- 1Arctic Geology, The University Centre in Svalbard, Longyearbyen, Norway
- 2Department of Environmental Sciences, Western Norway University of Applied Sciences, Sogndal, Norway
- 3Department of Earth Sciences, University of Cambridge, Cambridge, United Kingdom
Despite scientific interest in the investigation of biogeochemical changes in meltwaters of the Antarctic Peninsula, we still lack an understanding of the seasonal dynamics and release of dissolved and particulate carbon, nutrients, as well as trace metals from Antarctic snowpacks. Harsh conditions, lack of appreciation of the heterogeneity of the environment, as well as logistical constraints during fieldwork mean there is great demand for more detailed and comprehensive research. Therefore, a unique, comprehensive study of snowpack biogeochemistry was performed in the Ryder Bay area of the Antarctic Peninsula during the entire 2016/2017 melt season. Two-hundred snowpack and snowmelt samples were collected throughout the campaign, to quantify for the first time, seasonal dynamics and export of dissolved carbon, in-vivo chlorophyll, nutrient, and trace metals from Antarctic snowpack in various locations. Our study uncovered the importance of environmental heterogeneity with respect to the export of solutes and carbon. A distinctive split in the temporal dynamics of solute export was found, suggesting that some solutes are rapidly delivered to coastal environments early in the summer whilst others are delivered more gradually throughout it. Coastal, low elevation snowpacks were identified as “power plants” of microbial activity, playing an important role in the regulation of land-ocean fluxes of labile carbon and bio-limiting macro- and micro-nutrients. We also found that multiannual snow residing deep below the surface can further contribute to biogeochemical enrichment of coastal ecosystems. Additionally, inland snowpack have been identified as a store for nutrients, dissolved organic carbon and chlorophyll. Lastly, we show that a number of factors (environmental characteristics, geochemical heterogeneity, and internal biogeochemical processes in snow) make simple snowpack surveys insufficient for the prediction of biogeochemical fluxes carried by snowmelt runoff into the marine environment. A return to significant fieldwork-based research in Antarctica is therefore necessary to advance our knowledge of the complex biogeochemical processes occurring there. This study provides crucial data and process insights for more accurate predictions of how changing climate will influence the Antarctic carbon cycle and the globally important Southern Ocean ecosystem.
Introduction
Future predictions of climate change draw increasing scientific attention to the fertilization potential of increased runoff from the Antarctic Peninsula into the globally important Southern Ocean ecosystem. Estimates of nutrient fluxes from the Antarctic continent have too often resorted to single-time-point measurements and assumptions that nutrient production and export is uniform across the whole region (e.g., Death et al., 2014). However, the results of hydrochemical studies suggest a marked heterogeneity in the chemical signature of meltwaters and snowpacks across Antarctica (e.g., Bertler et al., 2005). This heterogeneity is likely driven by abiotic factors such as geology, elevation, air temperature, distance from the coast or topographic factors that control water flowpaths and snowpack thickness (Hodson et al., 2009; Welch et al., 2010; Lyons et al., 2013). Surprisingly, although chemical weathering processes in ice melt or snowmelt are reasonably well-established, discrepancies exist between our understanding of the sources and process influencing the concentration of nutrients. For example, it is widely accepted that nitrogen, phosphorus, iron and dissolved organic carbon, contained in ice-melt, facilitate microbially-mediated reactions (Downes et al., 1986). Yet, in snow, nutrient dynamics are usually thought to be associated with photolytic reactions within the snowpack itself or allochthonous transport (e.g., Bertler et al., 2005; Lyons et al., 2007; Groot Zwaaftink et al., 2013; Budhavant et al., 2014; Buys, 2014). The allochthonous sources include dust and marine or terrestrial aerosols transported in the atmosphere from lower latitudes (e.g., South America, South Africa, Australia, the Pacific, and the Southern Ocean), as well as local emissions from penguin colonies and seal wallows (Legrand et al., 1998; Krinner and Genthon, 2003).
However, snow can also acquire nutrients and carbon from the direct effects of active microbial communities (especially the so-called snow algae and bacteria: Vincent, 2004). While the presence of viable microorganisms across the Antarctic snowpack surface has been confirmed, even at the South Pole (Carpenter et al., 2000), only one study has quantified the net impact of microbiologically-mediated nutrient turnover in the snowpack (Hodson, 2006).
Hodson (2006) also stressed the possible importance of the snowpack elution process (i.e., rapid removal of solutes by snowmelt, causing their high initial concentrations in runoff) for nutrient cycling and delivery to downstream terrestrial and marine environments. This study was, however, only performed at one glacial location on Signy Island (South Orkney Islands) and direct measurements of the microbial activity or the concentration of dissolved organic carbon were not conducted. Over a decade later, these links remain unexplored outside of Signy Island while seasonal dynamics of snowpack-derived dissolved organic carbon and micro-nutrients (such as iron) have yet to be examined in Antarctica.
The demand for detailed research on the above topics remains great, especially if we aspire to constrain Antarctica's influence upon the global carbon cycle and how this influence will change under increasing snowmelt. Accurate models predicting changes in terrestrial and marine ecosystems caused by increasing snowmelt demand far greater understanding of snowpack processes in Antarctica, especially with respect to the more climatically-sensitive coastal snow.
To address above knowledge gaps, a unique comprehensive study of the snowpack was performed in the Ryder Bay area of the Antarctic Peninsula over the entire 2016/2017 melt season. Two hundred snowpack and snowmelt samples were collected to reveal, for the first time, seasonal dynamics in the chemistry of both the parent snowpack and its meltwater runoff. In so doing, we quantify how dissolved carbon, nutrient and trace metals were exported by snowmelt and transferred to downstream aquatic and marine ecosystems. Furthermore, by establishing sampling sites at different elevations and variable distances from the sea, we have uncovered the importance of environmental heterogeneity in coastal and inland zones with respect to the export of dissolved ions.
Methods
Location
Snowpack as well as snowmelt samples were collected between 6th December 2016 and 23rd January 2017 at 11 locations on the Antarctic Peninsula (Figure 1). Continuous monitoring was performed at four locations at Adelaide Island (Beach, Inland, Snowfield 1 and Snowfield 2), while opportunistic snowmelt samples were collected from Anchorage Island (Coastal 1–4) and Alexander Island (Mountains 1–3).
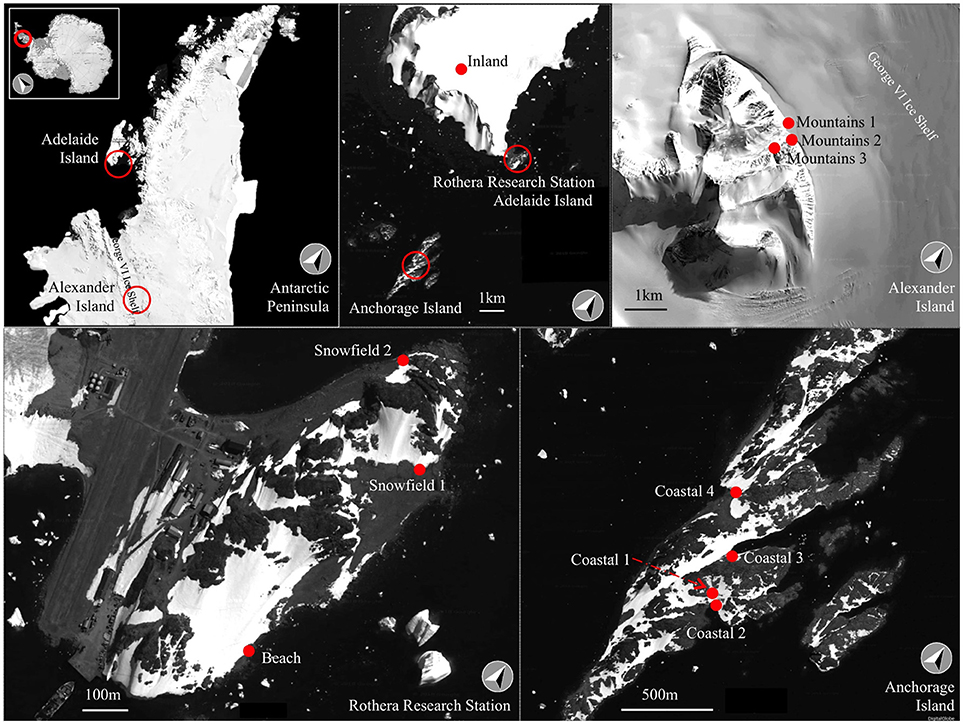
Figure 1. Study location. Images modified from googlemaps.com. Imagery @2018 DigitalGlobe. Map data Google@2018.
