Experimental Approach to the Direct Interaction Between the H2O-CO2 Atmosphere and the Crust on the Earliest Earth: Implications for the Early Evolution of Minerals and the Proto-Atmosphere
- 1Key Laboratory of Gas Hydrate, Ministry of Natural Resources, Qingdao Institute of Marine Geology, Qingdao, China
- 2Laboratory for Marine Mineral Resources, Qingdao National Laboratory for Marine Science and Technology, Qingdao, China
- 3Department of Earth Sciences, The University of Hong Kong, Pokfulam, Hong Kong
Batch experiments between solid materials including komatiite, peridotite and basalt with an H2O-CO2 atmosphere were performed at temperatures from 200°C to 500°C to simulate the interaction between the new rocky crust formed after the magma ocean stage and the concurrent proto-atmosphere of the early Earth. Electron microscopic observations show that clay mineral flakes were generated in all experiments. In komatiite/peridotite reaction systems, fibrous actinolite was generated in experiments conducted at higher temperatures (>400°C). Different carbonate species were produced in experiments conducted at temperatures no higher than 400°C. Formation of these carbonates and their diverse crystal habits may indicate varied extraction rates of calcium, magnesium and SiO2 from the original ultramafic rocks resulted from different experimental temperatures. Our results imply that clay minerals and carbonates could probably be formed extensively in the early Hadean by the intense interaction between the ultramafic rocky crust and the H2O-CO2 atmosphere before the formation of the earliest ocean. Rapid sequestration of the atmospheric CO2 caused by the massive precipitation of carbonates might have led to the rapid cooling of the Earth’s atmosphere and the formation of the earliest oceans.
Introduction
The Hadean was the first eon that was critical for the Earth being evolved to a habitable planet and the origin of life. However, there is no petrologic record preserved for this chapter in history because of the highly active geological reworking. Right after the Moon-forming impact that occurred about 4.5 billion years ago (Valley et al., 2014), the surface of the Earth probably turned into a magma ocean and was too hot for life to start. Therefore, there should be some critical steps for the early Earth to evolve from a fiery hell to a habitable planet in the Hadean. The direct interaction between the proto-atmosphere and the newly solidified crust was probably one of these important steps because of its impact on the chemistries of the early atmosphere, the very surface of the crust and latterly, the formation of the ocean.
Since the geological record of the Hadean eon have been destroyed by the highly active plate tectonics, mountain building and erosion (Martin et al., 2006), the primordial mineralogical composition of the Earth’s surface is still a mystery. Constrained by the evolution of the magma ocean, the earliest Earth’s surface (newly solidified proto-crust) was speculated to be basaltic/komatiitic in composition (Martin et al., 2006). The composition of the Earth’s earliest atmosphere is also debatable. Assuming that it was produced during the late planetary accretion by early magmatic degassing processes (Shaw, 2008), including impact degassing (e.g., Lange and Ahrens, 1982; Ahrens et al., 1989) and volcanic degassing (e.g., Holland, 1984), the detailed degassing processes are critical in determining the species of the produced gasses. For instance, the gas species produced before and after the sinking of Fe–Ni into the core might have different oxidation states (Holland, 1984). Previous studies of the trace elements of mantle-derived komatiites, kimberlites, basalts, volcanic materials and zircons suggested that the oxidation state of the uppermost mantle was within ±2 log units of the fayalite-magnetite-quartz (FMQ) buffer probably at ∼4.4 billion years ago (Canil, 1997; Delano, 2001; Li and Lee, 2004; Trail et al., 2011). In equilibrium with this relatively oxidized mantle, magmatic degassing would have probably generated gasses mainly composed of CO2 and H2O (Trail et al., 2011). Assuming that this magmatic degassing process dominated at this stage, the early atmosphere of the Earth would mainly consist of CO2 and H2O around 4.4 Ga when the earliest ocean formed. Several studies have suggested that the early atmosphere of the Earth was likely composed of several tens MPa of water steam, 4–21 MPa of CO2 and small amount of CO, H2, etc. (Condie, 1980; Walker, 1985; Liu, 2004; Zahnle, 2006; Zahnle et al., 2007; Sleep, 2010) a few million years after the Moon-forming impact. The continuous cooling of the Earth’s surface led to the condensation of the atmospheric water steam, and subsequently, the formation of the earliest ocean before 4.4 Ga (Wilde et al., 2001; Pinti, 2005; Martin et al., 2006; Valley et al., 2014). Since the proto-atmosphere was probably enriched in CO2 (Kasting, 1993; Sleep and Zahnle, 2001), clay minerals and carbonates could be produced by fluid-rock interactions at an early age (Hazen et al., 2013). However, no outcrops with an unambiguous age older than 4.3 billion years could survive the dynamic evolution of the Earth (Tessalina et al., 2010). Thus, the existence of clay minerals and ancient carbonates on the Hadean Earth is speculative and void of solid supporting evidence.
Experiments (Berndt et al., 1996; Seyfried et al., 2007; Marcaillou et al., 2011) with similar P-T conditions to the earliest Earth have been conducted to study the process of serpentinization in the presence of liquid water. However, the reactions in these experiments could be quite different from a direct interactions between the H2O-CO2 atmosphere and ultramafic rocks, since CO2 was either not included or added as a minor component in the form of sodium bicarbonate in studies on the abiotic synthesis of hydrocarbons (Berndt et al., 1996; Seyfried et al., 2007; Marcaillou et al., 2011). Moreover, most of the experimental data were acquired at temperatures on or below 300°C. In recent years, the reaction between ultramafic/mafic rocks and minerals and CO2-rich fluid has gained more interest and several experiments have been conducted to study the carbonation process in the reaction (Dufaud et al., 2009; Jones et al., 2010; Klein and McCollom, 2013; Shibuya et al., 2013). These studies suggested that the existence of high levels of CO2 could significantly change the resulting minerals and affect the generation of CH4 by the formation of carbonates. Although certain amounts of CO2 were added in these experiments, its applied concentrations were not high enough for the reaction to represent the interaction between the H2O-CO2 atmosphere and ultramafic rocks before the formation of the ocean. Therefore, the mineralogical consequence of this direct interaction and the generation of hydrogen and hydrocarbons during this process need reassessment. Recently, the model of the mineral evolution on the Hadean Earth proposed by Hazen et al. (2008, 2013) suggested that the interactions between Earth’s atmosphere, lithosphere and hydrosphere would lead to a widespread serpentinization of Earth’s mafic crust and result in the first significant production of clay minerals, with magnesite as a possible accessory mineral. Hazen’s models did not consider the direct interaction between the H2O-CO2 proto-atmosphere and proto-crust before the formation of the earliest ocean. This process happened prior to the aqueous alteration of the proto-crust and its impact on the mineralogical diversification has not yet been experimentally tested.
In this study, experiments were carried out to simulate the direct interaction between the rocky crust and the proto-atmosphere of the early Hadean Earth. By examination of the formation of secondary minerals, hydrogen and hydrocarbons, we suggest the mineral evolution coupled the thermal and chemical evolutions of the atmosphere and ocean that have led to the formation of a habitable environment on the Earth at a very early age.
Materials and Methods
As the reduced chemical species (i.e., CO, CH4, and H2) in the proto-atmosphere only constituted small fractions of Earth’s earliest atmosphere (Condie, 1980; Walker, 1985; Liu, 2004; Zahnle, 2006; Zahnle et al., 2007; Sleep, 2010), they probably would not play substantial roles in the interaction between the proto-atmosphere and the ultramafic crust. Therefore, a H2O-CO2 system was used to represent the main geochemically active compositions of the proto-atmosphere. In our experiments, we used the following materials to represent the earliest crustal ultramafic/mafic rocks: fresh komatiite from Zimbabwe with a high Mg content and a relatively high content of Ni and Cr (Nisbet et al., 1987; Berry et al., 2008), peridotite xenolith hosted by alkali basalts from the Panshishan of Jiangsu Province of eastern China (Yu et al., 2005a,b; Hao and Li, 2013), and iron-rich alkali basalt from the Dahalajunshan Formation in the northeast Ili Block of western Tianshan. The mineralogy and chemical compositions of the starting rocks are summarized in the Supplementary Table S1. The values of Fe2+/ΣFe in komatiite and basalt were measured by 57Fe Mössbauer spectroscopy (MS) with a 25mCi 57Co/Pb source at the University of Hong Kong. For MS measurements, the finely ground rock samples were mounted in an acrylic holder with relative thicknesses of ∼5 mg Fe/cm2. Spectra were collected at room temperature in transmission mode with a constant acceleration mode. The velocity scale was calibrated relative to a 25-μm α-Fe foil at room temperature. Lorentzian doublets were used for fitting the areas of the paramagnetic doublets in those silicates.
The experiments were carried out at the Hydrothermal Laboratory at Guangzhou Institute of Geochemistry, Chinese Academy of Sciences. Fresh Ag2C2O4, distilled deionized water and powdered rock samples (<200 mesh) were weighed and loaded into a flexible Au capsule, which was subsequently sealed by a welding machine in an atmosphere of argon. The water-rock ratio (weight ratio) was adjusted to approximately 2:1, and Ag2C2O4 was restricted to a suitable amount to avoid the explosion of the sealed Au capsule. The sealed Au capsule was then put into a steel alloy autoclave. The pressure inside the autoclave was maintained by the injection of argon gas using a booster pump and was monitored by a barometer. A digital temperature controller was used to maintain a constant temperature. Batch experiments were carried out by heating the autoclave to a constant temperature under 50 MPa pressure for 2 weeks. For the komatiite-(H2O-CO2) system, the temperature of batch experiments varied from 200 to 500°C with an increment of 50°C; while for the peridotite/basalt-(H2O-CO2) systems, the temperature of batch experiments varied from 200 to 500°C with an increment of 100°C. At the end of each experiment, the autoclave was quenched to room temperature quickly with ice water. Experiments loaded with a small piece of komatiite (∼4 mm), Ag2C2O4 and H2O were also conducted to study the contact feature of the secondary minerals and the precursor rock. Control experiments loaded with rock samples, pure Ag2C2O4 and water were performed at varied temperatures (see Supplementary Table S2) to examine the possible gas products of thermal decomposition of rock samples or Ag2C2O4. The experimental conditions are summarized in Table 1.
The solid products of batch experiments were investigated by a Hitachi S-4800 field emission scanning electron microscope (SEM) and a LEO 1530 FEG SEM at the University of Hong Kong. Each sample was distributed on a small piece of silica plate by ethyl alcohol. The sample was then coated with an ultra-thin film of Au before being placed in the sample chamber of SEM. For SEM observation, the accelerating voltage was set to 3–5 kV to capture the secondary electron images, typically showing detailed surface morphology. Energy dispersive X-ray spectroscopy (EDS) measurement was also carried out at 20 kV to measure the in situ chemical compositions. The EDS analyses could only provide limited information because the secondary minerals often adhered to the host rock and contributed strong background signals. A FEI Tecnai G2 20 S-TWIN Scanning Transmission Electron Microscope (STEM) was used to characterize the crystallographic structures of the secondary minerals. Specimens were loaded on copper grids with carbon-coated formvar and examined in the normal STEM mode to obtain bright images using an accelerating voltage of 200 kV. Detailed structural information of minerals was obtained by selected area electron diffraction (SAED) patterns and high-resolution transmission electron microscopy (HRTEM) micrographs.
At the end of each experiment, the volatile components in the Au capsule were analyzed by an Agilent 6890N gas chromatograph (GC) connected with a gas-collection system at Guangzhou Institute of Geochemistry, Chinese Academy of Sciences. Firstly, the sealed Au capsule was cleaned and put into the gas-collection system. After the whole system was evacuated, the Au capsule was pierced and the sealed volatile components would fill the gas-collection system. Then the valve between the gas-collection system and the 6890N GC was opened to allow gasses to diffuse into the GC. The schematic diagram of the gas-collection system is shown in Figure 1. The concentration of H2 and hydrocarbons sealed in the Au capsule were analyzed in an automatically controlled procedure. The analytical results are summarized in Table 2.
