- 1Institute of Loess Plateau, Shanxi University, Taiyuan, China
- 2MOE Key Laboratory of Surficial Geochemistry, Ministry of Education, School of Earth Sciences and Engineering, Nanjing University, Nanjing, China
- 3College of Physical Science and Technology, Guangxi University, Nanning, China
- 4Centre of Excellence for Soil Biology, College of Resources and Environment, Southwest University, Chongqing, China
- 5School of Biological Sciences, The University of Western Australia, Perth, WA, Australia
Organic amendments from animal production are commonly used for promoting soil fertility, and their impacts on the residual soil organic carbon (SOC) are of both agricultural and environmental interest. Iron (Fe) in the form of (oxyhydr)oxides has been proposed to play a critical role in long-term SOC preservation by forming Fe-organic associations, though currently a comprehensive understanding of how these Fe-organic associations are regulated by long-term organic amendments is limited. Here, we synthesize information to link Fe (oxyhydr)oxides, SOC sequestration, and long-term organic inputs from both field and laboratory studies. The results show that vigorous Fe mobilization can be regulated by long-term application of organic amendments, and these organically amended soils contained significantly higher concentrations of poorly crystalline Fe that was closely related to SOC storage in both upland and paddy soils. Potential mechanisms are proposed as follows: (1) DOM from the organically amended soils is more likely to co-precipitate with poorly crystalline Fe, and DOM from the inorganically fertilized soils is to a larger extent adsorbed on poorly crystalline Fe. The co-precipitated Fe-OM complexes are more resistant to desorption than the adsorbed OM. (2) DOM extracts from soils treated with organic amendments exhibit a stronger inhibitory effect on the crystallization of poorly crystalline Fe than DOM from inorganically fertilized soils, which may be the consequence of increased numbers of aromatic functional groups. Organic acids in root exudates increased soil mineral availability and the formation of poorly crystalline minerals. Compared to inorganic fertilizers, organic amendments significantly increase (>20%, p < 0.05) the concentration of poorly crystalline minerals in the presence of actual roots. (3) Microbially mediated Fe cycling is strongly linked to the Fe mineralogy in soils, and regulated by long-term organic amendments. Greater consumption of poorly crystalline Fe was observed in inorganically fertilized soil than that in organically amended soil, due to a higher relative abundance of well-known Fe(III) reducers. Conversely, Fe(II) oxidizers, were more abundant, and produced higher levels of poorly crystalline Fe under organic amendments. In conclusion, continuous organic amendments initialize a positive feedback loop for the maintenance of poorly crystalline Fe in soils, which can contribute to enhanced SOC storage.
Introduction
Nearly 80% of the total carbon (C) in terrestrial ecosystems is preserved in soils, and ∼75% of this is in the form of soil organic carbon (SOC) (Lal, 2004). As the largest pool of biologically active C on earth, SOC is a primary indicator of soil health and plays a critical role in maintaining soil fertility. It is thus important for agricultural food production, and the control of greenhouse gas emissions (Schmidt et al., 2011; Lorenz and Lal, 2016). The consensus view of mechanisms of SOC sequestration has changed greatly in recent years, as a result of progress in physics, material sciences, genomics, and computation, as well as in the application of the new techniques of in situ soil microscopy and mass spectrometry. Now there is an increasing view that the persistence of SOC is not primarily a molecular property, but an ecosystem property (Mikutta et al., 2006; Rasmussen et al., 2006; Schmidt et al., 2011; Kleber et al., 2015; Xiao et al., 2015). In particular, interactions with reactive mineral phases, such as poorly crystalline or short-range ordered (SRO) Al-/Fe- (oxyhydr)oxides, has been identified as an important mechanism for stabilization and long-term protection of SOC (Kleber et al., 2005; Kögel-Knabner et al., 2008). Iron (Fe) is the most abundant transition metal on the Earth’s surface, and its biogeochemical cycle is closely related to the dynamics of soil organic matter (Weber et al., 2006a). The poorly crystalline Fe (oxyhydr)oxides, which have been isolated from many soil types, are more effective than the crystalline Fe oxides or oxyhydroxides in stabilizing soil aggregates, even though they may be present in smaller concentrations (Duiker et al., 2003). Moreover, up to 21.5% of the global OC are associated with reactive forms of Fe in soils and sediments (Lalonde et al., 2012).
The maintenance and improvement of soil quality is crucial for sustainable agricultural productivity (Rasmussen et al., 1998; Ludwig et al., 2011), and land use and associated management affect soil structure, properties, and SOC reserves. Organic amendments, such as manure from pig and cattle farms, are commonly used as a substitute for inorganic nitrogen and phosphorus fertilizers for agricultural crop production worldwide. Such continuous organic inputs to soil have led to improvements in crop yields and SOC stocks (Griffiths et al., 2010; Gattinger et al., 2012; Maillard and Angers, 2014; Wen Y.L. et al., 2014). However, caution is also needed in the prolonged agricultural use of heavy metal-containing organic wastes, which can lead to substantial amounts being accumulated in the SOC (e.g., Goodman et al., 1991a, b), and available to plants. Soil minerals can also be affected by fertilization practices, and some recent investigations have shown that management practices, such as the application of organic amendments, can convert crystalline minerals to nano-size forms, which have higher capacity for C binding (Yu et al., 2012; Wen Y. et al., 2014). However, significant changes in SOC due to land management practices occur slowly, and may require many years before they can be detectable by present analytical methods (Rasmussen et al., 1998). Therefore, long-term experiments with agro-ecosystems are crucial for better evaluating the effects of different fertilization regimens on soil quality and system sustainability.
Although there has been increasing recognition of the importance of SRO/reactive Fe minerals in protecting soil C, there is still a gap in our understanding of Fe-mediated protection of SOC in agricultural soils under long-term fertilization. It has been long known that poorly ordered Fe oxide species are associated with the SOC extracted from podzol soils by alkaline EDTA and NH4OH (McBride et al., 1983), but it is only relatively recently that Yu et al. (2012) proposed that non-crystalline minerals were formed in soluble SOC from 21 years’ long-term organically amended soils. Subsequent research based on both field and laboratory studies has provided more information on the regulation of interactions between Fe (oxyhydr)oxides and SOC by long-term application of organic amendments. Still, a systematic and mechanistic knowledge of effects of soil organic amendments on the SOC storage ability of Fe (oxyhydr)oxides is scarce, although organic amendments were proposed to increase the amount of SOC by direct C input from the organic amendments and an indirect effect from increased net primary production in most of the current studies. The aim of this synthesis article is to present a collection of recent arguments that address improvements in SOC stability through regulation of the species and mineralogy of Fe by long-term organic amendments. We hypothesize that long-term organic amendments could markedly influence the soil biogeochemical properties which benefit the existence of poorly crystalline Fe in soils, and further favor long-term SOC protection. First, we integrated the information regarding the effects of organic amendments on the reactivity and crystallinity of Fe (oxyhydr)oxides in field studies. Then, potential mechanisms were proposed from the results of controlled microcosm incubation studies, i.e., the effects of organic amendments on the reactivity of Fe (oxyhydr)oxides with soil dissolved organic matter as well as Fe redox cycling microorganisms, and on the occurrence of co-precipitation or adsorption processes between SRO Fe and DOM in those fertilized soils.
Soil Physicochemical Properties, Iron Fractions in Field Samples Under Long-Term Organic or Inorganic Amendments
A description of the physicochemical properties of the soils reviewed in this work is presented in Table 1. Overall, there was considerable variation in the soil pH, SOC content, and Fe mineral fractions among sites and long-term amendments (Table 1). From all of the sites in Table 1 and other studies (Whalen and Chang, 2002; Bhattacharyya et al., 2010; Gattinger et al., 2012; Maillard and Angers, 2014), it is seen that continuous input of organic amendments to the soils markedly increased the amount of SOC compared with no fertilization. This is due to both direct C input from the organic amendments and an indirect effect from increased net primary production. Inorganic amendments increased SOC concentrations to a lesser extent, and was solely the result of input from crop residues (Halvorson et al., 1999; Galantini and Rosell, 2006; Tong et al., 2014). Inorganic amendments (NPK) greatly decreased soil pH compared to organic amendments and no fertilization treatments (i.e., NPKM and Control, respectively). Soil acidification in acidic soils was seriously accelerated under NPK treatment, but was effectively alleviated by organic amendments. Enhancement of soil SRO nanominerals under long-term organic amendments has been suggested as a potential mechanism for increasing the pH in acid soils (Wen Y.L. et al., 2014).
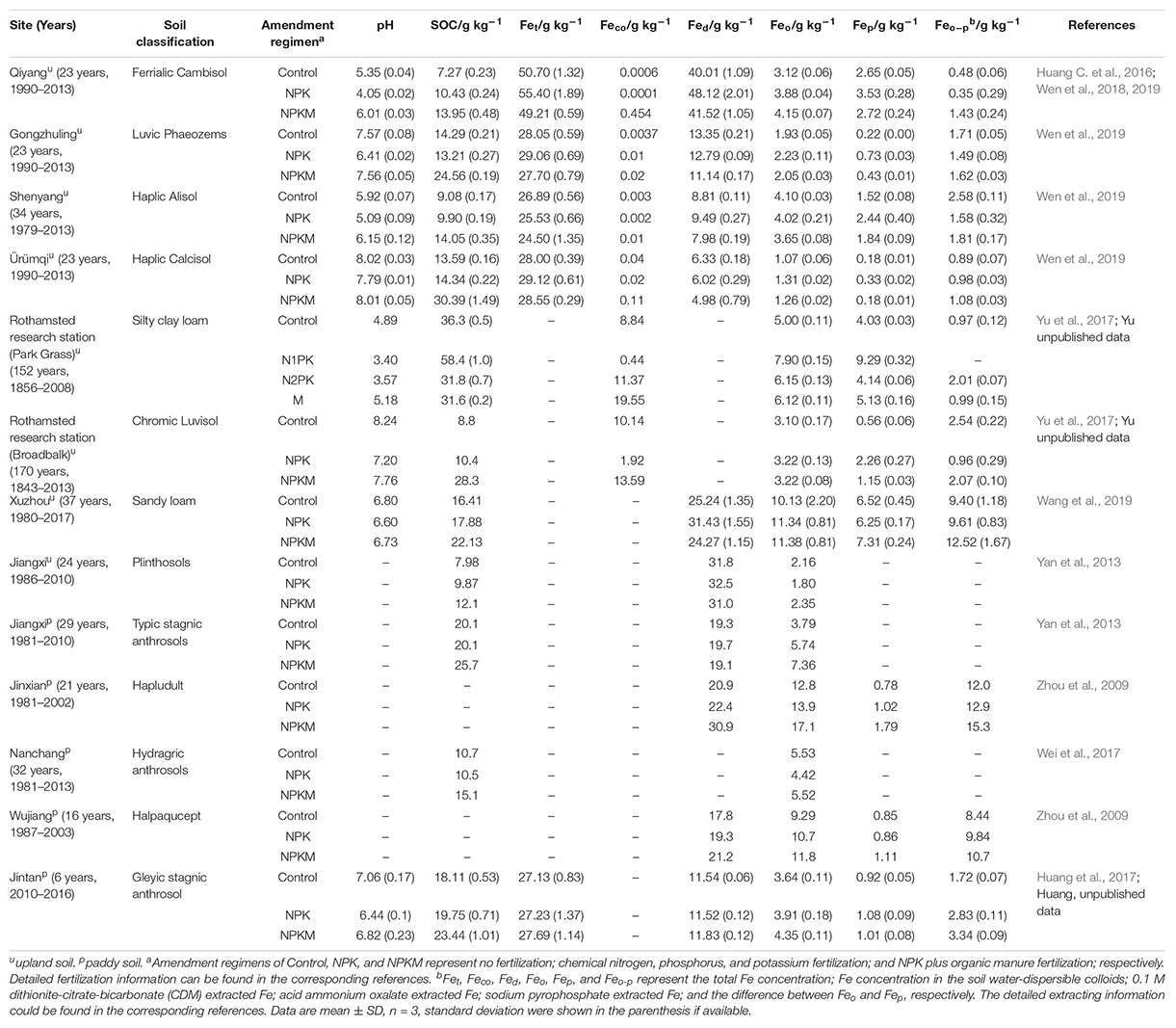
Table 1. Soil physicochemical characteristics and Fe fractions under long-term (6–170 years) organic or inorganic amendments in long-term fertilization experiment sites.