This variety of sampling locations ensured that the samples characterized the diverse environments found on the Antarctic Peninsula, as much as was feasibly possible. Therefore, sites were chosen to include different elevations, distance from the shore, occurrence of algal blooms, and presence of marine fauna (see Figures 1, 2 and Supplementary Table S1).
Sampling design
Two primary sites established at Adelaide Island (“Beach” and “Inland”) served as the main snow observatory where samples were collected throughout entire melt season (see Figure 1). An unspoiled snowfield was selected at both locations, and a 30 m2 exclusion zone established. A grid of ablation stakes was created (12 at Beach and 8 at Inland) in each zone to monitor snow depth changes (see Figure 2 for an example). In addition, at each location, in the middle of the exclusion zone, a snowpit was dug to two depths. The shallower depth (~1.6 m at the Beach and 2.3 m at the Inland) was the previous winter's accumulation, where lysimeters were installed to monitor the dynamics of physical and chemical properties of the snowmelt throughout entire ablation season (Figure 3). The second depth (~2.6 and 2.8 m, respectively) was dug to investigate the snow/basal-ice interface for ion, nutrient and carbon enrichment/depletion.
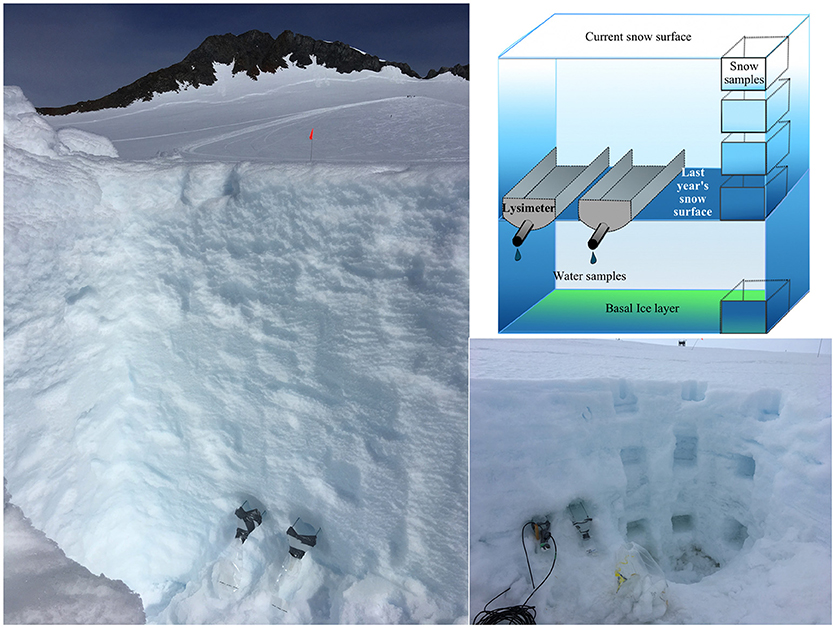
Figure 3. Snowpack sampling design and execution at two monitoring sites, the Beach (right) and the Inland (left). Photographs taken by Nowak A.
Four snowpack sampling campaigns were performed at each location: before, during (twice) and after the melt period. Each time, triplicate snow samples from multiple depths within each snow pit were collected into sterile Whirlpack bags. At the beach, snow was collected from four depths: 0–20, 70–90, 140–160 cm for samples of the previous winter's accumulation and 240–260 cm for samples of multiannual snowpack at the snow-basal ice interface. Inland, due to increased snow accumulation in the area, snow was collected from five depths: 0–20, 70–90, 140–160, 210–230 cm for previous winter's accumulation and 260–280 cm for multiannual snow at the snow-basal ice interface.
In addition to snowpack sampling, snowmelt monitoring was performed at both locations from the onset of melt in December until the freeze up in February. The melt was captured by acid washed acrylic lysimeters (100 × 15 cm L × W) inserted at the bottom of the snowpits (see Figure 3). Snowmelt was collected into 1.5 L sterile Whirlpack bags every day that melt occurred. These data were used to estimate solute fluxes from the product of the daily runoff volume and individual solute concentrations. The fluxes were then standardized for the contributing area of the snow to estimate solute yields (by dividing the flux estimate by the surface area of the lysimeters). Finally, snowmelt in other locations was sampled opportunistically due to weather conditions and/or access restrictions (see Supplementary Table S2 for more detail).
Analyses
Samples were processed immediately after collection at Bonner Laboratory, Rothera Research Station. Snow was allowed to thaw in the dark, at room temperature for up to 24 h, and processed in the same way as snowmelt. All samples were divided for immediate determination of pH, electrical conductivity (EC), concentration of in-vivo chlorophyll (with UNILUX Fluoremeter, Chelsea Instruments, UK) and filtration through sterile filter paper, and acid washed Nalgene filtration unit, for later analyses in the UK. Whatman cellulose nitrate filter papers (0.45 μm) were used during filtration for dissolved ions, while Whatman GF/F filter papers (0.7 μm) were used during filtration for dissolved carbon. After filtration, samples were divided for ion and trace metal analyses, transferred into sterile, acid washed (with 10% HCl) Corning centrifuge tubes (60 and 15 ml, respectively), and stored in the dark in +4°C for up to 3 months. Samples for major ions and nutrients were stored with no air, whilst samples dedicated for trace metal analyses were acidified with 100 μl of ultra-pure 1 M HNO3 prior to storage.
High-Precision Ion chromatography (Dionex ICS-5000+ SP) was undertaken at the University of Cambridge to analyse the concentration of anions. The sample was passed through an anion column IonPac AG18 with 31 mM of potassium hydroxide (KOH) as eluent. Calibration standards were prepared by dilution of OSIL Atlantic Seawater. The detection limit (three times the standard deviation of 10 blanks) and was below 0.05 mg/L. Concentration of cations and trace metals were determined at Leeds University. Cations were determined by the use of ICP-OES with a Thermo Fisher iCAP 7400 Radial ICP-OES, while trace metals were determined by ICP-MS using a Thermo Fisher iCAPQc. Detection limits calculated from triplicate blank samples were for major ions between 0.0015 and 0.010 mg/L, and for trace metals, between 0.005 (recorded for Mn) and 0.38 μg/L (recorded for DFe: see Supplementary Table S3 for details). Precision errors were always below 2%. To ensure high quality of the trace metal analyses, all sample vessels were washed with trace metal grade acids, and the instrument was cleaned before use. All samples as well as standards were prepared in a laminar flow cabinet to prevent contamination, and the sampler with a hood was used during the analyses to prevent dust settling into the vials. A Sievers 5310C Organic Carbon Analyser at the University of Sheffield was used to determine concentration of organic carbon (DOC), total inorganic carbon (TIC) and total carbon (TC). Detection limit was 0.007 mg/L.
Results
Antarctic Snowpack Biogeochemistry
The mean, range, and standard deviation of all parameters measured in the snowpack are presented in Table 1.
The two sites selected for continuous monitoring showed distinct differences in the physical characteristics of the snowpack as well as their chemical properties. The snowpack at the beach was 40% thinner, contained multiple ice lenses of variable thickness and its basal layer showed a clear indication of the presence of dust and marine fauna effects (hairs, manuring, and feathers). As the season progressed, the stratigraphy of the beach site changed rapidly and snow density increased markedly (from 375 to 600 g/L). In contrast, seasonal changes at the inland snowpack were less rapid and pronounced. Here, melt progressed much slower and only five ice lenses were visible. No visible signs of biological enrichment (such as in snowpack at the beach) nor dust were detectable within the snowpack throughout the entire season (see Figures 2, 3 for visual comparison of both sites).
Discrepancies in the physical properties of the snowpack coincided with distinct differences in their chemical properties. Although snowpack pH stayed below neutral throughout the monitoring period (5.43–6.58), snow at the beach was generally less acidic, contained, on average, almost double the amount of dissolved ions, nutrients, in-vivo chlorophyll, and showed evidence for enrichment of DOC (see Table 1 and Figures 4, 5 for more details).