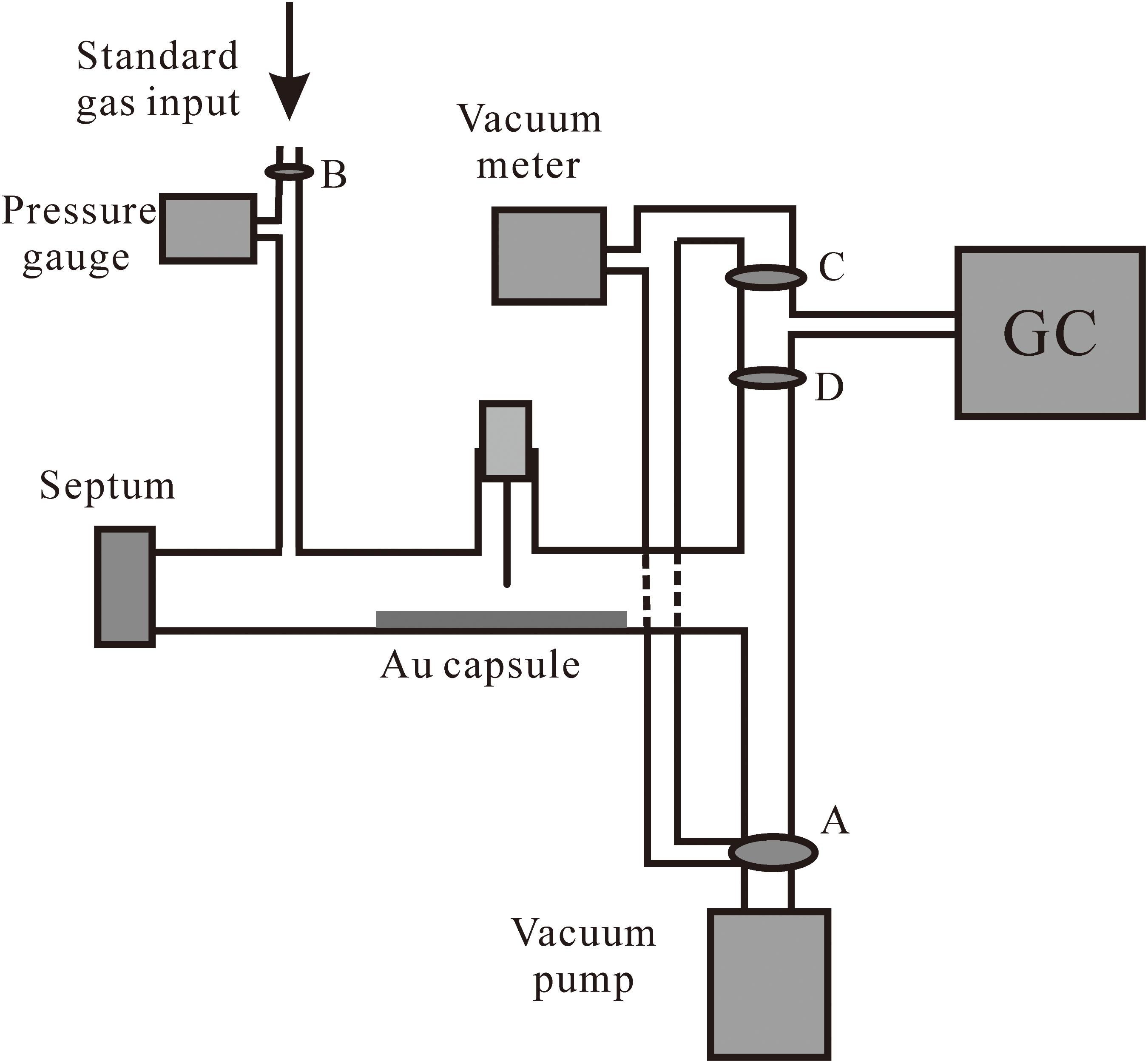
FIGURE 1. Schematic diagram of the gas collection system connected with GC. After the sealed Au capsule was cleaned and put into the gas-collection system. Valve B was closed while valves A, C, and D were open. The whole system was evacuated by the vacuum pump. Then the Au capsule was pierced by the needle on top of it to release the sealed volatile components which would then fill the space entrapped by valves A, B, and D. Finally, valve D was open to allow the gasses to be analyzed by the 6890N GC.
Results
Mössbauer Spectroscopy and Fe2+/ΣFe of the Rock Samples
The komatiite and basalt used in these experiments consist of multiple minerals/materials that showed multiple crystallographic sites of Fe2+ and Fe3+. As a result, the MS profile of komatiite was fitted with two Fe2+-doublets and one Fe3+-doublet (Figure 2). The doublet with QS = 0.53 mm/s and IS = 0.25 mm/s was assigned to paramagnetic Fe3+ in silicates; while the doublet with QS = 2.58 mm/s and IS = 1.01 mm/s and the doublet with QS = 1.85 mm/s and IS = 1.02 mm/s were both assigned to paramagnetic Fe2+ in silicates. The Fe2+/ΣFe value of the initial komatiite was 0.91 based on the fitting of the spectrum. Similarly, the spectroscopic profile of basalt was also fitted with two Fe2+-doublets and one Fe3+-doublet (Figure 2). As the result of MS of basalt, the doublet with QS = 0.46 mm/s and IS = 0.22 mm/s was assigned to paramagnetic Fe3+ in silicates; while the doublet with QS = 2.53 mm/s and IS = 1.01 mm/s and the doublet with QS = 2.17 mm/s and IS = 0.96 mm/s were both assigned to paramagnetic Fe2+ in silicates. The Fe2+/ΣFe value of the basalt was 0.67 based on the fitting of the spectrum. The MS and Fe2+/ΣFe values of the minerals in peridotite have been reported previously (Fe3+/ΣFeol = 0, Fe3+/ΣFeopx = 0.08–0.13, Fe3+/ΣFecpx = 0.19–0.31, Fe3+/ΣFesp = 0.13–0.23) (Hao and Li, 2013). The relatively high Fe2+/ΣFe values for these ultramafic/mafic rocks indicate that they undergo negligible alteration and are suitable for the batch experiments.
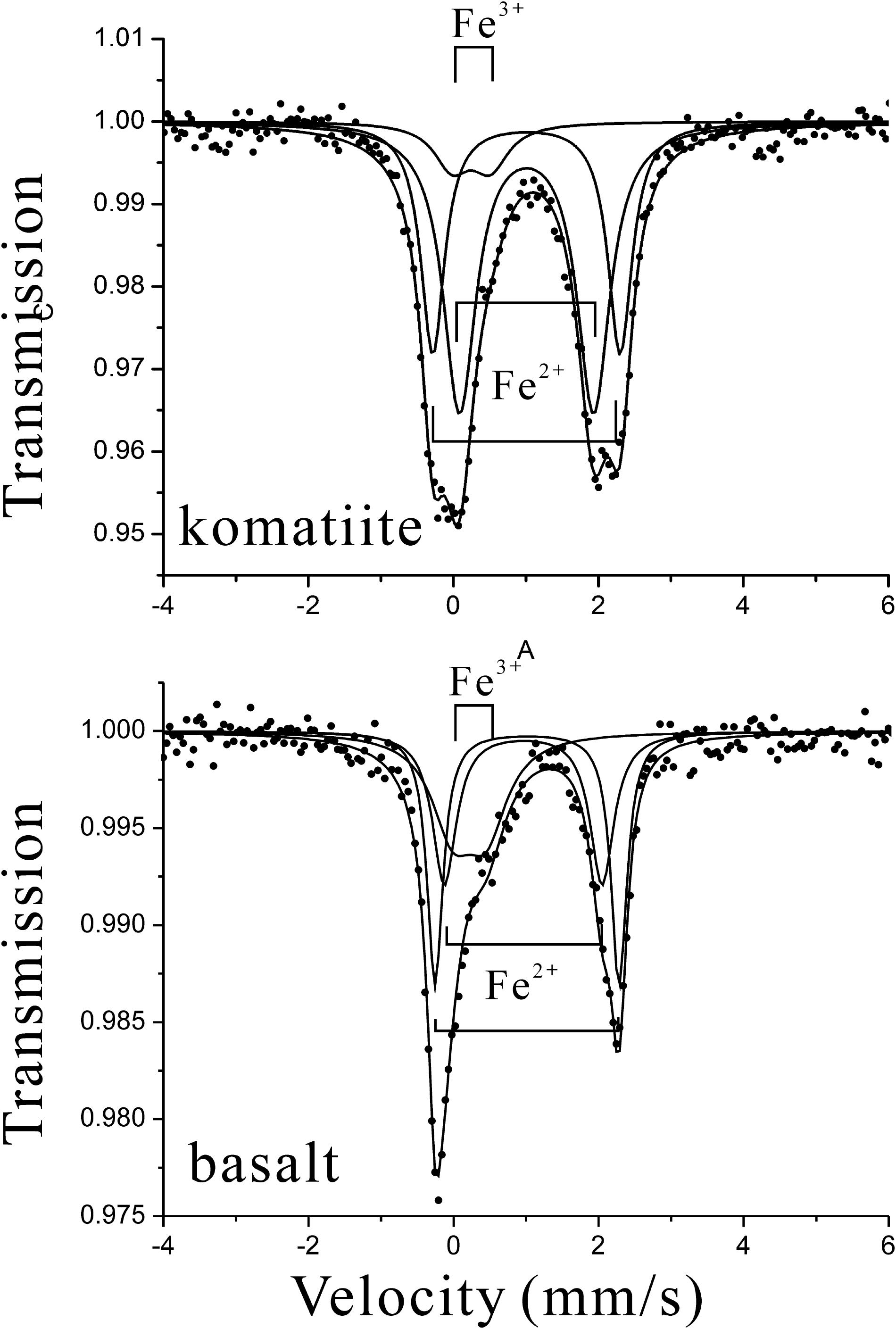
FIGURE 2. Mössbauer spectroscopy of komatiite and basalt used for the batch experiments. As both komatiite and basalt are made of multiple minerals/materials with multiple crystallographic sites of Fe2+ and Fe3+, their Mössbauer spectroscopic profiles were fitted with two paramagnetic Fe2+-doublets and one Fe3+-doublet.
Crystallography of Clay Minerals
Komatiite-H2O-CO2 System
Clay minerals, of various sizes and flaky structures, were the most abundant secondary minerals that could be observed in the komatiite-(H2O-CO2) experiments carried out from 200 to 500°C (Figure 3). The EDS analysis showed that the subsequently formed clay minerals contained substantial amounts of Si (20.0 wt%), Al (2.9 wt%), Mg (15.0 wt%), and Fe (5.9 wt%). The SEM and TEM images showed that the clay minerals generally occurred as irregularly-shaped thin flat sheets with the thickness of a few tens of nm, although some crystals have subhedral or euhedral outlines displaying 60 or 120° angles. SAED analysis of the clay crystals yielding (hkl) of (110), (200), (040), (310), and (330), indicating the electron diffraction pattern of the disordered stacking of individual crystals of Mg–Fe–Al smectite (Figure 3F). The formation of smectite was also indicated by the presence of a sharp hexagonal lattice embedded in the ring (Figure 3F).
