Upper Paleozoic to Lower Mesozoic Tetrapod Ichnology Revisited: Photogrammetry and Relative Depth Pattern Inferences on Functional Prevalence of Autopodia
- 1Staatliches Museum für Naturkunde Stuttgart, Stuttgart, Germany
- 2Institut Català de Paleontologia Miquel Crusafont, Cerdanyola del Vallès, Spain
- 3Urweltmuseum GEOSKOP/Burg Lichtenberg (Pfalz), Tallichtenberg, Germany
In recent years photogrammetry has become an essential tool in the study of tetrapod footprints. Morphological analyses of footprints are interpretative; thus, researchers should use as much information as possible in order to eventually provide an objective conclusion. In this regard, photogrammetry is an extremely helpful tool to avoid potential biases and to better present ichnological data. We review the use of this technique in several Permian and Triassic tetrapod ichnological studies, with considerations on (1) ichnotaxonomy, (2) track-trackmaker correlation, (3) locomotion and/or behavior, (4) substrate induced effects, and (5) preservation of the fossil record and heritage. Furthermore, based on the available three-dimensional (3D) data on Permian and Triassic material, we present a first qualitative interpretation of relative depth patterns and the related functional prevalence (most deeply impressed area) within footprints. We identified three main groups: (1) anamniote, captorhinomorph/parareptile tracks (medial-median functional prevalence), (2) diapsid tracks (median functional prevalence), and (3) synapsid tracks (median-lateral functional prevalence). The use of 3D photogrammetric models brings new light to the tetrapod footprint record, helping to better understand tetrapod communities throughout the late Paleozoic (and the end-Guadalupian and end-Permian extinctions) and the tetrapod recovery during the early Mesozoic.
Introduction
Tetrapod footprints are abundant vertebrate remains in upper Paleozoic to lower Mesozoic terrestrial successions (Klein and Lucas, 2010; Lucas, 2019). Their study facilitates the reconstruction of past ecosystems, especially where skeletal remains are absent, scattered, or poorly preserved. Tetrapod tracks of late Carboniferous to Middle Triassic age have been intensively studied since the 19th century, and especially during the 20th century, with the publication of an extensive literature that includes research articles, books, and monographies (among many other publications: Kaup, 1835; Geinitz, 1861, 1863; Curioni, 1870; Geinitz and Deichmüller, 1882; Pabst, 1895, 1908; Marsh, 1894; Maidwell, 1911; Nopcsa, 1923; Gilmore, 1927; Moodie, 1929, 1930; Abel, 1935; Peabody, 1948; Heyler and Lessertisseur, 1963; Haubold, 1970, 1971a,b; Gand, 1987). These works often include morphological analyses, usually comprising (1) a graphic interpretation (mostly represented by line and shadow drawings) of footprints and (2) a quantification of the track and trackway proportions by measuring different features (for measurement standards: Haubold, 1971b; Leonardi, 1987). Such analyses form the basis of ichnotaxonomy that follows the rules of the International Code of Zoological Nomenclature (ICZN; International Commission on Zoological Nomenclature, 1999). Based on these morphological analyses, hundreds of ichnotaxa (grouped in ichnogenera and ichnospecies, and sometimes in ichnofamilies as well) have been erected. Nevertheless, in several studies the conditions of the original substrate (e.g., composition, granulometry, original moisture, rheology) where footprints were impressed and the behavior of the trackmakers have not been fully considered, though such factors are the main constraints (together with the autopodia anatomy) of the final track morphology (Falkingham, 2014; Gatesy and Falkingham, 2017; Belvedere et al., 2018; Marchetti et al., 2019a). Therefore, several ichnotaxa have been erected on the basis of extramorphological features, which represent variations not due to the foot anatomy (Peabody, 1948; Haubold, 1996). This has resulted in an oversplitting of ichnotaxa and also, in particular cases, in an oversimplification (McKeever and Haubold, 1996; Marchetti et al., 2019c, d). This is why footprints useful for ichnotaxonomy need to be selected by means of morphological preservation, which is the preservation of features derived from the foot anatomy (Marchetti et al., 2019a).
At the end of the 20th century, new techniques to create 3D digital models started to be applied in tetrapod ichnology, revolutionizing this field. These techniques allowed a better understanding of the processes of footprint formation, and thus of producers’ locomotion, by creating virtual 3D models of this mechanism (e.g., Gatesy et al., 1999; Falkingham and Gatesy, 2014). 3D digital modeling rapidly expanded in dinosaur ichnology by digitizing actual fossil footprints in order to provide more objective interpretations and measurements (e.g., Leonardi and Mietto, 2000; Bates et al., 2008a, b, 2010; Petti et al., 2008). However, 3D modeling has not been widely used in the study of Permian and Triassic tetrapod ichnofossils, with few exceptions including 3D models, mostly used as complementary representations of footprints (e.g., de Klerk, 2002; Petti et al., 2009). In the beginning, the use of 3D techniques, often based on laser scanning, was very limited, especially due to the cost and limited portability of the scanners; however, this completely changed with the rapid advances of photogrammetric techniques (Matthews, 2008; Remondino et al., 2010; Falkingham, 2012; Mallison and Wings, 2014; Falkingham et al., 2018). In fact, because of its low cost and efficiency, photogrammetry rapidly spread out in ichnological studies, especially in dinosaur ichnology. The introduction of 3D models brought more objectivity to the analyses of a given morphology. Several studies focused in the best procedure to obtain the raw data for 3D photogrammetric models (i.e., digital photos), as well as in the methodologies to create models and/or how they should be studied and presented in scientific articles (e.g., Matthews, 2008; Petti et al., 2008; Falkingham, 2012; Mallison and Wings, 2014; Belvedere et al., 2018; Falkingham et al., 2018). Historical photogrammetry, in which 3D digital models are created from scans of analog photographs taken before photogrammetry even existed (e.g., Falkingham et al., 2014; Lallensack et al., 2015), is now also being used. Only recently, several works dealing with tetrapod footprints from the Permian and Triassic (and, to a lesser degree, the upper Carboniferous) used photogrammetry to better interpret the morphology of the tetrapod footprints and locomotion (e.g., Mujal et al., 2015, 2016b,2017a; Marchetti et al., 2017a,2019c,d; Citton et al., 2018; Lagnaoui et al., 2019; Mujal and Marchetti, 2020; Mujal and Schoch, 2020; and references therein).
