- 1Departamento de Vulcanología, Instituto de Geofísica, Universidad Nacional Autónoma de México, Mexico City, Mexico
- 2Posgrado en Ciencias de la Tierra, Centro de Ciencias de la Atmósfera, Universidad Nacional Autónoma de México, Mexico City, Mexico
- 3Laboratorio Nacional de Geoquímica y Mineralogía, Universidad Nacional Autónoma de México, Mexico City, Mexico
- 4Posgrado en Ciencias de la Tierra, Universidad Nacional Autónoma de México, Mexico City, Mexico
- 5Seismology-Gravimetry, Royal Observatory of Belgium, Brussels, Belgium
How do lava domes release volcanic gases? Studying this problem is crucial to understand, and potentially anticipate, the generation of the sudden and dangerous explosive eruptions that frequently accompany dome extrusions. Since its awakening in 1994, Popocatépetl volcano has produced more than 50 lava domes and has been consistently among the strongest permanent emitters of volcanic gases. In this work, we have characterized the passive and explosive degassing between 2013 and 2016 at a high time resolution using an SO2 camera, to achieve a better understanding of the conduit processes. Our 4-year average SO2 flux is 45 kg/s, in line with the long-term average of the whole current eruptive period. We show that Popocatépetl volcano is essentially an open system and that passive degassing, i.e., degassing with no associated emission of lava or ash, dominates >95% of the time. This passive degassing is continuous and sustained, whether the crater contains a lava dome or not. It shows most of the time a strong periodic component, with a pseudo-period of ~5 min, and amplitudes of 30 to 60% of the average value. We could distinguish two types of explosions based on their SO2 flux patterns. The first type (E1) occurs in the middle of the normal passive degassing and is followed by a rapid return of the SO2 flux down to its pre-explosive level. The second type (E2), which corresponds to the strongest events, is anticipated by a rapid decrease of the SO2 flux to abnormally low values and is followed by a return to its normal values. The E2 explosions are probably caused by the accumulation of gas below a rapidly compacting permeable dome. We suggest that transient episodes of gravitational compaction of the usually permeable dome and the upper conduit is the only mechanism that is fast enough to explain the sharp decrease of the SO2 flux that anticipates the E2 explosions. Our model is potentially applicable to a large number of andesitic volcanoes that undergo passive degassing interspersed with short-lived explosions.
Introduction
Lava domes are structures that result from the extrusion and accumulation of extremely viscous, quasi solid, lava and that are commonly formed at andesitic stratovolcanoes. They are often affected by dangerous eruptive phases involving partial collapse and/or the sudden transition to highly explosive activity (e.g., Boudon et al., 2015) that result in potentially dangerous pyroclastic density currents. The generation of explosive eruptions from lava domes is thought to be caused by spatial and temporal changes of their permeability (e.g., Collinson and Neuberg, 2012) and of their ability to exsolve and release volatiles (e.g., Sparks, 1997; Stix et al., 1997), but in detail, the causes are still a matter of debate. Recent work has been done on measuring experimentally the porosity and permeability of lava dome samples (e.g., Gaunt et al., 2014; Farquharson et al., 2015), but relatively few studies have focused on field measurements of gas fluxes from lava dome eruptions. Most of these studies have reported SO2 fluxes that were generally low (0.5–10 kg/s), highly variable, or even intermittent (e.g., Young et al., 2003; Holland et al., 2011; Smekens et al., 2015), indicating that the studied domes (Soufriere Hills in Montserrat, Santiaguito in Guatemala and Semeru in Indonesia, respectively) were relatively weak emitters of SO2 and that cyclic extrusion processes were controlling the release of the gas. Two volcanoes with lava domes, Lascar (Matthews et al., 1997) and Popocatépetl (Delgado-Granados et al., 2001; Delgado-Granados, 2008) were found to emit significantly larger SO2 fluxes in the 1990s. While the former is not erupting anymore nor is it emitting large quantities of gas, the latter has been carrying up with its eruptive period and strong gas emission as of 2018, and is the subject of the present study.
Popocatépetl volcano (5,452 m a.s.l.) is a large compound stratovolcano located in central Mexico (Figure 1), between the megacities of Mexico City (~25 million inhabitants, distant 70 km) and Puebla (~7 million inhabitants, distant 40 km). It has been active since ~500,000 years, erupting lava that ranged from basaltic andesites to dacites belonging to the calc-alkaline series (e.g., Siebe and Macías, 2006). Its historical activity has consisted of small to medium-scale explosions accompanying or alternating with extrusions of viscous intracrateric lava flows or domes. However, large effusive eruptions (Espinasa-Pereña and Martín-Del Pozzo, 2006), five powerful plinian eruptions (Siebe and Macías, 2006) and one massive sector collapse (Siebe et al., 2017) have occurred at the volcano during the last 25,000 years. The high recurrence of such events, coupled with the extraordinary large population living around Popocatépetl, makes the volcano one of the most probable candidate for a large volcanic disaster in the future (Siebe et al., 1996; De la Cruz-Reyna and Tilling, 2008; Delgado Granados and Jenkins, 2016).
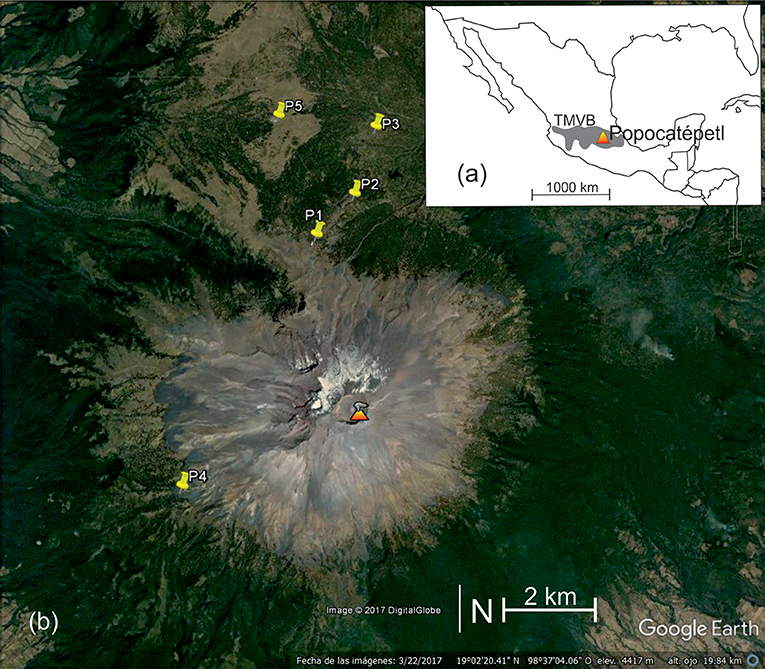
Figure 1. (a) Location of Popocatépetl Volcano within the Trans-Mexican Volcanic Belt. (b) Satellite image of Popocatépetl Volcano showing the viewing points used in this study.
After several years of increasing seismic and fumarolic unrest, a new eruptive period started at Popocatépetl in December 1994, and is still going on at the time of writing. The activity initially consisted of vent-clearing explosions and ash emission of phreatic origin until 1996 when, for the first time, a flat shaped lava dome was observed in the crater (De la Cruz-Reyna and Siebe, 1997). Since then, cycles of dome building and destruction have characterized the activity of the volcano (Gómez-Vazquez et al., 2016), slowly filling its 850 × 600 m wide summit crater (Figure 2). The total volume of erupted lava has not exceeded 4 107 m3 (Gómez-Vazquez et al., 2016). The domes usually grow relatively quickly (within a few days to weeks), stall in the crater without further growth during a period that can last between a few days to a few years, until an explosion or a series of explosions destroys them. Gómez-Vazquez et al. (2016) found a weak correlation between the size of the domes and the magnitude of the explosions that destroy them. The mechanism of these explosions has been postulated to be gas accumulation beneath (or within) the cooling and crystallizing lava dome (Stremme et al., 2011; Gómez-Vazquez et al., 2016). The peak of activity, in 2000–2003, was characterized by the rapid growth of large lava domes, tens of strong vulcanian explosions, SO2 fluxes up to 1,700 kg/s and a powerful subplinian phase that sent an ash column up to 17 km a.s.l. and produced 5 km long pyroclastic flows (Martin-Del Pozzo et al., 2003; Delgado-Granados, 2008). Evacuation of the closest villages was ordered during this eruptive phase. Several other phases of strong activity have occurred since then, such as in March-June 2012, April-July 2013, or January-May 2015 and September-November 2017.
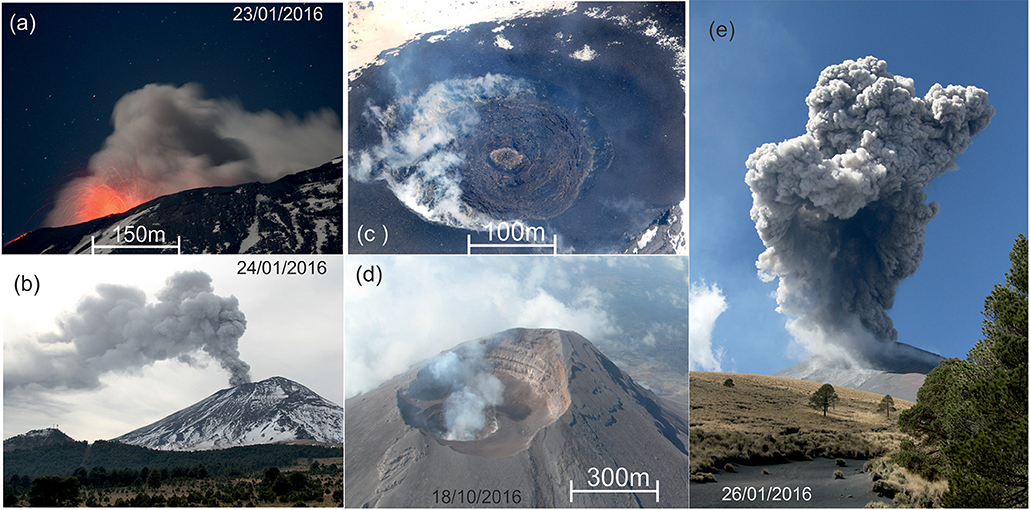
Figure 2. Typical styles of activity occurring at Popocatépetl Volcano. (a) Weakly explosive activity and (b) continuous ash emission associated to the construction of a lava dome. (c) Lava dome filling the crater and degassing passively. (d) Passive Degassing without a lava dome. (e) Vulcanian explosion associated to dome destruction. All photos by R.C. except (c) by Ramon Espinaza.