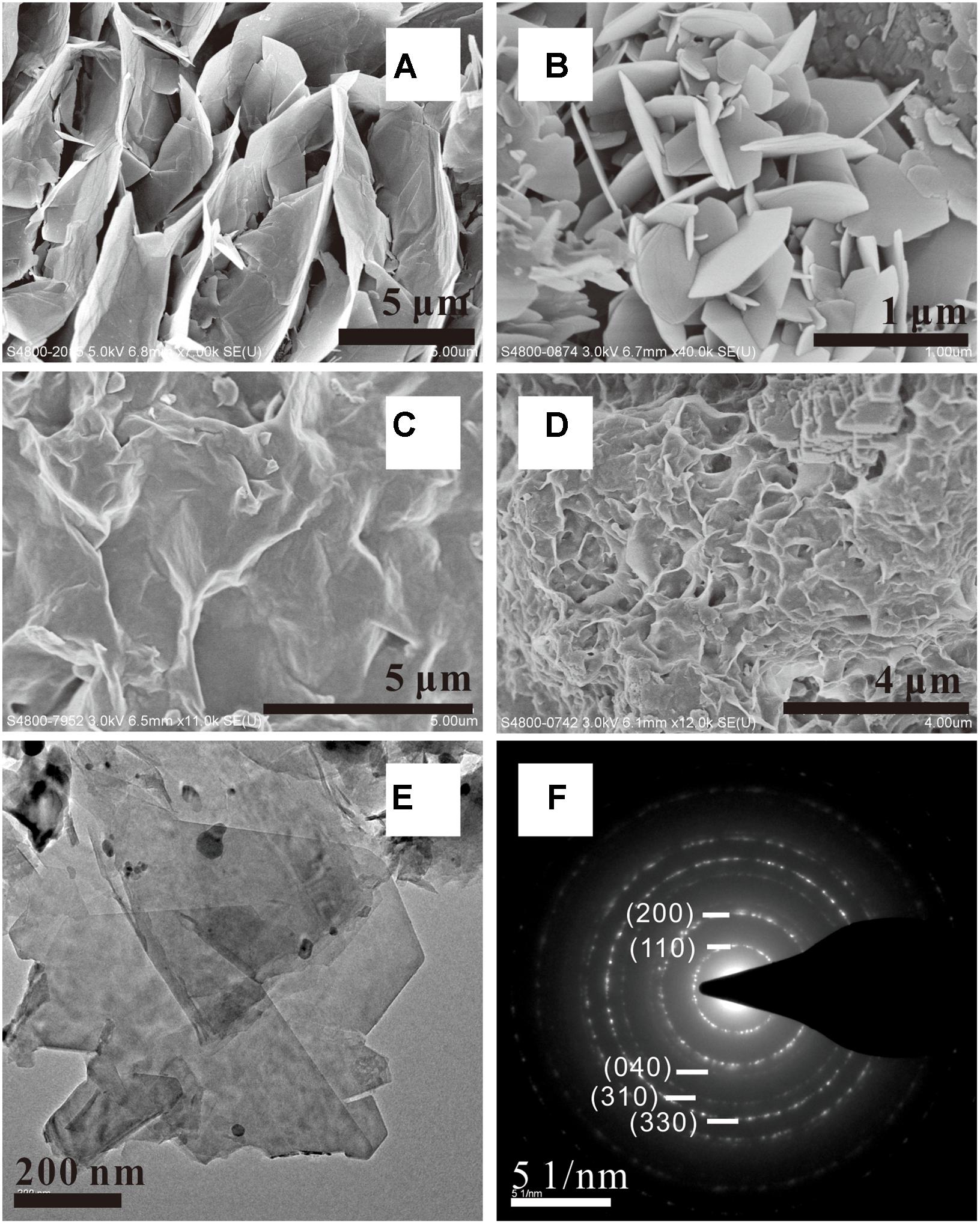
FIGURE 3. SEM (A–D) and TEM (E) observations of Mg–Al–Fe smectite formed in [H2O-CO2]-komatiite system. The SAED pattern (F) shows clear diffraction rings indicating disordered stacking of individual crystal.
Peridotite-H2O-CO2 System
Clay minerals were also identified in peridotite-(H2O-CO2) experiments conducted from 200 to 500°C (Figure 4). SEM observations showed flaky structures similar to the clay mineral found in the komatiite-(H2O-CO2) system (Figure 3). EDS results showed that the clay minerals contained mainly Mg and Fe besides Si and O. SAED analysis of the clay crystals formed at the temperatures of 400 and 500°C (Figures 4G,H,J,K) yielded similar diffraction pattern as Mg–Fe–Al smectite. However, the clay minerals formed in lower temperature experiments (200 and 300°C) did not generate diffraction rings in SAED patterns but had similar chemical compositions, indicating their amorphous nature (Figures 4I,L). The different crystallinities of the Mg–Fe smectite in the experiments imply that the higher temperatures favor better crystallinity.
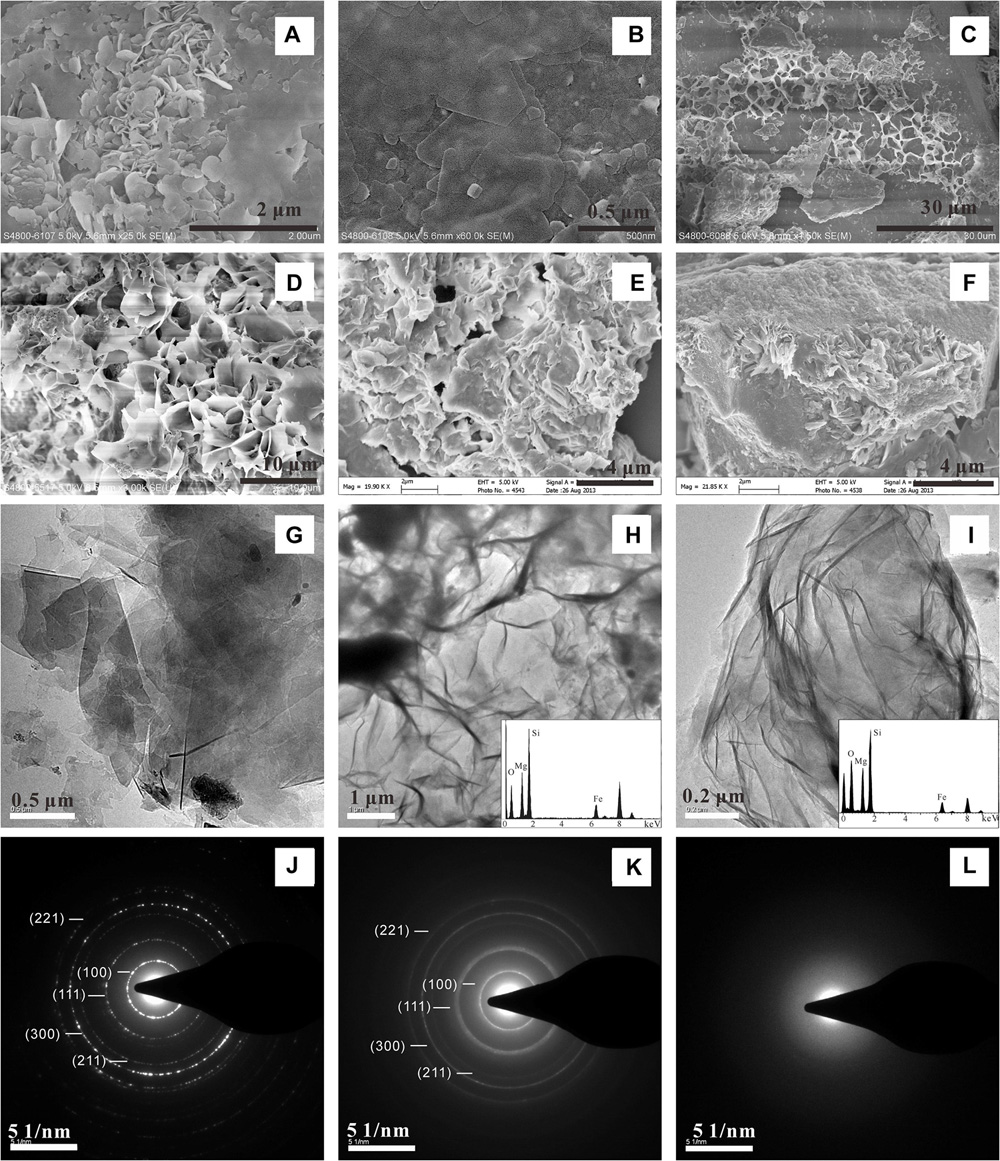
FIGURE 4. SEM (A–F) and TEM (G–I) characterizations of Mg–Fe smectite produced in [H2O-CO2]-peridotite system. The SAED patterns show well crystallized (J,K) Mg–Fe smectite and amorphous (L) Mg–Fe smectite formed in high and low temperature experiments, respectively.
Basalt-H2O-CO2 System
Clay minerals were also identified in basalt-(H2O-CO2) experiments conducted from 200 to 500°C (Figure 5). Different from the above two reaction systems, the clay minerals formed in the basalt-(H2O-CO2) system appeared as more regular hexagonal tablets with the size of a few μm and the thickness of ∼100 nm at higher temperatures (400 and 500°C). EDS analysis showed that the clay mineral contains substantial amounts of K (∼5.2 wt%) besides Mg, Fe, Al, Si, and O. SAED analysis of crystals generated a set of clear diffraction spots. Together with the EDS measured chemical composition, this clay mineral was confirmed to be illite.
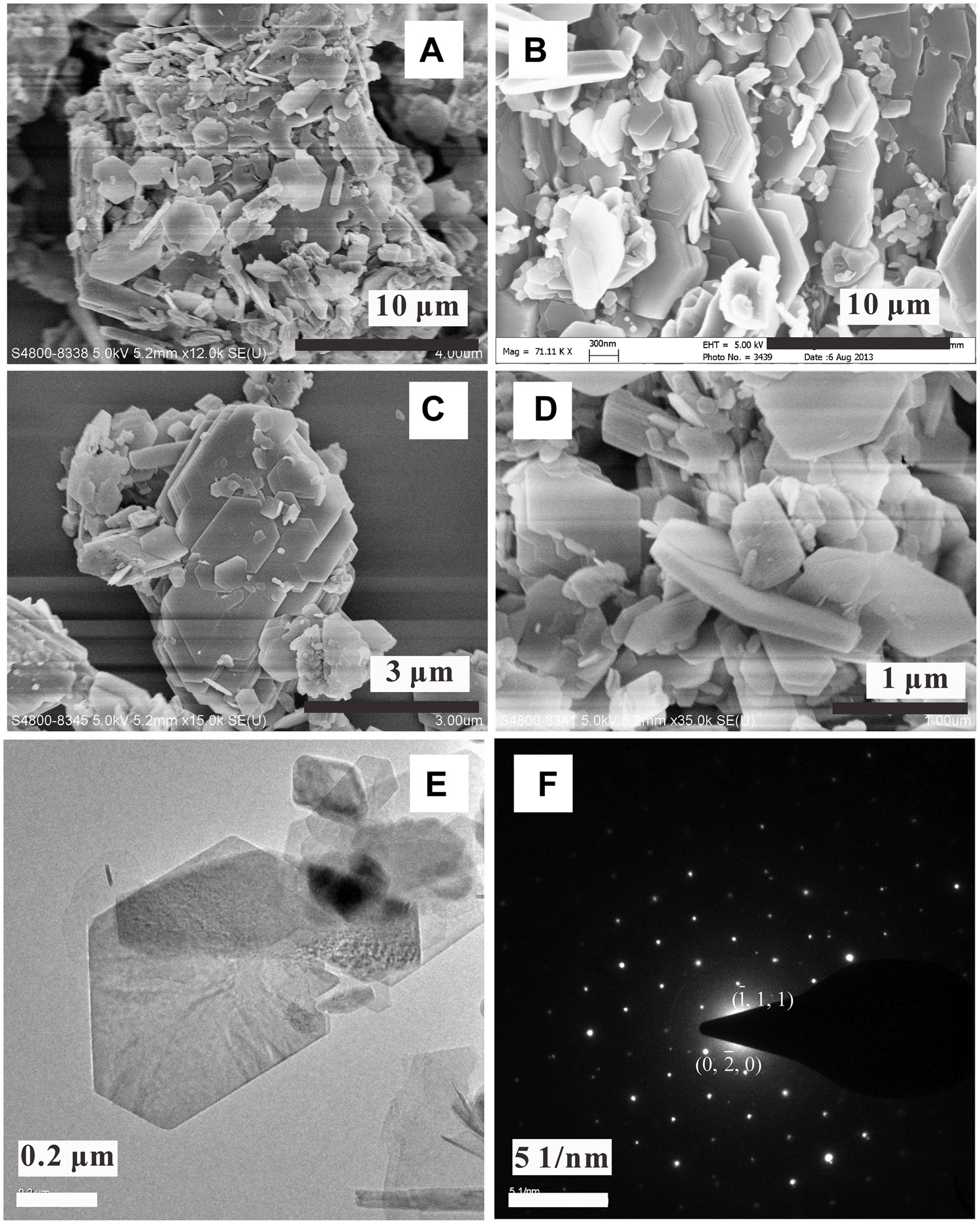
FIGURE 5. SEM (A–D) and TEM (E) characterizations of illite formed in [H2O-CO2]-basalt system. The SAED pattern (F) shows a set of clear diffraction spots indicating single crystalline nature.
Crystallography of Carbonates
Calcium Carbonate
Calcium carbonate was identified in the komatiite-(H2O-CO2) experiments carried out at 400°C and basalt-(H2O-CO2) experiments conducted at 300°C (Figure 6). The SEM observation showed that the calcium carbonate formed in both reaction systems were rhombohedral polycrystals of a few μm in size. EDS analysis showed that the mineral contained small amounts of Mg (∼1.9 wt%) and Fe (∼0.6 wt%). The obtained SAED pattern showed clear diffraction spots, indicating the high crystallinity of calcite. Experiments loaded with a small piece of komatiite (∼4 mm in diameter) showed that calcite crystals mainly stacked on the glass matrix (Figure 7), which is the Ca-containing part of komatiite (5.0 wt% Mg, 5.0 wt% Al, 16.1 wt% Ca, and 12.6 wt% Fe as measured by EDS). This close relationship indicated the extraction of Ca from the glass matrix under the experimental conditions.