The study between non-avian dinosaur ichnology and Permian–Triassic tetrapod ichnology is slightly different, though they both follow the same conventions and use the same methodologies to correctly interpret the footprint morphology. In fact, non-dinosaur tetrapod footprints are generally smaller in comparison with most dinosaur footprints and show a higher degree of complexity, whereas dinosaur tracks (with some exceptions related to small-sized dinosaur ichnotaxa) show more complex depth patterns and thus difficulties to trace footprint outlines (e.g., Lallensack, 2019). In this work, we review different ichnofossil records in which photogrammetry was used, ranging from the lower Permian (Cisuralian) to the Middle Triassic. We review previously published works on tetrapod ichnology including 3D models. In addition, in order to provide a wider overview and for further comparisons, we also create new 3D models of some tracks and ichnotaxa, from which photogrammetric studies have not been carried out. We synthesize examples with the aim to demonstrate how photogrammetry helped in the ichnological analyses, not only for newly uncovered fossils but also in ichnotaxonomic revisions. We identify up to five fields in which photogrammetry is of great use: (1) ichnotaxonomy, (2) track-trackmaker correlations, (3) locomotor and/or behavioral considerations, (4) substrate induced effects, (5) preservation of the fossil record and heritage. Moreover, for the first time, we discuss the footprint relative depth patterns of the most important upper Carboniferous, Permian, and Triassic tetrapod groups using 3D digital models and compare them to the phylogenies of these groups.
Materials and Methods
Elaboration of 3D Photogrammetric Models
Photogrammetry plays a prominent role in tetrapod ichnological analyses and is essential in some cases (e.g., in the identification of the true shape of footprints when other techniques fail). Furthermore, a single 3D model can be used for different purposes. Photogrammetry provides important information in ichnotaxonomy, in track-trackmaker correlations, in elucidating the locomotion of the producers, in the reconstruction of taphonomic processes and of paleoenvironmental settings, as well as in the digital preservation of this heritage.
Herein, we provide a brief resume of how we created 3D photogrammetric models. Noteworthy, the majority of the examples reviewed in this work are uncollected specimens. In this case, the photographs for the 3D models were obtained during fieldwork, so the data collection was usually constrained by the natural light conditions. The following work flow is modified from Mujal (2017).
Overall, photogrammetry relies on obtaining digital 3D models of an object. The photogrammetric models are built from photos of the specimens (Figure 1). For detailed explanations on the procedures of photo acquisition, we refer to Matthews (2008), Falkingham (2012), and Mallison and Wings (2014). In the examples presented here, these procedures were set according to the (field) conditions of each specimen. First of all, the track-bearing surface must be cleaned. Then, centimetric and/or millimetric scales, as well as white paper squares with specific black geometric forms (being easily recognizable by the modeling software), are placed around the footprint or trackway to be modeled (Figure 1A). Afterward light conditions are evaluated, as specimens must not have too much shadow nor too many bright zones during the photo acquisition. If necessary, a (weak) shadow is projected over the entire specimen to be modeled in order to obtain homogeneous light conditions. Once light conditions are suitable, photographs can be taken in these ways: (1) photos of the specimen from all its perspectives and angles, i.e., moving along a cupola- or umbrella-like form around the specimen while photographing (Figure 1B), and/or (2) photos orthogonal to the surface, covering the entire area to be modeled and taking into account that two consecutive photos must overlap at least one third (Figure 1C). On average, 30 photos per footprint/pes-manus couple were taken, but this number varies according to the size and complexity of each specimen (e.g., some models are built up with 50, 60, and 70 photos, or in specific cases, such as whole trackways, with more than 200 photos). The use of camera tripods is recommended, although in most cases (for specimens in the field) they cannot be used due to the location of the samples. Different digital cameras were used, all being characterized by having at least 8.1 Megapixel of resolution. The cameras we used are Sony DSC-T200, device standard lens 35–175 mm F3.5–F4.4; Sony DSC-H50, device standard lens 31–465 mm F2.7–F4.5; Panasonic DMC-FZ18, device standard lens 28–504 mm F2.8–F4.2; Canon PowerShot SX410 IS, device standard lens 4.3–172 mm F.3.5–F6.3; Canon EOS 70D, lenses 18–135 mm and 10–18 mm; focal lengths are usually of 4, 5, or 6 mm. Once the photos were obtained, they were processed by a workstation, with recommended minimum requirements: i7 processor (or equivalent-updated) with minimum 32GB RAM and graphic card GeForce RTX 2060 equivalent or updated. Photos were taken (in JPG format) without any size reduction in different software, most of which are freely available:
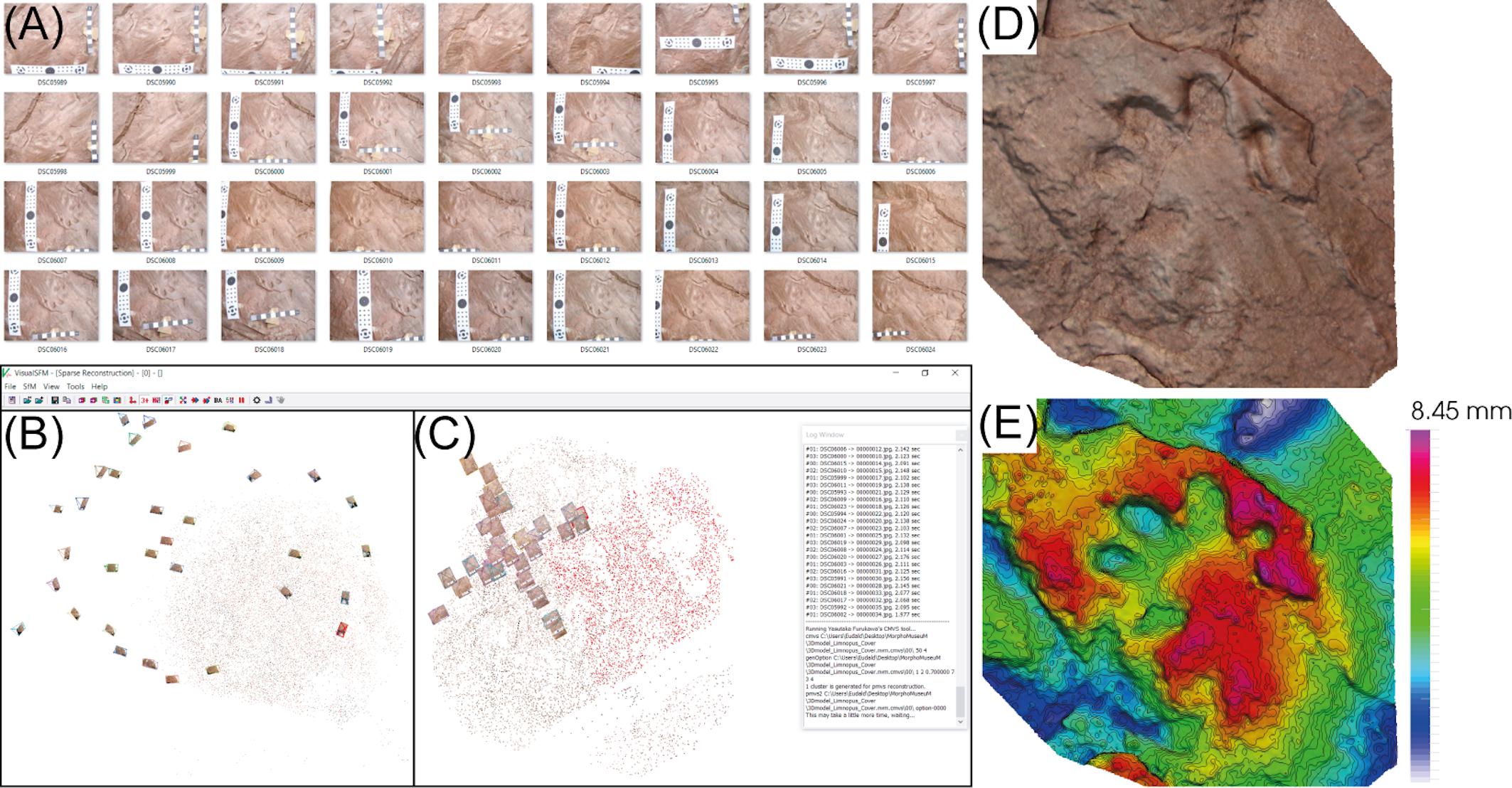