Iron fractions could be significantly altered by long-term fertilization, and the total Fe concentration in the soils reviewed here ranged from 24.5 to 55.4 g kg–1 (Table 1), with the highest in the Ferrialic Cambisol. The different fertilization treatments had little or no effect on the total Fe concentration, although some observations reported a slight decrease in total Fe in NPK and NPKM treated soils compared with the no fertilization control. This is probably a result of Fe uptake by plants or leaching (Wen et al., 2018). However, the Fe concentrations in water-dispersible soil colloids, which could be a good indicator of the availability of a mineral for C binding, was significantly enhanced by long-term organic amendments (by over 2 orders of magnitude at the Qiyang Experiment, and 2–12 times at the Shenyang, Ürümq, Gongzhuling, Park Grass and Broadbalk sites) (Table 1). This index has been ignored by most studies of Fe (oxyhydr)oxides and C associations in soils, including those listed in Table 1 and the reports of Arias Estévez et al. (2016), Coward et al. (2017), and Wang et al. (2019).
It has also been reported that reactive Fe concentrations (or SRO minerals) are significantly increased by organic amendments in both upland and paddy soils (Zhou et al., 2009; Yan et al., 2013; Huang X. et al., 2016; Yu et al., 2017; Wen et al., 2018; Wang et al., 2019). Although this observation is confused by the definition of reactive Fe minerals, it is interesting that the amounts of Fe extracted by acid ammonium oxalate (i.e., Feo) in Table 1 were consistently greater under organic amendments in all paddy soils, but not in upland soils, whereas long-term organic amendments increased the Feo–p values (which is the difference between Feo and Fep), in upland soils. In general, acid ammonium oxalate extracts poorly crystalline Fe (oxyhydr)oxides and organically complexed Fe, whereas pyrophosphate extracts Fe (Fep) primarily from Fe-humus complexes (López-Ulloa et al., 2005; Zhang et al., 2013; Wen et al., 2018). The stabilization by SOC of poorly crystalline Fe is through organo-mineral associations, whereas the Fep is a measure of the formation of organo-metal complexes (Van De Vreken et al., 2016). Studies have demonstrated positive relationships between Feo and SOC (Adams and Kassim, 1984; Huang et al., 2017), and also between Feo–p and SOC (Supplementary Table S1; Torn et al., 1997; Rasmussen et al., 2006; Zhang et al., 2013; Porras et al., 2017; Wen et al., 2019). However, in forest soils it has been observed that the poorly crystalline minerals were not consistently correlated with SOC concentrations, but with SOC turnover times (Porras et al., 2017). This might reflect the comparatively low abundances of poorly crystalline minerals in these soils, as has also been postulated for grassland soils (Masiello et al., 2004). Thus poorly crystalline Fe could better predicate SOC turnover times, whereas organically complexed Fe could be a better predictor of SOC concentrations (Porras et al., 2017), and the processes measured by Feo and Feo–p could both be important for soil C sequestration.
Selective extraction methods may give only operationally defined pools of reactive Fe minerals, and chemical selective extractions have intrinsic limitations due to the artifacts associated with reagent selectivity and their inability to differentiate specific reactive minerals. In recent years, the application of the synchrotron-based X-ray absorption fine structure (XAFS) spectroscopy has been shown to provide complementary information to chemical extraction, and could provide direct identification of reactive Fe minerals (Yu et al., 2017). Compared to inorganic amendments, results from the Fe K-edge XANES (near edge part of XAFS) showed that soil treated with organic amendments contained significantly higher concentrations of poorly crystalline ferrihydrite (Xiao et al., 2015; Huang C. et al., 2016; Yu et al., 2017; Wen et al., 2019). Poorly crystalline Fe minerals have larger specific surface area (about 800 m2 g–1, ferrihydrite) than crystalline forms of Fe (about 200 m2 g–1, goethite) (Eusterhues et al., 2005), and have a greater capacity to stabilize SOC than crystalline minerals (Torn et al., 1997). It has also been well documented that poorly crystalline ferrihydrite and SOC are closely related (Supplementary Tables S1, S2; Eusterhues et al., 2011; Yu et al., 2017; Wen et al., 2019). Thus, the poorly crystalline Fe phase plays a proportionately more important role in long-term SOC storage and stabilization by providing extensive surface areas and chelation capacity for organic molecules to form stable organic-mineral bonds (Torn et al., 1997; Wen et al., 2019). Additional information on the forms of Fe in soil samples can be obtained by Mössbauer spectroscopy. Not only are each of the crystalline Fe minerals characterized by distinctly different spectral parameters, but these parameters are also sensitive to crystallinity and the extent of isomorphous substitution of Fe by Al (Murad and Cashion, 2004). Furthermore, this technique can be used for tracer studies to follow the fate of Fe added to a system in forms enriched in 57Fe. As an example, it was used to determine the effect of pH on the redox status of Fe, and to distinguish between chelated and oxide forms in the presence of large excesses of SOC (Goodman et al., 1991c; Goodman and Cheshire, 2002). Unfortunately, Mössbauer spectroscopy is insensitive and very time-consuming for routine investigations of environmental samples.
Overall, long-term organic amendments could enhance SOC stability by increasing the amounts of reactive poorly crystalline Fe minerals in soils, which then interact with SOC by adsorption or co-precipitation. This is one of the most important mechanisms that could stabilize a significant proportion of SOC over long timescales (Kögel-Knabner et al., 2008; Rumpel et al., 2015), as SOC stability is governed by accessibility rather than recalcitrance (Schmidt et al., 2011; Dungait et al., 2012).
Association Between DOM Under Different Fertilization Regimens and Fe via Co-Precipitation or Adsorption
Microbially- or abiotically mediated Fe redox cycling in the Earth’s crust could trigger the precipitation and dissolution of Fe-rich minerals, and these are thus strongly interlinked with SOC preservation. Adsorption or co-precipitation between SOC and ferrihydrite are the main pathways for the formation of ferrihydrite-OC associations in the natural environment (Pohlman and McColl, 1988; Dahlgren and Marrett, 1991; Eusterhues et al., 2005; Wagai and Mayer, 2007; Chen et al., 2014). In soils, OC could adsorb on pre-existing ferrihydrite surfaces, and the so called “sorptive protection” by ferrihydrite could stabilize OC from biological degradation as well as retarding the transformation of ferrihydrite into more crystalline Fe (oxyhydr)oxides (Kalbitz et al., 2005). Meanwhile, carboxyl groups that dominate DOM structures can provide abundant binding sites for co-precipitation with Fe(III), resulting in adsorption and occlusion of organic molecules in the interstices of ferrihydrite crystals (Eusterhues et al., 2011; Chen et al., 2014; Mikutta et al., 2014). Indeed, the co-precipitation or adsorption of DOM and Fe (oxyhydr)oxides has been proposed to be an important process for stabilizing C and Fe forms in the soil system (Mikutta et al., 2014).
To date, there are limited studies that compare the effects of different associations resulting from OC adsorption vs. co-precipitation on the stability of OC under different fertilizations regimens in agricultural soils. However, the adsorption and co-precipitation processes have been compared by using pure ferrihydrite and DOM extracted from soils at the Qiyang site with different amendments, and Fe K-edge XANES spectra coupled with LCF analysis was applied to determine the relative amounts of insoluble Fe(III) - organic complexes and unreacted ferrihydrite in co-precipitates and adsorption complexes. In the co-precipitation assays, 73% of the total ferrihydrite was precipitated with DOM to form insoluble OM-Fe complexes under NPKM, whereas only 34% was precipitated under NPK (Figure 1, and unpublished data). Significantly, the opposite trend was observed in the adsorption assays. These showed that more than 50% ferrihydrite under NPK, but only 12–20% under NPKM, were adsorbed on the DOM (Figure 1, and unpublished data). Lower pH values in the reaction systems increased the amount of DOM adsorbed on ferrihydrite, especially for the Control and NPKM treatments, and this result is similar to that reported by Chen et al. (2014). More importantly, these results indicate that DOM from soils treated with long-term organic amendments (NPKM) is more likely to co-precipitate with ferrihydrite, whereas adsorption processes are favored by DOM from NPK treated soils. This might be the consequence of different C/Fe ratios in the reaction systems as well as the composition of the DOM in different fertilized soils (Chen et al., 2016). In the initial systems in this work, the molar C/Fe ratio ranged from 0.14 to 1.41, with the highest C/Fe molar ratio in DOM extracted from the NPKM-treated soil, followed by the Control, and lowest for the NPK treatment (Wen et al., unpublished data). However, the overall C/Fe ratios are at the low end of the C/Fe ratio range described in previous research (Chen et al., 2014). That work reported the presence of higher amounts of aromatic groups during adsorption and co-precipitation at low C/Fe ratios, which is consistent with our recent studies. Other researchers have also reported that adsorption and co-precipitation of lignin resulted in a preferential association of aromatic C (Eusterhues et al., 2011). Therefore, higher amounts of aromatic C groups in the tested organically treated soils (Supplementary Table S3) might be responsible for the dominance of co-precipitation processes.