Arguably the most distinctive aspect of the whole eruptive period has been the extremely high emissions of volcanic gases, and the extreme disproportion between the emitted gas and the erupted lava. The SO2 flux has been measured at Popocatépetl since 1994, first with a COSPEC instrument (Delgado-Granados et al., 2001; Delgado-Granados, 2008), then with a network of scanning DOAS spectrometers, and more recently using satellites. The long-term average of SO2 emission rates over the 24-year (1994–2017) eruptive phase has been around 55 kg/s (~4,800 tons/day), while the peak emission rate reached the extraordinary value of 1,700 kg/s in December 2000. The cumulative SO2 release over these 24 years of activity reaches the extremely large value of 4 ± 1 107 tons. For comparison, this amounts to twice as much as what Pinatubo emitted during its large plinian eruption of 1991, which is the highest measurement of eruptive SO2 release on record. If the amount of gas emitted mostly passively by Popocatépetl during these 24 years had escaped massively in a short lapse like at Pinatubo, it could have fueled a plinian eruption comparable to those that the volcano produced in the last 25,000 years. Based on melt inclusions data in scarce olivine crystals, Roberge et al. (2009) concluded that this amount of gas could have been produced by the degassing of at least 3 km3 of volatile-rich basaltic magma, which intrudes at depth >10 km and has remained essentially unerupted. Here we investigate the conduit processes that allow such a high and sustained degassing using measurements acquired with an SO2-camera at a high time resolution.
Methodology
Our measurements were obtained with an ultraviolet SO2 camera (Mori and Burton, 2006; Kern et al., 2010b, 2015) during punctual campaigns through 2013–2016. Our instrumental set-up is composed of two co-aligned Alta U260 cameras equipped with Pentax BUV2528 silica lenses, and UV band pass filters centered at 310 and 330 nm, respectively (Asahi XBPA310 and XBPA330, of 10 nm FWHM, and 75% peak transmittance), located in front of the lens. An additional Hoya340 filter was placed in front of each bandpass filter to avoid longer wavelength radiation to reach the CCD sensors through the leaks of the filters' transmittance function off their main peak. The instrument was operated from one of the spots shown in Figure 1, which are located at distances of 4–7.5 km from the crater. This range of distance, given the large size of the Popocatépetl volcanic plume, is considered as the best compromise for limiting the effect of light dilution (Mori et al., 2006; Kern et al., 2010a; Campion et al., 2015) and having the plume well-framed within the instrument's field of view (23°). Images were acquired at a sampling rate of 5–15 s, depending on the distance to the plume and on the wind speed. The images were processed using the methodology described in Campion et al. (2015). The scattering coefficient of the atmosphere was first retrieved based on the exponential attenuation of the contrast with respect to the distance in a scattering atmosphere. Then, the differential absorbance was calculated for every pair of images after having them corrected for the light dilution effect using the scattering coefficient retrieved earlier. Finally, the 2D distribution of the SO2 in the plume was obtained by multiplying the absorbance images with a calibration coefficient that was obtained by imaging a series of 5 calibration cells containing known concentrations of SO2 (0, 500, 1,000, 1,500, and 3,000 ppm.m). The SO2 emission rate was calculated by integrating the column amount measured along a profile perpendicular to the plume and multiplying this quantity by the projected velocity of the plume, which was measured by autocorrelation on a profile that was drawn parallel to the plume direction. All the results presented in this study were obtained during optimal measurement conditions, i.e., no clouds or haze between the plume and the volcano, plume fully framed in the field of view, well defined plume transport direction, distances inferior to 7 km and optically thin plume. Therefore, we estimate the total error on the flux to be below 25% (Campion et al., 2015). However, a larger error likely affects the column amounts retrieved just above the vent and in the very proximal parts of the plume because the high aerosol optical densities and SO2 columns, which often exceeded our highest calibration cell, make the plume nearly opaque at 310 nm. We avoided this problem by measuring the SO2 flux downwind of the crater where the plume has already been diluted enough to be optically thin and have its SO2 column in the range of our calibration cells. Ash in the plume causes a systematic underestimation of the retrieved SO2 column, because in that case the light reaching the camera originates mostly from reflection of the sun light on the particles of the outer shell of the plume.
Results
Over the 4-year period (2013–2016), we collected SO2 camera data fulfilling the above-defined quality criteria over 20 days, amounting to ~80 h of recordings (Table 1). Based on the visual observations during the measurements, we distinguished three types of activity: passive degassing, explosions and continuous ash emissions. Passive degassing, by far the most common form of activity at Popocatépetl, is defined as the continuous release of ash-free plume. Explosions are short-lasting energetic emissions of ash-laden plume that occasionally eject rock fragments outside of the crater. Dense juvenile material is by far the dominant component in the ashes produced by the explosions. Episodes of continuous ash venting, the less frequent form of activity, usually last from a few hours to a few days and are associated with the growth of lava domes. Abundant lava fragments are also ejected in -or outside of the crater during these episodes. The ash emitted during these episodes is a mixture of dense and vesiculated juvenile fragment, unlike the ash from explosions. Observation through four permanent webcams shows that the volcano has a rather monotonous behavior and that these three styles are enough to describe the whole activity of the volcano in the last 4 years (see also Gómez-Vazquez et al., 2016 and Centro Nacional de Prevención de Desastre, 1995). We acknowledge that the low number of measurements hours and days over the reporting period is insufficient for establishing the long-term evolution of the SO2 flux, and emphasize that this study focuses on rapid fluctuations associated to conduit processes. However, we obtained SO2 camera measurements of each eruptive style, so that, although the total duration of our measurements only amounts to 80 h, they can be considered as representative of the short-term volcano behavior.
Passive Degassing
Passive degassing at Popocatépetl is permanent and our measurements showed that typical SO2 fluxes range between 20 and 80 kg/s, with a 4-year average of 45 kg/s (3,900 tons/day). SO2 fluxes measured a few hours to weeks after a dome growth episode are similar to periods where no dome was present. This implies that the presence of a lava dome does not seem to decrease the overall permeability of the conduit system. A distinctive characteristic of Popocatépetl degassing is its puffing behavior, which is characterized by quasi-periodic oscillations of the SO2 flux time series, whose relative amplitude is typically 30–50% of the mean value (Figure 3 and Video 1). The SO2 mass of individual puffs ranges between 0.5 and 10 tons. This is the first time that puffing is quantified at Popocatépetl volcano, although some hints of its existence had been previously obtained by visual observation and by flying with a COSPEC parallel to the plume axis (Delgado-Granados, unpublished data). Puffing at Popocatépetl is observed systematically every time the wind speed is below ~15 m/s. At higher wind speeds, the plume is forced back into the crater by a strong vortex that develops downwind from the summit and subsequently bent down along the upper slope of the volcano. This homogenizes the plume and blurs the puffing signature. We applied a Fourier Transform to the time series of SO2 flux to derive their power spectra. The spectra show a prominent peak corresponding to the periodic puffing (Figures 3D,E), whose fundamental period is systematically between 200 and 400 s. Longer period flux variations dominate only on days where explosions occur, and are associated with the decrease of the SO2 flux before them.
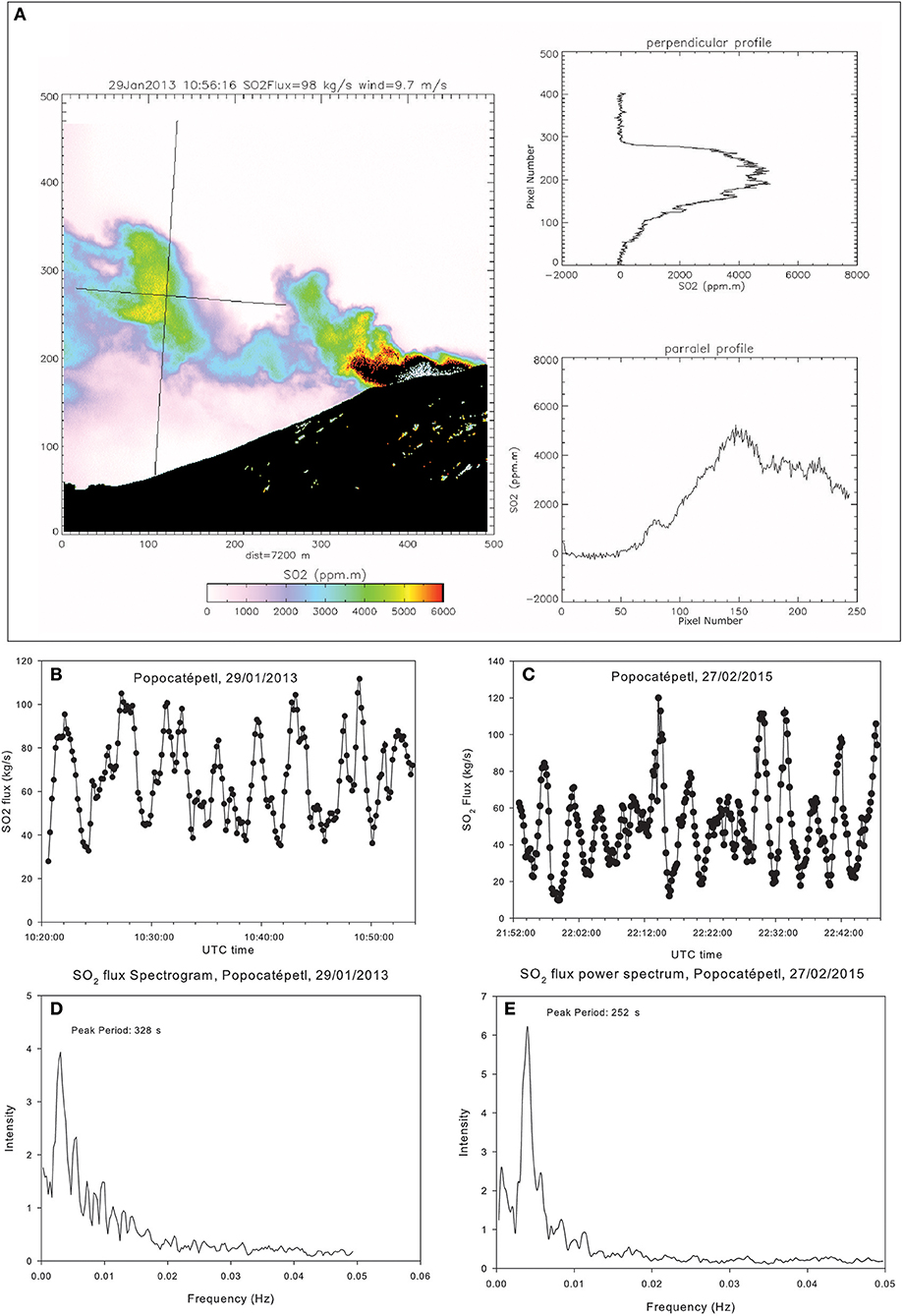
Figure 3. Pulsating passive degassing at Popocatépetl. (A) Aspect of the puffs on an SO2 distribution map taken from Paso de Cortés (location P5 in Figure 1, 7.5 km South of the crater). Two time series of SO2 flux during passive degassing measured on 29/01/2013 (B) and 27/02/2015 (C) showing the quasi-periodic puffing behavior (D) and (E) discrete Fourier Transform spectra of the time series presented in Figures 3B,C, respectively highlighting the ~300 s periodicity.