Figure 1. Photogrammetry workflow (from Mujal, 2017). (A) Set of photos to generate the 3D model with different centimetric scales. (B,C) Relative position of the photos as seen in Visual SfM software; photos were obtained changing the perspective in each one (B) and moving the camera laterally (C). (D) 3D model with the original texture of the specimen as prepared with MeshLab. (E) Depth color map and contours of the 3D model as prepared with ParaView. (A,C–E) Correspond to the same modeling process. The example used is a right manus-pes couple of Limnopus isp. from the Pyrenean Basin (corresponding mold and replica are IPS82608).
(1.1) Visual SfM (open access; v.0.5.22 to v.0.5.251): Generation of the dense point cloud on which the 3D model is based (Figures 1B,C).
(1.2) Agisoft Photoscan (standard version 1.1.42): Generation of the dense point cloud and the triangular mesh, and application of the original texture (i.e., color) of the specimen.
(2) MeshLab (open access; v.1.3.2 and v.2016.123): (1) generation of the triangular mesh based on the dense point cloud; (2) application of the original texture (i.e., color) of the specimen; (3) cleaning (removing) of the generated odd points; (4) aligning, scaling, and orientation of the 3D model (Figure 1D). Note that the two first steps are unnecessary if Agisoft Photoscan is used.
(3) ParaView (open access; v.3.98.1 to v.4.1.04): Generation of the depth map by using a color scale and application of the contours (Figure 1E). In order to provide a homogeneous view of the whole track sample, in this work we have used the “Rainbow Desaturated” filter for the elevation map. Otherwise, the number of contours is set according to the complexity of each specimen; the number of lines in each model generally varies between 30 and 60.
Depth Pattern Analysis
We conducted a qualitative analysis aiming to identify and classify depth patterns among tetrapod ichnotaxa from the upper Carboniferous to the Middle Triassic (see section “Relative Depth Patterns on Permian to Triassic Tetrapod Footprints” below). The database includes new data and a review of the published 3D data (de Klerk, 2002; Mujal et al., 2015, 2016a, 2016b, 2017a, 2017b, 2018b; Citton et al., 2016, 2018, 2019a, 2019b,2020; Meade et al., 2016; Milàn et al., 2016; Marchetti et al., 2017a,b, 2018a,b, 2019b,c,d; Francischini et al., 2018, 2020; Lagnaoui et al., 2019; Marchetti, 2019; Cavin and Piuz, 2020; Cisneros et al., 2020; Farman and Bell, 2020; Mujal and Marchetti, 2020; Mujal and Schoch, 2020; Reolid et al., 2020). Note that the number of tracks and localities from the upper Carboniferous is notably lower than those from the lower Permian. Nevertheless, most of the upper Carboniferous ichnotaxa are also present in the lower Permian (Lucas, 2019, and references therein).
In order to avoid misinterpretations, here we define the functional prevalence of footprints as the part of the footprint that is more deeply impressed than the other parts of the same footprint. This character must be consistent along a trackway and/or between tracks assigned to the same ichnotaxon, and under different substrate (as well as different paleoenvironments) and locomotory conditions.
The relative depth patterns of footprints are independent from all the ichnotaphonomic effects as defined by Marchetti et al. (2019a). Therefore, it should be related to the functional anatomy of the trackmakers’ postcranial skeleton (or, more specifically, autopodia). Medial, median, and lateral refer to the inner, middle, and outer parts, respectively, of a footprint as defined by Leonardi (1987). To characterize the track-bearing substrate (e.g., mudstone, sandstone, limestone), we evaluated composition, granulometry, and sedimentary structures. Such information, when available (e.g., some tracks are currently only documented by artificial casts), was integrated with data from the geological successions that yielded the studied specimens. For the sake of clarity, and as further explained in section “Relative Depth Patterns on Permian to Triassic Tetrapod Footprints” below, the substrate features, if not relevant for the analysis, are not specified nor discussed.
Tetrapod Ichnoassociations From the Lower Permian to the Middle Triassic
Here, we list the localities yielding the tetrapod ichnofossil record (for which 3D photogrammetric models were created) reviewed in section “Results and Discussion” below; further details on such localities are found in the Supplementary Data S1. The label of each analyzed specimen is mentioned through the present work and/or in the corresponding figure caption (see also institutional abbreviations below). For further specimen numbers we also refer the reader to the publications herein cited.
- Pyrenean Basin (northeastern Iberian Peninsula):
∘ Artinskian (Cisuralian) ichnoassociations from the Lower Red Units (time-equivalent to the Peranera Formation) (see Gisbert, 1981; Voigt and Haubold, 2015; Mujal et al., 2016a, 2018a) analyzed by Mujal et al. (2016b), who included 3D models.
∘ Guadalupian–Lopingian ichnoassociation from the Upper Red Unit analyzed by Mujal et al. (2017b), who included 3D models.
∘ Lower–Middle Triassic ichnoassociations from the Buntsandstein facies unit analyzed by Mujal et al. (2016a, 2017a), who included 3D models.