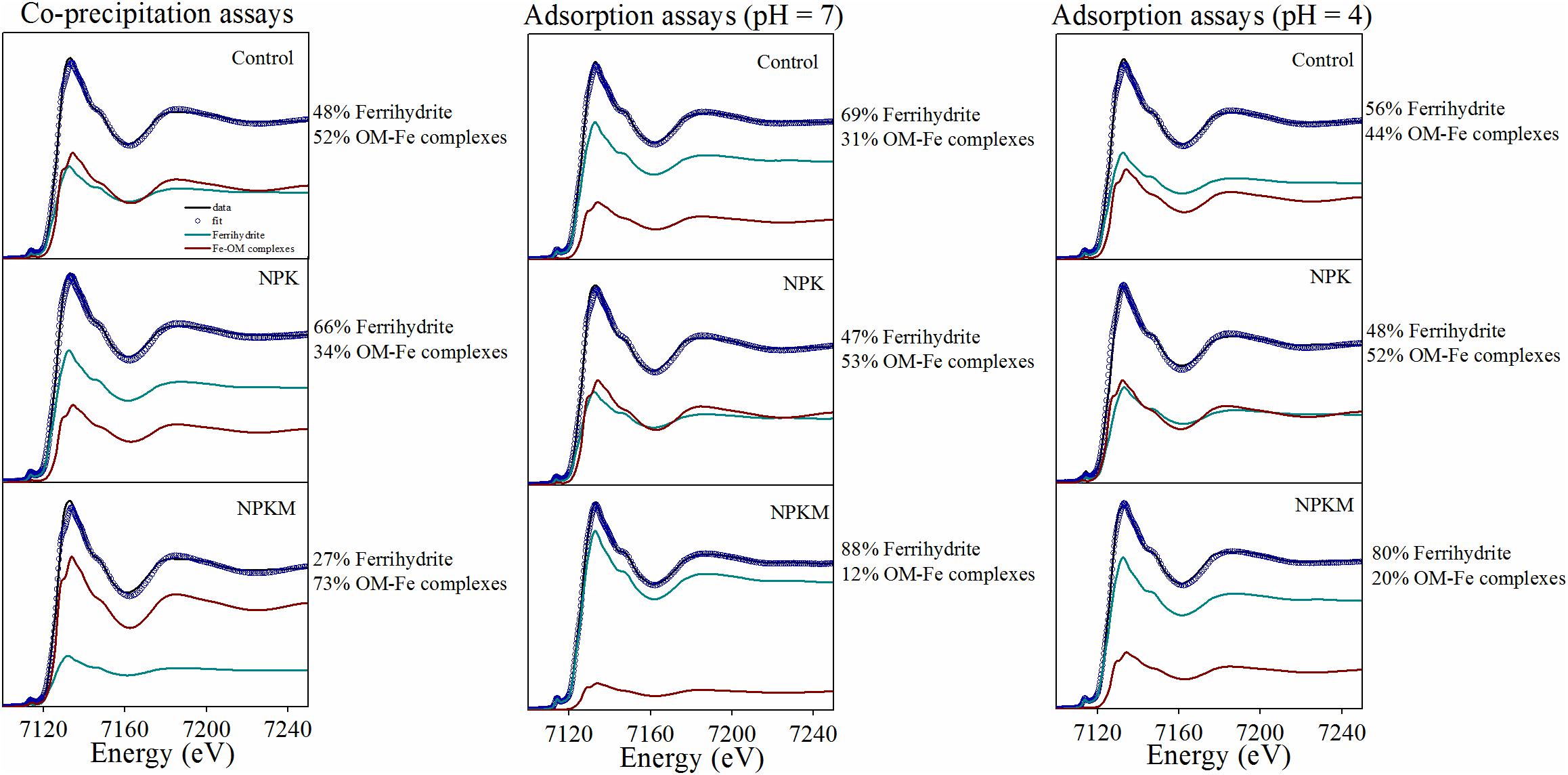
Figure 1. Fits of the Fe K edge XANES spectra of samples from the adsorption and co-precipitation experiments. The black lines represent spectra of samples from the adsorption and co-precipitation experiment, the blue scattered circles represent the LCF results of the sample spectra, and the colored lines represent the reference components for each best fit, i.e., ferrihydrite and insoluble Fe-OM complexes. This figure was adapted from Wen et al., unpublished data.
As the presence of DOM during the co-precipitation process inhibits ferrihydrite growth by blocking the mineral surface sites, and leads to the formation of large aggregates, co-precipitated ferrihydrite often has smaller crystal sizes, greater structural disorder, and lower specific surface area than pure ferrihydrite (Eusterhues et al., 2008; Mikutta et al., 2008; Cismasu et al., 2011; Mikutta, 2011). It has been reported that co-precipitation of ferrihydrite with DOM lowers its crystallinity, as well as the magnetic ordering temperature and internal magnetic field in Mössbauer spectroscopic measurements (Schwertmann et al., 2005), whereas ferrihydrite precipitated with synthetic acid polysaccharides showed stronger aggregation due to the presence of the organic polymers, but only a small decrease in the magnetic hyperfine field, and no significant change in crystal structure (XRD) or the local Fe coordination (Mikutta et al., 2008). Furthermore, co-precipitated C has a relatively higher stabilization than adsorbed C (Chen et al., 2014; Mikutta et al., 2014), and co-precipitation of DOM and Fe makes the more important contribution to SOC storage (Lalonde et al., 2012). Overall, the use of organic amendments can promote the co-precipitation of DOM and ferrihydrite in soils (Figure 1), and thus enhance the long-term protection of SOC.
DOM Composition Influences Crystallization of Fe
Poorly crystalline ferrihydrite is the first solid to precipitate during hydrolysis of Fe(III). But it is thermodynamically unstable. It is likely to and ages to more stable crystalline forms, such as goethite or hematite, after a few months, even at room temperature (Raiswell, 2011; Chen et al., 2015). However, it is well known that there are often large quantities of poorly crystalline ferrihydrite in soils. This discrepancy was firstly explained by the inhibitory effect of SOC five decades ago (Schwertmann, 1966), and later by other researchers (Schwertmann, 1970; Kaiser and Guggenberger, 2007; Kaiser et al., 2007; Chen et al., 2015), who showed that the aging transformations of ferrihydrite can be inhibited or even reversed. It was proposed that boiling for hours in strong alkaline solution would favor the transformation of ferrihydrite to its crystalline counterparts (Schwertmann, 1966), and our unpublished synchrotron-based Fe K-edge XANES data showed that after aging the synthetic ferrihydrite for 4 h in 1 mol L–1 KOH at 100°C, almost all of the poorly crystalline ferrihydrite had been converted to crystalline forms (Figures 2A,B and Table 2). By adding dissolved organic matter (DOM) extracted from soils treated with different long-term amendments, the transformation process was significantly inhibited; only ∼28% of the ferrihydrite was transformed to goethite with OM from the NPKM treatment, but ∼55 and 40% were transformed with OM from NPK and Control treatments, respectively (Figures 2A,B and Table 2). Similar results were observed in another study (Yu et al., 2017). When the DOM solution from NPKM treated soil was diluted by 5 times, so that its concentration was comparable to that in the NPK-treated soil (Supplementary Table S3), ∼ 40% of the ferrihydrite was transformed (Figures 2A,B and Table 2). Therefore, both the DOC concentration and DOM composition could play important roles in the ferrihydrite aging processes.
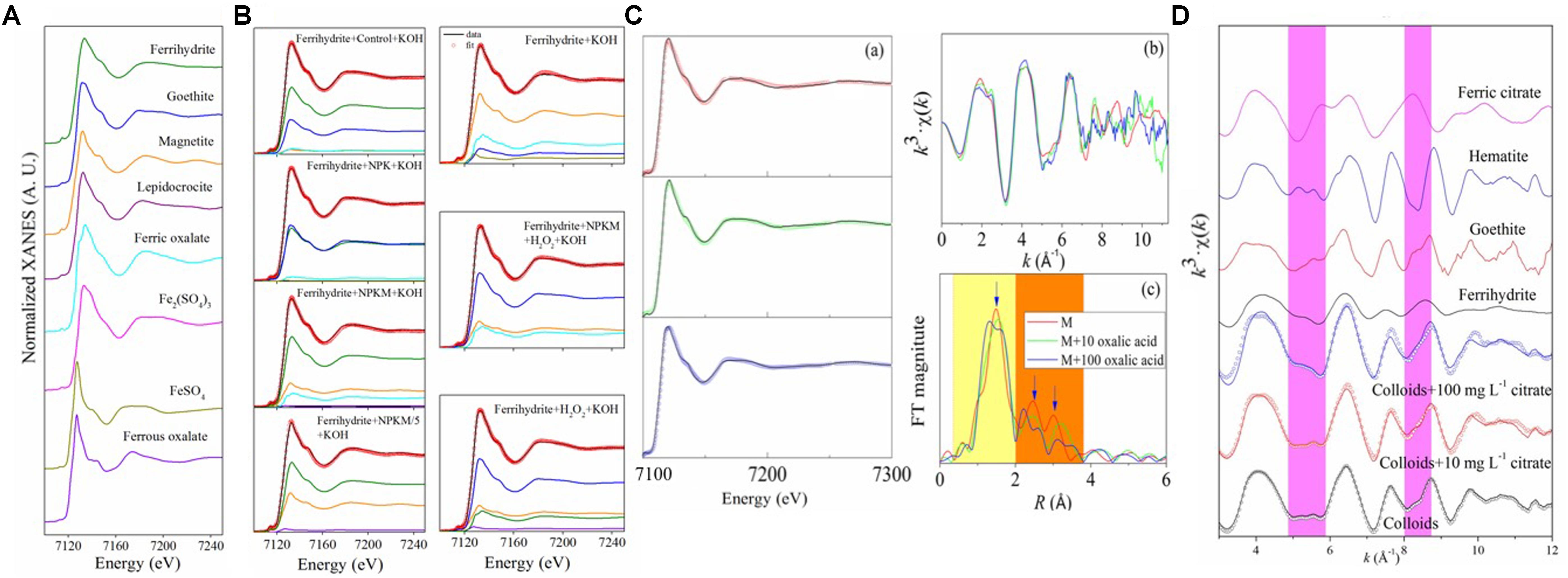
Figure 2. Spectra of the fits of the Fe K edge XANES spectra of samples (B) and references used in the fitting processes (A) from a ferrihydrite aging incubation experiments, soil colloids in the oxalic acid (C), and citric acid (D) stimulated studies. The black line in the (B) is the spectra of each sample, and the red scattered circles represent the LCF results of Fe K-edge XANES normalized sample spectra. The colored lines in the (A) are the spectra of references, and smaller colored lines in the (B) represent the reference components for each best fit in the LCF analysis. (C) Includes LCF results of Fe K-edge XANES normalized sample spectra (scattered circles, a) and spectra of samples (black lines, a), Fe K-edge EXAFS (b), and radial structure function (RSFs, c) in oxalate simulated studies. In (D), the scattered circles represent the LCF results of the sample Fe K-edge EXAFS spectra in the citric simulated studies, the black, and colored lines are the spectra of the samples and references used in the LCF analysis, respectively. This figure was derived from Wen et al. unpublished data (A,B), Huang C. et al. (2016) (C), Yu et al. (2017) (D), and more details can be found in the latter references.