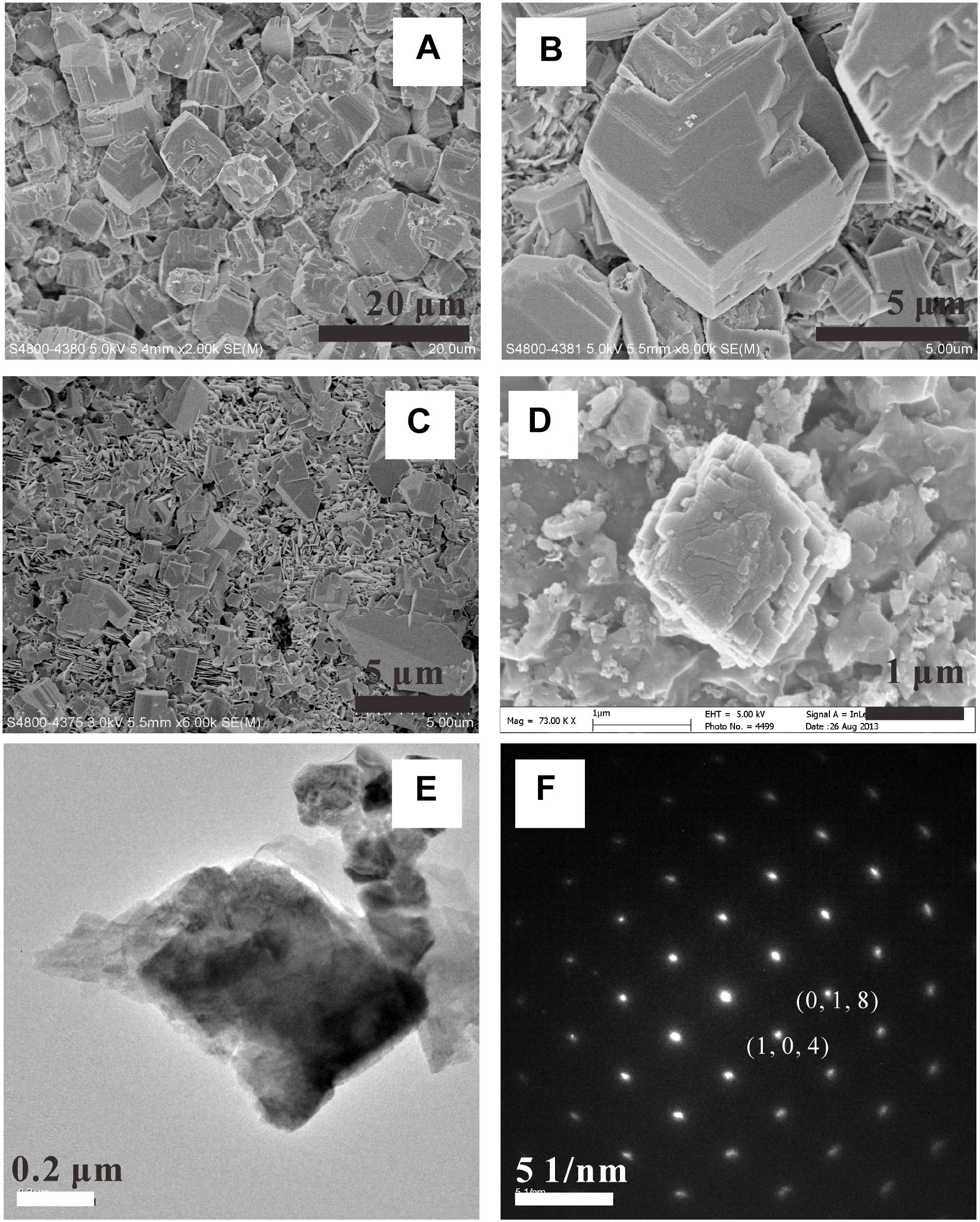
FIGURE 6. SEM observations of calcite crystals formed in the [H2O-CO2]-komatiite system (A–C) and [H2O-CO2]-basalt system (D). (F) TEM image of calcite and the related SAED pattern (E).
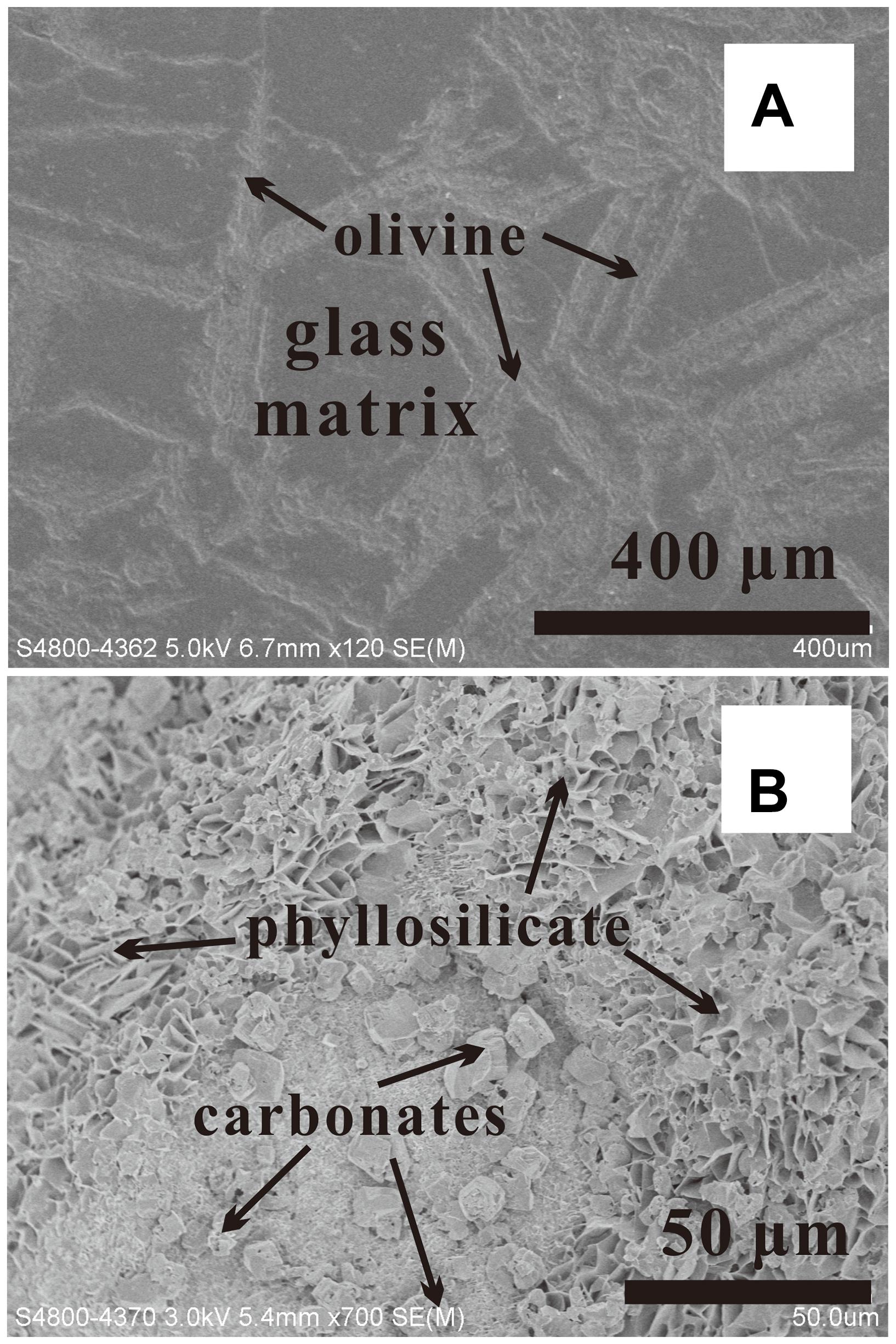
FIGURE 7. SEM observations showing the surface of komatiite before (A) and after (B) experiment. The typical secondary minerals are phyllosilicate and carbonate (400°C).
Magnesium Carbonate
In komatiite-(H2O-CO2) experiments, magnesium carbonate was generated at 200 and 250°C (Figure 8). Similar to calcite, magnesite crystals (MCs) also appeared as rhombohedral polycrystals of a few μm in size. In the peridotite-(H2O-CO2) system, MCs were observed in experiments carried out at 200 and 300°C. In the 300°C experiment, magnesite showed a similar crystal shape as that in the komatiite-(H2O-CO2) experiments; while in the 200°C experiment, MCs appeared as dodecahedron polycrystals with a uniform size of 2–3 μm (Figure 9).
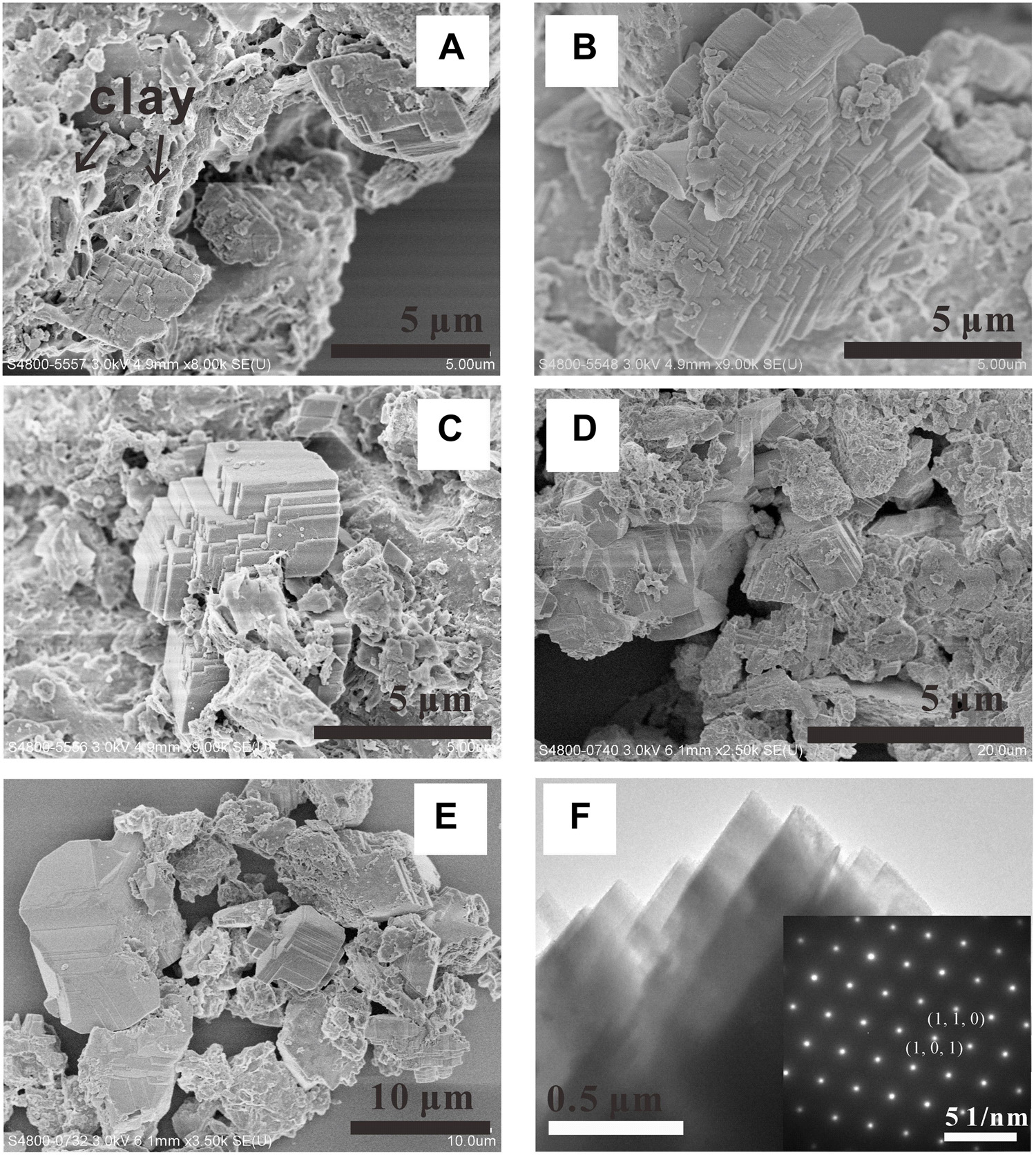
FIGURE 8. SEM observations (A–E) of magnesite formed in the [H2O-CO2]-komatiite system. (F) TEM image of magnesite crystal and the related SAED pattern.