- Catalan Basin (northeastern Iberian Peninsula): Middle Triassic ichnoassociations from the Buntsandstein facies (early–middle Anisian; Fortuny et al., 2011, who did not include 3D models) and middle Muschelkalk facies (late Anisian–middle Ladinian; Mujal et al., 2015, 2018b, who included 3D models).
- Lodève Basin (southern France; for units datations see Michel et al., 2015):
∘ Asselian (Cisuralian) trackways of Ichniotherium cottae from the Usclas Saint Privat or the Tuillières-Loiras formations (previously studied by Heyler and Lessertisseur, 1963; recently reanalyzed by Mujal and Marchetti, 2020, who included 3D models).
∘ Artinskian (Cisuralian) ichnoassociations from the Rabejac Formation (e.g., Gand, 1987; Heyler and Gand, 2000; Gand and Durand, 2006, who did not include 3D models).
∘ Guadalupian tracks of Merifontichnus from the La Lieude Formation (e.g., Gand et al., 2000, who did not include 3D models).
∘ Middle Triassic archosauromorph tracks from red-bed deposits (e.g., Demathieu, 1985; Demathieu and Demathieu, 2004; Gand et al., 2007, and references therein, who did not include 3D models).
- Orobic Basin (northern Italy): Kungurian (Cisuralian) trackway of Amphisauropus kablikae from the Pizzo del Diavolo Formation of the Orobic Basin (see Marchetti et al., 2015; Marchetti, 2016) analyzed by Marchetti et al. (2017a), who included 3D models.
- Hessian Basin (Germany): Lopingian ichnoassociations from eolian units of the Cornberg Formation (see Schmidt, 1959) analyzed by Marchetti et al. (2019c), who included 3D models.
- Central European Basin (Germany): Ladinian (Middle Triassic) tracks from the base of the Antrhakonitbank at the Vellberg Fossil-Lagerstätte, Lower Keuper (Erfurt Formation; see Schoch and Seegis, 2016) analyzed by Mujal and Schoch (2020), who included 3D models.
- Lochmaben and Dumfries basins (Scotland, United Kingdom): Lopingian ichnoassociations from eolian units of the Cornockle and Locharbriggs formations (see Anonymous, 1828; Jardine, 1853; McKeever and Haubold, 1996) analyzed by Marchetti et al. (2019c), who included 3D models.
- Arizona (United States): Kungurian (Cisuralian) ichnoassociations from the eolian units of the De Chelly and Coconino formations (see Gilmore, 1927; Haubold et al., 1995a) analyzed by Marchetti et al. (2019b), who included 3D models.
- Karoo Basin (South Africa): Guadalupian to Lower Triassic ichnoassociations from continental fluvial units of the Abrahamskraal, Teekloof, and Balfour formations (see Smith and Botha-Brink, 2014; Klein et al., 2015) analyzed by Marchetti et al. (2019d), who included 3D models.
Institutional Abbreviations
AM, Albany Museum, Grahamstown (Makhanda, as of 4 October 2018), South Africa.
DUMFM, Dumfries Museum and Camera Obscura, Dumfries, Scotland, United Kingdom.
EMVG, ‘Casa del Tempo’, Ecomuseo della Val Gerola, Sondrio, Lombardy, Italy.
IPS, Institut Català de Paleontologia Miquel Crusafont, Sabadell, Catalonia, Spain.
MF-LOD, Lodève collection at Musée Fleury, Lodève, France.
MNA, Museum of Northern Arizona, Flagstaff, AZ, United States.
MNHN.F.LOD, Lodève collection at Muséum National d’Histoire Naturelle, Paris, France.
NHMUK PV, British Museum of Natural History, London, United Kingdom.
NMK, Museum of Natural History in the Ottoneum, Kassel, Germany.
RAM, Raymond M. Alf Museum of Paleontology, Claremont, CA, United States.
RMS, National Museum of Scotland, Edinburgh, Scotland, United Kingdom.
SMNS, Staatliches Museum für Naturkunde Stuttgart, Stuttgart, Germany.
UCMP, University of California, Berkeley, CA, United States.
UG, Faculty of Geoscience and Geography, Göttingen University, Göttingen, Germany.
UGKU, Urweltmuseum GEOSKOP/Burg Lichtenberg (Pfalz), Tallichtenberg, Germany.
UM-LOD, Lodève collection at Université de Montpellier, Montpellier, France.
USNM, Smithsonian – National Museum of Natural History, Washington, DC, United States.
YPM, Yale Peabody Museum of Natural History, New Haven, CT, United States.
Results and Discussion
Herein, we present a review of research on tetrapod tracks involving 3D photogrammetric models. Taken together, the analyzed examples cover both a long age interval (Cisuralian to Middle Triassic) and a wide range of substrates and paleoenvironments (eolian, fluvial, lacustrine, and coastal settings). Subsequently, we provide a first analysis based on the relative depth pattern of tetrapod footprints, suggesting a link with the functional prevalence of autopodia.
Photogrammetry: A Toolkit for Ichnologists
Following we identify different ichnological fields in which photogrammetry is useful, independently from the analyzed ichnotaxon, age, substrate, and paleoenvironment.
Ichnotaxonomy
Tetrapod ichnotaxonomy is primarily based on the morphology of footprints (distinguishing manual and pedal impressions for quadruped trackmakers) and, to a lesser extent, on the trackway pattern. In ideal conditions, the footprint morphology and the trackway pattern are considered to reflect anatomical traits of the trackmaker (e.g., Olsen, 1995; Carrano and Wilson, 2001; Belvedere et al., 2018; Marchetti et al., 2019a). In fact, each ichnotaxon could be potentially correlated with a specific trackmaker group, often at family or higher level groups (Falcon-Lang et al., 2010).
Anatomy-consistent morphology is the very base of ichnotaxonomy (e.g., Baird, 1957; Haubold et al., 1995b; Haubold, 1996; Marchetti et al., 2019a). Autopodial anatomy is recorded during the trackmaker’s locomotion, i.e., footprints record a dynamic behavior. Therefore, before doing ichnotaxonomical inferences, the process of autopodia impression in the sediment should be understood (e.g., Gatesy et al., 1999; Falkingham and Gatesy, 2014; Gatesy and Falkingham, 2017; Mujal and Schoch, 2020). In addition, the effects of the substrate conditions at the time of impression should be considered (see also section “Relative Depth Patterns on Permian to Triassic Tetrapod Footprints” below), because they can alter significantly the morphological traits linked to anatomy (Falkingham, 2014). Moreover, a number of other effects occurring after the track recording, such as trace fossil and sedimentary structure superimpositions and the sediment erosion, should be correctly interpreted (e.g., Marty et al., 2009). Nonetheless, as shown in section “Relative Depth Patterns on Permian to Triassic Tetrapod Footprints” below, we observe that 3D models help to identify the anatomy-related ichnotaxa morphology through the analysis of shape and relative depth patterns of imprints, even if the track morphologies are apparently different due to different substrates. Therefore, 3D models help in comparing track morphologies useful for ichnotaxonomy, thus avoiding oversplitting or overlumping of ichnotaxa that would have no faunistic and hence no evolutionary, biomechanic, biostratigraphic, and paleobiogeographic meaning (e.g., Marchetti et al., 2019a, d; Mujal and Marchetti, 2020).
