: 10.1016/j.taap.2016.02.004
24. Larese Filon F, Bello D, Cherrie JW, Sleeuwenhoek A, Spaan S, Brouwer DH. Occupational dermal exposure to nanoparticles and nano-enabled products: Part I—Factors affecting skin absorption. Int J Hyg Environ Health. (2016) 219:536–44. doi: 10.1016/j.ijheh.2016.05.009
25. Sohal IS, O'Fallon KS, Gaines P, Demokritou P, Bello D. Ingested engineered nanomaterials: state of science in nanotoxicity testing and future research needs. Part Fibre Toxicol. (2018) 15:29. doi: 10.1186/s12989-018-0265-1
26. Bouwmeester H, Dekkers S, Noordam MY, Hagens WI, Bulder AS, Heer Cde, et al. Review of health safety aspects of nanotechnologies in food production. Regul Toxicol Pharmacol. (2009) 53:52–62. doi: 10.1016/j.yrtph.2008.10.008
27. Pietroiusti A, Bergamaschi E, Campagna M, Campagnolo L, Palma GDe, Iavicoli S, et al. The unrecognized occupational relevance of the interaction between engineered nanomaterials and the gastro-intestinal tract: a consensus paper from a multidisciplinary working group. Part Fibre Toxicol. (2017) 14:47. doi: 10.1186/s12989-017-0226-0
28. Geraets L, Oomen AG, Krystek P, Jacobsen NR, Wallin H, Laurentie M, et al. Tissue distribution and elimination after oral and intravenous administration of different titanium dioxide nanoparticles in rats. Part Fibre Toxicol. (2014) 11:30. doi: 10.1186/1743-8977-11-30
29. Yokel RA, Hussain S, Garantziotis S, Demokritou P, Castranova V, Cassee FR. The yin: an adverse health perspective of nanoceria: uptake, distribution, accumulation, and mechanisms of its toxicity. Environ Sci Nano. (2014) 1:406–28. doi: 10.1039/C4EN00039K
30. Kreyling WG, Holzwarth U, Schleh C, Kozempel J, Wenk A, Haberl N, et al. Quantitative biokinetics of titanium dioxide nanoparticles after oral application in rats: Part 2. Nanotoxicology. (2017) 11:443–53. doi: 10.1080/17435390.2017.1306893
31. Rasmussen K, Gonzalez M, Kearns P, Sintes JR, Rossi F, Sayre P. Review of achievements of the OECD Working Party on Manufactured Nanomaterials' Testing and Assessment Programme From exploratory testing to test guidelines. Regul Toxicol Pharmacol. (2016) 74:147–60. doi: 10.1016/j.yrtph.2015.11.004
32. Stone V, Johnston H, Schins RPF. Development of in vitro systems for nanotoxicology: methodological considerations. Crit Rev Toxicol. (2009) 39:613–26. doi: 10.1080/10408440903120975
33. Schmidt BZ, Lehmann M, Gutbier S, Nembo E, Noel S, Smirnova L, et al. In vitro acute and developmental neurotoxicity screening: an overview of cellular platforms and high-throughput technical possibilities. Arch Toxicol. (2017) 91:1–33. doi: 10.1007/s00204-016-1805-9
34. Sachana M, Shafer TJ, Terron A. Toward a better testing paradigm for developmental neurotoxicity: OECD efforts and regulatory considerations. Biology (Basel). (2021) 10:86. doi: 10.3390/biology10020086
35. Hartmann NB, Jensen KA, Baun A, Rasmussen K, Rauscher H, Tantra R, et al. Techniques and protocols for dispersing nanoparticle powders in aqueous media-is there a rationale for harmonization? J Toxicol Environ Health B Crit Rev. (2015) 18:299–326. doi: 10.1080/10937404.2015.1074969
36. Petersen EJ, Ceger P, Allen DG, Coyle J, Derk R, Garcia-Reyero N, et al. US federal agency interests and key considerations for new approach methodologies for nanomaterials. ALTEX. (2022) 39:183–206. doi: 10.14573/altex.2105041