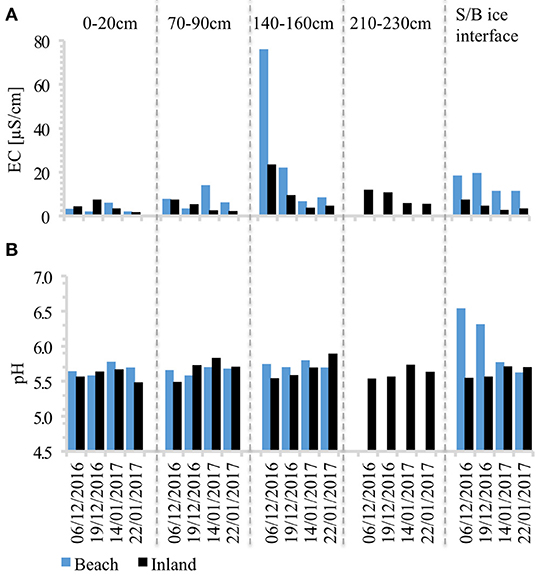
Figure 4. (A) Electrical conductivity (EC); and (B) pH dynamics in the snowpack. Values are averaged from triplicate samples at each depth. S/B ice interface represents snow collected at depth 240–280 cm at the beach site, and 260–280 cm at the inland site. Standard deviations are shown in Table 1. Note that snow was shallower at the beach (hence no data for 210–230 cm).
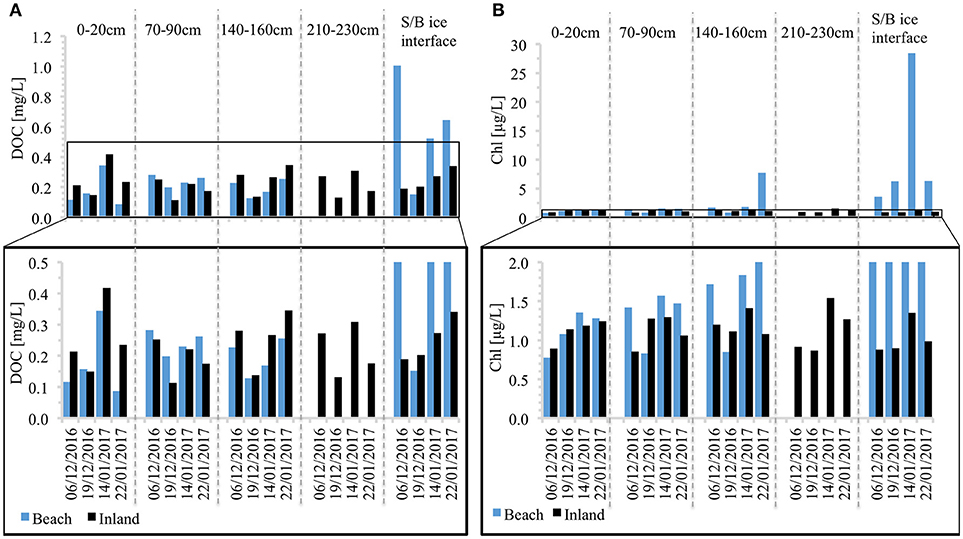
Figure 5. Snowpack (A) Dissolved organic carbon (DOC); and (B) in-vivo chlorophyll concentration. For clarity the graphs at the bottom show variability at the lower end of each scale.
The purest snow with the lowest concentrations of DOC, in-vivo chlorophyll, major ions, nutrients and trace metals at both sites was observed at the surface (0–20 cm), whilst considerable solute enrichment was found at the base of the previous winter's accumulation layer (~160–230 cm). Furthermore, there is clear evidence of the elution of ions throughout the ablation season, because electrical conductivity (EC) at almost all depths decreased with time (Figure 4). A seasonal ion elution pattern (high initial ion concentrations at the onset of melt, followed by a marked drop and considerably lower values thereafter) was also observed at the snow/basal ice interface (~240–280 cm).
A striking feature is also the enrichment of DOC and chlorophyll at the snow/basal ice interface observed at the beach site (Figure 5). Otherwise, temporal variations in snowpack DOC are broadly similar throughout the snow column and between both sites, while chlorophyll concentrations show a marked seasonal increase at all depths only at the beach site (Figure 5B).
Antarctic Snowmelt Biogeochemistry
The mean, range, and standard deviation of all parameters measured in the snowmelt are presented in Table 2.
The chemical characteristics of snowmelt at the different sites also showed marked differences, with a strong elution signal at the beach that resulted in high EC early in the season (before 1st Jan, hereafter the “elution phase”; see Figure 6). By contrast, inland snowmelt as well as stream waters showed much less variability and concentrations remained five to ten times lower. It needs to be noted that the absence of data from the early season at the inland site resulted from a lack of melt at higher elevation rather than insufficient sampling. Samples from other low elevation sites such as Snowfield 1 and 2 were collected immediately after a visible streamflow was formed. It is, however possible that, if lysimeters were used there, a similar snowmelt elution phase as seen at the beach site would most likely have been recorded, on account of their low elevation, proximity to the coast, and sporadic occurrence of marine fauna in the area.
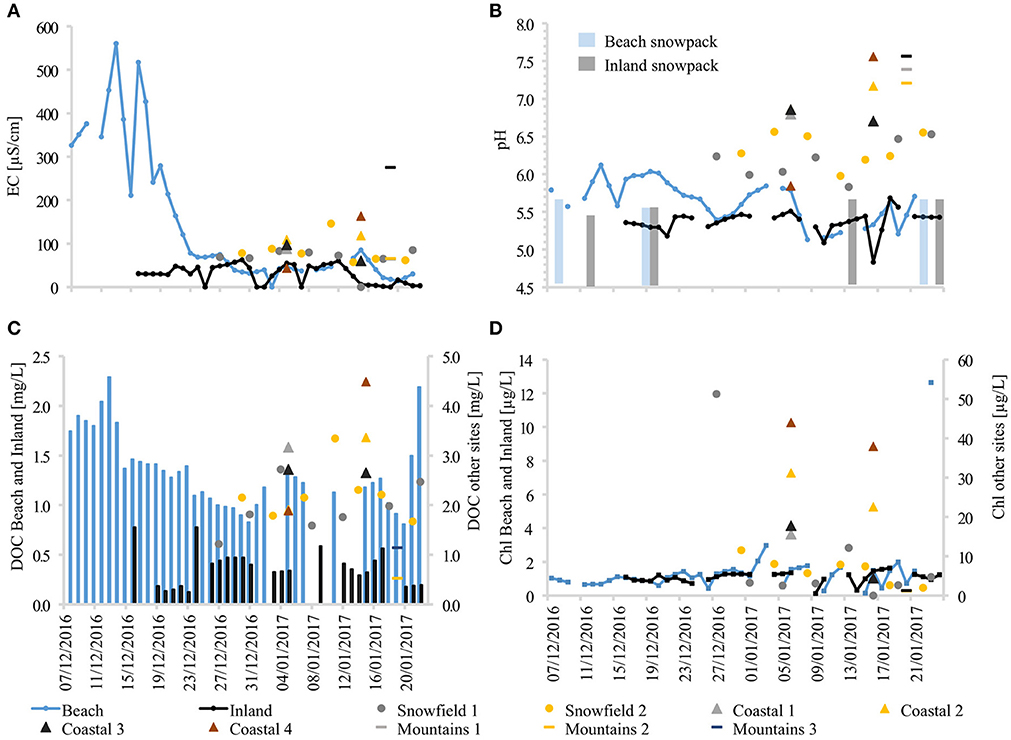
Figure 6. Seasonal snowmelt dynamics and snowmelt chemical characteristics at all sampling sites. (A) Electrical conductivity, (B) pH, (C) DOC, and (D) Chlorophyll concentrations.
A strong elution signal in beach site snowmelt was also demonstrated by the pH, and DOC values. Interestingly, the same elution pattern was also observed for some of the trace metals (Figure 7), such as key bio-limiting micronutrients like zinc (Zn) and dissolved iron (DFe) whose concentrations were below detection limit in the snowpack (0.38 μg/L). Although the elution phase was not apparent at other sites, stream waters were characterized by much higher concentrations of major ions, trace metals, DOC, pH, and in-vivo chlorophyll.