3D models help to identify important anatomy-consistent features of both new and already known ichnotaxa that may remain unseen to the naked eye. Indeed, this is the case of several ichnotaxa (including different morphologies and sizes) from previously published specimens. Herein we review and summarize examples from some of our studies (see section “Tetrapod Ichnoassociations from the Lower Permian to the Middle Triassic” above and Supplementary Data S1) showing the use of 3D photogrammetric models in recognizing relevant ichnotaxonomic features:
- The complete shape of pedal impressions in MNHN.F.LOD83 from the Cisuralian of southern France re-analyzed by Mujal and Marchetti (2020) was identified through the study of the 3D model that these authors carried out (Figure 2A). Impressions of pedal digits IV and V (roman numbers and red arrows in Figure 2A) are unclear when observed in the original specimen, but a consistent depth pattern was observed in the false color depth maps. The 3D model also facilitated the identification of two trackways, which were also unclear from the previous analysis by Heyler and Lessertisseur (1963). The actual length of several digit impressions, as well as the shape of sole and palm impressions, is masked by the presence of digit scratches and other irregularities of the surface; nevertheless, the false color maps allowed for the discrimination of all these features. Because of the correct recognition of these features, Mujal and Marchetti (2020) assigned the tracks of MNHN.F.LOD83 to Ichniotherium cottae, being the first unambiguous report of this ichnogenus from the French Lodève Basin.
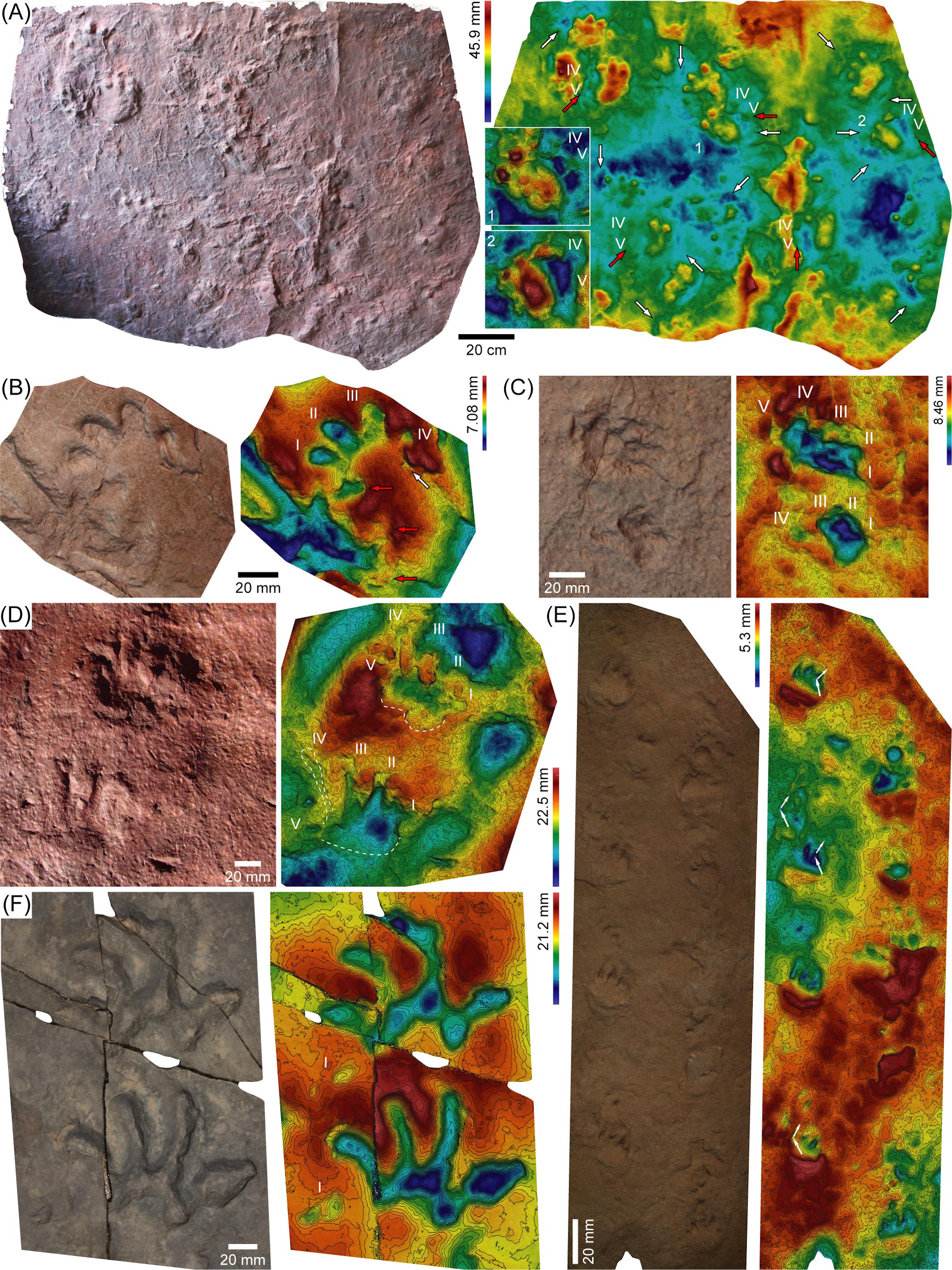
Figure 2. 3D models [except (A,D), which include a photo instead] and corresponding depth maps of Cisuralian (A–E) and Lopingian (F) ichnotaxa. (A) Tracks and trackways of Ichniotherium cottae (MNHN.F.LOD83), Lodève Basin; arrows indicate expulsion rims (white) and pedal digit V impressions (red); 1 and 2 indicate detailed 3D models of pes tracks showing digits IV and V. (B) Right manus-pes couple of Limnopus isp. (mold and replica IPS82608), Pyrenean Basin; arrows indicate parts of the pes track (red) and a hole on the substrate that apparently looked like a digit impression (white). (C) Left manus-pes couple of cf. Varanopus (mold and replica IPS82607), Pyrenean Basin. (D) Left manus-pes couple of Hyloidichnus isp., Pyrenean Basin. (E) Trackway in upslope progression of cf. Varanopus (YPM 2143), Coconino Formation; arrows indicate sliding and true orientation of digit impressions. (F) Right manus-pes couple of Karoopes gansfonteinensis (GF-TR 1), Karoo Basin.