Explosions
Explosions occur rather frequently at Popocatépetl volcano (e.g., Figure 2e), varying in size from small ash puffs to strong vulcanian explosions showering the slopes of the volcano with ballistic fragments up to distances of 4 km. The high ash content of the explosions plumes induces a systematic underestimation of the SO2 measurements, and can even completely hamper the retrieval if the plume is completely opaque. A total of 17 explosions were captured by the camera during the campaigns. Based on the evolution of the flux before, during and after each explosion, we could recognize two types of explosions. Explosions of the first type (hereafter called E1) produce a peak of SO2 flux interrupting the normal passive degassing, and are followed by a rapid (a few minutes) return to the pre-explosion flux values (Figure 4). The E1 explosions usually produce low to moderate amounts of ashes. In some instances, once the plume was sufficiently diluted, we could obtain a lower constraint on the SO2 mass released by each explosion by integrating the SO2 flux peak above a baseline, defined as the SO2 flux measured just before the leading edge of the explosion plume reaches the integration line. Several of these explosions appear as spikes emphasized with red arrows, in the graph of Figure 4B, which shows one of the longest time series we have been able to obtain so far on a day where explosions were occurring. The resulting values range between 2 and 12 tons of SO2. Assuming a standard subduction zone magmatic gas that contains 2 mol% SO2, 90 mol% H2O, and 8 mol% CO2 (e.g., review by Taran and Zelenski, 2014), these SO2 masses translate into total amounts of released gas is in the range of ~30–200 tons.
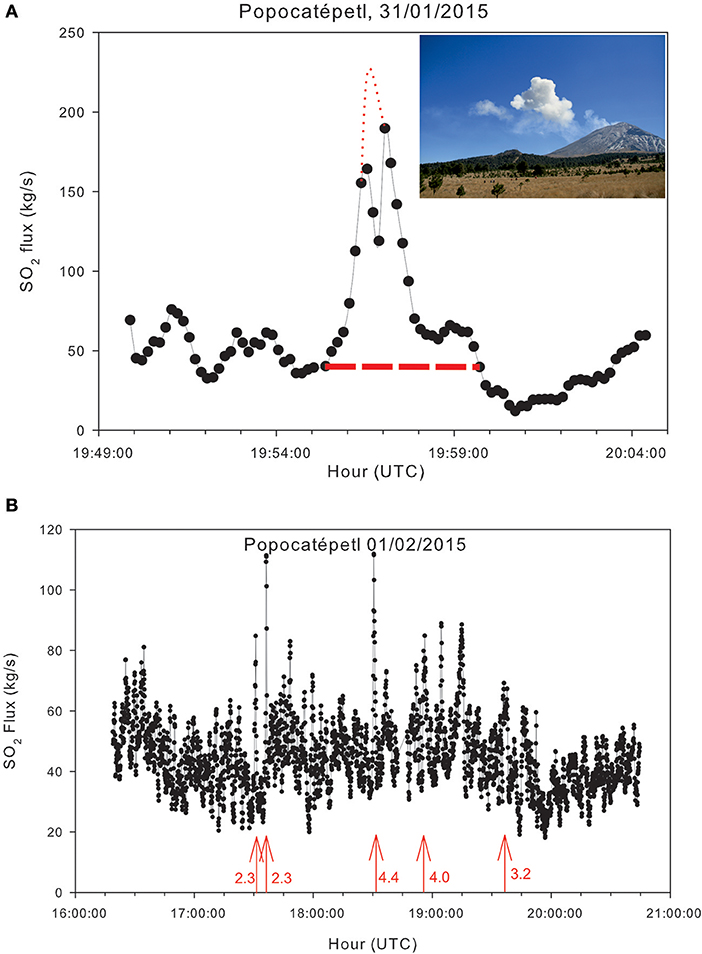
Figure 4. (A) Typical SO2 time series for an E1 type explosion, showing a sharp peak in midst of the normal degassing, followed by a rapid return to the pre-explosion values. The SO2 mass (in tons) emitted by the explosion is calculated by integrating the curve above the background flux, highlighted by the thick dotted line. The presence of ash in the plume causes an underestimation of the real flux values, tentatively represented by the thin red line. (B) A 5 h long time series of SO2 fluxes on a day where E1 explosions (shown as red arrows) were occurring frequently. The mass released by each explosion is written next to its arrow, when it was possible to calculate it.
The second type of explosions (E2) is characterized by a period of anomalously low SO2 flux preceding the explosion and a return to the more typical high and sustained flux after the explosion has occurred (Figure 5). An animation made from SO2 measurements during a moderate E2 explosion is provided as Supplementary Material (Video 2). The E2 explosions seem to be less common than the E1, as only four of them (compared to 13 E1) were recorded with the UV camera over the measurement period. They are also usually more energetic, produce larger quantities of ash and sometimes eject bombs outside of the crater. The SO2 flux pattern associated with these explosions suggests that they are triggered by the accumulation of gas under a temporary plug or seal of the upper conduit, as is the case for the vulcanian explosions of Sakurajima (e.g., Iguchi et al., 2008; Kazahaya et al., 2016). The high ash content in E2 explosions unfortunately prevents quantifying their SO2 content using an SO2 camera, because of the complete opacity of the plume close to the crater. However, since the decrease of the flux preceding these explosions was on some occasions well characterized as a sharp drop of SO2 emissions from their initial values, we could estimate the mass of accumulated gas by integrating the flux curve below its former baseline. This yields values of 10 to 50 tons of SO2 per explosion, which, assuming the same gas composition as earlier, correspond to total amounts of accumulated gases in the range of ~160 to 800 tons. However, it should be emphasized that the four E2 explosions that we have measured are by far not the largest (in terms of the number of ballistic fragments expelled, the distance they reach and the eruptive column height) that the volcano has produced over the reporting period. These stronger E2 explosions, observed both in the field and with the webcam images, have visually the same behavior as the small and moderate E2 explosions that we were able to measure. They are preceded by a period of reduced gas emissions, have an impulsive start and are followed by a prolonged period of stronger, pulsating gas emissions.
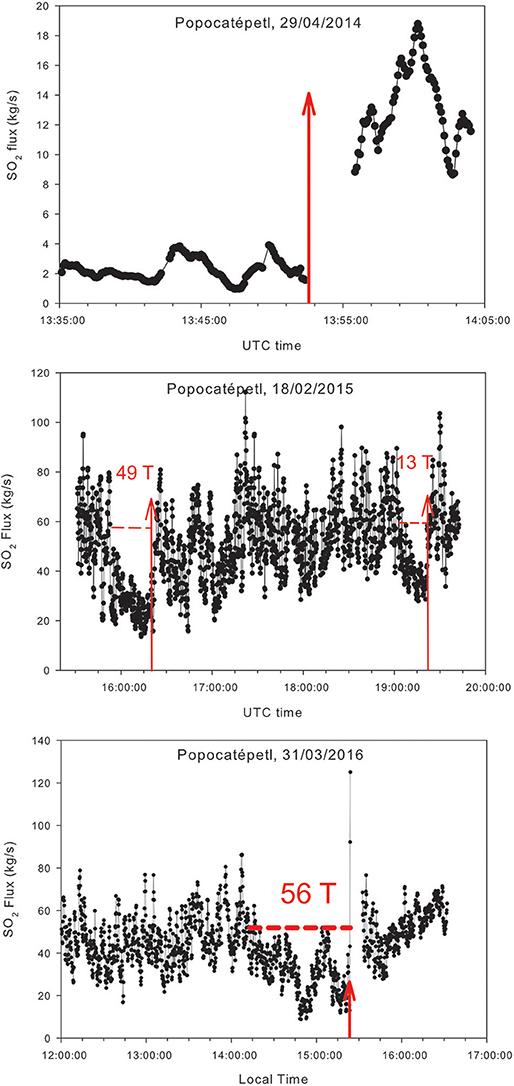
Figure 5. SO2 time series for the four E2-type explosions that we recorded so far. Except for the explosion of 29/04/2014, for which the SO2 measurements started just 20 min before the event and the flux decrease was probably missed, all the explosions share a common pattern, featuring a rapid decrease of the SO2 flux 20–60 min before the eruption, a period of low flux where gas accumulates and pressure builds up, and significantly higher post explosive flux values. The SO2 mass accumulated before the explosions (whose time is indicated as the red arrow) is calculated by integrating the curve below the background flux (highlighted by the red dashed line).