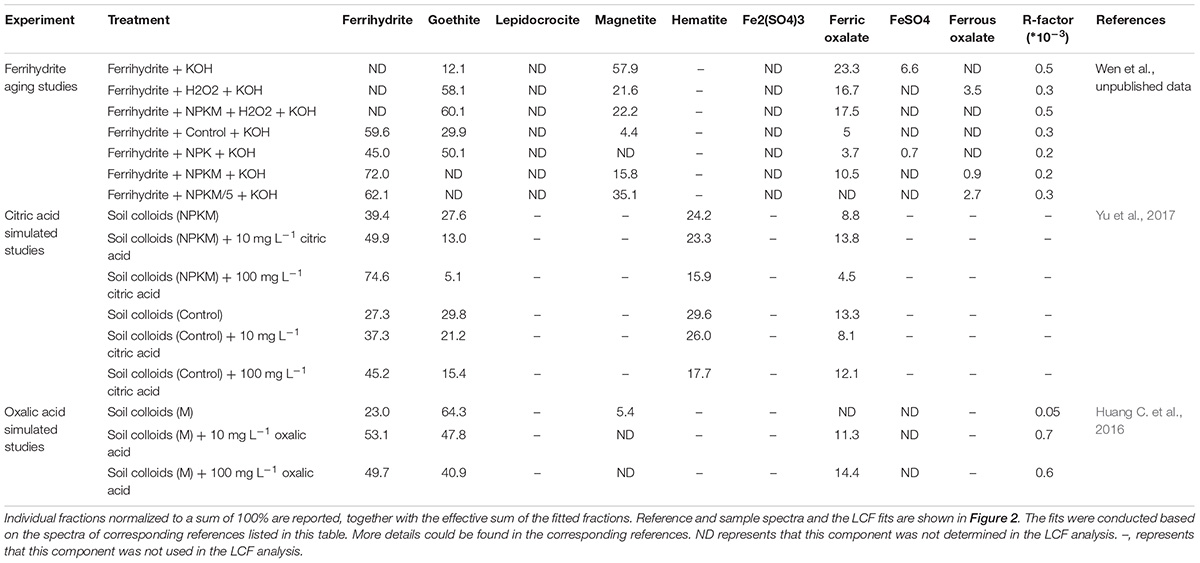
Table 2. Linear combination fitting (LCF) of the XANES models of the adsorbed Fe spectra of samples from the ferrihydrite aging incubation experiment, soil colloids in the citric acid, and oxalate acid stimulated studies with the contributions (in%) of the various standard components required to achieve the best fit.
The results obtained by X ray photoelectron spectroscopy show that aliphatic carbon was dominant and played an important role in binding exogenous Fe in all treatments (Supplementary Table S3). Aliphatic compounds were reported not to interact substantially with kaolinite, smectite, or poorly crystalline Fe, because this fraction was bound to crystalline Fe oxides (Barbera et al., 2008). Soils treated with organic amendments (NPKM) contained more than twice as much aromatic C as those with the NPK treatment (Supplementary Table S3), which was also found in other studies (Lima et al., 2009; Wen et al., 2019). It has been reported that the amounts of aromatic C, originating from either lignin, tannins, or low molecular weight compounds (e.g., simple phenols, phenolic acids, and flavonoids), are positively correlated with the reactive Fe fractions, including Feo (Huang et al., 2018), Feo–p (Wen et al., 2019), and SRO minerals (calculated as Alo–p + 1/2 Feo–p) (Kramer et al., 2012). Precipitation of reactive Fe (oxyhydr)oxides following Fe(II) oxidation could also specifically suppress decomposition of lignin (Hall et al., 2016), and the biogeochemical cycles of Fe and aromatic compounds are thus coupled, especially under repeated redox cycling conditions (Riedel et al., 2013). As a result, the greater amounts of DOC and aromatics that accumulate in organically amended soils could exhibit a strong affinity for poorly crystalline Fe (oxyhydr)oxides, and protect them from transformation to stable crystalline phases, whilst at a same time further improving the SOC sequestration potential in organically amended soils.
Meanwhile, studies have also investigated the impact of root exudates, (i.e., single low-molecular-weight (LMW) acids in artificial root systems or real root exudates), on the chemistry of Fe (oxyhydr)oxides (Colombo et al., 2013; Mimmo et al., 2014; Keiluweit et al., 2015; Huang C. et al., 2016; Yu et al., 2017). A simulation study was conducted by adding citric acid and the colloidal suspensions derived from soils under 23 years’ long-term organic amendments at the Qiyang station, Hunan, China, and incubating for 1 day prior to analyzing the samples by the Fe K-edge EXAFS (extended edge part of XAFS) technique and LCF analysis. The results showed that incubation of soil colloids with citric acid at a concentration of 10 mg L–1 for 1 day decreased the formation of goethite from 28 to 13%, and simultaneously increased ferrihydrite from 39% to 50% of the total Fe; the corresponding numbers for a 100 mg L–1 citric acid treatment were 5% goethite and 75% ferrihydrite (Figure 2D from Yu et al., 2017, Table 2). Similar results have also been reported for simulation experiments based on oxalic acid addition [Figure 2C from Huang C. et al., 2016, Table 2, and (Cheah et al., 2003)]. Therefore, LMW organic acids, could enhance the concentration of poorly crystalline Fe (oxyhydr)oxides in soils, probably by binding to their surfaces and inhibiting the transformation to crystalline phases (Xu et al., 2010; Riedel et al., 2013). Furthermore, the presence of actual roots has been reported to double the release of Fe from soils with or without the application of fertilizers. Besides, compared to inorganic fertilization, organic amendments significantly decreased the mineral mobilization, but markedly increased the concentration of SRO minerals (>20%, p < 0.05) in the presence of roots (Yu et al., 2017). Thus, plant roots, along with organic amendments, act a pivotal part in the formation and maintenance of reactive Fe mineral phases in soils. However, more work is needed to investigate any differences in organic acids in root exudates between organic and inorganic amended soils, and to provide direct evidence as to whether organic acids in root exudates from soil with or without organic amendments can have different effects on the poorly crystalline Fe.
Microbially Mediated Iron Redox Cycling
A variety of Fe (oxyhydr)oxide forms and crystallinities coexist in soils and can be transformed to one another as a result of Fe redox cycling driven by both abiotic and biotic processes (Fortin and Langley, 2005; Kappler and Straub, 2005). The biotic transformations of Fe (oxyhydr)oxides mediated by microorganisms in most soils and sediments are often much faster than the corresponding abiotic reactions (Weber et al., 2006a). Fe has a central role in mediating electron-transfer reactions within all living cells, and undergoes extracellular redox transformations linked to microbial energy generation. Fe(III) can act as a terminal electron acceptor for Fe-reducing microorganisms (Weber et al., 2006a), and the reduced Fe(II) can then be re-oxidized under anoxic conditions either by phototrophic (Hegler et al., 2008) or nitrate-reducing bacteria (Straub et al., 1996). Such reactions help produce the biogenic reactive Fe(III) (oxyhydr)oxides that are then used by Fe(III)-reducing bacteria to sustain Fe(II) production. Thus, Fe can be rapidly recycled within a redox loop, and microbial communities can engage in cyclic coupled iron oxidation and reduction along transition zones (Roden, 2012).
A considerable diversity of microorganisms can reduce Fe(III) using an assortment of electron donors, such as acetate, lactate and H2 (Melton et al., 2014), with the most notable examples being Geobacter (Lovley and Phillips, 1988; Lovley et al., 2011), and Shewanella (Myers and Nealson, 1988). Geobacter are among the first dissimilatory Fe(III) reducers that were shown to be able to reduce Fe(III) minerals. Among Fe(II) oxidizing bacteria, two reactions are potentially widespread in neutral-pH anoxic habitats, anoxygenic photosynthesis and Fe(II) oxidation coupled to NO3– reduction (Miot et al., 2009a). At the genus level, NO3–-dependent Fe(II) oxidation has been documented for Acidovorax, Dechloromonas (Chakraborty and Picardal, 2013), and Psedumonas (Straub et al., 1996). Various photoautotrophic Fe(II)-oxidizing microorganisms including Chlorobium ferrooxidans and Thiodictyon, have been found in natural environments (Croal et al., 2004). The Fe(III) that is produced by microaerophilic Fe(II) oxidizers rapidly precipitates as Fe(III) minerals, which are often referred to as biogenic Fe minerals, and usually occur as nanocrystals. The mineralogy of biogenic Fe(III) phases depends on the rate of Fe(II) oxidation, solution chemistry, and the presence of pre-existing minerals for seeding growth (Larese-Casanova et al., 2010; Posth et al., 2010, 2014; Dippon et al., 2012). However, co-precipitation of Fe and organic substances may disrupt mineral crystallinity, whilst the initial Fe(III) (oxyhydr)oxide nucleation is influenced by sorption of organic molecules, which then inhibit crystal development. As a result, ferrihydrite is the main product from Fe(II) oxidation when substantial quantities of organic matter are present (Childs, 1992).
Long-term organic or inorganic amendments directly or indirectly induce changes in soil physiochemical and biological properties, including Fe redox cycling microbial communities. However, there are only a few studies which address the linkage between soil bacterial communities, microbial Fe redox processes, and soil Fe mineralogy under different long-term fertilization regimens in agricultural soils. Seasonal alternation of wetting and drying serves as a driver of redox cycling of Fe in paddy soils (Ginn et al., 2017), and it has been demonstrated that 20 years’ long-term nitrogen fertilization modulates communities of Fe(III) reducing bacteria, and promotes Fe(III) reduction. Particularly, the well-known Geobacter have been identified as active Fe-reducing bacteria that use ferrihydrite and goethite as electron acceptors, and their activities are greatly increased by long-term nitrogen fertilization in paddy soils (Ding et al., 2015). In addition, by enriching the Fe-redox microbial communities in an incubation experiment, it was observed that the Fe redox processes in upland soils can be strongly influenced by fertilization treatments (Wen et al., 2018). Specifically, 23 years’ long-term inorganic fertilization enhanced Fe(III)-reducing bacteria, especially Geobacter (Figure 3), and resulted in increased ferrihydrite consumption. In contrast, soils that experienced long-term organic amendments contained more Fe(II) oxidizers, most notably Anaerolinea, and these can oxidize Fe(II) when NO3– is present. Furthermore, Pseudononas, a NO3– dependent Fe(II) oxidizer (Straub et al., 1996), was also enriched in organically treated soil incubations (Figure 3). Therefore, in organic fertilization treatments, large amounts of poorly crystalline ferrihydrite are produced at the end of the Fe(II) oxidation stage of the microcosm incubation.