- In some Limnopus isp. specimens (some of the originals were not collected; e.g., mold and replica IPS82608), from the Artinskian of the Catalan Pyrenees studied by Mujal et al. (2016b), the actual shape of the tracks (including also diagnostic features of the ichnogenus, such as the number of digit impressions and their relative length and proportions) was inferred from the false color depth maps, which also revealed the presence of deformed pedal impressions (Figure 2B).
- The tracks referred to cf. Varanopus (original not collected; mold and replica IPS82607) by Mujal et al. (2016b) (Figure 2C) are notably deformed (most probably due to an originally water-saturated substrate), and the digit impressions were recognized only after the study of the false color depth maps. The 3D model that Mujal et al. (2016b) published better illustrates the orientation of the footprints, allowing observation of the trackway arrangement and thus providing correct measurement of the trackway parameters. In the same way, some digital impressions (e.g., from the pedal track in Mujal et al., 2016a; Figure 2C) were only recognized through the study of the 3D model, because their relief is relatively low. All these features are diagnostic of Varanopus; nonetheless, given the relatively poor preservation of the tracks, an open nomenclature was preferred by Mujal et al. (2016b), which we follow here.
- The Hyloidichnus isp. specimens (not collected) studied by Mujal et al. (2016b) could only be identified after analyzing the 3D model of a manus-pes set (Figure 2D). The impression of pedal digit IV was first identified in the false color depth map provided by Mujal et al. (2016b): such impression is partially masked (overprinted) by rain drop impressions and flow ripples. In fact, it is a very shallow impression that could not be distinguished on photographs due to the light conditions. After this first identification, further tracks from the same surface and stratigraphically close levels (not collected) were also classified as Hyloidichnus isp. by Mujal et al. (2016b).
- The type material of Laoporus (YPM 2143, 2144, USNM V 8422) from the Kungurian Coconino Fm. of the United States was recently re-analyzed by Marchetti et al. (2019d), who concluded that the supposed diagnostic features of this ichnogenus (subequal pes and manus with digit impressions of subequal length) were actually due to the locomotion on sandy inclined planes (dune foreset surfaces). The 3D model created by Marchetti et al. (2019d) allowed to identify both the generally deeper, parallel, and sub-equal digit drag traces and the actual digit imprints, which are generally shorter, shallower, and with a different orientation (arrows in Figure 2E). Therefore, the inferred digit impressions of Laoporus are actually digit drag traces parallel to the slope and they actually represent the digit sliding during locomotion. Therefore, this ichnogenus is considered a nomen dubium and this material is assigned to cf. Varanopus isp. based on anatomy-consistent features, i.e., the actual length of digit impressions and their relative proportions (for further details, see Marchetti et al., 2019d) (Figure 2E).
- The holotype of Chelichnus duncani (DUMFM 5) from the Lopingian of Scotland, United Kingdom, was re-analyzed with the aid of photogrammetry by Marchetti et al. (2019c). The 3D model shows a ridge between the digit tip and the digit base impressions. This was an important feature for the assignment of this material to cf. Dicynodontipus and for its attribution to therapsid tracks. C. duncani is considered a nomen dubium due to its incompleteness, especially as regards the pes imprints.
- The study of the false color depth maps on 3D models was central for the ichnotaxonomic revision of the Guadalupian–Lower Triassic tetrapod tracks from South Africa (Marchetti et al., 2019d). This is true especially for the erection of the ichnotaxon Karoopes gansfonteinensis, which is characterized by tracks more deeply impressed laterally (e.g., GF-TR 1, 2, 3, SAM-NN 3) (Figure 2F). This is an important feature different from some morphologically similar captorhinomorph tracks such as Hyloidichnus and Merifontichnus. The same is observed in material assigned to cf. Capitosauroides isp. (GF-TR 9, GFTS 1, SAM-PK-K 7878a, b). Because of the deeper lateral impression of these tracks, an assignment to Batrachichnus or Limnopus (anamniote ichnogenera) could be discarded by Marchetti et al. (2019d), although the overall morphology is quite similar, especially in incomplete imprints. Therefore, as we further discuss in section “Relative Depth Patterns on Permian to Triassic Tetrapod Footprints” below, the relative depth pattern allows the clear distinction of Karoopes and Capitosauroides from captorhinomorph and anamniote ichnotaxa, which are instead more deeply impressed medially.
- Several anatomical traits of Prorotodactylus mesaxonichnus from the Lower–Middle Triassic of the Catalan Pyrenees described by Mujal et al. (2017a), including impressions of pedal digits IV and V, as well as the proximal impression of pedal digit III, were first recognized in 3D models (red arrows in Figures 3A,B). This allowed the measurement of the actual length of the digit impressions and of the digit divarication. In addition, the equivalent depth patterns observed in specimens with an apparently different morphology (e.g., Figures 3A,B) allowed to assign this material to the same ichnotaxon.