Sustained Ash Emissions
Due to their rarity, only one episode of sustained ash emission could be measured over the reported period, on the 26/01/2016, during an episode of dome growth that lasted for 3 days and emplaced ~2 106 m3 of lava (Centro Nacional de Prevención de Desastre, 1995). The average SO2 emission rate for this day is 120 kg/s, which corresponds to the highest value measured with the camera over the reporting period. Yet, this value is probably still an underestimation because of the presence of ash in the plume. The processing of an image taken by the satellite-based Ozone Monitoring Instrument (OMI, Carn et al., 2008) on the next day, while the episode was still in progress, yields an emission rate of ~250 kg/s (Figure 6). Interestingly, since the beginning of the current eruptive period, the highest SO2 flux measured with COSPEC have also been systematically associated with episodes of lava dome growth.
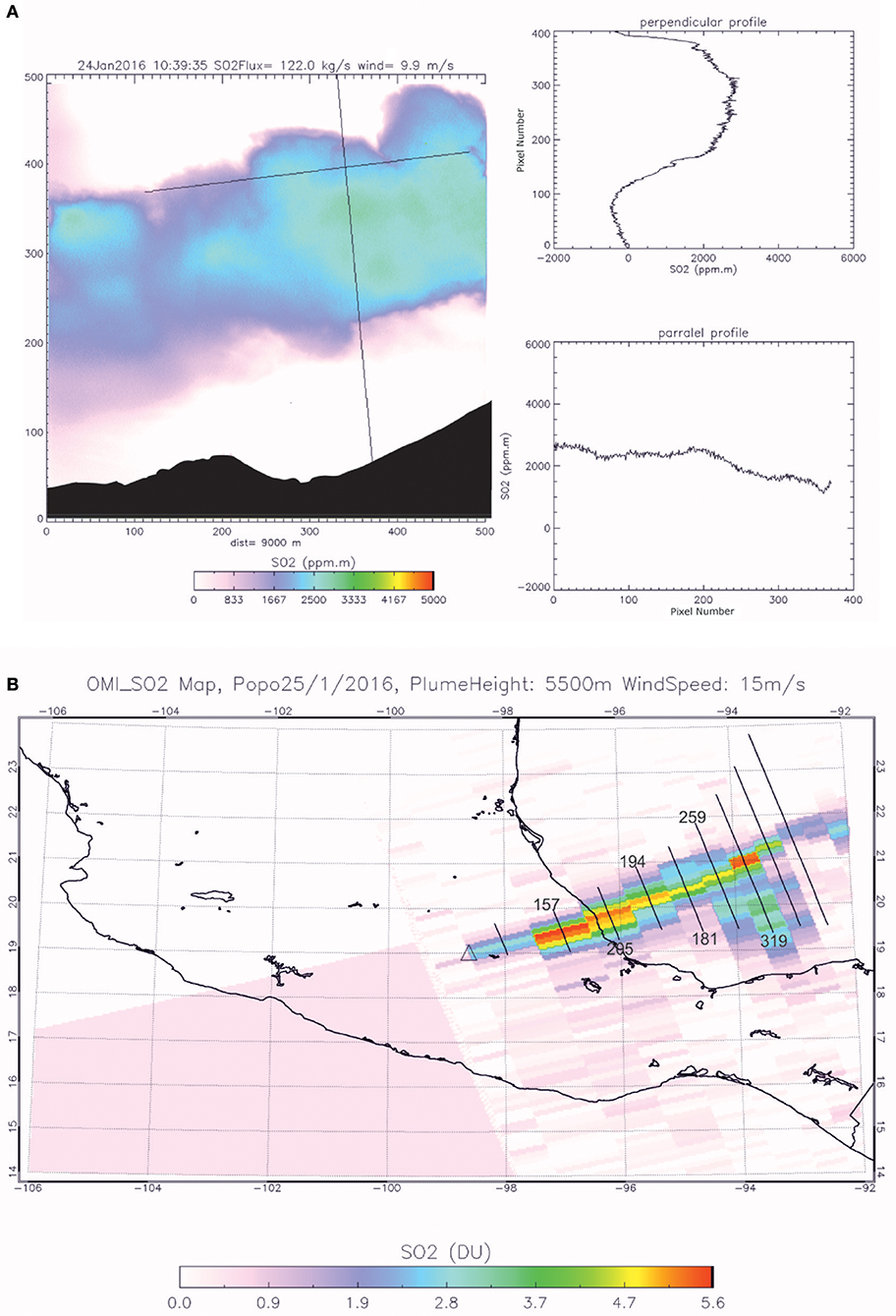
Figure 6. Measurements of the SO2 flux during an ash venting and dome growth episode. Measurements with the SO2 camera (A) show an underestimation compared to measurements obtained by processing the OMI image of the following day (B) using the traverse method (Campion, 2014). The SO2 flux, in kg/s, measured on each traverse downwind of the plume is annotated next to its corresponding traverse.
Discussion
Comparison With Previous Studies at Popocatépetl Volcano
In this section we compare our SO2 measurements with the previous published studies, summarized in Table 2. Delgado-Granados et al. (2001) and Delgado-Granados (2008) have reported measurements of the SO2 flux with a COSPEC during the earliest part of the current eruptive period. Their measurements had a long-term average of 100 kg/s (8,600 tons/day) and ranged from 10 to 1,500 kg/s during the most intense volcanic activity, in December 2000-January 2011. Grutter et al. (2008) reported results from a 3-week long multidisciplinary campaign in March 2006, involving Mobile DOAS traverses, COSPEC traverses and a fixed scanning DOAS instrument. The average SO2 flux values resulting from their study was 28 kg/s (2,450 tons/day). Lübcke et al. (2013) measured the SO2 flux using an SO2 camera. They reported an average flux of 13 kg/s (1,120 tons/day), without light dilution correction. These last two studies were made when Popocatépetl was in a notably lower state of activity than during 1997–2003 or since 2012. Finally those two last studies and our results are systematically higher, by a factor of about two, than the corresponding yearly-averaged fluxes computed by Carn et al. (2017) using the images of OMI. We suspect that the cause of this discrepancy lies in the turbidity and thickness of the boundary layer (the three lowermost kilometers of the troposphere where most of the water vapor and aerosols reside) over central Mexico, which alters the radiative transfer and the air mass factor compared to the model parameters used in OMI retrievals.
Comparison With Other Volcanoes
The long-term average of our SO2 emission rate measurements at Popocatépetl, 45 kg/s, places the volcano as one of the five strongest permanent emitters of volcanic SO2 over 2013–2016, together with Ambrym (100 kg/s; Allard et al., 2016), Nevado del Ruiz (20–80 kg/s; Lübcke et al., 2014), Kilauea (10–60 kg/s; Nadeau et al., 2014), and Nyamuragira (20–60 kg/s; Coppola et al., 2016). It should be noted that over the considered period, Popocatépetl has been a stronger SO2 emitter than other volcanoes well known for their strong degassing such as Etna, Masaya, or Nyiragongo.
Relatively few studies are available on high time resolution SO2 measurements at dome volcanoes with intermittent explosive activity. Fischer et al. (2002) investigated the degassing of Karymsky volcano and reported very low passive emission of SO2, interrupted by short period of higher flux (up to 3 kg/s) associated to mild explosive activity. They attributed this pattern to the pressurization of the upper conduit beneath a lava plug that quickly sealed after releasing its pressure through explosive degassing. They could distinguish two types of explosions, those followed by a rapid return of the SO2 emission to their very low background level and those followed by a gradual, waxing and waning decay toward background. Smekens et al. (2015) reported the very same type of behavior at Semeru volcano (Indonesia). Holland et al. (2011) measured slightly higher inter-explosive SO2 emissions at Santiaguito (Guatemala) and gave a different interpretation of the degassing mechanism, calling forth enhanced exsolution and release of gas through ring fractures during the stick-slip upwards motion of the lava plug. The pattern reported in this study differs significantly from all those other dome-bearing volcanoes. The SO2 emissions of Popocatépetl are two orders of magnitude higher than at Semeru, Karimsky and Santiaguito and unlike these volcanoes, are sustained permanently. Even the maximal SO2 fluxes measured at the above-mentioned volcanoes during explosive activity is still largely inferior to the emission rates emitted by Popocatépetl between two puffs of purely passive degassing. Such high and persistent gas flux values imply that sealing of the conduit does usually not occur at Popocatépetl and the degassing models developed for the afore mentioned volcanoes may not be applied.
Measurements of SO2 at the actively growing lava dome of Soufriere Hills volcano (e.g., Young et al., 2003) showed significantly higher SO2 fluxes (typically 3–15 kg/s) than for those three former volcanoes. The fluxes were sometimes following well-defined periodic cycles of several hours, which were interpreted as being caused by the pressurization of the magma conduit and associated to changes of extrusion rate. The degassing of Popocatépetl, which is nearly one order of magnitude stronger than that of Soufriere Hills is also different in its behavior because the fluctuations of the SO2 flux at Popocatépetl are much faster (a few minutes vs. several hours) and not associated to the growth of the lava dome.
SO2 camera measurements at Sakura-jima volcano (Kazahaya et al., 2016) have shown a very similar pattern to the one reported here for Popocatépetl, with high, sustained flux and occasional short-lived drops preceding explosions. Although Sakura-jima does not often build volcanic domes, geophysical and gas measurements have been inferred to be modulated by the temporary formation and destruction of lava plugs (Iguchi et al., 2008; Kazahaya et al., 2016), which can be viewed as lava domes at embryonic stages. Visual observations suggest that a number of volcanoes in the world share a similar behavior to Popocatépetl, among which Tungurahua (Ecuador, Hall et al., 2015), Ubinas and Sabancaya (Peru, Author's observations), Dukono and Agung (Indonesia, Syahbana, pers. com.) and Nevado del Ruiz (Colombia, Chacón-Ortíz, pers. com.).