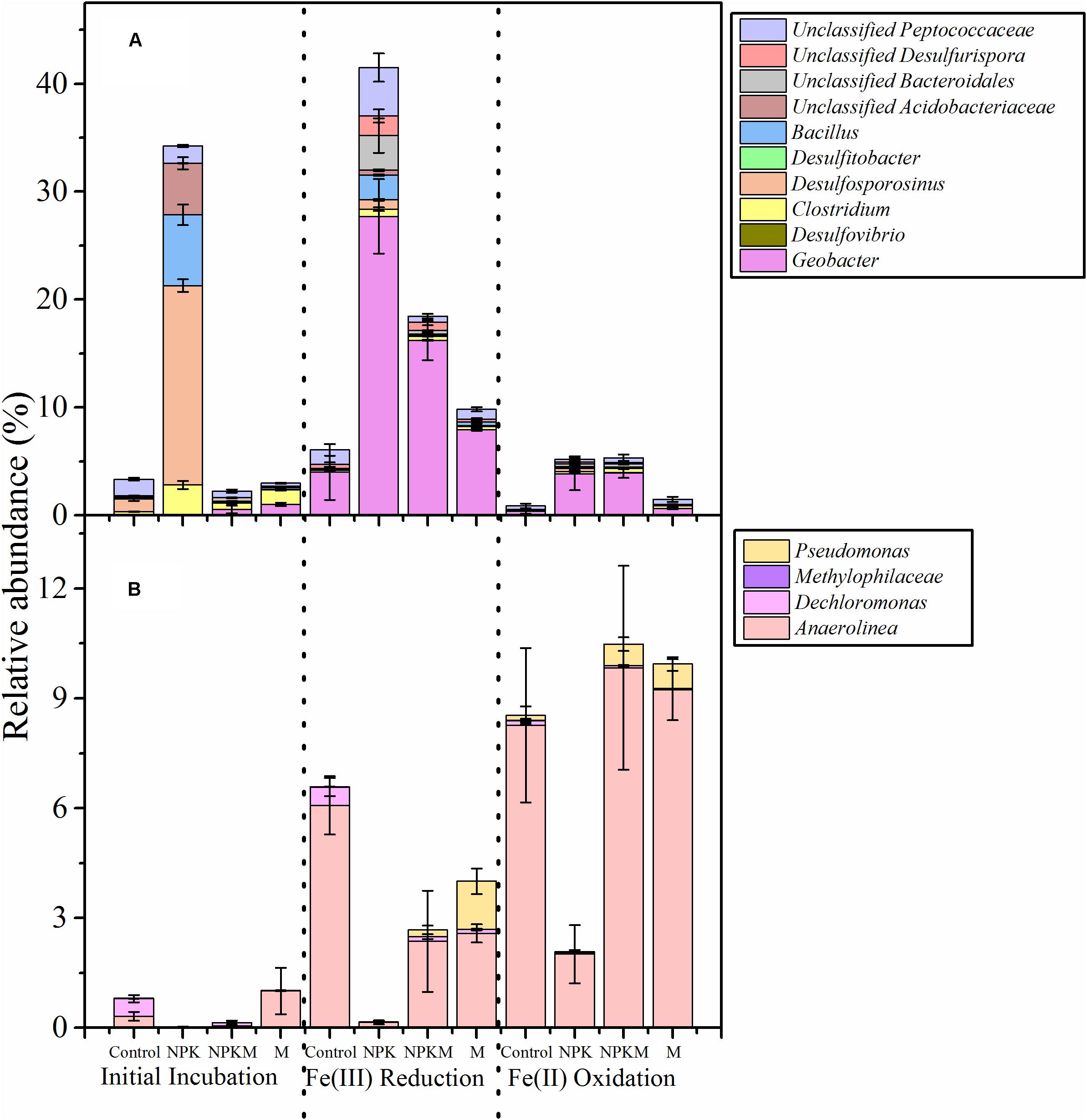
Figure 3. Relative abundance of putative dissimilatory Fe(III)-reducing bacterial (A) and Fe (II)-oxidizing bacterial (B) community structures at the genus level at the initial incubation stage, the end of the Fe(III) reduction stage, and the end of the Fe(II) oxidation stage in each sample. Abundance is expressed as the average percentage of targeted sequences out of the total high-quality bacterial sequences of samples from triplicate plots from each fertilization treatment. Error bars indicate standard error of triplicates. This figure was adapted from Wen et al. (2018) and more details can be found in this reference.
In upland soils, the occurrence of heavy rainfall creates oscillations between reducing and oxidizing conditions, and anoxic micro-climate gradients can be created by interactions between plant roots and soil aggregates. Long-term applications of organic amendments increase the contents of macro-aggregates (Tong et al., 2014). These then potentially create more anoxic environments for Fe redox microbes, as well as building up elevated SOC and DOC levels, and supporting greater diversity and abundance of anaerobic Fe microbial communities. In addition, microbial Fe-cycling communities that are present and active in anoxic soil environments that are experiencing shifts in organic C and NO3– are likely to be analogous to those in aerobic/anaerobic interfacial environments (Weber et al., 2006b; Coby et al., 2011). In soils treated with organic amendments with higher levels of organic C and NO3– (Wen et al., 2018), and larger amounts of aggregates, it could be inferred that initially the inputs of organic C are relatively high compared with NO3–, and available NO3– might be depleted by organotrophic NO3– reduction, which then allows microbial Fe(III) reduction to occur according to the thermodynamic sequence (Schlesinger, 2005). During subsequent periods of reduced organic C loading, but with sustained nitrification, the rates of NO3– re-supply may exceed the rates of organotrophic NO3– reduction, and result in the availability of NO3– for NO3–-dependent Fe(II) oxidation. In NPK-fertilized soils with lower levels of organic C and NO3–, the absence of available NO3– may result in the loss of Fe(II) oxidation, and the production of relatively lower levels of poorly crystalline ferrihydrite (Wen et al., 2018).
The above-mentioned results now show a basic understanding of the linkage between microbially mediated Fe cycling, soil Fe mineralogy, and long-term fertilization in agricultural soils, but more work is still needed. For instance, the scenario proposed above was deduced for a Ferralic Cambisol soil (Wen et al., 2018), but a wider range of field experiments is needed to provide a more general understanding of the effects of different fertilization regimens on Fe redox cycling. In addition, photoferrotrophs have been reported to produce poorly crystalline Fe(III) (oxyhydr)oxides by oxidizing Fe(II) using light energy (Kappler and Newman, 2004; Miot et al., 2009b). Although they are common in numerous environments (Hegler et al., 2012), the ecological roles of these phototrophic Fe(II) oxidizers in Fe redox cycling are still unknown, particularly in agricultural soils under long-term organic or inorganic amendments (Melton et al., 2014). Furthermore, the quantitative contributions of different types of Fe oxidizers to Fe cycling in agricultural soils have not yet been reported. Moreover, the gene families that encode proteins with a potential role in Fe cycling within different microbial taxa are generally non-specific and until now have low sequence identity (Melton et al., 2014). To date few studies have investigated functional gene differences in the microbial communities, which could better link Fe-redox community structure to function, and there are currently no reliable quantitative, direct methods to determine the rates of Fe reduction, or oxidation.
Finally, other mechanisms might also be involved in the Fe-OC dynamics in soils under different fertilization treatments. For instance, in a rice-wheat cropping system, seasonal shifts in redox conditions, coupled with corresponding dynamic processes of soil aggregation, disaggregation and re-aggregation, could be an important driver of the C cycle (Huang et al., 2018). During seasonal dynamic redox cycling, organic amendments could improve soil macro-aggregation, and promote liberation of Fe (oxyhydr)oxides, and production of SRO-Fe minerals. These could selectively adsorb aromatic organic compounds by forming aromatic-Fe complexes, which then co-precipitate at the aggregate interfaces for further SOC protection (Huang et al., 2018).
Conclusion
This paper has synthesized information that is currently available on the interactions between Fe (oxyhydr)oxides and SOC that result from long-term application of organic amendments. Poorly crystalline Fe minerals in long-term amended soils were significantly increased by several mechanisms that might interfere with the so-called “aging-rejuvenation cycle” in soil as proposed in Figure 4. First, DOM from the organically amended soils is more likely to co-precipitate with poorly crystalline Fe, and DOM from the inorganically amended soils is to a larger extent adsorbed on poorly crystalline Fe. The co-precipitated Fe-OM complexes are more resistant to desorption than the adsorbed OM. Second, the DOM extracts from organic amended soils contain higher concentration of aromatic functional groups, and exhibit stronger inhibitory effects on crystallization of poorly crystalline Fe compared with inorganically amended soils. Furthermore, microbially mediated Fe cycling is regulated by long-term inorganic/organic inputs. A higher relative abundance of the Fe(III) reducer Geobacter resultes in greater consumption of poorly crystalline Fe in inorganically amended soils, conversely, Pseudomonas and Anaerolinea are more abundant, and produce higher levels of poorly crystalline Fe under organic amendments. Overall, this work implies that continuous organic amendments to soils could initialize a positive feedback loop for the presence and maintenance of poorly crystalline Fe, which in turn helps preserve SOC over longer time scales. Thus the development of a comprehensive understanding of SOC-Fe associations regulated by agricultural management practices might be of crucial importance for enhancing soil carbon sequestration for sustainable management of SOC in modern agroecosystems, and for improving our understanding of C cycling processes under the global environmental change scenarios.
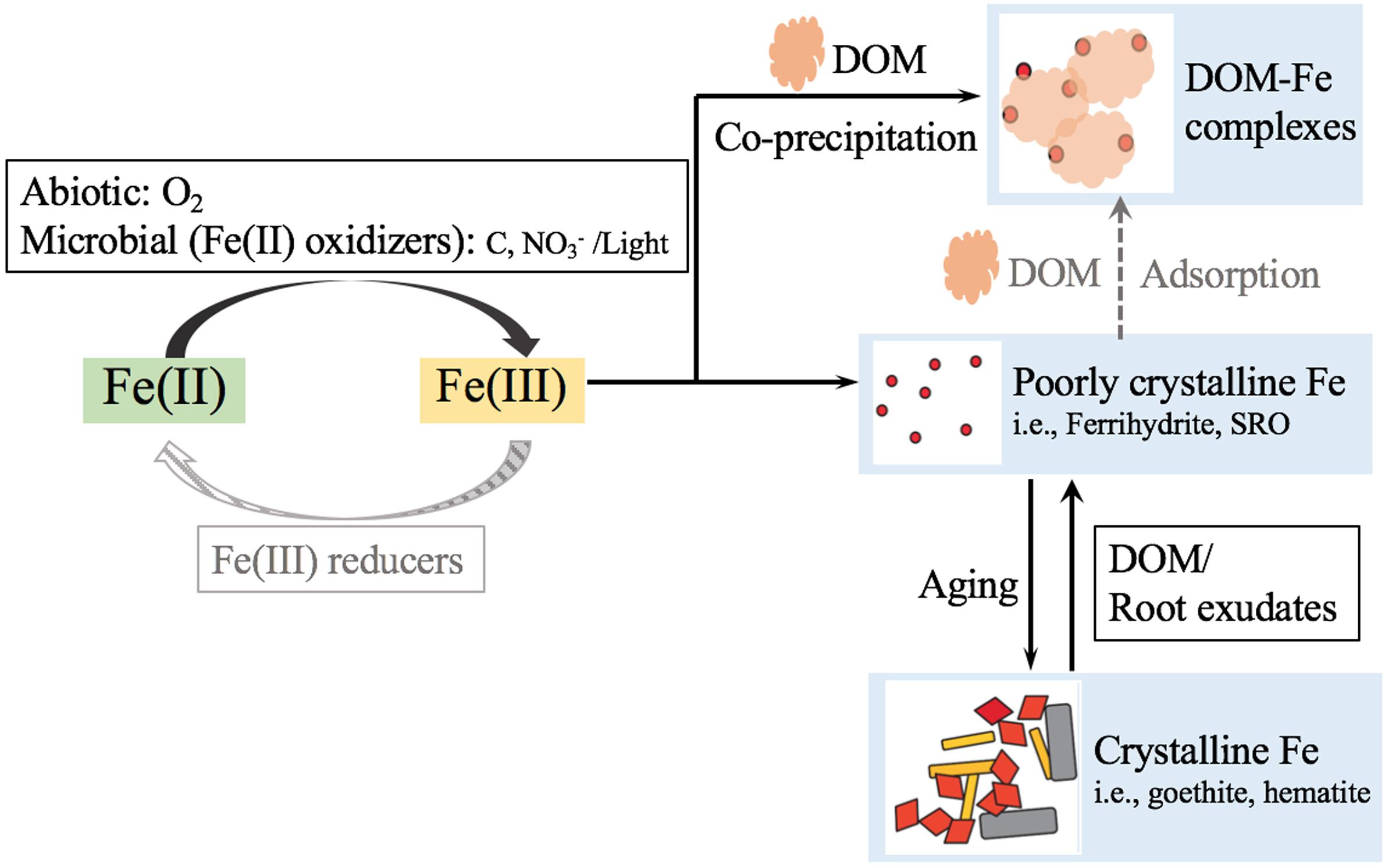
Figure 4. A simplified diagram indicates potential mechanisms on how organic amendments could improve poorly crystalline Fe in soils. The darker dash lines represent a greater extent of the corresponding processes in organic amendments treated soils compared with inorganic fertilization.