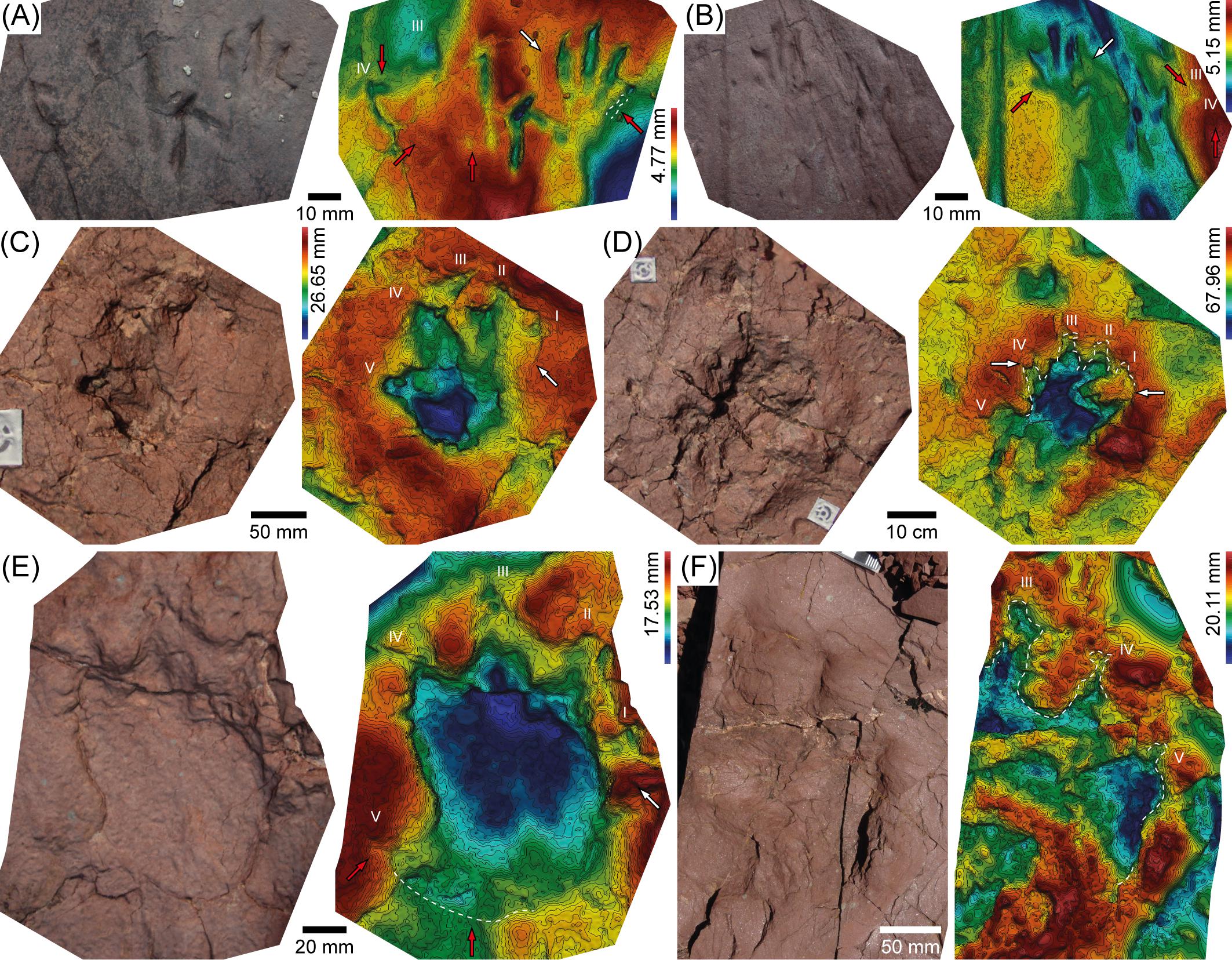
Figure 3. 3D models [except (F), which includes a photo instead] and corresponding depth maps of Lower–Middle Triassic archosauromorph tracks from the Pyrenean Basin. (A,B) Left (A) and right (B) manus-pes couples of Prorotodactylus mesaxonichnus. (C,D) Manus (C) and pes (D) tracks of a left couple of Chirotheriidae indet. (similar to Protochirotherium; mold and replica IPS82616). (E) Chirotheriidae indet. left track similar to Protochirotherium. (F) Large partial right pes track of Chirotheriidae indet. Arrows indicate anatomical (red) and sedimentary (e.g., digits collapse) (white) features; see text for discussion.
- The large archosauromorph tracks from the Lower–Middle Triassic of the Catalan Pyrenees described by Mujal et al. (2017a) were identified as Chirotheriidae footprints resembling Protochirotherium through the analysis of the 3D models. Several digit impressions are partially collapsed (white arrows in Figures 3C–E), and some parts of the impressed surface are missing due to natural erosion (Figures 3C–E); in one case a pes track preserves only three digit impressions (Figure 3F). Nonetheless, the false color maps allowed Mujal et al. (2017a) a correct interpretation of the digits outline and their relative position, showing the typical morphology of chirotheriid ichnotaxa.
Generally, despite different types of preservation and even in different (or slightly different) trackway parameters, examining the whole set of 3D models we observe a consistent (“homogeneous”) relative depth pattern within tracks of a single ichnotaxon, even if impressed in different substrates. This is because the tracks assigned to an anatomy-based ichnotaxon are impressed by the same type of trackmakers, which probably had autopodia with analog structure and morphology (e.g., Voigt et al., 2007; Marchetti et al., 2017a; Mujal et al., 2017a; Mujal and Schoch, 2020).
According to the recommendations by Belvedere et al. (2018) and Falkingham et al. (2018), 3D models, when possible, should be used in the definition of ichnotaxa, being tools to recognize ichnotaxobases (see also Bertling et al., 2006). When depth patterns are considered in ichnotaxonomy (i.e., contributing in ichnotaxobases), the use of 3D models is recommended. Henceforth, a further consideration must be raised: several holotypes of tetrapod ichnotaxa, from different geological periods correspond to tracks and trackways that were documented but not collected. In these cases, high fidelity molds and replicas were created instead. Here the question regarding such type material is highlighted. According to the ICZN rules (International Commission on Zoological Nomenclature, 1999), a holotype must correspond to an original fossil stored in an adequate repository (museum, research institute, university, etc.) for its safe keeping. However, this is not always feasible (especially in the case of trace fossils), and in most cases, the uncollected type material remains unprotected, and sooner or later will be lost due to erosion (Lucas and Harris, 2020).
Molds and casts of holotype specimens (i.e., “plastotypes”) are stored in institutions, but they can eventually deteriorate (as it could also occur with original specimens) as the life time of the mold/cast material is limited and suffers degradation through time (see also section “Preservation and Heritage” below). In this case further molds from the original fossil should be created through time, but the preparation of new molds from the first cast would easily imply a loss of fidelity with respect to the original fossil. As already suggested by Adams et al. (2010) [being further supported by Mallison and Wings (2014), as well as by Belvedere et al. (2018), and Lucas and Harris (2020)], we support the use of digital (3D) models as type material, i.e., digitypes (see further discussion on physical replicas by Lucas and Harris, 2020). 3D models have great advantages in the study, as well as in their sharing and repository. A recent study has partially applied such solution: Lockley et al. (2019) erected a new ichnotaxon of Pleistocene marine turtle tracks, the holotype of which remains in the field; the authors created a 3D model of the holotype trackway and printed part of it in order to appropriately store it in fossil collections, even if possibly the lifetime of the replica material is also limited.