37. Wilhelmi V, Fischer U, van Berlo D, Schulze-Osthoff K, Schins RP, Albrecht C. Evaluation of apoptosis induced by nanoparticles and fine particles in RAW 2647 macrophages: facts and artefacts. Toxicol In Vitro. (2012) 26:323–34. doi: 10.1016/j.tiv.2011.12.006
38. MacCormack TJ, Meli MV Ede JD, Ong KJ, Rourke JL, Dieni CA. Commentary: Revisiting nanoparticle-assay interference: There's plenty of room at the bottom for misinterpretation. Comp Biochem Physiol B Biochem Mol Biol. (2021) 255:110601. doi: 10.1016/j.cbpb.2021.110601
39. Wang L, Castranova V, Mishra A, Chen B, Mercer RR, Schwegler-Berry D, et al. Dispersion of single-walled carbon nanotubes by a natural lung surfactant for pulmonary in vitro and in vivo toxicity studies. Part Fibre Toxicol. (2010) 7:31. doi: 10.1186/1743-8977-7-31
40. Llewellyn SV, Kämpfer A, Keller JG, Vilsmeier K, Büttner V, Ag Seleci D, et al. Simulating nanomaterial transformation in cascaded biological compartments to enhance the physiological relevance of in vitro dosing regimes: optional or required? Small. (2021) 17:e2004630. doi: 10.1002/smll.202004630
41. Ma-Hock L, Sauer UG, Ruggiero E, Keller JG, Wohlleben W, Landsiedel R. The use of nanomaterial in vivo organ burden data for in vitro dose setting. Small. (2021) 17:2005725. doi: 10.1002/smll.202005725
42. Sofranko A, Wahle T, Heusinkveld HJ, Stahlmecke B, Dronov M, Pijnenburg D, et al. Evaluation of the neurotoxic effects of engineered nanomaterials in C57BL/6J mice in 28-day oral exposure studies. Neurotoxicology. (2021) 84:155–71. doi: 10.1016/j.neuro.2021.03.005
43. Kämpfer AAM, Busch M, Schins RPF. Advanced in vitro testing strategies and models of the intestine for nanosafety research. Chem Res Toxicol. (2020) 33:1163–78. doi: 10.1021/acs.chemrestox.0c00079
44. Filipov NM. Chapter Six - Overview of peripheral and central inflammatory responses and their contribution to neurotoxicity. In: Aschner M, Costa LG, editors. Advances in Neurotoxicology. Academic Press. (2019). pp. 169–93.
45. Lamas B, Martins Breyner N, Houdeau E. Impacts of foodborne inorganic nanoparticles on the gut microbiota-immune axis: potential consequences for host health. Part Fibre Toxicol. (2020) 17:19. doi: 10.1186/s12989-020-00349-z
46. Li D, Morishita M, Wagner JG, Fatouraie M, Wooldridge M, Eagle WE, et al. In vivo biodistribution and physiologically based pharmacokinetic modeling of inhaled fresh and aged cerium oxide nanoparticles in rats. Part Fibre Toxicol. (2016) 13:45. doi: 10.1186/s12989-016-0156-2
47. Recordati C, Maglie MDe, Cella C, Argentiere S, Paltrinieri S, Bianchessi S, et al. Repeated oral administration of low doses of silver in mice: tissue distribution and effects on central nervous system. Part Fibre Toxicol. (2021) 18:23. doi: 10.1186/s12989-021-00418-x
48. Carlander U, Moto TP, Desalegn AA, Yokel RA, Johanson G. Physiologically based pharmacokinetic modeling of nanoceria systemic distribution in rats suggests dose- and route-dependent biokinetics. Int J Nanomedicine. (2018) 13:2631–46. doi: 10.2147/IJN.S157210
49. Bal-Price A, Lein PJ, Keil KP, Sethi S, Shafer T, Barenys M, et al. Developing and applying the adverse outcome pathway concept for understanding and predicting neurotoxicity. Neurotoxicology. (2017) 59:240–55. doi: 10.1016/j.neuro.2016.05.010