Data Availability Statement
The datasets generated for this study are available on request to the corresponding author.
Author Contributions
YW wrote the first draft of the manuscript. JX, BG, and XH added to and corrected the original version. All authors listed have made a substantial, direct and intellectual contribution to this work, and approved it for publication.
Funding
This work was funded by the National Natural Science Foundation of China (41807025, 41807072, and 31700465), the Higher Education Institution Project of Shanxi Province: Ecological Remediation of Soil Pollution Disciplines Group (20181401), and the “1331 Project” Key Innovation Team Construction Plan of Shanxi Province, China (PY201806).
Conflict of Interest
The authors declare that the research was conducted in the absence of any commercial or financial relationships that could be construed as a potential conflict of interest.
Acknowledgments
The authors gratefully acknowledge Jingyuan Ma for help and support at the BL14W1 beamline of the Shanghai Synchrotron Radiation Facility (SSRF), and Jian Wang for his helpful and detailed comments on earlier versions of this manuscript.
Supplementary Material
The Supplementary Material for this article can be found online at: https://www.frontiersin.org/articles/10.3389/feart.2019.00257/full#supplementary-material
References
Adams, W. A., and Kassim, J. K. (1984). Iron oxyhydroxides in soils developed from lower palaeozoic sedimentary rocks in mid-wales and implications for some pedogenetic processes. J. Soil Sci. 35, 117–126. doi: 10.1111/j.1365-2389.1984.tb00266.x
Arias Estévez, M., Conde Cid, M., and Paradelo Núñez, R. (2016). Poorly-crystalline components in aggregates from soils under different land use and parent material. Catena 144, 141–150. doi: 10.1016/j.catena.2016.05.012
Barbera, V., Raimondi, S., Egli, M., and Plötze, M. (2008). The influence of weathering processes on labile and stable organic matter in mediterranean volcanic soils. Geoderma 143, 191–205. doi: 10.1016/j.geoderma.2007.11.002
Bhattacharyya, R., Prakash, V., Kundu, S., Srivastva, A. K., Gupta, H. S., and Mitra, S. (2010). Long term effects of fertilization on carbon and nitrogen sequestration and aggregate associated carbon and nitrogen in the Indian sub-himalayas. Nutr. Cycling Agroecosyst. 86, 1–16. doi: 10.1007/s10705-009-9270-y
Chakraborty, A., and Picardal, F. (2013). Induction of nitrate-dependent Fe (II) oxidation by Fe (II) in dechloromonas sp. strain UWNR4 and acidovorax sp. strain 2AN. Appl. Environ. Microbiol. 79, 748–752. doi: 10.1128/AEM.02709-2712
Cheah, S. F., Kraemer, S. M., Cervini-Silva, J., and Sposito, G. (2003). Steady-state dissolution kinetics of goethite in the presence of desferrioxamine B and oxalate ligands: implications for the microbial acquisition of iron. Chem. Geol. 198, 63–75. doi: 10.1016/S0009-2541(02)00421-427
Chen, C., Dynes, J. J., Wang, J., and Sparks, D. L. (2014). Properties of Fe-organic matter associations via coprecipitation versus adsorption. Environ. Sci. Technol. 48, 13751–13759. doi: 10.1021/es503669u
Chen, C., Kukkadapu, R., and Sparks, D. L. (2015). Influence of coprecipitated organic matter on Fe2+(aq)-catalyzed transformation of ferrihydrite: implications for carbon dynamics. Environ. Sci. Technol. 49, 10927–10936. doi: 10.1021/acs.est.5b02448
Chen, K. Y., Chen, T. Y., Chan, Y. T., Cheng, C. Y., Tzou, Y. M., Liu, Y. T., et al. (2016). Stabilization of natural organic matter by short-range-order iron hydroxides. Environ. Sci. Technol. 50, 12612–12620. doi: 10.1021/acs.est.6b02793
Childs, C. W. (1992). Ferrihydrite: a review of structure, properties and occurrence in relation to soils. J. Plant Nutr. Soil Sci. 155, 441–448. doi: 10.1002/jpln.19921550515
Cismasu, A. C., Michel, F. M., Tcaciuc, A. P., Tyliszczak, T., and Brown, J. G. E. (2011). Composition and structural aspects of naturally occurring ferrihydrite. Comptes Rendus Geosci. 343, 210–218. doi: 10.1016/j.crte.2010.11.001
Coby, A. J., Picardal, F., Shelobolina, E., Xu, H., and Roden, E. E. (2011). Repeated anaerobic microbial redox cycling of iron. Appl. Environ. Microbiol. 77, 6036–6042. doi: 10.1128/AEM.00276-211
Colombo, C., Palumbo, G., He, J. Z., Pinton, R., and Cesco, S. (2013). Review on iron availability in soil: interaction of Fe minerals, plants, and microbes. J. Soils Sediments 14, 538–548. doi: 10.1007/s11368-013-0814-z
Coward, E. K., Thompson, A. T., and Plante, A. F. (2017). Iron-mediated mineralogical control of organic matter accumulation in tropical soils. Geoderma 306, 206–216. doi: 10.1016/j.geoderma.2017.07.026
Croal, L. R., Johnson, C. M., Beard, B. L., and Newman, D. K. (2004). Iron isotope fractionation by Fe(II)-oxidizing photoautotrophic bacteria. Geochim. Cosmochim. Acta 68, 1227–1242. doi: 10.1016/j.gca.2003.09.011
Dahlgren, R. A., and Marrett, D. J. (1991). Organic carbon sorption in arctic and subalpine spodosol B horizons. Soil Sci. Soc. Am. J. 55, 1382–1390. doi: 10.2136/sssaj1991
Ding, L. J., Su, J. Q., Xu, H. J., Jia, Z. J., and Zhu, Y. G. (2015). Long-term nitrogen fertilization of paddy soil shifts iron-reducing microbial community revealed by RNA-(13)C-acetate probing coupled with pyrosequencing. ISME J. 9, 721–734. doi: 10.1038/ismej.2014.159
Dippon, U., Pantke, C., Porsch, K., Larese-Casanova, P., and Kappler, A. (2012). Potential function of added minerals as nucleation sites and effect of humic substances on mineral formation by the nitrate-reducing Fe(II)-oxidizer acidovorax sp. BoFeN1. Environ. Sci. Technol. 46, 6556–6565. doi: 10.1021/es2046266
Duiker, S. W., Rhoton, F. E., Torrent, J., Smeck, N. E., and Lal, R. (2003). Iron (hydr)oxide crystallinity effects on soil aggregation. Soil Sci. Soc. Am. J. 67, 606–611.
Dungait, J. A. J., Hopkins, D. W., Gregory, A. S., and Whitmore, A. P. (2012). Soil organic matter turnover is governed by accessibility not recalcitrance. Glob. Chang. Biol. 18, 1781–1796. doi: 10.1111/j.1365-2486.2012.02665.x
Eusterhues, K., Rennert, T., Knicker, H., Kögel-Knabner, I., Totsche, K. U., and Schwertmann, U. (2011). Fractionation of organic matter due to reaction with ferrihydrite: coprecipitation versus adsorption. Environ. Sci. Technol. 45, 527–533. doi: 10.1021/es1023898
Eusterhues, K., Rumpel, C., and Kogel-Knabner, I. (2005). Organo-mineral associations in sandy acid forest soils: importance of specific surface area, iron oxides and micropores. Eur. J. Soil Sci. 56, 753–763. doi: 10.1111/j.1365-2389.2005.00710.x
Eusterhues, K., Wagner, F. E., Hausler, W., Hanzlik, M., Knicker, H., Totsche, K. U., et al. (2008). Characterization of ferrihydrite-soil organic matter coprecipitates by X-ray diffraction and mossbauer spectroscopy. Environ. Sci. Technol. 42, 7891–7897. doi: 10.1021/es800881w
Fortin, D., and Langley, S. (2005). Formation and occurrence of biogenic iron-rich minerals. Earth Sci. Rev. 72, 1–19. doi: 10.1016/j.earscirev.2005.03.002
Galantini, J., and Rosell, R. (2006). Long-term fertilization effects on soil organic matter quality and dynamics under different production systems in semiarid pampean soils. Soil Till. Res. 87, 72–79. doi: 10.1016/j.still.2005.02.032
Gattinger, A., Muller, A., Haeni, M., Skinner, C., Fliessbach, A., Buchmann, N., et al. (2012). Enhanced top soil carbon stocks under organic farming. Proc. Natl. Acad. Sci. U.S.A. 109, 18226–18231. doi: 10.1073/pnas.1209429109
Ginn, B., Meile, C., Wilmoth, J., Tang, Y. Z., and Thompson, A. (2017). Rapid iron reduction rates are stimulated by high-amplitude redox fluctuations in a tropical forest soil. Environ. Sci. Technol. 51, 3250–3259. doi: 10.1021/acs.est.6b05709
Goodman, B. A., and Cheshire, M. V. (2002). Characterisation by Mössbauer spectroscopy of the forms of iron in sulfide-rich fulvic acid solutions. Hyperfine Interact. 142, 549–558. doi: 10.1023/A:1022415731470
Goodman, B. A., Cheshire, M. V., and Berrow, M. L. (1991a). “Characterization by electron paramagnetic resonance of the organic forms of copper in a soil subjected to prolonged treatment with distillery waste,” in Heavy Metals in the Environment, ed. J. G. Farmer, (Edinburgh: CEConsultants), 55–58.
Goodman, B. A., Cheshire, M. V., and Berrow, M. L. (1991b). “Copper complexes in a soil treated with pig slurry: characterization of soil organic fractions with electron paramagnetic resonance spectroscopy,” in Heavy Metals in the Environment, ed. J. G. Farmer, (Edinburgh: CEP Consultants), 571–574.