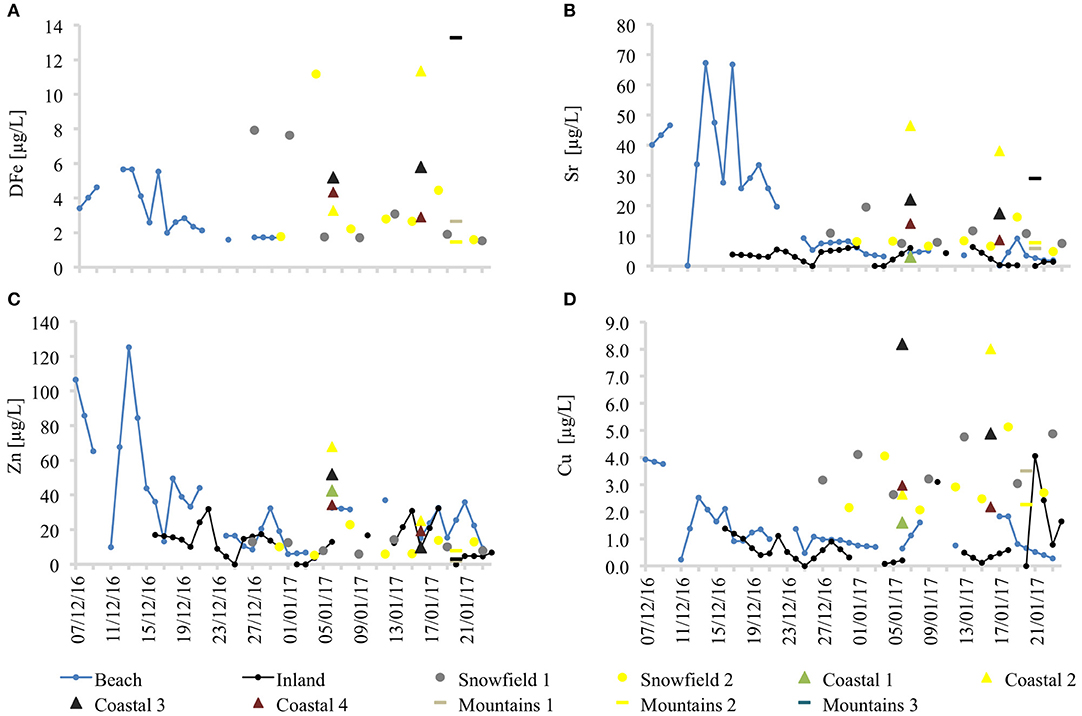
Figure 7. Selected trace element dynamics in snowmelt at all sampling sites. (A) Dissolved iron, (B) Strontium, (C) Zinc, and (D) Copper.
Discussion
Seasonal Dynamics of Solute Production Within the Snowpack and Their Release With Snowmelt
The above results clearly show that Antarctic snow and snowmelt carry rather distinctive hydrological and biogeochemical signatures specific to the environment from which the samples were collected. Low elevation sites are characterized by thinner snow cover and thaw that progressed steadily throughout the ablation season. As a consequence, ions (mono- and bivalent) were effectively eluted from the snowpack within the first 3 weeks from the onset of melt (Figures 6A, 7, 8). The process did not seem to be markedly influenced by the presence of multiple ice lenses within the snowpack, nor the occasional cessation of flow and refreezing during either night-time or cold spells. At higher elevation, the inland snowpack was much thicker, dryer and the onset of melt was observed over a week later. The sporadic occurrence of ice lenses within the snowpack indicated that melt/refreeze occurred here rarely, or at least less frequently than closer to the coast. Lack of an ion elution curve and much lower dissolved ion concentrations were also indicative of a less dynamic snowpack environment.
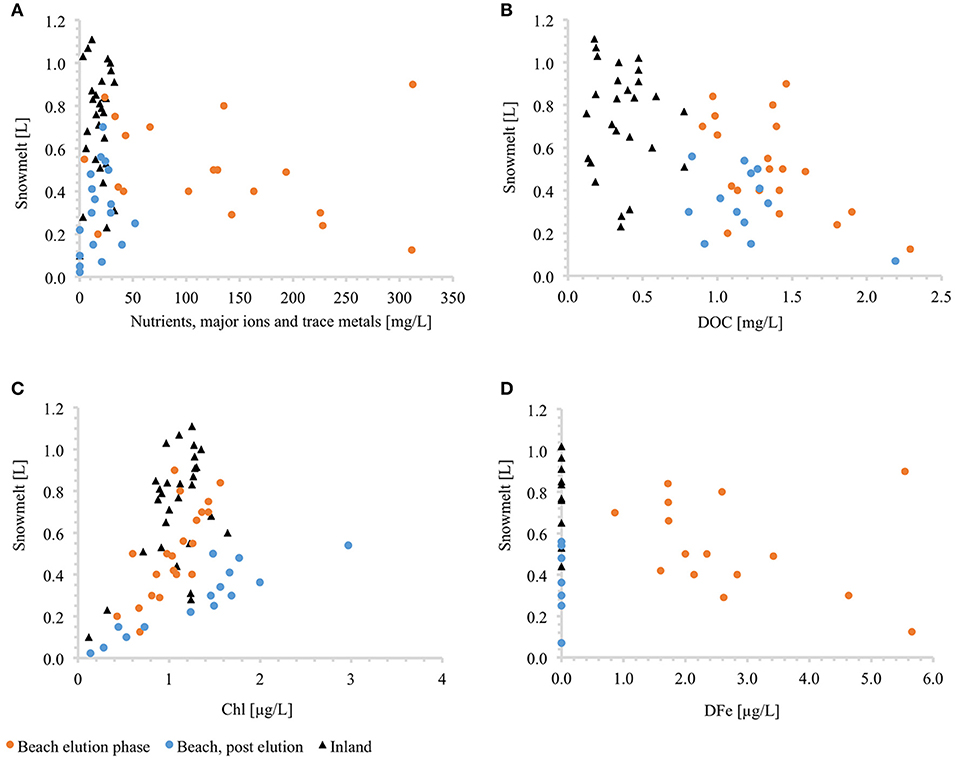
Figure 8. Correlations of lysimeter discharge with concentrations of dissolved ions (A), organic carbon (B), in-vivo chlorophyll (C) and dissolved iron (D) at the beach and the inland sites. The elution phase represents period from 6th December 2016 until 1st January 2017.
Nonetheless, it is clear that both snowpack environments investigated in this study showed marked biogeochemical differences within the snow column, as well as noticeable ion dynamics throughout the melting season. At both environments, the top layer of the snow (0–20 cm) contained the lowest concentration of dissolved ions, DOC and in-vivo chlorophyll, all of whose concentrations generally increased with depth in the snowpack, peaking at the previous summer surface (~160 and 230 cm, see Figure 5). This is an interesting result as it hampers the use of surface remote sensing techniques for snowpack biomass detection, as has already been employed elsewhere using reflectance measurements for snow algal pigment detection (see Painter et al., 2001; Takeuchi et al., 2006). For the same reason, remote sensing technology that offers new methods for monitoring global carbon levels [e.g., Orbiting Carbon Observatory (OCO-2)] or the use of sun induced fluorescence (SIF) as a proxy for gross primary production (Frankenberg et al., 2014; Koffi et al., 2015; Zhang et al., 2016; Sun et al., 2018), cannot be applied to Antarctic snow on the basis of our results. Similar limitations to remote sensing use, but for Antarctic sea-ice studies, were also suggested by Lieser et al. (2015).