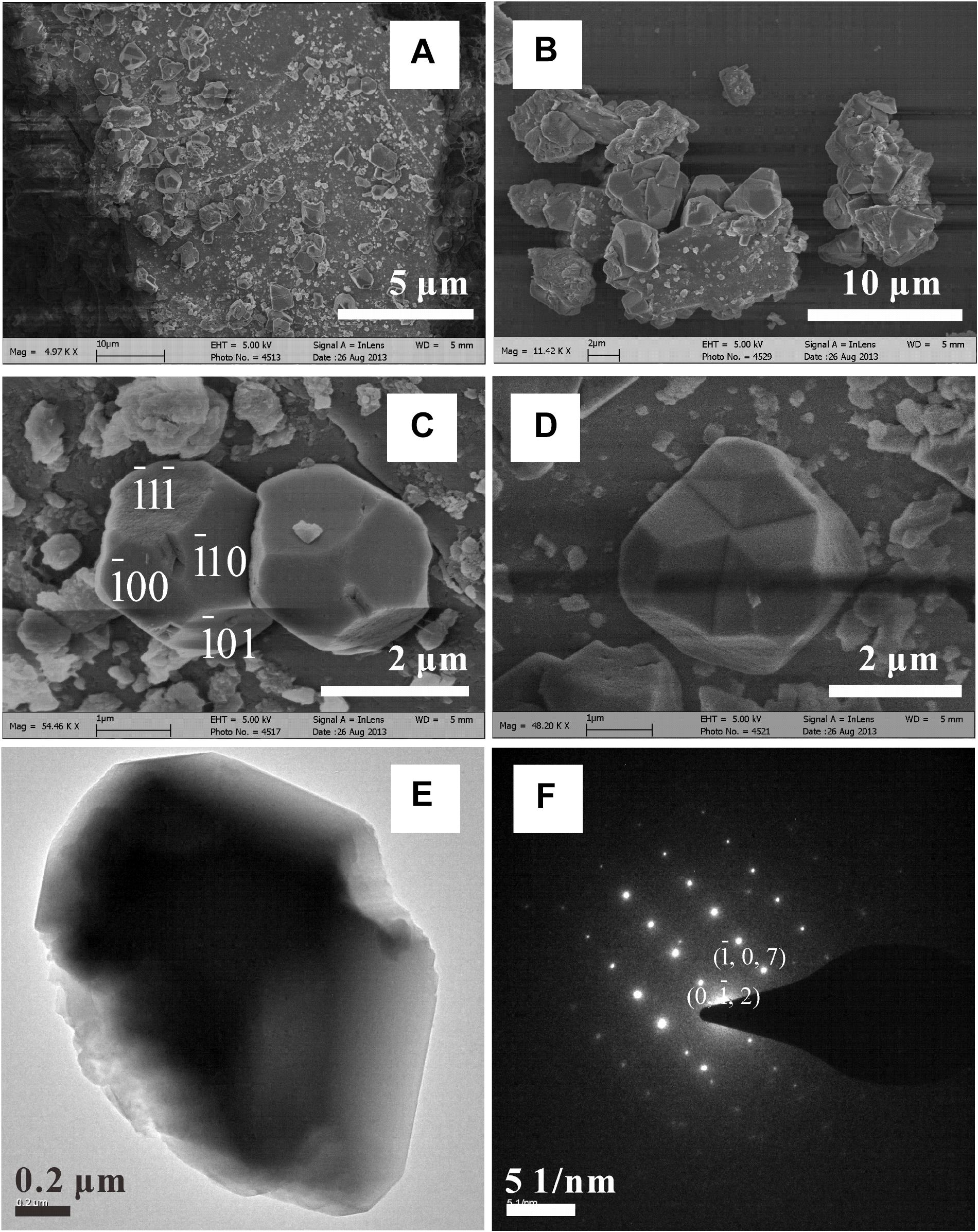
FIGURE 9. SEM characterizations (A–D) of magnesite crystals formed in the [H2O-CO2]-peridotite system at 200°C. (E) TEM characterization of one single magnesite crystal and the related SAED pattern (F).
Ca–Mg Carbonates
In three reaction systems, Ca–Mg carbonates formed at different temperatures. It formed at 300–350°C, 400°C, and 200°C in the komatiite-(H2O-CO2) system, peridotite-(H2O-CO2) system and basalt-(H2O-CO2) system, respectively. Similar to calcite crystals, the crystals formed in all systems appeared as rhombohedrons (Figure 10) and were determined by SAED to be dolomite in structure. Surface morphologies of dolomite crystals in komatiite-(H2O-CO2) system changed along with the starting states of the initial komatiite. Smooth polyhedral single-crystal particles were formed from small pieces of komatiite (∼4 mm) (Figure 10A) while crystallite aggregations with rough surfaces were obtained from the powdered komatiite (Figure 10C).
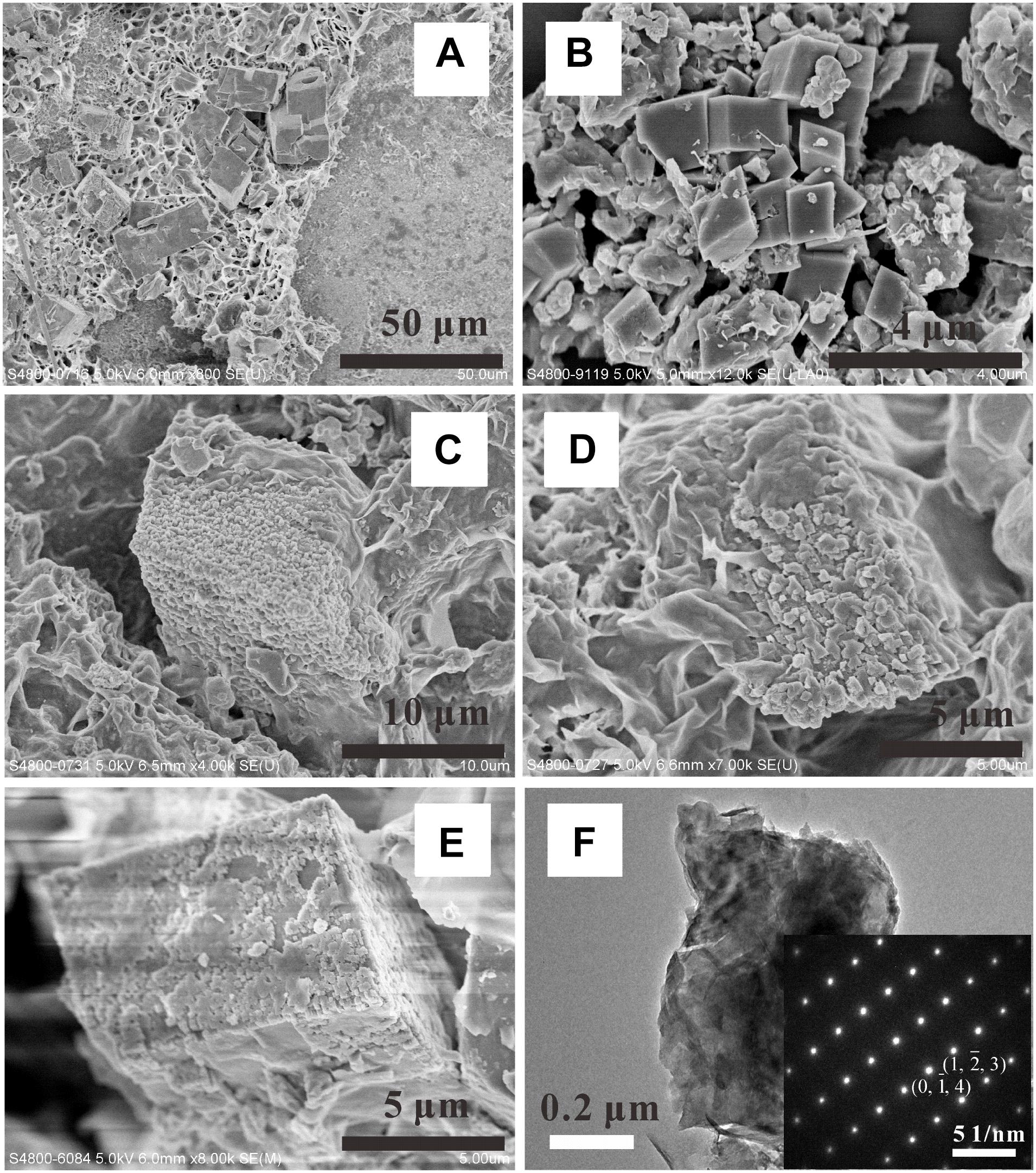
FIGURE 10. SEM observations of dolomite crystals formed in [H2O-CO2]-komatiite system (A,C), [H2O-CO2]-basalt system (B), and [H2O-CO2]-peridotite system (D,E). (F) TEM characterization of one dolomite crystal and the related SAED pattern.
Crystallography of the Other Silicates
Silicates other than clay minerals and carbonates were also identified in komatiite-(H2O-CO2) and peridotite-(H2O-CO2) systems with experimental temperatures higher than 450°C. SEM and TEM observations showed that the silicate crystals occurred as fibrous bundles that could grow up to a few tens of μm long (Figure 11). EDS analysis indicated that the silicate was mainly composed of Si, O, Ca, Mg, Fe, and trace Al. The SAED pattern of the crystals showed a set of clear diffraction spots (Figure 11F). Combined with its chemical compositions, the silicate was determined to be actinolite. HRTEM images of crystals showed clear lattice fringes, indicating their monocrystalline nature. The measured spacing values of the crystallographic planes were 0.845, 0.509, and 0.452 nm (Figure 11E) for the monocrystal, which were close to the d-spacing of (110), (001), and (040) planes of actinolite crystals.
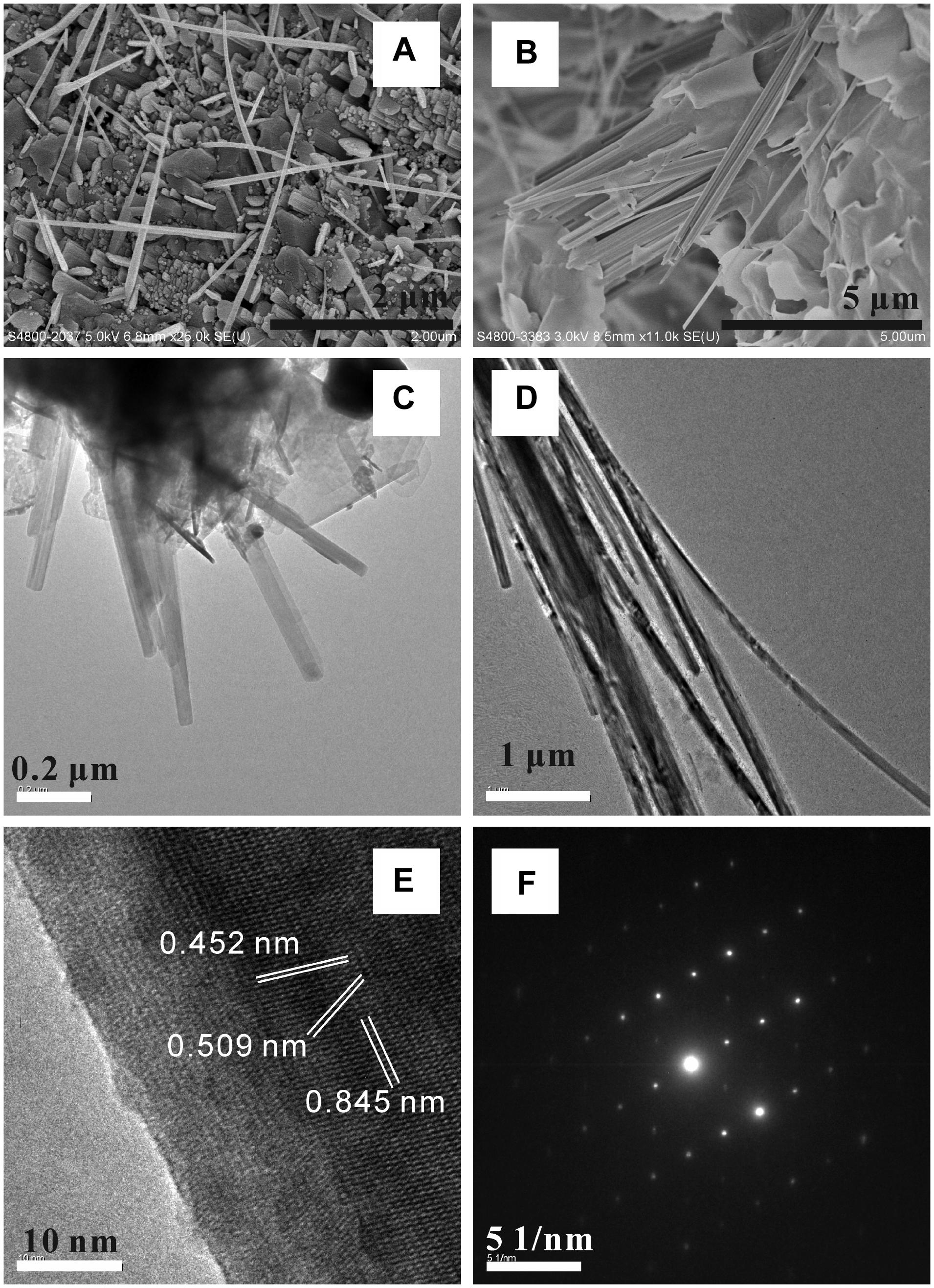
FIGURE 11. SEM and TEM characterizations of actinolite fiber bundles formed in [H2O-CO2]-komatiite system (A,C) and [H2O-CO2]-peridotite system (B,D). (E) HRTEM image of the crystal showing clear lattice fringes. The measured spacings of the crystallographic planes are 0.845, 0.509, and 0.452 nm, respectively. (F) SAED pattern of one single actinolite crystal.