Origin of the Periodic Puffing
Periodicity in passive degassing has been observed in time series of SO2 fluxes of many other volcanoes, such as Stromboli (period of around 1 s, Tamburello et al., 2012), Turrialba (period of about 100 s, Campion et al., 2012), Erebus (period of 500–1,000 s, Boichu et al., 2010) and Etna (Tamburello et al., 2013). Two processes can be envisaged as a cause of the puffing: pulsating release of gas directly at the vent area or turbulent entrainment of atmospheric air when the hot gases mix with the colder atmosphere. Moussallam et al. (2016) have argued that since turbulence is a chaotic process, it should not produce periodical puffs. However, a chaotic behavior can include, time to time, intermittency that means periods of regular and/or periodic behavior. The pulsating behavior is already present when measured on a transect drawn very close (200 m) to the crater rim, where it is actually stronger and better-defined (Figure 7). This argues against the hypothesis that the puffing is a transport effect (Tamburello et al., 2013). In the case of Popocatépetl, we propose that the puffing likely has a volcanic origin because its regularity and its characteristic frequency are independent of the climatic conditions. A strong argument in favor of the volcanic origin, is provided by the higher altitude reached by the distinct gas pulses (Figure 3A), which results from a higher thermal energy of the puffs. The more energetic release of the puffs is well perceptible in Video 1.
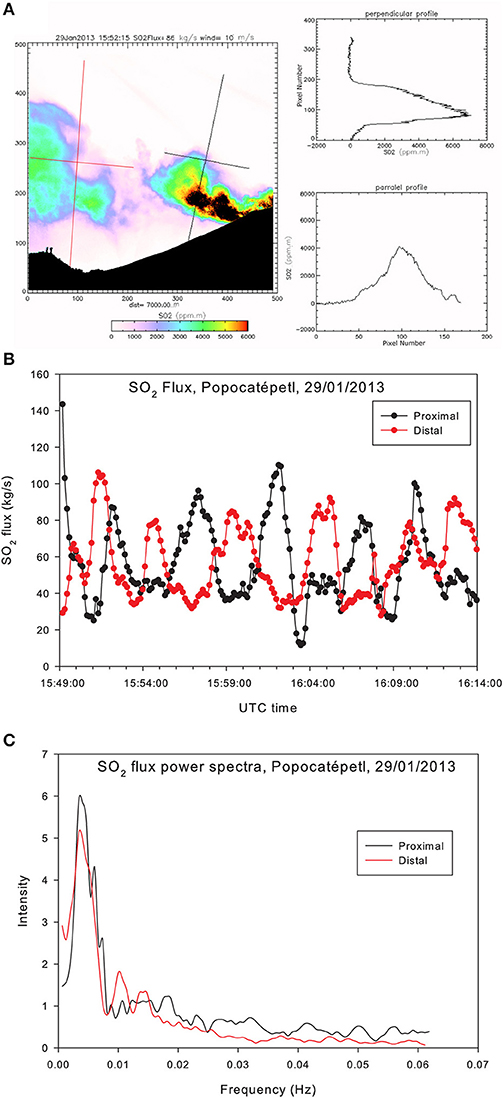
Figure 7. Comparison between SO2 flux measurements obtained very close and further downwind of the volcano. (A) Distribution map of the SO2 in the plume, with the proximal transect shown in black (traced ~200 m downwind of the crater), and the distal transect shown in red (~2.3 km from the crater). (B) Respective time series of SO2 measured on the two transects. (C) Respective power spectra computed from these time series. The spectra of the distal time series shows a less prominent peak at the puffing frequency, and higher power distribution at the lower frequencies, indicating a smoothing of the puffs with transport.
Permeable Dome and Gas Transfer Mechanisms
Similarly to the earlier (1994–2003) stages of the current eruptive period that started in 1994 and is ongoing as of 2018 (Delgado-Granados et al., 2001; Delgado-Granados, 2008), the SO2 flux measured during the reporting period (2013–2016) is more than an order of magnitude too high to result solely from the degassing of the erupted magma. The estimated ~107 m3 of magma emitted over the 2013–2016 should have produced an average SO2 flux of at least 0.8–1.8 kg/s, estimated assuming the complete degassing of a primitive magma having a density of 2.5 g/cm3 and containing an initial S content of 2,500 ppm (Witter et al., 2005; Roberge et al., 2009). This is much smaller than the average value of 45 kg/s measured over the whole survey period. It is thus clear that the degassing of the sulfur from the magma is taking place in the deeper part of the magmatic system (>10 km according to Roberge et al., 2009) and that < 2% of the intruded magma reaches the surface, while the gas that this magma produces is efficiently transferred through the conduit system. Our results show that the whole conduit system of Popocatépetl volcano is essentially permeable to this deep gas flow, whether being capped by a lava dome or not. This is supported by airborne observations that the domes are affected by numerous fractures that, together with the dome-conduit boundary, let the gas escape freely to the atmosphere. It is likely that this fracture-network permeability develops as early as the growth stage of the dome. The gas transfer mechanism within the deep conduit system is not known with certainty, magma convection, and gas fluxing within interconnected vesicles being the most likely candidates. These mechanisms are not mutually exclusive. Depending on the magma viscosity, vesicularity, and percentage of interconnected vesicles, each of them may dominate at certain depths or time.
Immediately beneath the dome and in the upper part of the magma column, the gas transfer is probably achieved through a network of highly connected vesicles (e.g., Burgisser and Gardner, 2005; Schipper et al., 2013) that pervades the highly viscous magma in prolongation of the fractures of the dome. At Popocatépetl volcano, the magma column in the upper conduit is stalled for much of the time, except during the relatively infrequent and short episodes of dome growth. Thus, magma shearing cannot be invoked as a factor that helps maintaining the bubble network connected, as it has been inferred from laboratory experiments (Okumura et al., 2006) and field/sample studies (Schipper et al., 2013). The absence of magma movement also excludes the stick-slip mechanisms and the associated repeated fracturing of the magma/conduit interface that is thought to foster relatively quiet degassing in lava dome eruption (e.g., Holland et al., 2011). Therefore, the gas flow pressure is the only mechanism that may explain that the fracture networks in the shallow dome and the vesicle networks in the upper magma column below the dome stay open and permeable. The continuous fluxing of pressurized gases from depth is maintaining the vesicles network and fracture network of the upper conduit, acting against lithostatic pressure that tends to compact and close the system. Pressure oscillations resulting of the opposition between these two forces may be the cause of the puffing behavior of the passive degassing. An increase in magma pressure or an upward movement of the underlying magma column could also theoretically promote the compaction of the upper conduit system, but we believe that the gas flow would increase accordingly, maintaining the permeable networks open. In addition, if the compaction of the upper conduit was due to an increase of the magma pressure, the explosions would be followed by an episode of magma emission at the surface, once the dome is destroyed and is no more an obstacle to the further rise of magma, which has not been observed. At higher depth, the magma should be less viscous and support bubble flow and/or magma convection to transport gas toward the surface, but more work on the depth-dependence of the magma viscosity is needed to identify the gas transfer mechanism in the deep conduit system.
The E1 Explosion: Percolating Slugs or Bigger Than Normal Puffs?
In this section, we discuss the possible origin of the E1 explosions. E1 explosions usually produce small plumes with relatively little ash, allowing sometimes to quantify the SO2 with only a modest underestimation. These explosions occur in the midst of the normal degassing and are characterized in the SO2 flux time series by a spike lasting a few minutes followed by a rapid return to the pre-explosion flux values. This SO2 flux pattern associated with the E1 explosions is similar to the explosions of Stromboli volcano (Tamburello et al., 2012), which are thought to be caused by the bursting of gas slugs ascending through the conduit system (e.g., Vergniolles et al., 1996; Burton et al., 2007). Based on this similarity, E1 explosions could be caused by the ascent of slugs through the deep conduit and their successive percolation through the dome. Instead of bursting at a free magma interface like in Stromboli and other strombolian volcanoes, a gas slug reaching the upper part of Popocatépetl volcano would have to percolate through the interconnected vesicles zone and through the fractured dome. The increase of SO2 associated with the E1 explosions is emergent rather than impulsive, which is consistent with the percolation of the gas slug rather than its bursting. The gas masses calculated for E1 explosions are about two orders of magnitudes larger than the SO2 masses emitted by the typical strombolian explosions in Stromboli (Mori and Burton, 2009; Tamburello et al., 2012; Delle Donne et al., 2016) but this scales generally with the difference in the SO2 flux of the two volcanoes.
The SO2 masses released by the E1 explosions, although likely underestimated, are higher than those released by individual puffs, but not completely out of their range, as shown in the histogram (Figure 8). This suggests the alternative hypothesis that E1 explosions might share a common process of formation with the puffs, and be actually larger or more energetic puffs involving coalescence events and fragmentation in the interconnected vesicles zone.
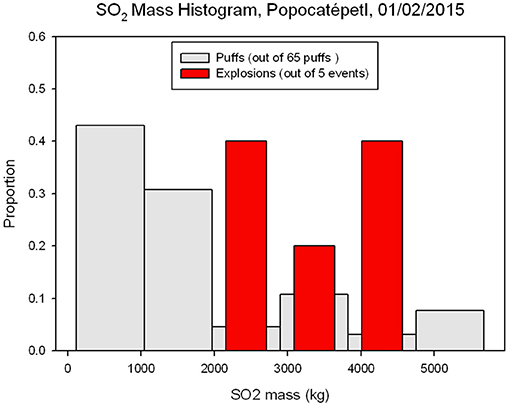
Figure 8. Histogram comparing the frequency distribution of the SO2 masses emitted by individual puffs of passive degassing and by E1 explosions, on a same day.
A New Model for the Explosion Mechanism and Triggering
Vulcanian explosions at Popocatépetl have been proposed by various authors (Love et al., 2000; Schaaf et al., 2005; Stremme et al., 2011; Gómez-Vazquez et al., 2016) to be caused by the gas accumulation below a dome that is cooling until it plugs the conduit. Positive feedback between crystallization and degassing in the shallow magma column was also invoked (Stix et al., 1997; Schaaf et al., 2005) to produce the overpressure necessary for the strong vulcanian explosions. Arguments in favor of this model were:
1) Most vulcanian explosions postdate the growth of large lava domes in the crater and destroy them partially or totally (Gómez-Vazquez et al., 2016).