The results of our study also indicate that at both the beach and the inland site, a chemically, and possibly microbially-enriched layer of multiannual snow resides just above the basal ice (at ~260 and 280 cm, respectively). The enrichment in DOC and in-vivo chlorophyll in this layer was particularly visible at the beach, where the effects of marine fauna were evident. Here, the snow/basal ice interface was also characterized by seasonally decreasing pH and a lack of nutrients, which we presume were assimilated or otherwise processed by heterotrophic microorganisms, because these nutrients were recorded in the other snow layers. Collectively, these characteristics indicate that the most active microbial environments exist within the snowpack immediately adjacent to coastal ecosystems. This coastal fringe of biogeochemically-active Antarctic snow will play a significant role in the regulation of the land-ocean fluxes of labile carbon and nutrients caused by snowmelt runoff. Therefore, future studies of Antarctic snowpack biogeochemistry should include the particularly important role played by older, multiannual snow at the base of the snowpack, especially since there have been marked changes in summer melt rates over recent decades in the Antarctic Peninsula region (Turner et al., 2014; Trusel et al., 2015). In addition, large increases in the extent of wet perennial snowpacks and firn in the maritime Antarctic of the Peninsula region have also been observed in recent years (Thomas et al., 2015; Oliva et al., 2017; Petlicki et al., 2017; Engel et al., 2018). These conditions create more possibilities for active snowpack habitats to develop, thrive and enrich coastal waters.
Although the onset of snow melt at the beach site produced bio-limiting, nutrient-rich waters, this study clearly shows that the enrichment is only temporary. For example, the majority (if not all) of the nitrate (NO), DFe and Zn fluxes produced during the ablation season were exported within the elution phase of the first 2 weeks of melt (see Figures 7–9). However, although this enriched flux of nutrients was not sustained, it occurred at a critical time early in the summer when marine primary production typically experiences its first bloom (Dierssen et al., 2002). Furthermore, whilst Zn in meltwaters has received almost none of the scientific attention as a bio-limiting micro-nutrient that has been directed toward DFe, studies from marine environments suggest that Zn can have a larger impact on the global carbon cycle than DFe (Torel et al., 1994). A recent study by Weber et al., 2018) also suggests that Zn deficiencies in the Southern Ocean are more sensitive to climate-driven changes than previously thought. Therefore, identifying a “new” early season source of this bio-limiting micro-nutrient in the form of melting snow, is an important result and deserves attention in future studies of the Polar Regions.
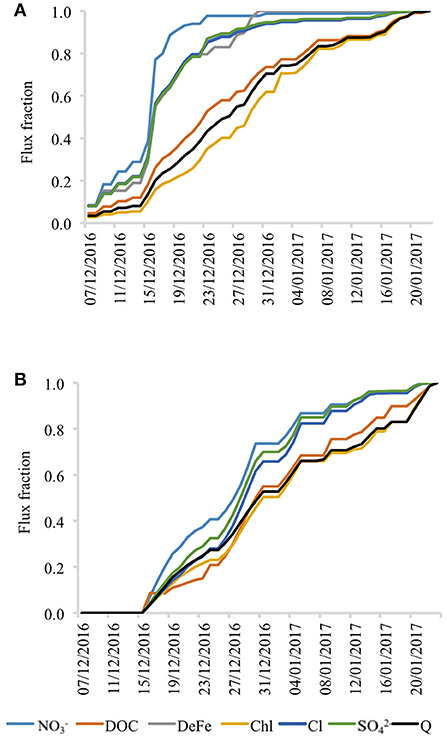
Figure 9. Normalized flux of selected chemical species indicating differences in seasonal dynamics between monitoring sites. (A) Beach snowmelt and (B) Inland snowmelt.
A “split” dynamic in temporal distribution of solute export from beach and inland snowpack (whereby certain species are delivered early in the summer during the elution phase, whilst others are delivered more gradually throughout it) is also demonstrated by Figure 9. At the beach, species such as NO, DFe, Cl, and SO were released from the snowpack rapidly at the beginning of the season. Otherwise DOC or in-vivo chlorophyll were gradually exported by snowmelt throughout the entire summer period. In contrast, the inland site showed rather different solute export dynamics by lacking the distinctive split behavior (and lacking an elution phase) because all the ions were released with broadly similar rates and timing.
Since the contribution of runoff to the fluxes of nutrients was negligible after the elution period, it is most likely that they were consumed/assimilated by microbial activity. These processes should be visible in the solute mass balance (Hodson, 2006), and so we compared the cumulative mass fluxes in runoff to the initial mass loading in the snowpack (Figure 10). The comparison revealed that the seasonal export of NO by snowmelt at the beach site amounted to just 10 % (0.1 in Figure 10) of the initial snowpack load, demonstrating clear assimilation effects, because the corresponding value for Cl−, conservative ion, was ca. 93% (0.93). In contrast to the snowpack at the beach, export efficiencies of NO and Cl− at the inland site were similar (0.22 and 0.21, respectively), suggesting a less active microbial environment (i.e., less NO assimilation) and sporadic melt. The inland snowpack was also able to produce NO fluxes throughout the entire runoff period, although with much lower concentrations (and also no DFe). Interestingly, the inland snowpack also demonstrated modest NO assimilation, as well as a loss of DOC.
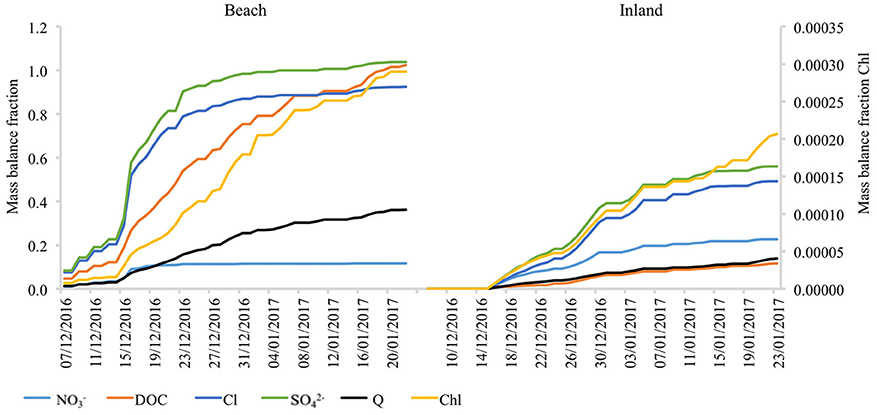
Figure 10. Ratio of cumulative export of species to initial snowpack loading at the beach site and at the inland site. Values of 1 represent the total export by meltwater matching the total initial snowpack loading. Values below 1 suggest assimilation or retention within the snowpack, while values above 1 indicate that production must have occurred.
Lastly, sites where only stream waters were sampled, such as Snowfields 1–2 and Coastal 1–4, indicate that environments influenced by the presence of marine fauna and increased rock-water contact produce meltwaters markedly enriched in DOC, NO, PO, DFe, Zn, and other trace metals. Here, the recorded DOC concentrations were on average up to 500% higher than elsewhere, whilst heavily manured coastal sites produced meltwaters containing a staggering two orders-of-magnitude more NO.
Snowmelt collected in the mountains, where no marine fauna was present as a result of the high elevation and significant distance from the coast, only showed increased concentrations of major ions and trace metals due to ion acquisition through rock-water contact. Here DOC and NO levels remained low and no PO was detected. This once again shows that low altitude, coastal/beach environments are important “power plants” of biological activity.
Our study therefore shows that the complexity and heterogeneity of the Antarctic snowpack environment must be appreciated in environmental studies investigating the effects of climate change on biogeochemical cycles of carbon, iron, nitrogen or other chemical species. Furthermore, it is time to acknowledge that the Antarctic contribution to the global carbon cycle is a process far more complex than current models can simulate, therefore upscaling to account for vast areas of the Antarctic continent that are likely to harbor ecosystems must include significant ground truthing to produce robust and accurate results.
Solute Acquisition by Antarctic Snowpack and Snowmelt
In order to further explore the source of solutes in the snowpack and snowmelt Principal Component Analysis was undertaken using SPSS software. This statistical method is commonly used to describe multivariate data by reducing the number of variables to a small amount of Factors or Components, while still retaining the information in the original data set. The analyses performed on both snowpack and snowmelt samples extracted two major components from the snowpack data and four from the snowmelt. Therefore, the results (presented in Table 3) indicated that the snowpack ions were primarily associated with marine aerosols (Component 1, on the account of strong loadings of Na+, Cl−, Br−, K+, Ca2+, Mg2+, Sr, SO) and microbial activity (Component 2, on the account of strong loadings of DOC, TIC, TC, Mn, Zn). However, in the case of the snowmelt analysis, other components were also significant. Marine aerosols (Component 1, Na+, Cl−, Br−, K+, Mg2+, Sr), were accompanied by Component 2, indicative of chemical weathering of dust and debris (on the account of strong loadings of Ca2+, SO, TIC, DFe), Component 3, representing trace metal acquisition (such as DFe, Cu and Co), as well as Component 4, representing biological activity (NO, DOC, TC, PO).