Hydrogen and Hydrocarbons Produced in the Batch Experiments
Hydrogen was produced in all the three reaction systems at all experimental temperatures (from 200 to 500°C). When experimental temperatures were lower than 400°C, the productivity rate of H2 was relatively low and showed no obvious correlation with temperature, especially for peridotite/basalt reaction systems. The generation of H2 was enhanced when the experiments were conducted at 500°C. CH4 was produced in most experiments, except the one conducted at 200°C for the peridotite-(H2O-CO2) reaction system. Higher temperatures would lead to higher productivity rates of CH4. The productions of C2H6 and C3H8 were GC-detectable in the three reaction systems at relatively higher temperatures, which were much lower than that of the CH4 in the same experiment (Table 2).
Discussion
Experimental Conditions Constrained Clay and Carbonate Speciation
Clay Speciation
Clay minerals can be observed in all experiments at various temperatures. The results show straightforward evidence that the speciation of clay minerals was only affected by the petrologic features of the precursor ultramafic/mafic rocks. The change of experimental temperature appears to contribute to the nature of the crystallinity but has little influence on the chemical composition. In the komatiite- and peridotite-systems, the formed clay minerals appear similar and they both belong to the smectite group. The difference between these two kinds of clay minerals is the concentration of Al. Possibly owing to the different Al-containing materials in komatiite and peridotite, smectite formed in komatiite-(H2O-CO2) systems contains substantial amounts of Al, which is, however, negligible in smectite formed in peridotite-(H2O-CO2) systems. For komatiite system, the main Al-containing part is the glass matrix that is much easier to be altered than that in peridotite (spinel and pyroxenes). Therefore, the alteration of komatiite is likely to result in relatively higher activity of Al in the fluid and lead to the partitioning of Al into smectite. The alteration of alkali basalt has subtle difference from that of komatiite and peridotite. Due likely to its substantial amount of K (2.96 wt% K2O by EPMA), the activity of alkali metal ions (e.g. K+) could be higher in the fluid and lead to the formation of illite rather than smectite. It is worth noting that the alkali basalt contains almost 10 times more K2O than the tholeiitic basalt (Pearce, 1976). Using regular tholeiitic basalt as the starting material in the basalt reaction system would decrease the activity of K+ in the solution. In this case, the run products would be no difference in the three reaction systems in terms of clay minerals.
Since the experimental P-T conditions of previous experiments in H2O-system (Berndt et al., 1996; Seyfried et al., 2007) are similar to those in this study, serpentine, as an important secondary minerals commonly found in these studies, was never observed in our experiments. The major difference between this study and previous experiments is the role of CO2. Compared with previous experiments (Berndt et al., 1996; Seyfried et al., 2007; Dufaud et al., 2009; Jones et al., 2010; Marcaillou et al., 2011; Klein and McCollom, 2013; Shibuya et al., 2013), the concentration of CO2 in this study is significantly higher. During the experiments, the minerals would react with water to liberate Mg2+, Fe2+, and SiO2(aq) to the solution. If the solution is rich in CO2, the dissolved Mg2+ and Fe2+ will react with CO2(aq) to form Fe-bearing magnesite. This reaction can be enhanced under high CO2 partial pressures as achieved in this study. Meanwhile, the concentration of the residual Mg2+ and SiO2(aq) is critical in determining the species of the formed silicates. This phenomenon is previously reported by Klein and McCollom (2013), in which they found that serpentinization would cease after the injection of the CO2-rich fluid to the reaction system and followed by the formation of talc. Otherwise, serpentine will form under the CO2-free condition (Berndt et al., 1996; Seyfried et al., 2007; Marcaillou et al., 2011).
Carbonate Speciation
The results indicate that both the reaction temperature and precursor rock type contribute to the speciation of carbonates. Carbonates could not be observed in experiments carried out at temperatures higher than 400°C for all the three reaction systems. Instead of carbonates, fibrous actinolite was formed in komatiite/peridotite systems at higher temperatures. The petrologic difference of the precursor rocks also has an effect on the speciation of carbonate, for example, dolomite is produced in basalt-(H2O-CO2) systems while magnesite is formed in the komatiite/peridotite-(H2O-CO2) systems at the same reacting temperature (e.g., 200°C).
Because of the high CO2 partial pressures and relatively small amount of starting rock powder in the gold capsule throughout those experiments, the alkali metal ions extracted by the fluid-rock interaction were insufficient to make the solution alkaline. As a result, the fluid in the Au capsule was probably slightly acidic. Under such considerations, carbonate speciation is controlled by the ionic composition of the fluid in each experiment. As shown in Figure 12, the formation of carbonates is constrained by the activities of Ca2+, Mg2+, and dissolved SiO2(aq) in the fluid. Higher temperatures (>400°C) would speed up the alteration and increase the activity of dissolved SiO2 in the fluid, which gives rise to the formation of actinolite (Fe-containing tremolite) and inhibits the formation of carbonates. When the experimental temperature is lower than 400°C, the reaction becomes slower and the lower activity of dissolved SiO2 makes the formation of carbonates possible. In this situation, the speciation of carbonate is controlled by the activities of Ca2+ and Mg2+ in the fluid.
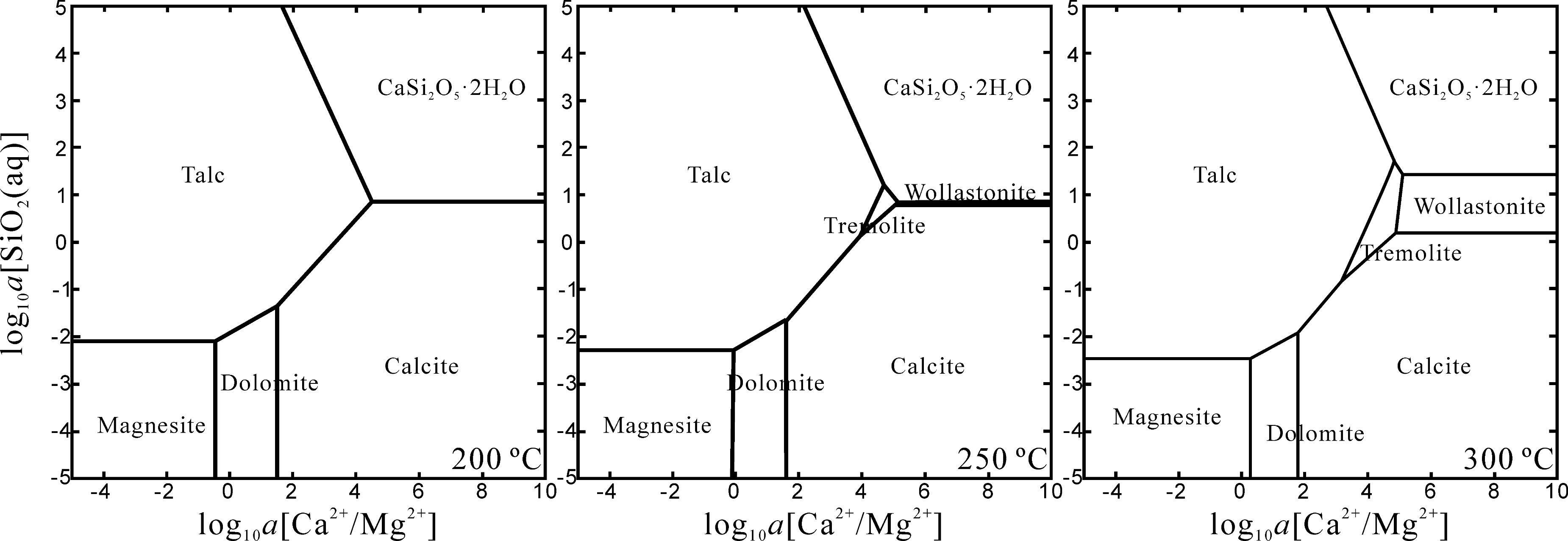
FIGURE 12. The activity diagrams of the Ca-Mg-Si-H-O-C system at 200–300°C and 50 MPa. The diagrams are calculated with Geochemist’s Workbench (Bethke, 1996) using the Lawrence Livermore National Laboratory database. Although the upper limit of temperature is restricted to 300°C, the change of the equilibrium area of tremolite with temperature suggests that it could probably become the most stable phase with relatively higher SiO2(aq) activity and log10a[Ca2+]/[Mg2+] with higher temperature.
Experimental Conditions That Affect the Production of H2 and Light Alkanes
GC analyses have shown that H2 and light alkanes were produced in the batch experiments. Further investigations would be required to ascertain the exact nature of the formation process of these auxiliary compounds, however, we can offer rational explanations. As described in previous studies (Barnes et al., 1972; Moody, 1976; Berndt et al., 1996; Marcaillou et al., 2011), H2 could be produced during the serpentinization of ultramafic rocks owing to the oxidation of Fe(II) to Fe(III) by water. Control experiments (C-400 and C-500) showed that detectable H2 could be produced without the addition of ultramafic rocks under higher temperatures (above 400°C), probably due to the oxidation of Ag generated by thermal decomposition of Ag2C2O4 (Supplementary Table S3).
Light alkanes can form by a variety of chemical pathways. Since H2 can be produced directly by the water–rock interactions, coupled with the reduction of ferrous iron and probably the formation of magnetite (Hao and Li, 2015), Fischer-Tropsch synthesis of alkanes might have occurred in our reaction systems with the absence of initial H2. Previous studies (McCollom and Seewald, 2001; Foustoukos and Seyfried, 2004; Lazar et al., 2012) have shown that light alkanes including CH4, C2H6, and C3H8 can be produced in hydrothermal experiments, however, the 13C labeling technique used in those experiments indicated that only a proportion of CH4 was produced by the reduction of CO2. Therefore, further experiments performed with 13C-labeled carbon source (e.g., Ag213C2O4) need to be conducted to evaluate the possible contribution of background sources to the observed light alkanes.
Compositional Change of the Proto-Atmosphere and Influence on the Earth’s Surface Temperature
After the surface heat flow of the Earth was lower than 150 W/m2, the surface of the Earth rapidly cooled down and the ultramafic proto-crust was formed (Pinti, 2005; Zahnle et al., 2007), which could react with the super-critical H2O-CO2 atmosphere immediately, as experimentally demonstrated in this study. Once Earth’s surface temperature fell below 400°C, the planetary-wide precipitated carbonates would sequestrate the atmospheric CO2. Consequently, the rapid removal of CO2 from the atmosphere would significantly reduce the greenhouse effect and accelerate the cooling of Earth’s surface. This cooling facilitated condensation of atmospheric water vapor and the formation of the earliest ocean (Pinti, 2005).