2) Increased SiF4/SO2 ratio in the gas plume before and during the explosions (Love et al., 2000; Stremme et al., 2011; Taquet et al., 2017). SiF4 is a relatively little abundant gas which is formed by reaction.
whose equilibrium is displaced to the right at low temperature (Symonds and Reed, 1993). Love et al. (2000) and Stremme et al. (2011) interpreted the increase of SiF4 to result from colder equilibrium temperature of the gas, and to record the cooling of the lava dome. However, the equilibrium temperatures calculated by these authors were unrealistically low (150–180°C) and in contradiction with the continuous incandescence observed in the crater at night.
However, our results and other observations do not support the cooling and crystalizing model of the explosions generation. These are:
1) Our measurements show that the emplaced domes are permeable to a high flux of gas for long and variable periods after their emplacement.
2) The drop of the gas flux before E2 explosions is rapid, not more than a few minutes to a few tens of minutes, while cooling, crystallization and solidification of lava domes require periods of weeks to years depending on their volume (e.g., Hicks et al., 2009).
3) The time between dome emplacement and its destruction is highly variable from a few days to several years (Gómez-Vazquez et al., 2016), and shows no correlation with the size of the domes, whereas it should do if the cooling of the domes was responsible for their loss of permeability. Larger domes should take a much longer time than smaller ones to cool and achieve the low enough permeability that would supposedly lead to their destruction.
4) The domes are often destroyed not by a single explosion but by a series of several ones that occur over a period of a few days, and destroy the dome incrementally.
The model, illustrated in Figure 9, that we propose for the generation of the E2 explosions also assumes accumulation of gas before the explosions, but differs in the cause of this accumulation. It accounts for the above-mentioned observations as well as for those in favor of the old cooling and crystalizing model. In our model, the accumulation of the gas is due to a compaction of the permeable networks that normally allows the gas to flow through the upper conduit and dome. This lithostatic squeezing leads to a dramatic decrease of the upper conduit permeability, which promotes the accumulation of the deep gas until it reaches enough pressure to disrupt the blockage through an explosion. Laboratory experiments of uniaxial, gravitational compaction of rhyolitic magmas by Okumura and Sasaki (2014) have shown drastic decreases of permeability achieved in timescales of 100–1,000 s. These timescales are strikingly similar to the decrease in SO2 flux that we observe preceding E2 explosions, and way faster than any other mechanisms able to decrease permeability, such as cooling or mineral deposition in fractures and pores. An additional argument in favor of our model of compacting-induced explosions is that inward sagging and deflation of the dome have often been observed at Popocatépetl during occasional surveillance overflights, although their infrequence hampers to establish a univocal systematic time correlation between these phenomena and the explosions (Centro Nacional de Prevención de Desastre, 1995). Since we have shown in section Origin of the Periodic Puffing that the high pressure of the gas flow is the main factor that maintains open the fracture network in the dome and the vesicle network in the upper magma column a slight reduction of the gas flux would leave these permeable networks unsupported and would allow the lithostatic pressure and the weight of the dome to compact them, initiating the gas accumulation. One of the key observations invoked to support the earlier cooling and crystalizing dome model was the increased SiF4/SO2 ratio in the emissions before and during an explosion (Love et al., 2000; Stremme et al., 2011). However, thermodynamic data reported by De Hoog et al. (2005) for equation (1) show that the pressure dependence of this equilibrium is actually much stronger than its temperature dependence, especially at the pressures corresponding to a shallow magmatic column. More recently, Taquet et al. (2017) measured the SiF4/SO2 ratio over a period of several months and reported increases so large and so fast associated with explosive events that they are explained much more convincingly by an increase of the equilibrium pressure of the emitted gas than by a decrease of its equilibrium temperature. Our model readily explains this increase of equilibrium pressure by the pressurization of the gas rapidly accumulating below a gravity-compacted dome and underdome. A similar dynamics has been proposed to explain the eruptive behavior of Lascar volcano (Northern Chile) between 1984 and 1994, which was characterized by high gas fluxes, and cycles of building of low aspect ratio lava domes, decreasing of the degassing, subsidence of the dome and strong vulcanian explosions (Matthews et al., 1997). If our model is correct, then E2 explosions should be preceded by a small transient deflationary signal in the tilt accompanying the dome compaction, followed by a slow inflation corresponding to the phase of gas accumulation and finally a rapid deflation associated with the explosive decompression of the upper conduit system. Due to the relatively superficial origin inferred here for the E2 explosions, it would be important to place tiltmeters as high and close to the crater as possible.
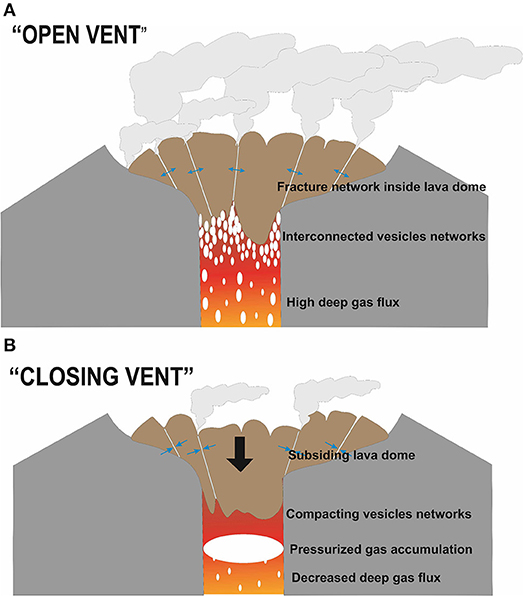
Figure 9. (A,B) Conceptual model of the upper conduit of Popocatépetl volcano (vertical scale not respected). In the deep conduit, the magma is likely fluid enough to support conduit convection (Witter et al., 2005) and/or bubble flow. In the upper conduit, the rheology is likely too viscous to allow flowing, but a high degree of vesicularity, likely inherited from the vesiculation of the magma at the time of the dome emplacement, allows the gas to flow through a network of interconnected vesicles. In the dome, fractures and tuffisite veins (e.g., Kendrick et al., 2016), also inherited from the dome formation stage, form a permeable network for the gas flow at a shallower level.
Conclusion and Future Work
SO2 camera measurements at Popocatépetl confirm that this volcano emits extraordinarily high SO2 fluxes despite having its crater occupied most of the time by a stalled lava dome. This implies that this lava dome and the underlying upper conduit are mostly permeable to the flux of gas coming from the deeper parts of the magmatic system. This high permeability is maintained for long periods (up to several months) despite the absence of magma motion in the conduit, which has been often invoked as a factor enhancing the permeability of the magma-conduit interface. We thus propose that the gas flux is maintaining open the fracture network in the dome and the interconnected vesicles network below it. These permeable networks, however, can close rapidly through compaction if the gas flux slightly decreases, causing gas accumulation and pressurization that eventually leads to an explosion. The puffing and the frequent E1 explosions maintain the upper conduit permeable, while the E2 explosions restore its permeability when it drops due to compaction. Future work toward a more complete understanding of the degassing dynamics should include the installation of a web camera on the crater rim, to investigate the distribution of the degassing vents inside the crater, and the time relationship between the inferred dome subsidence and explosions. Installation of close-field tiltmeters would also help to validate our new model for the generation of E2 explosions and to constrain the depth of the gas accumulation. Infrasound measurements would help to elucidate the origin of the puffing, which we tentatively attribute to pressure oscillations in the gas flow through the permeable networks. Measurements of the gas composition could be performed more systematically to elucidate the origin of the E1 explosions. The recognition of two different types of explosions and the hypothesis we formulate on their mechanism could form a process-based fundament for the seismic-based distinction between exhalation and explosion (De la Cruz-Reyna and Tilling, 2008). Finally, our model of explosion generation by rapid compaction of the upper magma column is applicable to other andesitic volcanoes that exhibit sustained gas emissions and undergo frequent, rapid transitions to explosive activity, such as Tungurahua, Ubinas, Sabancaya, Nevado del Ruiz, Sakura-jima and Dukono. We suggest that at those volcanoes, similarly to what happens at Popocatépetl, a decrease in the gas flux could actually foster the lithostatic compaction of the upper magma column and trigger a transition from passive degassing toward more intermittent and violent release of gas through the so-called vulcanian explosions.
Author Contributions
RC make the measurements in the field, wrote the code for processing the data, interpreted the data, wrote the manuscript. HD-G impulsed this research, NT and SP-E took part to the fieldwork. SP-E contributed to the code for processing the data. TL wrote the code for operating the Camera. All discussed the data and their interpretation and revised the manuscript.
Conflict of Interest Statement
The authors declare that the research was conducted in the absence of any commercial or financial relationships that could be construed as a potential conflict of interest.
Acknowledgments
This research was funded thanks to the proyect Papiit IA103816. We thank Servando de la Cruz-Reyna and Yuri Taran for fruitful discussions. Two reviewers as well as editors Tarsilo Girona and Valerio Acocella are thanked for helping to improve the manuscript.