Goodman, B. A., Cheshire, M. V., and Chadwick, J. (1991c). Characterization of the Fe(III)-fulvic acid reaction by mössbauer spectroscopy. J. Soil Sci. 42, 25–38. doi: 10.1111/j.1365-2389.1991.tb00088.x
Griffiths, B. S., Ball, B. C., Daniell, T. J., Hallett, P. D., Neilson, R., Wheatley, R. E., et al. (2010). Integrating soil quality changes to arable agricultural systems following organic matter addition, or adoption of a ley-arable rotation. Appl. Soil Ecol. 46, 43–53. doi: 10.1016/j.apsoil.2010.06.012
Hall, S. J., Silver, W. L., Timokhin, V. I., and Hammel, K. E. (2016). Iron addition to soil specifically stabilized lignin. Soil Biol. Biochem. 98, 95–98. doi: 10.1016/j.soilbio.2016.04.010
Halvorson, A. D., Reule, C. A., and Follett, R. F. (1999). Nitrogen fertilization effects on soil carbon and nitrogen in a dryland cropping system contribution from USDA-ARS. Agric. Res. Serv. 63, 912–917. doi: 10.2136/sssaj1999.634912x
Hegler, F., Losekann-Behrens, T., Hanselmann, K., Behrens, S., and Kappler, A. (2012). Influence of seasonal and geochemical changes on the geomicrobiology of an iron carbonate mineral water spring. Appl. Environ. Microbiol. 78, 7185–7196. doi: 10.1128/Aem.01440-1412
Hegler, F., Posth, N. R., Jiang, J., and Kappler, A. (2008). Physiology of phototrophic iron (II)-oxidizing bacteria: implications for modern and ancient environments. FEMS Microbiol. Ecol. 66, 250–260. doi: 10.1111/j.1574-6941.2008.00592.x
Huang, C., Liu, S., Li, R., Sun, F., Zhou, Y., and Yu, G. (2016). Spectroscopic evidence of the improvement of reactive iron mineral content in red soil by long-term application of swine manure. PLoS One 11:e0146364. doi: 10.1371/journal.pone.0146364
Huang, X., Jiang, H., Li, Y., Ma, Y., Tang, H., Ran, W., et al. (2016). The role of poorly crystalline iron oxides in the stability of soil aggregate-associated organic carbon in a rice–wheat cropping system. Geoderma 279, 1–10. doi: 10.1016/j.geoderma.2016.05.011
Huang, X., Feng, C., Zhao, G., Ding, M., Kang, W., Yu, G., et al. (2017). Carbon sequestration potential promoted by oxalate extractable iron oxides through organic fertilization. Soil Sci. Soc. Am. J. 81, 1359–1370. doi: 10.2136/sssaj2017.02.0068
Huang, X., Tang, H., Kang, W., Yu, G., Ran, W., Hong, J., et al. (2018). Redox interface-associated organo-mineral interactions: a mechanism for C sequestration under a rice-wheat cropping system. Soil Biol. Biochem. 120, 12–23. doi: 10.1016/j.soilbio.2018.01.031
Kaiser, K., and Guggenberger, G. (2007). Sorptive stabilization of organic matter by microporous goethite: sorption into small pores vs. surface complexation. Eur. J. Soil Sci. 58, 45–59. doi: 10.1111/j.1365-2389.2006.00799.x
Kaiser, K., Mikutta, R., and Guggenberger, G. (2007). Increased stability of organic matter sorbed to ferrihydrite and goethite on aging. Soil Sci. Soc. Am. J. 71, 711–719. doi: 10.2136/sssaj2006.0189
Kalbitz, K., Schwesig, D., Rethemeyer, J., and Matzner, E. (2005). Stabilization of dissolved organic matter by sorption to the mineral soil. Soil Biol. Biochem. 37, 1319–1331. doi: 10.1016/j.soilbio.2004.11.028
Kappler, A., and Newman, D. K. (2004). Formation of Fe(III)-minerals by Fe(II)-oxidizing photoautotrophic bacteria. Geochim. Cosmochim. Acta 68, 1217–1226. doi: 10.1016/j.gca.2003.09.006
Kappler, A., and Straub, K. L. (2005). Geomicrobiological cycling of iron. Rev. Mineral. Geochem. 59, 85–108. doi: 10.1128/AEM.01151-16
Keiluweit, M., Bougoure, J. J., Nico, P. S., Pett-Ridge, J., Weber, P. K., and Kleber, M. (2015). Mineral protection of soil carbon counteracted by root exudates. Nat. Clim. Chang. 5, 588–595. doi: 10.1038/nclimate2580
Kleber, M., Eusterhues, K., Keiluweit, M., Mikutta, C., Mikutta, R., and Nico, P. S. (2015). Mineral–organic associations: formation, properties, and relevance in soil environments. Adv. Agron. 130, 1–140. doi: 10.1016/bs.agron.2014.10.005
Kleber, M., Mikutta, R., Torn, M. S., and Jahn, R. (2005). Poorly crystalline mineral phases protect organic matter in acid subsoil horizons. Eur. J. Soil Sci. 56, 717–725. doi: 10.1111/j.1365-2389.2005.00706.x
Kögel-Knabner, I., Guggenberger, G., Kleber, M., Kandeler, E., Kalbitz, K., Scheu, S., et al. (2008). Organo-mineral associations in temperate soils: integrating biology, mineralogy, and organic matter chemistry. J. Plant Nutr. Soil Sci. 171, 61–82. doi: 10.1002/jpln.200700048
Kramer, M. G., Sanderman, J., Chadwick, O. A., Chorover, J., and Vitousek, P. M. (2012). Long-term carbon storage through retention of dissolved aromatic acids by reactive particles in soil. Glob. Chang. Biol. 18, 2594–2605. doi: 10.1111/j.1365-2486
Lal, R. (2004). Soil carbon sequestration impacts on global climate change and food security. Science 304, 1623–1627. doi: 10.1126/science.1097396
Lalonde, K., Mucci, A., Ouellet, A., and Gélinas, Y. (2012). Preservation of organic matter in sediments promoted by iron. Nature 483, 198–200. doi: 10.1038/nature10855
Larese-Casanova, P., Haderlein, S. B., and Kappler, A. (2010). Biomineralization of lepidocrocite and goethite by nitrate-reducing Fe(II)-oxidizing bacteria: effect of pH, bicarbonate, phosphate, and humic acids. Geochim. Cosmochim. Acta 74, 3721–3734. doi: 10.1016/j.gca.2010.03.037
Lima, D. L. D., Santos, S. M., Scherer, H. W., Schneider, R. J., Duarte, A. C., Santos, E. B. H., et al. (2009). Effects of organic and inorganic amendments on soil organic matter properties. Geoderma 150, 38–45. doi: 10.1016/j.geoderma.2009.01.009
López-Ulloa, M., Veldkamp, E., and de Koning, G. H. J. (2005). Soil carbon stabilization in converted tropical pastures and forests depends on soil type. Soil Sci. Soc. Am. J. 69, 1110–1117. doi: 10.2136/sssaj2004.0353
Lorenz, K., and Lal, R. (2016). Environmental impact of organic agriculture. Adv. Agron. 139, 99–152. doi: 10.1016/bs.agron.2016.05.003
Lovley, D. R., and Phillips, E. J. P. (1988). Novel mode of microbial energy metabolism: organic carbon oxidation coupled to dissimilatory reduction of iron or manganese. Appl. Environ. Microbiol. 54, 1472–1480.
Lovley, D. R., Ueki, T., Zhang, T., Malvankar, N. S., Shrestha, P. M., Flanagan, K. A., et al. (2011). Geobacter: the microbe electric’s physiology, ecology, and practical applications. Adv. Microb. Physiol. 59, 1–100. doi: 10.1016/B978-0-12-387661-4.00004-5
Ludwig, B., Geisseler, D., Michel, K., Joergensen, R. G., Schulz, E., Merbach, I., et al. (2011). Effects of fertilization and soil management on crop yields and carbon stabilization in soils. Rev. Agron. Sustain. Dev. 31, 361–372. doi: 10.1051/agro/2010030
Maillard, E., and Angers, D. A. (2014). Animal manure application and soil organic carbon stocks: a meta-analysis. Glob. Chang. Biol. 20, 666–679. doi: 10.1111/gcb.12438
Masiello, C. A., Chadwick, O. A., Southon, J., Torn, M. S., and Harden, J. W. (2004). Weathering controls on mechanisms of carbon storage in grassland soils. Glob. Biogeochem. 18, GB4023, 1–9. doi: 10.1029/2004gb002219
McBride, M. B., Goodman, B. A., Russell, J. D., Fraser, A. R., Farmer, V. C., and Dickson, D. P. E. (1983). Characterization of iron in alkaline EDTA and NH4OH extracts of podzols. J. Soil Sci. 34, 825–840. doi: 10.1111/j.1365-2389.1983.tb01075.x
Melton, E. D., Swanner, E. D., Behrens, S., Schmidt, C., and Kappler, A. (2014). The interplay of microbially mediated and abiotic reactions in the biogeochemical Fe cycle. Nat. Rev. Micro. 12, 797–808. doi: 10.1038/nrmicro3347
Mikutta, C. (2011). X-ray absorption spectroscopy study on the effect of hydroxybenzoic acids on the formation and structure of ferrihydrite. Geochim. Cosmochim. Acta 75, 5122–5139. doi: 10.1016/j.gca.2011.06.002
Mikutta, C., Mikutta, R., Bonneville, S., Wagner, F., Voegelin, A., Christl, I., et al. (2008). Synthetic coprecipitates of exopolysaccharides and ferrihydrite. Part I: characterization. Geochim. Cosmochim. Acta 72, 1111–1127. doi: 10.1016/j.gca.2007.11.035
Mikutta, R., Kleber, M., Torn, M. S., and Jahn, R. (2006). Stabilization of soil organic matter: association with minerals or chemical recalcitrance? Biogeochemistry 77, 25–56. doi: 10.1007/s10533-005-0712-716
Mikutta, R., Lorenz, D., Guggenberger, G., Haumaier, L., and Freund, A. (2014). Properties and reactivity of Fe-organic matter associations formed by coprecipitation versus adsorption: clues from arsenate batch adsorption. Geochim. Cosmochim. Acta 144, 258–276. doi: 10.1016/j.gca.2014.08.026
Mimmo, T., Del Buono, D., Terzano, R., Tomasi, N., Vigani, G., Crecchio, C., et al. (2014). Rhizospheric organic compounds in the soil–microorganism–plant system: their role in iron availability. Eur. J. Soil Sci. 65, 629–642. doi: 10.1111/ejss.12158
Miot, J., Benzerara, K., Morin, G., Kappler, A., Bernard, S., Obst, M., et al. (2009a). Iron biomineralization by anaerobic neutrophilic iron-oxidizing bacteria. Geochim. Cosmochim. Acta 73, 696–711. doi: 10.1016/j.gca.2008.10.033
Miot, J., Benzerara, K., Obst, M., Kappler, A., Hegler, F., Schadler, S., et al. (2009b). Extracellular iron biomineralization by photoautotrophic iron-oxidizing bacteria. Appl. Environ. Microbiol. 75, 5586–5591. doi: 10.1128/Aem.00490-499
Murad, E., and Cashion, J. (2004). Mössbauer Spectroscopy of Environmental Materials and Their Industrial Utilization. Dordrecht: Kluwer Academic Oublishers.