50. Driscoll KE, Carter JM, Howard BW, Hassenbein DG, Pepelko W, Baggs RB, et al. Pulmonary inflammatory, chemokine, and mutagenic responses in rats after subchronic inhalation of carbon black. Toxicol Appl Pharmacol. (1996) 136:372–80. doi: 10.1006/taap.1996.0045
51. Donaldson K, Poland CA, Schins RPF. Possible genotoxic mechanisms of nanoparticles: Criteria for improved test strategies. Nanotoxicology. (2010) 4:414–20. doi: 10.3109/17435390.2010.482751
52. Ganguly K, Ettehadieh D, Upadhyay S, Takenaka S, Adler T, Karg E, et al. Early pulmonary response is critical for extra-pulmonary carbon nanoparticle mediated effects: comparison of inhalation versus intra-arterial infusion exposures in mice. Part Fibre Toxicol. (2017) 14:19. doi: 10.1186/s12989-017-0200-x
53. Aragon MJ, Topper L, Tyler CR, Sanchez B, Zychowski K, Young T, et al. Serum-borne bioactivity caused by pulmonary multiwalled carbon nanotubes induces neuroinflammation via blood-brain barrier impairment. Proc Natl Acad Sci U S A. (2017) 114:E1968–76. doi: 10.1073/pnas.1616070114
54. Disdier C, Chalansonnet M, Gagnaire F, Gate L, Cosnier F, Devoy J, et al. Brain inflammation, blood brain barrier dysfunction and neuronal synaptophysin decrease after inhalation exposure to titanium dioxide nano-aerosol in aging rats. Sci Rep. (2017) 7:12196. doi: 10.1038/s41598-017-12404-5
55. Bredeck G, Kampfer AAM, Sofranko A, Wahle T, Lison D, Ambroise J, et al. Effects of dietary exposure to the engineered nanomaterials CeO2, SiO2, Ag, and TiO2 on the murine gut microbiome. Nanotoxicology. (2021) 15:934–50. doi: 10.1080/17435390.2021.1940339
56. Dempsey JL, Little M, Cui JY. Gut microbiome: an intermediary to neurotoxicity. Neurotoxicology. (2019) 75:41–69. doi: 10.1016/j.neuro.2019.08.005
57. Morais LH, Schreiber HL, Mazmanian SK. The gut microbiota–brain axis in behaviour and brain disorders. Nat Rev Microbiol. (2021) 19:241–55. doi: 10.1038/s41579-020-00460-0
58. Costa LG. Neurotoxicity testing: a discussion of in vitro alternatives. Environ Health Perspect. (1998) 106:505–10. doi: 10.1289/ehp.98106505
59. Smith RA. Twenty-first century challenges for in vitro neurotoxicity. Altern Lab Anim. (2009) 37:367–75. doi: 10.1177/026119290903700407
Keywords: neurotoxicity, nanomaterials, in vitro, toxicokinetic, engineered nanomaterials
Citation: Scarcello E, Sofranko A, Wahle T and Schins RPF (2022) Neurotoxicity of Engineered Nanomaterials: Testing Considerations. Front. Public Health 10:904544. doi: 10.3389/fpubh.2022.904544
Received: 25 March 2022; Accepted: 20 June 2022;
Published: 13 July 2022.
Edited by:
Kevin Driscoll, Rutgers, The State University of New Jersey, United StatesReviewed by:
Jian-jun Chen, Chongqing Medical University, ChinaThomas Webster, Interstellar Therapeutics, United States
Deborah A. Cory-Slechta, University of Rochester, United States
Copyright © 2022 Scarcello, Sofranko, Wahle and Schins. This is an open-access article distributed under the terms of the Creative Commons Attribution License (CC BY). The use, distribution or reproduction in other forums is permitted, provided the original author(s) and the copyright owner(s) are credited and that the original publication in this journal is cited, in accordance with accepted academic practice. No use, distribution or reproduction is permitted which does not comply with these terms.
*Correspondence: Roel P. F. Schins, cm9lbC5zY2hpbnNAaXVmLWR1ZXNzZWxkb3JmLmRl