Supplementary Material
The Supplementary Material for this article can be found online at: https://www.frontiersin.org/articles/10.3389/feart.2018.00163/full#supplementary-material
References
Allard, P., Aiuppa, A., Bani, P., Métrich, N., Bertagnini, A., et al. (2016). Prodigious emission rates and magma degassing budget of major, trace and radioactive volatile species from Ambrym basaltic volcano, Vanuatu island Arc. J. Volcanol. Geother. Res. 322, 119–143. doi: 10.1016/j.jvolgeores.2015.10.004
Boichu, M., Oppenheimer, C., Tsanev, V., and Kyle, P. R. (2010). High temporal resolution SO2 flux measurements at Erebus volcano, Antarctica. J. Volcanol. Geotherm. Res. 190, 325–336. doi: 10.1016/j.jvolgeores.2009.11.020
Boudon, G., Balcone-Boissard, H., Villemant, B., and Morgan, D. J. (2015). What factors control superficial lava dome explosivity? Sci. Rep. 5:14551. doi: 10.1038/srep14551
Burgisser, A., and Gardner, J. E. (2005). Experimental constraints on degassing and permeability in volcanic conduit flow. Bull. Volcanol. 67, 42–56. doi: 10.1007/s00445-004-0359-5
Burton, M., Allard, P., Muré, F., and La Spina, A. (2007). Magmatic gas composition reveals the source depth of slug-driven Strombolian explosive activity. Science 317, 227–230 doi: 10.1126/science.1141900
Campion, R. (2014). New lava lake at Nyamuragira volcano revealed by combined ASTER and OMI SO2 measurements. Geophys. Res. Lett. 41, 7485–7492, doi: 10.1002/2014GL061808
Campion, R., Delgado-Granados, H., and Mori, T. (2015). Image-based correction of the light dilution effect for SO2 camera measurements. J. Volcanol. Geother. Res. 300, 48–57 doi: 10.1016/j.jvolgeores.2015.01.004
Campion, R., Martinez-Cruz, M., Lecocq, T., Caudron, C., Pacheco, J., Pinardi, G., et al. (2012). Space- and ground-based measurements of sulphur dioxide emissions from Turrialba Volcano (Costa Rica). Bull. Volcanol. 74, 1757–1770. doi: 10.1007/s00445-012-0631-z
Carn, S. A., Fioletov, V. E., McLinden, C. A., Li, C., and Krotkov, N. A. (2017). A decade of global volcanic SO2 emissions measured from space. Sci. Rep. 7:44095. doi: 10.1038/srep44095
Carn, S. A., Krueger, A. J., Arellano, S., Krotkov, N. A., and Yang, K. (2008). Daily monitoring of Ecuadorian volcanic degassing from space. J. Volcanol. Geotherm. Res. 176:141–150. doi: 10.1016/j.jvolgeores.2008.01.029
Centro Nacional de Prevención de Desastre (1995). CENAPRED Reports, Centro Nacional de Prevención de Desastre. Available online at: http://www.cenapred.unam.mx:8080/reportesVolcanGobMX/
Collinson, A. S. D., and Neuberg, J. W. (2012). Gas storage, transport and pressure changes in an evolving permeable volcanic edifice. J. Volcanol. Geotherm. Res. 243–244, 1–13. doi: 10.1016/j.jvolgeores.2012.06.027
Coppola, D., Campion, R., Laiolo, M., Cuoco, C., Balagizi, C., Ripepe, M., et al. (2016). Birth of a lava lake: Nyamulagira volcano 2011–2015. Bull. Volcanol. 78, 1–13. doi: 10.1007/s00445-016-1014-7
De Hoog, J. C. M., Van Bergen, M. J., and Jacobs, M. H. G. (2005). Vapour-phase crystallisation of silica from SiF4-bearing volcanic gases. Ann. Geophys. 48, 775–785. doi: 10.4401/ag-3233
De la Cruz-Reyna, S., and Siebe, C. (1997). The giant Popocatépetl stirs. Nature 388:227. doi: 10.1038/40749
De la Cruz-Reyna, S., and Tilling, R. I. (2008). Scientific and public responses to the ongoing volcanic crisis at Popocatépetl Volcano, Mexico: importance of an effective hazards-warning system. J. Volcanol. Geotherm. Res. 170, 121–134. doi: 10.1016/j.jvolgeores.2007.09.002
Delgado Granados, H., and Jenkins, S. (2016). “Chapter 13 - extreme volcanic risks 1: Mexico City,” in Volcanic Hazards, Risks and Disasters, eds P. Papale, and J. F. Shroder (Elsevier), 315–354.
Delgado-Granados, H. (2008). “Large Volcanic- SO2 fluxes: COSPEC measurements at Popocatépetl Volcano (Mexico),” in The COSPEC Cookbook, eds G. Williams-Jones, J. Stix, and C. Hickson (IAVCEI, Special Publications).
Delgado-Granados, H., González, L. C., and Sánchez, N. P. (2001). Sulfur dioxide emissions from Popocatépetl volcano (Mexico): case study of a high-emission rate, passively degassing erupting volcano. J. Volcanol. Geother. Res. 108, 107–120. doi: 10.1016/S0377-0273(00)00280-8
Delle Donne, D., Ripepe, M., Lacanna, G., Tamburello, G., Bitetto, M., and Aiuppa, A. (2016). Gas mass derived by infrasound and UV cameras: implications for mass flow rate. J. Volcanol. Geotherm. Res. 325, 169–178. doi: 10.1016/j.jvolgeores.2016.06.015
Espinasa-Pereña, R., and Martín-Del Pozzo, A. L. (2006). “Morphostratigraphic evolution of Popocatépetl volcano, México,” in Neogene-Quaternary continental margin volcanism: A perspective from Mexico: Geological Society of America Special Paper 402, eds C. Siebe, J. L. Macías, and G. J. Aguirre-Díaz (Geological Society of America Special), 101–123.
Farquharson, J., Heap, M. J., Varley, N. R., Baud, P., and Reuschlé, T. (2015). Permeability and porosity relationships of edifice-forming andesites: a combined field and laboratory study. J. Volcanol. Geotherm. Res. 297, 52–68. doi: 10.1016/j.jvolgeores.2015.03.016
Fioletov, V. E., McLinden, C. A., Krotkov, N. A., and Li, C. (2015). Lifetimes and emissions of SO2 from point sources estimated from OMI. Geophys. Res. Lett. 42, 1969–1976. doi: 10.1002/2015GL063148
Fischer, T. P., Roggensack, K., and Kyle, P. R. (2002). Open and almost shut case for explosive eruptions: vent processes determined by SO2 emission rates at Karymsky volcano, Kamchatka. Geology 30, 1059–1062. doi: 10.1130/0091-7613(2002)030<1059:OAASCF>2.0.CO;2
Gaunt, H. E., Sammonds, P. R., Meredith, P. G., Smith, R., and Pallister, J. S. (2014). Pathways for degassing during the lava dome eruption of Mount St. Helens 2004–2008. Geology 42, 947–950. doi: 10.1130/G35940.1
Gómez-Vazquez, A., De la Cruz-Reyna, S., and Mendoza-Rosas, A. T. (2016). The ongoing dome emplacement and destruction cyclic process at Popocatépetl volcano, central Mexico. Bull. Volcanol. 78, 2–15. doi: 10.1007/s00445-016-1054-z
Grutter, M., Basaldud, R., Rivera, C., Harig, R., Junkerman, W., Caetano, E., et al. (2008). SO2 emissions from Popocatepetl volcano: emission rates and plume imaging using optical remote sensing techniques. Atmos. Chem. Phys. 8, 6655–6663 doi: 10.5194/acp-8-6655-2008
Hall, M. L., Steele, A. L., Bernard, B., Mothes, P. A., Vallejo, S. X., Douillet, G. A., et al. (2015). Sequential plug formation, disintegration by Vulcanian explosions, and the generation of granular Pyroclastic Density Currents at Tungurahua volcano (2013–2014), Ecuador. J. Volcanol. Geother. Res. 306, 90–103. doi: 10.1016/j.jvolgeores.2015.09.009
Hicks, P. D., Matthews, A. J., and Cooker, M. J. (2009). Thermal structure of a gas-permeable lava dome and timescale separation in its response to perturbation. J. Geophys. Res. 114:B07201. doi: 10.1029/2008JB006198
Holland, P., Watson, I., Phillips, J., Caricchi, L., and Dalton, M. (2011). Degassing processes during lava dome growth: insights from Santiaguito lava dome, Guatemala. J. Volcanol. Geotherm. Res. 202, 153–166. doi: 10.1016/j.jvolgeores.2011.02.004
Iguchi, M., Yakiwara, H., Tameguri, T., Hendrasto, M., and Hirabayashi, J. (2008). Mechanism of explosive eruption revealed by geophysical observations at the Sakurajima, Suwanosejima and Semeru volcanoes. J. Volcanol. Geotherm. Res. 178, 1–9. doi: 10.1016/j.jvolgeores.2007.10.010
Kazahaya, R., Shinohara, H., Mori, T., Iguchi, M., and Yokoo, A. (2016). Preeruptive inflation caused by gas accumulation: insight from detailed gas flux variation at Sakurajima volcano, Japan. Geophys. Res. Lett. 43, 11219–11225. doi: 10.1002/2016GL070727
Kendrick, J. E., Lavallée, Y., Varley, N. R., Wadsworth, F. B., Lamb, O. D., and Vasseur, J. (2016). Blowing off steam: tuffisite formation as a regulator for lava dome eruptions. Front. Earth Sci. 4:41. doi: 10.3389/feart.2016.00041
Kern, C., Deutschmann, T., Vogel, L., Wöhrbach, M., Wagner, T., and Platt, U. (2010a), Radiative transfer corrections for accurate spectroscopic measurements of volcanic gas emissions. Bull. Volcanol. 72, 233–247. doi: 10.1007/s00445-009-0313-7
Kern, C., Kick, F., Lübcke, P., Vogel, L., Wöhrbach, M., and Platt, U. (2010b). Theoretical description of functionality, applications, and limitations of SO2 cameras for the remote sensing of volcanic plumes. Atmos. Meas. Tech. 3, 733–749. doi: 10.5194/amt-3-733-2010
Kern, C., Lübcke, P., Bobrowski, N., Campion, R., Mori, T., Smekens, J-F., et al. (2015). Intercomparison of SO2 camera systems for imaging volcanic gas plumes. J. Volcanol. Geotherm. Res. 300, 22–36. doi: 10.1016/j.jvolgeores.2014.08.026
Love, S. P., Goff, F., Schmidt, S. C., Counce, D., Pettit, D., Christenson, B. W., et al. (2000). “Passive infrared spectroscopic remote sensing of volcanic gases: ground-based studies at White Island and Ruapehu, New Zealand, and Popocatépetl, Mexico,” in Remote Sensing of Active Volcanism, eds P. J. Mouginis-Mark, J. A. Crisp, and J. H. Fink (Washington, DC: American Geophysical Union).