Myers, C. R., and Nealson, K. H. (1988). Microbial reduction of manganese oxides: interactions with iron and sulfur. Geochim. Cosmochim. Acta 52, 2727–2732. doi: 10.1016/0016-7037(88)90041-90045
Pohlman, A. A., and McColl, J. G. (1988). Soluble organics from forest litter and their role in metal dissolution. Soil Sci. Soc. Am. J. 52, 265–271.
Porras, R. C., Hicks Pries, C. E., McFarlane, K. J., Hanson, P. J., and Torn, M. S. (2017). Association with pedogenic iron and aluminum: effects on soil organic carbon storage and stability in four temperate forest soils. Biogeochemistry 133, 333–345. doi: 10.1007/s10533-017-0337-336
Posth, N. R., Canfield, D. E., and Kappler, A. (2014). Biogenic Fe(III) minerals: from formation to diagenesis and preservation in the rock record. Earth Sci. Rev. 135, 103–121. doi: 10.1016/j.earscirev.2014.03.012
Posth, N. R., Huelin, S., Konhauser, K. O., and Kappler, A. (2010). Size, density and composition of cell-mineral aggregates formed during anoxygenic phototrophic Fe(II) oxidation: impact on modern and ancient environments. Geochim. Cosmochim. Acta 74, 3476–3493. doi: 10.1016/j.gca.2010.02.036
Raiswell, R. (2011). Iron transport from the continents to the open ocean: the aging-rejuvenation cycle. Elements 7, 101–106. doi: 10.2113/gselements.7.2.101
Rasmussen, C., Southard, R. J., and Horwath, W. R. (2006). Mineral control of organic carbon mineralization in a range of temperate conifer forest soils. Glob. Chang. Biol. 12, 834–847. doi: 10.1111/j.1365-2486.2006.01132.x
Rasmussen, P. E., Goulding, K. W. T., Brown, J. R., Grace, P. R., Janzen, H. H., and Körschens, M. (1998). Long-term agroecosystem experiments: assessing agricultural sustainability and global change. Science 282, 893–896. doi: 10.1126/science.282.5390.893
Riedel, T., Zak, D., Biester, H., and Dittmar, T. (2013). Iron traps terrestrially derived dissolved organic matter at redox interfaces. Proc. Nat. Acad. Sci. U.S.A. 110, 10101–10105. doi: 10.1073/pnas.1221487110
Roden, E. E. (2012). Microbial iron-redox cycling in subsurface environments. Biochem. Soc. Trans. 40, 1249–1256. doi: 10.1042/BST20120202
Rumpel, C., Baumann, K., Remusat, L., Dignac, M. F., Barré, P., Deldicque, D., et al. (2015). Nanoscale evidence of contrasted processes for root-derived organic matter stabilization by mineral interactions depending on soil depth. Soil Biol. Biochem. 85, 82–88. doi: 10.1016/j.soilbio.2015.02.017
Schmidt, M. W. I., Torn, M. S., Abiven, S., Dittmar, T., Guggenberger, G., Janssens, I. A., et al. (2011). Persistence of soil organic matter as an ecosystem property. Nature 478, 49–56. doi: 10.1038/nature10386
Schwertmann, U. (1966). Inhibitory effect of soil organic matter on the crystallization of amorphous ferric hydroxide. Nature 212, 645–646. doi: 10.1038/212645b0
Schwertmann, U. (1970). Influence of various simple organic anions on formation of goethite and hematite from amorphous ferric hydroxide. Geoderma 3, 207–214. doi: 10.1016/0016-7061(70)90020-90020
Schwertmann, U., Wagner, F., and Knicker, H. (2005). Ferrihydrite-humic associations: magnetic hyperfine interactions. Soil Sci. Soc. Am. J. 69, 1009–1015.
Straub, K. L., Benz, M., Schink, B., and Widdel, F. (1996). Anaerobic, nitrate-dependent microbial oxidation of ferrous iron. Appl. Environ. Microbiol. 62, 1458–1460.
Tong, X., Xu, M., Wang, X., Bhattacharyya, R., Zhang, W., and Cong, R. (2014). Long-term fertilization effects on organic carbon fractions in a red soil of China. Catena 113, 251–259. doi: 10.1016/j.catena.2013.08.005
Torn, M. S., Trumbore, S. E., Chadwick, O. A., Vitousek, P. M., and Hendricks, D. M. (1997). Mineral control of soil organic carbon storage and turnover. Nature 389, 170–173. doi: 10.1038/38260
Van De Vreken, P., Gobin, A., Baken, S., Van Holm, L., Verhasselt, A., Smolders, E., et al. (2016). Crop residue management and oxalate-extractable iron and aluminium explain long-term soil organic carbon sequestration and dynamics. Eur. J. Soil Sci. 67, 332–340. doi: 10.1111/ejss.12343
Wagai, R., and Mayer, L. M. (2007). Sorptive stabilization of organic matter in soils by hydrous iron oxides. Geochim. Cosmochim. Acta 71, 25–35. doi: 10.1016/j.gca.2006.08.047
Wang, P., Wang, J., Zhang, H., Dong, Y., and Zhang, Y. (2019). The role of iron oxides in the preservation of soil organic matter under long-term fertilization. J. Soils Sed. 19, 588–598. doi: 10.1007/s11368-018-2085-1
Weber, K. A., Achenbach, L. A., and Coates, J. D. (2006a). Microorganisms pumping iron: anaerobic microbial iron oxidation and reduction. Nat. Rev. Microbiol. 4, 752–764. doi: 10.1038/nrmicro1490
Weber, K. A., Urrutia, M. M., Churchill, P. F., Kukkadapu, R. K., and Roden, E. E. (2006b). Anaerobic redox cycling of iron by freshwater sediment microorganisms. Environ. Microbiol. 8, 100–113. doi: 10.1111/j.1462-2920.2005.00873.x
Wei, Z. Q., Ji, J. H., Li, Z., and Yan, X. (2017). Changes in organic carbon content and its physical and chemical distribution in paddy soils cultivated under different fertilisation practices. J. Soils Sed. 17, 2011–2018. doi: 10.1007/s11368-017-1676-6
Wen, Y., Li, H., Xiao, J., Wang, C., Shen, Q., Ran, W., et al. (2014). Insights into complexation of dissolved organic matter and Al(III) and nanominerals formation in soils under contrasting fertilizations using two-dimensional correlation spectroscopy and high resolution-transmission electron microscopy techniques. Chemosphere 111, 441–449. doi: 10.1016/j.chemosphere.2014.03.078
Wen, Y. L., Xiao, J., Li, H., Shen, Q. R., Ran, W., Zhou, Q. S., et al. (2014). Long-term fertilization practices alter aluminum fractions and coordinate state in soil colloids. Soil Sci. Soc. Am. J. 78, 2083–2089. doi: 10.2136/sssaj2014.04.0147
Wen, Y., Xiao, J., Liu, F., Goodman, B. A., Li, W., Jia, Z., et al. (2018). Contrasting effects of inorganic and organic fertilisation regimes on shifts in Fe redox bacterial communities in red soils. Soil Biol. Biochem. 117, 56–67. doi: 10.1016/j.soilbio.2017.11.003
Wen, Y. L., Liu, W. J., Deng, W. B., He, X. H., and Yu, G. H. (2019). Impact of agricultural fertilization practices on organo-mineral associations in four long-term field experiments: implications for soil C sequestration. Sci. Total Environ. 651, 591–600. doi: 10.1016/j.scitotenv.2018.09.233
Whalen, J. K., and Chang, C. (2002). Macroaggregate characteristics in cultivated soils after 25 annual manure applications. Soil Sci. Soc. Am. J. 66, 1637–1647.
Xiao, J., Wen, Y., Li, H., Hao, J., Shen, Q., Ran, W., et al. (2015). In situ visualisation and characterisation of the capacity of highly reactive minerals to preserve soil organic matter (SOM) in colloids at submicron scale. Chemosphere 138, 225–232. doi: 10.1016/j.chemosphere.2015.05.089
Xu, R. K., Hu, Y. F., Dynes, J. J., Zhao, A. Z., Blyth, R. I. R., Kozak, L. M., et al. (2010). Coordination nature of aluminum (oxy)hydroxides formed under the influence of low molecular weight organic acids and a soil humic acid studied by X-ray absorption spectroscopy. Geochim. Cosmochim. Acta 74, 6422–6435. doi: 10.1016/j.gca.2010.07.029
Yan, X., Zhou, H., Zhu, Q. H., Wang, X. F., Zhang, Y. Z., Yu, X. C., et al. (2013). Carbon sequestration efficiency in paddy soil and upland soil under long-term fertilization in southern China. Soil Till. Res. 130, 42–51. doi: 10.1016/j.still.2013.01.013
Yu, G., Xiao, J., Hu, S., Polizzotto, M. L., Zhao, F., McGrath, S. P., et al. (2017). Mineral availability as a key regulator of soil carbon storage. Environ. Sci. Technol. 51, 4960–4969. doi: 10.1021/acs.est.7b00305
Yu, G. H., Wu, M. J., Wei, G. R., Luo, Y. H., Ran, W., Wang, B. R., et al. (2012). Binding of organic ligands with Al(III) in dissolved organic matter from soil: implications for soil organic carbon storage. Environ. Sci. Technol. 46, 6102–6109. doi: 10.1021/es3002212
Zhang, J. C., Zhang, L., Wang, P., Huang, Q. W., Yu, G. H., Li, D. C., et al. (2013). The role of non-crystalline Fe in the increase of SOC after long-term organic manure application to the red soil of southern China. Eur. J. Soil Sci. 64, 797–804. doi: 10.1111/ejss.12104
Keywords: organic amendments, poorly crystalline Fe(III) minerals, Fe (oxyhydr)oxides transformation, microbially mediated Fe cycling, SOC preservation
Citation: Wen Y, Xiao J, Goodman BA and He X (2019) Effects of Organic Amendments on the Transformation of Fe (Oxyhydr)Oxides and Soil Organic Carbon Storage. Front. Earth Sci. 7:257. doi: 10.3389/feart.2019.00257
Received: 24 June 2019; Accepted: 19 September 2019;
Published: 11 October 2019.
Edited by:
Guanghui Yu, Tianjin University, ChinaReviewed by:
Tida Ge, Institute of Subtropical Agriculture (CAS), ChinaJitao Lv, Research Center for Eco-Environmental Sciences (CAS), China
Bingzi Zhao, Institute of Soil Science (CAS), China
Copyright © 2019 Wen, Xiao, Goodman and He. This is an open-access article distributed under the terms of the Creative Commons Attribution License (CC BY). The use, distribution or reproduction in other forums is permitted, provided the original author(s) and the copyright owner(s) are credited and that the original publication in this journal is cited, in accordance with accepted academic practice. No use, distribution or reproduction is permitted which does not comply with these terms.
*Correspondence: Yongli Wen, eWx3ZW5Ac3h1LmVkdS5jbg==; eWx3ZW4xOTg4QHNpbmEuY29t