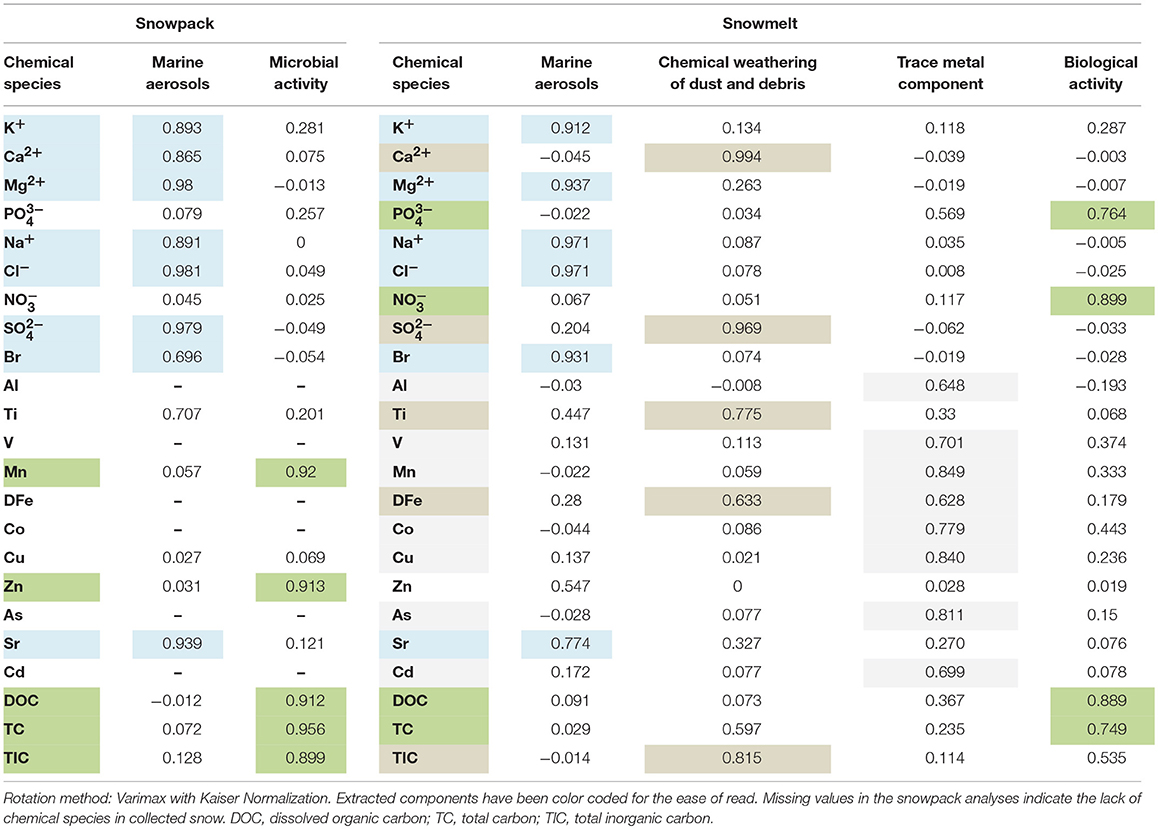
Table 3. Results of the principal component analyses performed on the snowpack and snowmelt samples.
Analyzing the results of principal component analyses requires certain amount of interpretation based upon prior knowledge of the processes within the environment that cause correlations between different solutes. While components containing Cl−, and Na+ are easy to interpret as marine aerosols, differentiating between chemical weathering and microbial activity is more challenging and depends on identifying whether solutes could have been involved in inorganic or microbially-mediated reactions. Here, in case of the snowpack component, we have decided to assign Component 2 to microbial processes, based on elimination of chemical weathering and known effects of microbial activity upon DOC, Mn, and Zn (see above). Similarly, the snowmelt Component 4 attributed to biological activity, most likely results from a combination of microbial processes and the presence of marine fauna (present only at coastal sites). It is well-known that seals, penguins, and other birds strongly influence biogeochemical cycling of nitrogen, phosphorus, and carbon in such environments (Lindeboom, 1984; Park et al., 2006). The enrichment in DOC, DFe, and in-vivo chlorophyll in those meltwaters is visible in Figures 6C,D, 7A, and could be most likely linked to fecal material. However, one also needs to consider that covariance between DOC, in-vivo chlorophyll and DFe in Antarctic meltwaters can be linked to microbial processes driven by primary production and involving either iron reduction or DOC-DFe binding (Hodson et al., 2017b).
The differences uncovered between the snow and its runoff therefore suggest that solute acquisition following microbial and weathering reactions must be accounted for in order to predict the impact of changing snowmelt runoff on the biogeochemistry of aquatic ecosystems in the maritime Antarctic. A similar conclusion was reached following the analyses of coastal snow in the South Shetland Islands (see Hodson et al., 2017a), where changes in the biogeochemical and optical properties of the snow were identified in the parent snow, yet no studies of snowmelt outflow were undertaken. The departure of the snowmelt runoff chemistry from that of the parent snowpack shown above clearly indicates that the composition of snowmelt runoff simply cannot be predicted only from snowpack surveys.
Ion Concentration vs. Loading; Location Relevance to Snowmelt Export
Despite the difference in ion concentrations between the sites (Tables 1, 2), Figure 11 indicates that loadings of dissolved ions and DOC in the parent snowpack were generally comparable and exhibited similar dynamics (see Table 4 for data on other ions). Interestingly, the inland snowpack developed greater in-vivo chlorophyll loadings despite being at higher elevation, much further from the coast and with no impact of marine fauna. This indicates that increased snowpack thickness at higher elevations can in fact compensate for the fact that snow there is less biologically active.
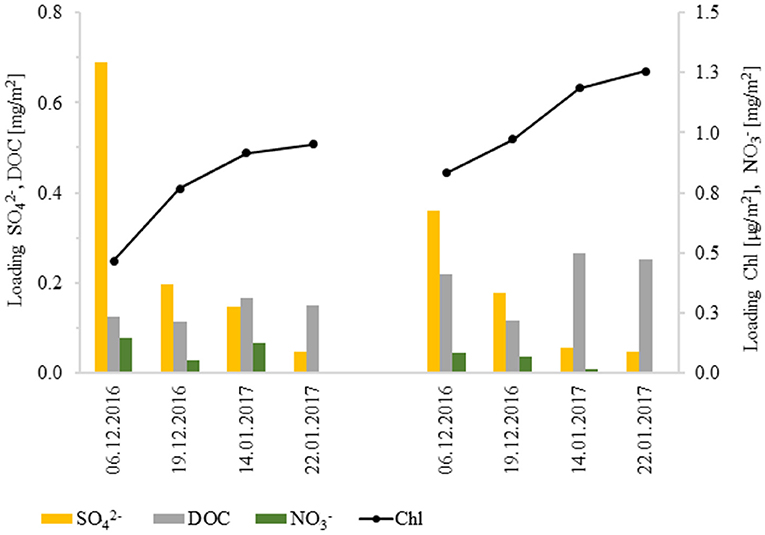
Figure 11. The comparison of snowpack loadings of selected ions, DOC and in-vivo chlorophyll at both the Beach (left) and the Inland (right) sites.
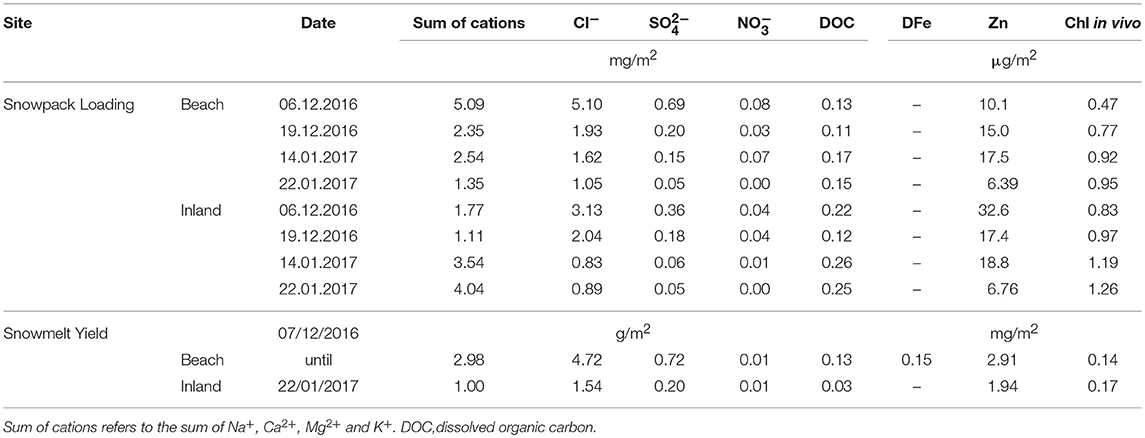
Table 4. Loadings and yields of selected dissolved ions, dissolved organic carbon as well as in-vivo chlorophyll in the snowpack and their corresponding snowmelt at the beach and the inland sites.