As the carbonates formed by the planetary-wide atmosphere-crust interactions would probably be transported to the mantle in several tens of millions of years (Zahnle et al., 2007), a relatively low CO2 partial pressure was assumed. The surface temperature of the Earth could fall below the freezing point of water with low CO2 concentrations because the Sun was approximately 30% less bright when it formed at 4.6 Ga (Schwarzschild, 1958). However, our experiments have shown that CH4 and H2 can be effectively produced by atmosphere-crust interactions. They are both candidates of strong greenhouse gasses to keep the Earth’s surface warm when the Sun was still young and faint.
Scenario of the Surface Interaction Occurred on the Early Earth and Earth-Like Planets
The Early Earth
Based on the experimental simulation, it is indicated that the interaction between lithological materials and the putative H2O-CO2 atmosphere could result in the formation of various clay minerals and carbonates before the formation of the earliest ocean when Earth’s surface temperature was higher than 300°C. Because of this planetary-widely alteration, carbonates and clay minerals may be the major secondary mineral species on Earth’s surface in the early Hadean and calcite and dolomite could be the dominant carbonate species. With the cooling of the Earth’s surface, magnesite might have eventually become the dominant carbonates species. According to this study, the co-existence of different ultramafic rock, clay and carbonate assemblages may reflect different forming temperatures. Calcite, clay and ultramafic rock assemblages may indicate a relatively higher forming temperature; while magnesite, clay and ultramafic rock assemblages may represent a relatively low temperature alteration.
Clay minerals (smectite and illite) are the most abundant secondary minerals produced by the H2O-CO2-rock reactions. These layered clay minerals possess good biocompatibility, strong adsorption, ion exchangeability and expansibility (Ertem, 2004; Zhang et al., 2010; Zhou, 2011; Tran and James, 2012; Zhou and Keeling, 2013). They can absorb various biomolecules including proteins, DNAs and RNAs in the natural environment (Biondi et al., 2007; Cai et al., 2008; Wu et al., 2012). Therefore, they could play an important role in the origin of life and biogeochemical evolution in the early biosphere. When the surface temperature fell below 300°C, the water vapor in the proto-atmosphere would condense to form the earliest oceans (Pinti, 2005). Previously formed clay minerals might pervade the earliest ocean. In this case, reactions associated with the concentration and polymerization of RNA and the production of biopolymers by clay minerals might have happened at an earlier stage. This process may happen several hundred million years before there is geological evidence for early life (Schidlowski, 1988). Meanwhile, the small crystal size of the clay minerals would cause surface reactions to occur at a rather high rate. The biomolecule-clay mineral complexes could protect the biopolymers against strong radiation, bio- or photo-degradation and inactivation (Ferris et al., 1996; Hanczyc et al., 2003; Huang and Ferris, 2003; Saladino et al., 2004) and finally facilitated the origin of life on Earth (Ferris, 2002; Franchi et al., 2003). Since the global occurrence of the reaction between the ultramafic crust and the CO2-H2O dominant atmosphere, these processes would probably be widespread instead of being restricted to hydrothermal environments.
Earth-Like Planet – Mars
The putative surface interactions occurring on the other rocky planets may be similar to that has happened to the earliest Earth. Taken Mars as an example, the earliest atmosphere of Mars was theorized to be rich in CO2 and water similar to Earth’s ancient atmosphere (O’Connor, 1968; Booth and Kieffer, 1978; Gooding, 1978; Pollack et al., 1987; Forget and Pierrehumbert, 1997; Catling, 1999; Morse and Marion, 1999; Nakamura and Tajika, 2001; Longhi, 2006; Quinn et al., 2006). Accordingly, the interaction between the atmosphere, the hydrosphere and the lithosphere occurred at the early stage might also lead to the precipitation of clay minerals and carbonates. Recent findings of carbonate by CRISM on MRO (Ehlmann et al., 2008) and the rover Spirit (Morris et al., 2010) provided the strongest lines of evidence for carbonates on Mars in its early evolutionary stage. Currently, igneous rocks interacting with the atmosphere (Farquhar et al., 1998), low temperature aqueous processes (Kirschvink et al., 1997; Valley et al., 1997) and hydrothermal processes (Treiman et al., 2002) have been considered to have contributed to the formation of Martian carbonates. Our experimental results provide an alternative interpretation. Although the atmospheric pressure on early Mars may not have been as high as on the early Earth, we could also expect the occurrence of similar interactions between its H2O-CO2 atmosphere and the crust after the planetary accretion. As a result, carbonates and phyllosilicates could have been generated and accumulated on the surface of the planet at the early age. Carbonates and clay minerals produced in this process might at least constitute part of the ancient rocks on Mars besides the genesis related to aqueous alterations.
Conclusion
In this study, batch experiments were performed to investigate the interaction between the proto-atmosphere and the rocky crust on the Hadean Earth and its impact on the subsequent evolution of the surface environment toward a habitable planet. The experimental simulation showed that phyllosilicates and carbonates could probably be produced extensively on the early Hadean Earth. Before the formation of the earliest ocean, the Earth’s surface was likely covered by massive carbonates and phyllosilicates, which can also be inferred by the recent discovery of layered clay minerals and carbonate assemblages on Mars. The volatile components in the Au capsule after the batch experiments include hydrogen, CH4, C2H6, and C3H8 for most experiments, however, the generation of C2H6, C3H8, and part of CH4 may be the result of the decomposition of organic contamination in batch experiments. The H2 and methane produced by the planetary-wide processes might have been accumulated to a relatively high concentration in the proto-atmosphere. The resulting greenhouse effect may have been strong enough for the early Earth to keep its surface warm against a faint young Sun and guarantee the continuous organochemical evolution toward life.
Author Contributions
Y-LL designed the project. X-LH wrote the main manuscript text, prepared the figures, performed the experiments, and all the related analyses. All authors participated in the discussion and commented on the paper.
Funding
This study was financially supported by Research Grants Council of Hong Kong (No. 17312016) and the National Natural Science Foundation of China (No. 41506082).
Conflict of Interest Statement
The authors declare that the research was conducted in the absence of any commercial or financial relationships that could be construed as a potential conflict of interest.
Acknowledgments
We thank Professor Weidong Sun and Dr. Xing Ding for their advice on our experiments and support from the Hydrothermal Laboratory at Guangzhou Institute of Geochemistry, Chinese Academy of Sciences.
Supplementary Material
The Supplementary Material for this article can be found online at: https://www.frontiersin.org/articles/10.3389/feart.2018.00180/full#supplementary-material
References
Ahrens, T. J., O’Keefe, J. D., and Lange, M. A. (1989). “Formation of atmospheres during accretion of the terrestrial planets,” in Origin and Evolution of Planetary and Satellite Atmospheres, eds S. K. Atreya, J. B. Pollack, and M. S. Matthews (Tucson, AZ: University of Arizona Press), 328–385.
Barnes, I., Sheppard, R. A., Gude, A. J., Rapp, J. B., and O’Neil, J. R. (1972). Metamorphic assemblages and the direction of flow of metamorphic fluids in 4 instances of serpentinization. Contrib. Mineral. Petrol. 35, 263–276. doi: 10.1007/BF00371220
Berndt, M. E., Allen, D. E., and Seyfried, W. E. (1996). Reduction of CO2 during serpentinization of olivine at 300°C and 500 bar. Geology 24, 351–354. doi: 10.1130/0091-7613(1996)024<0351:ROCDSO>2.3.CO;2
Berry, A. J., Danyushevsky, L. V., O’Neill, H. S. C., Newville, M., and Sutton, S. R. (2008). Oxidation state of iron in komatiitic melt inclusions indicates hot Archaean mantle. Nature 455, 960–963. doi: 10.1038/nature07377
Bethke, C. M. (1996). Geochemical Reaction Modeling: Concepts and Applications. New York, NY: Oxford University Press.
Biondi, E., Branciamore, S., Fusi, L., Gago, S., and Gallori, E. (2007). Catalytic activity of hammerhead ribozymes in a clay mineral environment: implications for the RNA world. Gene 389, 10–18. doi: 10.1016/j.gene.2006.09.002
Booth, M. C., and Kieffer, H. H. (1978). Carbonate formation in Mars-like environments. J. Geophys. Res. 83, 1809–1815. doi: 10.1029/JB083iB04p01809
Cai, P., Huang, Q. Y., Li, M., and Liang, W. (2008). Binding and degradation of DNA on montmorillonite coated by hydroxyl aluminum species. Colloids Surf. B Biointerfaces 62, 299–306. doi: 10.1016/j.colsurfb.2007.10.016
Canil, D. (1997). Vanadium partitioning and the oxidation state of komatiite magmas. Nature 389, 842–845. doi: 10.1038/39860
Catling, D. C. (1999). A chemical model for evaporites on early Mars: possible sedimentary tracers of the early climate and implications for exploration. J. Geophys. Res. Planets 104, 16453–16469. doi: 10.1029/1998JE001020
Condie, K. C. (1980). Origin and early development of the Earth’s Crust. Precambrian Res. 11, 183–197. doi: 10.1016/0301-9268(80)90064-9
Delano, J. W. (2001). Redox history of the Earth’s interior since 3900 Ma: implications for prebiotic molecules. Orig. Life Evol. Biosph. 31, 311–341. doi: 10.1023/A:1011895600380
Dufaud, F., Martinez, I., and Shilobreeva, S. (2009). Experimental study of Mg-rich silicates carbonation at 400 and 500 °C and 1 kbar. Chem. Geol. 262, 344–352. doi: 10.1016/j.chemgeo.2009.01.026
Ehlmann, B. L., Mustard, J. F., Murchie, S. L., Poulet, F., Bishop, J. L., Brown, A. J., et al. (2008). Orbital identification of carbonate-bearing rocks on Mars. Science 322, 1828–1832. doi: 10.1126/science.1164759
Ertem, G. (2004). Montmorillonite, oligonucleotides, RNA and origin of life. Orig. Life Evol. Biosph. 34, 549–570. doi: 10.1023/B:ORIG.0000043130.49790.a7
Farquhar, J., Thiemens, M. H., and Jackson, T. (1998). Atmosphere-surface interactions on Mars: delta O-17 measurements of carbonate from ALH 84001. Science 280, 1580–1582. doi: 10.1126/science.280.5369.1580
Ferris, J. P. (2002). Montmorillonite catalysis of 30-50 mer oligonucleotides: laboratory demonstration of potential steps in the origin of the RNA world. Orig. Life Evol. Biosph. 32, 311–332. doi: 10.1023/A:1020543312109
Ferris, J. P., Hill, A. R., Liu, R. H., and Orgel, L. E. (1996). Synthesis of long prebiotic oligomers on mineral surfaces. Nature 381, 59–61. doi: 10.1038/381059a0
Forget, F., and Pierrehumbert, R. T. (1997). Warming early Mars with carbon dioxide clouds that scatter infrared radiation. Science 278, 1273–1276. doi: 10.1126/science.278.5341.1273
Foustoukos, D. I., and Seyfried, W. E. (2004). Hydrocarbons in hydrothermal vent fluids: the role of chromium-bearing catalysts. Science 304, 1002–1005. doi: 10.1126/science.1096033
Franchi, M., Ferris, J. P., and Gallori, E. (2003). Cations as mediators of the adsorption of nucleic acids on clay surfaces in prebiotic environments. Orig. Life Evol. Biosph. 33, 1–16. doi: 10.1023/A:1023982008714
Gooding, J. L. (1978). Chemical weathering on Mars - Thermodynamic stabilities of primary minerals (and their alteration products) from mafic igneous rocks. Icarus 33, 483–513. doi: 10.1016/0019-1035(78)90186-0
Hanczyc, M. M., Fujikawa, S. M., and Szostak, J. W. (2003). Experimental models of primitive cellular compartments: encapsulation, growth, and division. Science 302, 618–622. doi: 10.1126/science.1089904
Hao, X. L., and Li, Y. L. (2013). Fe-57 Mössbauer spectroscopy of mineral assemblages in mantle spinel lherzolites from Cenozoic alkali basalt, eastern China: petrological applications. Lithos 156, 112–119. doi: 10.1016/j.lithos.2012.10.016
Hao, X. L., and Li, Y. L. (2015). Hexagonal plate-like magnetite nanocrystals produced in komatiite-H2O-CO2 reaction system at 450°C. Int. J. Astrobiol. 14, 547–553. doi: 10.1017/S1473550415000014
Hazen, R. M., Downs, R. T., Kah, L., and Sverjensky, D. (2013). Carbon mineral evolution. Carbon Earth 75, 79–107. doi: 10.2138/rmg.2013.75.4
Hazen, R. M., Papineau, D., Leeker, W. B., Downs, R. T., Ferry, J. M., McCoy, T. J., et al. (2008). Mineral evolution. Am. Mineral. 93, 1693–1720. doi: 10.2138/am.2008.2955
Holland, H. D. (1984). The Chemical Evolution of the Atmosphere and Oceans. Princeton, NJ: Princeton University Press, 582.