Lübcke, P., Bobrowski, N., Arellano, S., Galle, B., Garzón, G., Vogel, L., et al. (2014). BrO/SO2 molar ratios from scanning DOAS measurements in the NOVAC network. Solid Earth 5, 409–424. doi: 10.5194/se-5-409-2014
Lübcke, P., Bobrowski, N., Illing, S., Kern, C., Alvarez Nieves, J. M., Vogel, L., et al. (2013). On the absolute calibration of SO2 cameras. Atmos. Meas. Tech. 6, 677–696. doi: 10.5194/amt-6-677-2013
Martin-Del Pozzo, A. L., Cifuentes, G., Cabral-Cano, E., Bonifaz, R., Correa, F., and Mendiola, I. F. (2003). Timing magma ascent at Popocatépetl Volcano, Mexico, 2000-2001. J. Volcanol. Geotherm. Res. 125, 107–120. doi: 10.1016/S0377-0273(03)00091-X
Matthews, S., Gardeweg, M., and Sparks, R. (1997). The 1984 to 1996 cyclic activity of Lascar Volcano, northern Chile: cycles of dome growth, dome subsidence, degassing and explosive eruptions. Bull. Volcanol. 59, 72–82. doi: 10.1007/s004450050176
Mori, T., and Burton, M. (2006). The SO2 camera: a simple, fast and cheap method for ground-based imaging of SO2 in volcanic plumes. Geophys. Res. Lett. 33:L24804. doi: 10.1029/2006gl027916
Mori, T., and Burton, M. (2009). Quantification of the gas mass emitted during single explosions on Stromboli with the SO2 imaging camera. J. Volcanol. Geotherm. Res. 188, 395–400. doi: 10.1016/j.jvolgeores.2009.10.005
Mori, T., Mori, T., Kazahaya, K., Ohwada, M., Hirabayashi, J., and Yoshikawa, S. (2006). Effect of UV scattering on SO2 emission rate measurements. Geophys. Res. Lett. 33:L17315. doi: 10.1029/2006GL026285
Moussallam, Y., Bani, P, Curtis, A., Barnie, T., Moussallam, M., Peters, N., et al. (2016). Sustaining persistent lava lakes: observations from high-resolution gas measurements at Villarrica volcano, Chile. Earth Planet. Sci. Lett. 454, 237–247. doi: 10.1016/j.epsl.2016.09.012
Nadeau, P. A., Werner, C. A., Waite, G. P., Carn, S. A., Brewer, I. D., Elias, T., et al. (2014). Using SO2 camera imagery and seismicity to examine degassing and gas accumulation at Kilauea Volcano, May 2010. J. Volcanol. Geotherm. Res. 300, 70–80. doi: 10.1016/j.jvolgeores.2014.12.005
Okumura, S., Nakamura, M., and Tsuchiyama, A. (2006). Shear-induced bubble coalescence in rhyolitic melts with low vesicularity. Geophys. Res. Lett. 33:L20316. doi: 10.1029/2006GL027347
Okumura, S., and Sasaki, O. (2014). Permeability reduction of fractured rhyolite in volcanic conduits and its control on eruption cyclicity. Geology 42, 843–846. doi: 10.1130/G35855.1
Roberge, J., Delgado Granados, H., and Wallace, P. (2009). Mafic magma recharge supplies high CO2 and SO2 gas fluxes from Popocatépetl volcano, México. Geol. Geol. Soc. Am. 37, 107–110. doi: 10.1130/G25242A.1
Schaaf, P., Stimac, J., Siebe, C., and Macías, J. L. (2005). Geochemical evidence for mantle origin and crustal processes in volcanic rocks from Popocatépetl and surrounding monogenetic volcanoes, Central Mexico. J. Petrol. 46, 1243–1282. doi: 10.1093/petrology/egi015
Schipper, C. I., Castro, J. M., Tuffen, H., James, M. R., and How, P. (2013). Shallow vent architecture during hybrid explosive-effusive activity at Cordón Caulle (Chile, 2011–12): evidence from direct observations and pyroclast textures. J. Volcanol. Geotherm. Res. 262, 25–37. doi: 10.1016/j.jvolgeores.2013.06.005
Siebe, C., Abrams, M., Macías, J. L., and Obenholzner, J. (1996). Repeated volcanic disasters in Prehispanic time at Popocatépetl, central Mexico: past key to the future? Geology 24, 399–402. doi: 10.1130/0091-7613(1996)024 < 0399:RVDIPT>2.3.CO;2
Siebe, C., and Macías, J. L. (2006). “Volcanic hazards in the Mexico City metropolitan area from eruptions at Popocatépetl, Nevado de Toluca, and Jocotitlán stratovolcanoes and monogenetic scoria cones in the Sierra Chichinautzin Volcanic Field,” in Neogene–Quaternary Continental Margin Volcanism. A Perspective from Mexico, Geological Society of America, Special Publication, Vol. 402. eds C. Siebe, J. L. Macías, and G. Aguirre (Geological Society of America Special), 253–329. doi: 10.1130/2004.VHITMC.SP402
Siebe, C., Salinas, S., Gardner, J., and Bonasia, R. (2017). The ~ 23,500 y 14 C BP White Pumice Plinian eruption and associated debris avalanche and Tochimilco lava flow of Popocatépetl volcano, México. J. Volcanol. Geother. Res. 333, 66–95 doi: 10.1016/j.jvolgeores.2017.01.011
Smekens, J.-F., Clarke, A. B., Burton, M. R., Harijoko, A., and Wibowo, H. E. (2015). J. Volcanol.Geother. Res. 300, 121–128. doi: 10.1016/j.jvolgeores.2015.01.006
Sparks, R. S. J. (1997). Causes and consequences of pressurisation in lava dome eruptions. Earth Planet. Sci. Lett. 150, 177–189. doi: 10.1016/S0012-821X(97)00109-X
Stix, J., Torres, R. C., Narváez, L. M., Cortés, G. P. J., Raigos, J. A., Castonguay, R., et al. (1997). A model of vulcanian eruptions at Galeras volcano, Colombia. J. Volcanol. Geother. Res. 77, 285–303. doi: 10.1016/S0377-0273(96)00100-X
Stremme, W., and Ortega, I. (2011). Gas composition of Popocatépetl Volcano between 2007 and 2008: FTIR spectroscopic measurements of an explosive event and during quiescent degassing. Earth and Planet. Sci. Lett. 301, 502–510. doi: 10.1016/j.epsl.2010.11.032
Symonds, R. B., and Reed, M. H. (1993). Calculation of multicomponent chemical equilibria in gas-solid-liquid systems; calculation methods, thermochemical data, and applications to studies of high-temperature volcanic gases with examples from Mount St.Helens. Am. J. Sci. 293, 758–864. doi: 10.2475/ajs.293.8.758
Tamburello, G., Aiuppa, A., Kantzas, E. P., McGonigle, A. J. S., and Ripepe, M. (2012). Passive vs. active degassing modes at an open-vent volcano (Stromboli, Italy). Earth Planet. Sci. Lett. 359, 106–116 doi: 10.1016/j.epsl.2012.09.050
Tamburello, G., Aiuppa, A., McGonigle, A. J. S., Allard, P., Cannata, A., Pering, T. D., et al. (2013). Periodic volcanic degassing behavior: the Mount Etna example. Geophys. Res. Lett. 40, 4818–4822. doi: 10.1002/grl.50924
Taquet, N., Meza Hernández, I., Stremme, W., Bezanilla, A., Grutter, M., Campion, R., et al. (2017). Continuous measurements of SiF4 and SO2 by thermal emission spectroscopy: Insight from a 6-month survey at the Popocatépetl volcano. J. Volcanol. Geother. Res. 341, 255–268. doi: 10.1016/j.jvolgeores.2017.05.009
Taran, Y., and Zelenski, M. (2014). Systematics of water isotopic composition and chlorine content in arc-volcanic gases. Geol. Soc. Spec. Publ. 410, 237–262. doi: 10.1144/SP410.5
Vergniolles, S., Brandeis, G., and Mareschal, J.-C. (1996). Strombolian explosions: 2. Eruption dynamics determined from acoustic measurements. J. Geophys. Res. 101, 20449–20466.
Witter, J. B., Kress, V. C., and Newhall, C. G. (2005). Volcán Popocatépetl, Mexico. Petrology, Magma Mixing, and Immediate Sources of Volatiles for the 1994–Present Eruption. J. Petrol. 46, 2337–2366. doi: 10.1093/petrology/egi058
Keywords: volcanic degassing, SO2 camera, Popocatépetl, lava dome, permeability, explosions
Citation: Campion R, Delgado-Granados H, Legrand D, Taquet N, Boulesteix T, Pedraza-Espitía S and Lecocq T (2018) Breathing and Coughing: The Extraordinarily High Degassing of Popocatépetl Volcano Investigated With an SO2 Camera. Front. Earth Sci. 6:163. doi: 10.3389/feart.2018.00163
Received: 02 March 2018; Accepted: 28 September 2018;
Published: 19 October 2018.
Edited by:
Társilo Girona, Jet Propulsion Laboratory, United StatesReviewed by:
Giancarlo Tamburello, Istituto Nazionale di Geofisica e Vulcanologia, ItalyAlessandro Tibaldi, Università degli studi di Milano Bicocca, Italy
Copyright © 2018 Campion, Delgado-Granados, Legrand, Taquet, Boulesteix, Pedraza-Espitía and Lecocq. This is an open-access article distributed under the terms of the Creative Commons Attribution License (CC BY). The use, distribution or reproduction in other forums is permitted, provided the original author(s) and the copyright owner(s) are credited and that the original publication in this journal is cited, in accordance with accepted academic practice. No use, distribution or reproduction is permitted which does not comply with these terms.
*Correspondence: Robin Campion, cm9iaW5AZ2VvZmlzaWNhLnVuYW0ubXg=