Interestingly, increased inland snowpack thickness does not create larger yields of dissolved ions or organic carbon (Table 4) suggesting that retention of these species within the snowpack environment takes place. The preservation of solutes, DOC and in-vivo chlorophyll at inland sites will therefore most likely occur until either glacier flow advects the snow into the ablation area, or the ablation area expands in response to further climate warming. Nonetheless, quite marked changes in the ablation regime would be required before a significant proportion of the inland snowpack nutrient resources were mobilized into runoff. Unfortunately, climate change in Antarctica is a fact, and consequent adjustments of the ablation regime have already been forecasted in the Antarctic Peninsula region (see Trusel et al., 2015).
Conclusions
The rapidly changing environment of the Antarctic Peninsula pushes us to study current conditions in the region and to predict the environmental impact of its changing climate. Much attention has been given to the development of conceptual models estimating the influence of freshwater and nutrient export upon the Southern Ocean ecosystem, yet without sufficient data to incorporate all the processes and quantify the fluxes. The detailed seasonal dynamics and release of ions, nutrients and carbon from Antarctic snowpacks has until now been overlooked. Our first of its kind, comprehensive study of the Antarctic snowpack and snowmelt biogeochemistry therefore addresses this important knowledge gap.
1. We show that the snowpack environments closest to the coast produced meltwaters enriched in dissolved and particulate organic carbon (the latter being detected as chlorophyll-containing cells) as well as key bio-limiting nutrients such as DFe and Zn. Here, the early melt at the beginning of ablation season was enriched by the elution process. Later in the season, marked meltwater enrichment was associated with the presence of marine fauna and ion acquisition following weathering reactions. Low elevation sites closest to the coast and influenced by marine fauna produced meltwaters enriched in DOC and NO that were from 500% (DOC) to two orders of magnitude (NO) higher than elsewhere. We have therefore identified low elevation, coastal areas of the Antarctic Peninsula as “power plants” of microbial activity supplying (macro- and micro-) nutrients to near-shore marine ecosystem.
2. We also show that there is a split dynamic and a distinct seasonality in dissolved ion concentrations within Antarctic snow, as well its snowmelt, whereby species such as NO, SO, or Cl− are exported from the snowpack early in the summer (during the elution phase) whilst others such as dissolved and particulate carbon are delivered more gradually throughout it.
3. We show that the winter accumulation of coastal snow creates a coastal fringe of biogeochemically active snow that plays a significant role in the land-ocean flux of labile carbon and nutrients liberated by snowmelt.
4. In addition, we found that the underlying perennial snow layers also provide a major source of dissolved and particulate organic carbon to meltwater. Therefore, so far ignored multiannual snowpacks that have the potential to further enrich the biogeochemical impact of snowmelt runoff into downstream ecosystems, deserve our attention in future studies. Especially in the light of climatically driven changes that occur in the Antarctic Peninsula.
5. Differences in the biogeochemical signature also depend upon snowpack location. Sites further from the coast and/or at much higher elevation (i.e., not influenced by marine fauna) seem to lack the physical conditions and chemical characteristics to produce enriched melt. However, increasing snowfall with elevation means that the nutrient resource existing within the parent snowpack can be just as significant, even if it is more dilute. It needs to be taken into consideration that marked changes in the ablation regime have already been forecasted in the Antarctic Peninsula region (Trusel et al., 2015).
6. Lastly, we show that a number of factors including environmental characteristics (elevation and distance from the coast), geochemical heterogeneity and biogeochemical processes within snowpacks, make simple snowpack surveys performed in Antarctica insufficient to predict the nutrient, trace metals, labile carbon, and chlorophyll fluxes carried by snowmelt runoff into the marine environment.
Considering the above, we should rethink our approach to predictive and conceptual modeling of nutrient and carbon release from Antarctica, question the use of satellite measurements of snowpack chlorophyll as a basis for estimation of Antarctica's contribution to the global carbon cycle, and return to significant field-based research in Antarctica despite its challenges.
Author Contributions
AN acquired funding, collected samples, and wrote the manuscript, with editorial input from the remaining authors. The laboratory data obtained by AN, AVT, and AH were compiled and analyzed by AN.
Funding
The study was funded by British Antarctic Survey and Natural Environmental Research Council grant Collaborative Antarctic Science Scheme CASS-120 to AN. The authors also acknowledge Natural Environmental Research Council grant NE/H014446/1 to AH and ERC StG 307582 to AVT for analytical costs.
Conflict of Interest Statement
The authors declare that the research was conducted in the absence of any commercial or financial relationships that could be construed as a potential conflict of interest.
Acknowledgments
We thank British Antarctic Survey for logistical support, the staff of Rothera Research Station for support during fieldwork and the staff of Bonner Laboratory for support during laboratory work whilst at Rothera. We thank Dr. Stephen Reid from Leeds University for analytical services.
Supplementary Material
The Supplementary Material for this article can be found online at: https://www.frontiersin.org/articles/10.3389/feart.2018.00201/full#supplementary-material
References
Bertler, N., Mayewski, P. A., Aristarain, A., Barrett, P., Becagli, S., Bernardo, R. T., et al. (2005). Snow Chemistry across Antarctica. Earth Science Faculty Scholarship.
Budhavant, K. B., Rao, P. S. P., and Safai, P. D. (2014). Chemical composition of snow-water and scavenging ratios over coastal Antarctica. Aerosol Air Qual. Res. 14, 666–676. doi: 10.4209/aaqr.2013.03.0104
Buys, Z. (2014). Chemistry of the Sea Ice/Snowpack/Atmosphere System in Coastal Antarctica. Doctoral thesis, UEA.
Carpenter, E. J., Lin, S., and Capone, D. G. (2000). Bacterial activity in south pole snow. Appl. Environ. Microbiol. 66, 4514–4517. doi: 10.1128/AEM.66.10.4514-4517.2000
Death, R., Wadham, J. L., Monteiro, F., Le Brocq, A. M., Tranter, M., Ridgwell, A., et al. (2014). Antarctic ice sheet fertilises the Southern Ocean. Biogeosciences 11, 2635–2643. doi: 10.5194/bg-11-2635-2014
Dierssen, H. M., Smith, R. C., and Vernet, M. (2002). Glacial meltwater dynamics in coastal waters west of Antarcic peninsula. Proc. Natl. Acad. Sci. U.S.A. 99, 1790–1795. doi: 10.1073/pnas.032206999
Downes, M., Howard-Williams, C., and Vincent, W. F. (1986). Sources of organic nitrogen, phosphorus and carbon in Antarctic streams. Hydrobiologia 134, 215–225. doi: 10.1007/BF00008490
Engel, Z., Láska, K., Nývlt, D., and Stachon, Z. (2018). Surface mass balance of small glaciers on James Ross Island, north-eastern Antarctic Peninsula, during 2009–2015. J. Glaciol. 64, 349–361. doi: 10.1017/jog.2018.17
Frankenberg, C., O'Dell, C., Berry, J., Guanter, L., Joiner, J., Köhler, P., et al. (2014). Prospects for chlorophyll fluorescence remote sensing from the Orbiting Carbon Observatory-2. Remote Sens. Environ. 147, 1–12. doi: 10.1016/j.rse.2014.02.007
Groot Zwaaftink, C. D., Cagnati, A., Crepaz, A., Fierz, C., Macelloni, G., et al. (2013). Event-driven deposition of snow on the Antarctic Plateau: analyzing field measurements with SNOWPACK. Cryosphere 7, 333–347, doi: 10.5194/tc-7-333-2013
Hodson, A. (2006). Biogeochemistry of snowmelt in an Antarctic glacial ecosystem. Water Resources Res. 42:W11406. doi: 10.1029/2005WR004311
Hodson, A., Heaton, T., Langford, H., and Newsham, K. (2009). Chemical weathering and solute export by meltwater in a maritime Antarctic glacier basin. Biogeochemistry 98, 9–27. doi: 10.1007/s10533-009-9372-2
Hodson, A., Nowak, A., Cook, J., Sabacka, M., Wharfe, E. S., Pearce, D., et al. (2017a). Microbes influence the biogeochemical and optical properties of maritime Antarctic snow. J. Geophys. Res. Biogeosci. 122, 1456–1470. doi: 10.1002/2016JG003694
Hodson, A., Nowak, A., Sabacka, M., Jungblut, A., Navarro, F., Pearce, D., et al. (2017b). Climatically sensitive transfer of iron to maritime Antarctic ecosystems by surface runoff. Nat. Commun. 8:14499. doi: 10.1038/ncomms14499
Koffi, E. N., Rayner, P. J., Norton, A. J., Frankenberg, C., and Scholze, M. (2015). Investigating the usefulness of satellite-derived fluorescence data in inferring gross primary productivity within the carbon cycle data assimilation system. Biogeosciences 12, 4067–4084. doi: 10.5194/bg-12-4067-2015
Krinner, G., and Genthon, C. (2003). Tropospheric transport of continental tracers towards Antarctica under varying climatic conditions. Tellus B Chem. Phys. Meteorol. 55, 54–70. doi: 10.3402/tellusb.v55i1.16354.