Huang, W. H., and Ferris, J. P. (2003). Synthesis of 35-40 mers of RNA oligomers from unblocked monomers. A simple approach to the RNA world. Chem. Commun. 9, 1458–1459. doi: 10.1039/B303134A
Jones, L. C., Rosenbauer, R., Goldsmith, J. I., and Oze, C. (2010). Carbonate control of H2 and CH4 production in serpentinization systems at elevated P-Ts. Geophys. Res. Lett. 37:L14306. doi: 10.1029/2010GL043769
Kasting, J. F. (1993). Earth’s early atmosphere. Science 259, 920–926. doi: 10.1126/science.11536547
Kirschvink, J. L., Maine, A. T., and Vali, H. (1997). Paleomagnetic evidence of a low-temperature origin of carbonate in the Martian meteorite ALH84001. Science 275, 1629–1633. doi: 10.1126/science.275.5306.1629
Klein, F., and McCollom, T. M. (2013). From serpentinization to carbonation: new insights from a CO2 injection experiment. Earth Planet. Sci. Lett. 379, 137–145. doi: 10.1016/j.epsl.2013.08.017
Lange, M. A., and Ahrens, T. J. (1982). The evolution of an impact-generated atmosphere. Icarus 51, 96–120. doi: 10.1016/0019-1035(82)90031-8
Lazar, C., McCollom, T. M., and Manning, C. E. (2012). Abiogenic methanogenesis during experimental komatiite serpentinization: implications for the evolution of the early Precambrian atmosphere. Chem. Geol. 326–327, 102–112. doi: 10.1016/j.chemgeo.2012.07.019
Li, Z. X. A., and Lee, C. T. (2004). The constancy of upper mantle fO2 through time inferred from V/Sc ratios in basalts. Earth Planet. Sci. Lett. 228, 483–493. doi: 10.1016/j.epsl.2004.10.006
Liu, L. G. (2004). The inception of the oceans and CO2-atmosphere in the early history of the Earth. Earth Planet. Sci. Lett. 227, 179–184. doi: 10.1016/j.epsl.2004.09.006
Longhi, J. (2006). Phase equilibrium in the system CO2-H2O: application to Mars. J. Geophys. Res. Planets 111:E06011. doi: 10.1029/2005JE002552
Marcaillou, C., Munoz, M., Vidal, O., Parra, T., and Harfouche, M. (2011). Mineralogical evidence for H2 degassing during serpentinization at 300 degrees C/300 bar. Earth Planet. Sci. Lett. 303, 281–290. doi: 10.1016/j.epsl.2011.01.006
Martin, H., Albarede, F., Claeys, P., Gargaud, M., Marty, B., Morbidelli, A., et al. (2006). Building of a habitable planet. Earth Moon Planets 98, 97–151. doi: 10.1007/s11038-006-9088-4
McCollom, T. M., and Seewald, J. S. (2001). A reassessment of the potential for reduction of dissolved CO2 to hydrocarbons during serpentinization of olivine. Geochim. Cosmochim. Acta 65, 3769–3778. doi: 10.1016/S0016-7037(01)00655-X
Moody, J. B. (1976). Serpentinization: a review. Lithos 9, 125–138. doi: 10.1016/0024-4937(76)90030-X
Morris, R. V., Ruff, S. W., Gellert, R., Ming, D. W., Arvidson, R. E., Clark, B. C., et al. (2010). Identification of carbonate-rich outcrops on Mars by the Spirit Rover. Science 329, 421–424. doi: 10.1126/science.1189667
Morse, J. W., and Marion, G. M. (1999). The role of carbonates in the evolution of early martian oceans. Am. J. Sci. 299, 738–761. doi: 10.2475/ajs.299.7-9.738
Nakamura, T., and Tajika, E. (2001). Stability and evolution of the climate system of Mars. Earth Planets Space 53, 851–859. doi: 10.1186/BF03351682
Nisbet, E. G., Arndt, N. T., Bickle, M. J., Cameron, W. E., Chauvel, C., Cheadle, M., et al. (1987). Uniquely fresh 2.7 Ga komatiites from the belingwe greenstone-belt. Zimb. Geol. 15, 1147–1150. doi: 10.1130/0091-7613(1987)15<1147:UFGKFT>2.0.CO;2
O’Connor, J. T. (1968). Mineral stability at the Martian surface. J. Geophys. Res. 73, 5301–5311. doi: 10.1029/JB073i016p05301
Pearce, J. A. (1976). Statistical analysis of major element patterns in basalts. J. Petrol. 17, 15–43. doi: 10.1093/petrology/17.1.15
Pinti, D. L. (2005). “The origin and evolution of the oceans,” in Lectures in Astrobiology, Vol. 1, eds B. Barbier, H. Martin, and J. Reisse (Berlin: Springer), 83–112.
Pollack, J. B., Kasting, J. F., Richardson, S. M., and Poliakoff, K. (1987). The case for a wet, warm climate on early Mars. Icarus 71, 203–224. doi: 10.1016/0019-1035(87)90147-3
Quinn, R., Zent, A. P., and McKay, C. P. (2006). The photochemical stability of carbonates on Mars. Astrobiology 6, 581–591. doi: 10.1089/ast.2006.6.581
Saladino, R., Crestini, C., Ciambecchini, U., Ciciriello, F., Costanzo, G., and Di Mauro, E. (2004). Synthesis and degradation of nucleobases and nucleic acids by formamide in the presence of montmorillonites. Chembiochem 5, 1558–1566. doi: 10.1002/cbic.200400119
Schidlowski, M. (1988). A 3,800 million-year old record of life from carbon in sedimentary rocks. Nature 333, 313–318. doi: 10.1038/333313a0
Schwarzschild, M. (1958). Structure and Evolution of the Stars. Princeton, NJ: Princeton University Press. doi: 10.1515/9781400879175
Seyfried, W. E., Foustoukos, D. I., and Fu, Q. (2007). Redox evolution and mass transfer during serpentinization: an experimental and theoretical study at 200 degrees C, 500 bar with implications for ultramafic-hosted hydrothermal systems at Mid-Ocean Ridges. Geochim. Cosmochim. Acta 71, 3872–3886. doi: 10.1016/j.gca.2007.05.015
Shaw, G. H. (2008). Earth’s atmosphere - Hadean to early Proterozoic. Chem. Erde 68, 235–264. doi: 10.1016/j.chemer.2008.05.001
Shibuya, T., Yoshizaki, M., Masaki, Y., Suzuki, K., Takai, K., and Russell, M. J. (2013). Reactions between basalt and CO2-rich seawater at 250 and 350 degrees C, 500 bars: implications for the CO2 sequestration into the modern oceanic crust and the composition of hydrothermal vent fluid in the CO2-rich early ocean. Chem. Geol. 359, 1–9. doi: 10.1016/j.chemgeo.2013.08.044
Sleep, N. H. (2010). The Hadean-Archaean environment. Cold Spring Harb. Perspect. Biol. 2:a002527. doi: 10.1101/cshperspect.a002527
Sleep, N. H., and Zahnle, K. (2001). Carbon dioxide cycling and implications for climate on ancient Earth. J. Geophys. Res. Planets 106, 1373–1399. doi: 10.1029/2000JE001247
Tessalina, S. G., Bourdon, B., Van Kranendonk, M., Birck, J. L., and Philippot, P. (2010). Influence of Hadean crust evident in basalts and cherts from the Pilbara Craton. Nat. Geosci. 3, 214–217. doi: 10.1038/ngeo772
Trail, D., Watson, E. B., and Tailby, N. D. (2011). The oxidation state of Hadean magmas and implications for early Earth’s atmosphere. Nature 480, 79–82. doi: 10.1038/nature10655
Tran, A. T. T., and James, B. J. (2012). A study the interaction forces between the bovine serum albumin protein and montmorillonite surface. Colloids Surf. A Physicochem. Eng. Asp. 414, 104–114. doi: 10.1016/j.colsurfa.2012.08.066
Treiman, A. H., Amundsen, H. E. F., Blake, D. F., and Bunch, T. (2002). Hydrothermal origin for carbonate globules in Martian meteorite ALH84001: a terrestrial analogue from Spitsbergen (Norway). Earth Planet. Sci. Lett. 204, 323–332. doi: 10.1016/S0012-821X(02)00998-6
Valley, J. W., Cavosie, A. J., Ushikubo, T., Reinhard, D. A., Lawrence, D. F., Larson, D. J., et al. (2014). Hadean age for a post-magma-ocean zircon confirmed by atom-probe tomography. Nat. Geosci. 7, 219–223. doi: 10.1038/ngeo2075
Valley, J. W., Eiler, J. M., Graham, C. M., Gibson, E. K., Romanek, C. S., and Stolper, E. M. (1997). Low-temperature carbonate concretions in the Martian meteorite ALH84001: evidence from stable isotopes and mineralogy. Science 275, 1633–1638. doi: 10.1126/science.275.5306.1633
Walker, J. C. G. (1985). Carbon-dioxide on the early Earth. Orig. Life Evol. Biosph. 16, 117–127. doi: 10.1007/BF01809466
Wilde, S. A., Valley, J. W., Peck, W. H., and Graham, C. M. (2001). Evidence from detrital zircons for the existence of continental crust and oceans on the Earth 4.4 Gyr ago. Nature 409, 175–178. doi: 10.1038/35051550
Wu, L. M., Zhou, C. H., Keeling, J., Tong, D. S., and Yu, W. H. (2012). Towards an understanding of the role of clay minerals in crude oil formation, migration and accumulation. Earth Sci. Rev. 115, 373–386. doi: 10.1016/j.earscirev.2012.10.001
Yu, H. M., Xia, Q. K., Dallai, L., and Chazot, G. (2005a). Oxygen isotope and trace element compositions of peridotite xenoliths from Nushan volcano, SE China and implications for mantle metasomatism. Acta Petrol. Sin. 21, 829–838.
Yu, H. M., Xia, Q. K., Wang, R. C., and Chen, X. M. (2005b). Oxygen isotope and trace element compositions of peridotite xenoliths from Panshishan volcano, SE China. Acta Petrol. Sin. 21, 1609–1616.
Zahnle, K., Arndt, N., Cockell, C. S., Halliday, A., Nisbet, E., Selsis, F., et al. (2007). Emergence of a habitable planet. Space Sci. Rev. 129, 35–78. doi: 10.1007/s11214-007-9225-z
Zahnle, K. J. (2006). Earth’s earliest atmosphere. Elements 2, 217–222. doi: 10.2113/gselements.2.4.217
Zhang, D., Zhou, C. H., Lin, C. X., Tong, D. S., and Yu, W. H. (2010). Synthesis of clay minerals. Appl. Clay Sci. 50, 1–11. doi: 10.1016/j.clay.2010.06.019
Zhou, C. H. (2011). An overview on strategies towards clay-based designer catalysts for green and sustainable catalysis. Appl. Clay Sci. 53, 87–96. doi: 10.1016/j.clay.2011.04.016
Keywords: Hadean climate, phyllosilicates, carbonates, atmosphere–rock interaction, the earliest ocean
Citation: Hao X-L and Li Y-L (2018) Experimental Approach to the Direct Interaction Between the H2O-CO2 Atmosphere and the Crust on the Earliest Earth: Implications for the Early Evolution of Minerals and the Proto-Atmosphere. Front. Earth Sci. 6:180. doi: 10.3389/feart.2018.00180
Received: 15 May 2018; Accepted: 12 October 2018;
Published: 12 November 2018.
Edited by:
Mónica Sánchez-Román, VU University Amsterdam, NetherlandsReviewed by:
Nickolai Bagdassarov, Goethe-Universität Frankfurt am Main, GermanySujoy Ghosh, Indian Institute of Technology Kharagpur, India
Etienne Medard, Université Clermont Auvergne, France
Copyright © 2018 Hao and Li. This is an open-access article distributed under the terms of the Creative Commons Attribution License (CC BY). The use, distribution or reproduction in other forums is permitted, provided the original author(s) and the copyright owner(s) are credited and that the original publication in this journal is cited, in accordance with accepted academic practice. No use, distribution or reproduction is permitted which does not comply with these terms.
*Correspondence: Yi-Liang Li, yiliang@hku.hk