Legrand, M., Ducroz, F., Wagenbach, D., Mulvaney, R., and Hall, J. (1998). Ammonium in coastal Antarctic aerosol and snow: role of polar ocean and penguin emissions. J. Geophys. Res. Atmos. 103, 11043–11056.
Lieser, J. L., Curran, M. A. J., Bowie, A. R., Davidson, A. T., Fraser, A. D., Galton-Fenzi, B. K., et al. (2015). Antarctic slush-ice algal accumulation not quantified through conventional satellite imagery: beware of the ice of March. Cryosphere 9, 6187–6222. doi: 10.5194/tcd-9-6187-2015
Lindeboom, H. J. (1984). The Nitrogen pathway in a penguin rookery. Ecology 65.269–277. doi: 10.2307/1939479
Lyons, W. B., Welch, K. A., and Doggett, K. (2007). Organic carbon in Antarctic snow. Geophys. Res. Lett. 34:L02501. doi: 10.1029/2006GL028150
Lyons, W. B., Welch, K. A., Welch, S. A., Camacho, A., Rochera, C., Michaud, L., et al. (2013). Geochemistry of streams from Byers Peninsula, Livingston Island. Antarctic Sci. 25. 181–190. doi: 10.1017/S0954102012000776
Oliva, M., Navarro, F., Hrbáček, F., Hernández, A., Nývlt, D., Pereira, P., et al. (2017). Recent regional climate cooling on the Antarctic Peninsula and associated impacts on the cryosphere. Sci. Tot. Environ. 580, 210–223. doi: 10.1016/j.scitotenv.2016.12.030
Painter, T. H., Duval, B., Thomas, W. H., Mendez, M., Heintzelman, S., and Dozier, J. (2001). Detection and quantification of snow algae with an airborne imaging spectrometer. Appl. Environ. Microbiol. 67, 5267–5272. doi: 10.1128/AEM.67.11.5267-5272.2001
Park, J.-H., Day, T. A., Strauss, S., and Ruhland, C.h.T (2006). Biogeochemical pools and fluxes of carbon and nitrogen in maritime tundra near penguin colonies along the Antarctic Peninsula. Polar Biol. 30, 199–207. doi: 10.1007/s00300-006-0173-y
Petlicki, M., Szilo, J., MacDonell, S., Vivero, S., and Bialik, R. J. (2017). Recent deceleration of the ice elevation change of Ecology Glacier (King George Island, Antarctica). Remote Sens. 9:520. doi: 10.3390/rs9060520
Sun, Y., Frankenberg, C., Jung, M., Joiner, J., Guanter, L., Kohler, P., et al. (2018). Overview of solar-induced chlorophyll fluorescence (SIF) from Orbiting Carbon Observatory-2: retrieval, cross-mission comparison, and global monitoring for GPP. Rem. Sens. Environ. 209, 808–823. doi: 10.1016/j.rse.2018.02.016
Takeuchi, N., Dial, R., Kohshima, S., Segawa, T., and Uetake, J. (2006). Spatial distribution and abundance of red snow algae on the Harding Icefield, Alaska derived from a satellite image. Geophys. Res. Lett. 33:L21502. doi: 10.1029/2006GL027819
Thomas, E. R., Scott, H. J., Tuckwell, R. R., and Ludlow, E. C. (2015). Twentieth century increase in snowfall in coastal West Antarctica. Geophys. Res. Lett. 42, 9387–9393. doi: 10.1002/2015GL065750
Torel, F. M. M., Reinfelder, J. R., Roberts, S. G., Chamberlain, C. P., Lee, J. G., and Yee, D. (1994). Zink and carbon co-limitation of marine phytoplankton. Nature 369, 740–742.
Trusel, L. D., Frey, K. E., Das, S. B., Karnauskas, K. B., Munneke, P. K., van Meijgaard, E., et al. (2015). Divergent trajectories of Antarctic surface melt under two twenty-first-century climate scenarios. Nat. Geosci. 8, 927–932. doi: 10.1038/ngeo2563
Turner, J., Barrand, N. A., Bracegirdle, T. J., and Convey, P. (2014). Antarctic climate change and the environment: an update. Polar Record. 50, 237–259. doi: 10.1017/S0032247413000296
Vincent, W. F. (2004). Microbial Ecosystems of Antarctica. Cambridge, UK: Cambridge University Press.
Weber, T., John, S., Tagliabue, A., and DeVries, T. (2018). Biological uptake and reversible scavenging of zink in the global ocean. Science 361, 72–76. doi: 10.1126/science.aap8532
Welch, K. A., Lyons, B., Whisner, C., Gardner Ch, B., Gooseff, M. N., McNight, D. M., et al. (2010). Spatial variations in the geochemistry of glacial meltwater streams in the Taylor Valley, Antarctica. Antarctic Sci. 22, 662–672. doi: 10.1017/S0954102010000702
Zhang, Y, Guanter, L., Berry, J. A., van der Tol, C., Yang, X. J, Tang, J., et al. (2016). Model-based analysis of the relationship between sun-induced chlorophyll fluorescence and gross primary production for remote sensing applications. Remote Sens. Environ. 187, 145–155. doi: 10.1016/j.rse.2016.10.016
Keywords: Antarctic DOC, Antarctic snow biogeochemistry, Antarctic snowmelt biogeochemistry, Antarctic snowmelt enrichment, iron enrichment in Antarctic meltwaters, coastal fringe of Antarctic Peninsula, nutrients in snowmelt, snowmelt DOC
Citation: Nowak A, Hodson A and Turchyn AV (2018) Spatial and Temporal Dynamics of Dissolved Organic Carbon, Chlorophyll, Nutrients, and Trace Metals in Maritime Antarctic Snow and Snowmelt. Front. Earth Sci. 6:201. doi: 10.3389/feart.2018.00201
Received: 02 July 2018; Accepted: 26 October 2018;
Published: 21 November 2018.
Edited by:
Julia Ribeiro, Rice University, United StatesReviewed by:
William Berry Lyons, The Ohio State University, United StatesLuke Bridgestock, University of Oxford, United Kingdom
Copyright © 2018 Nowak, Hodson and Turchyn. This is an open-access article distributed under the terms of the Creative Commons Attribution License (CC BY). The use, distribution or reproduction in other forums is permitted, provided the original author(s) and the copyright owner(s) are credited and that the original publication in this journal is cited, in accordance with accepted academic practice. No use, distribution or reproduction is permitted which does not comply with these terms.
*Correspondence: Aga Nowak, YWdhLm5vd2FrLjA5QGdtYWlsLmNvbQ==