Track-Trackmaker Correlation
The analysis of relative depth patterns of footprints is strictly linked to morphofunctional features of the possible trackmakers (see section “Relative Depth Patterns on Permian to Triassic Tetrapod Footprints” below), which can be inferred from the analysis of articulated and complete appendicular skeletons. In addition, the whole set of movements performed by the trackmaker’s limbs (and, potentially, by the whole body of the trackmaker) can also be elucidated by the study of depth patterns (e.g., Gatesy et al., 1999; Falkingham and Gatesy, 2014; Gatesy and Falkingham, 2017; Marchetti et al., 2017a; Mujal et al., 2017a; Mujal and Schoch, 2020). Therefore, a thorough study of these features is of fundamental importance for the track-trackmaker correlations. Following, different case-studies discussions are presented based on above considerations:
- The large tracks of Ichniotherium cottae preserved in MNHN.F.LOD83 (Figure 2A) are attributed to large basal diadectomorph reptiliomorphs, being different from those of smaller specimens of I. cottae and of I. sphaerodactylum (Mujal and Marchetti, 2020), which were produced by more derived taxa of the group (Voigt et al., 2007). In fact, the comparison of 3D photogrammetric models of different specimens (see Figure 5 by Mujal and Marchetti, 2020) showed that the large French I. cottae tracks display a more deeply impressed medial area, whereas smaller Ichniotherium tracks are more deeply impressed in their median part (Mujal and Marchetti, 2020). In this case, the differences in the relative depth of tracks, together with the relative position (rotation) of each track respect to the trackway midline, are a key feature to distinguish the most probable trackmakers. Therefore, when trackways are not available, 3D models of isolated tracks may still provide information for the track-trackmaker correlation (see also Mujal and Schoch, 2020).
- The ichnogenus Amphisauropus shows tracks more deeply impressed in their medial part, as suggested by Voigt (2005) and Avanzini et al. (2008). The 3D model of the Amphisauropus kablikae trackway (EMVG 2), from the Kungurian of the Italian Southern Alps and created by Marchetti et al. (2017a), confirms this hypothesis. According to the analysis by Marchetti et al. (2017a) of the false color depth maps (Figure 4A), the most deeply impressed area is placed at the base of digits I and II, where it is possible to observe a large circular pad impression (see red arrows and zoom inset in Figure 4A). This is exactly the same area that is subject to the stronger strain within the tarsus and carpus of the seymouriamorph Seymouria sanjuanensis. Therefore, this constituted an important feature for the correlation of Amphisauropus to the seymouriamorph group (Marchetti et al., 2017a). Of note, a similar medial functional prevalence, with digits I and II and their bases being much deeper than the rest of the footprint, is also observed in 3D models of temnospondyl tracks (Mujal et al., 2016a) (Figure 2B).
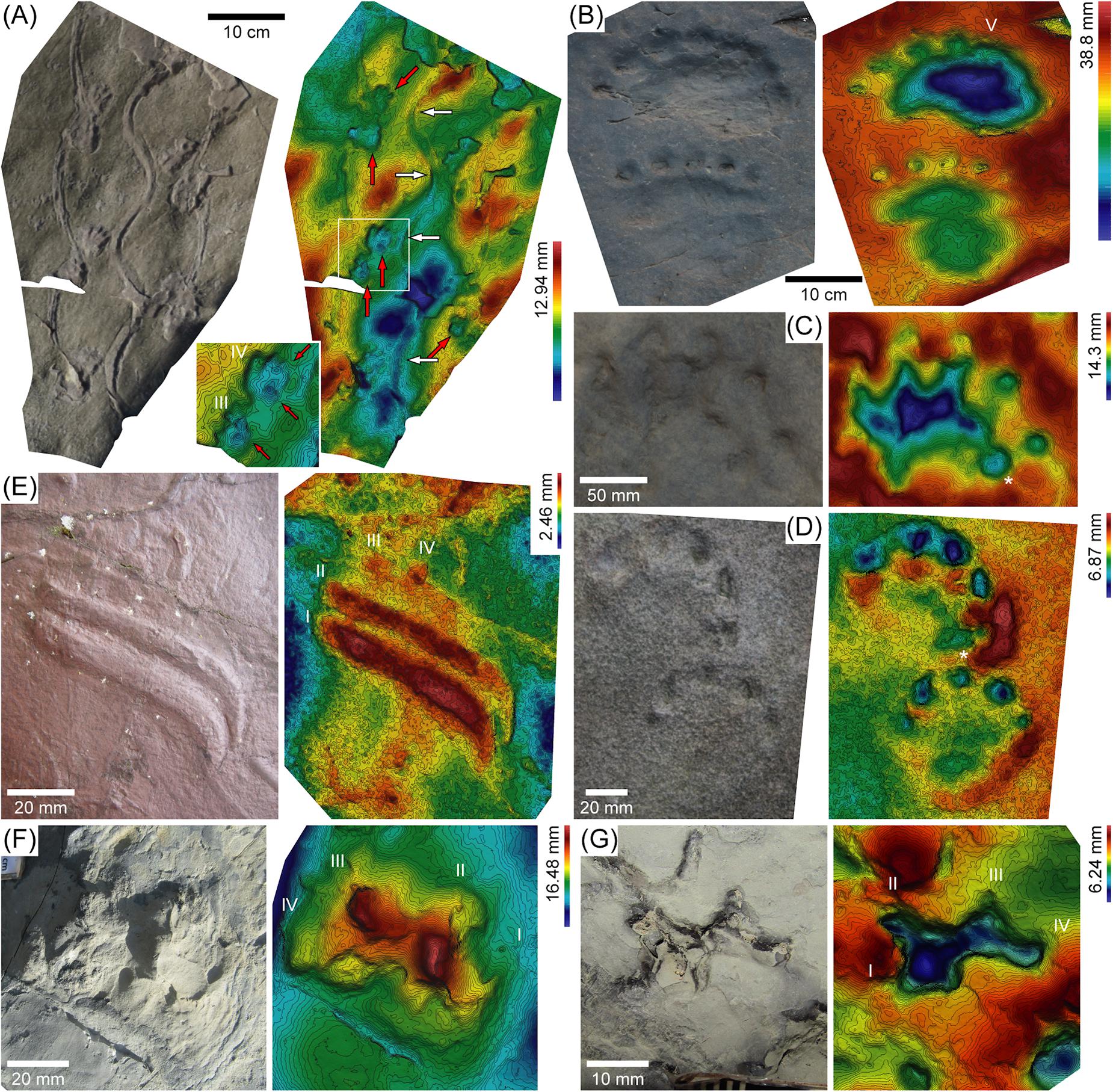
Figure 4. 3D models [except (E,F,G), which include photos instead] and corresponding depth maps of Cisuralian (A,E), Lopingian (B,C,D), and Ladinian (Middle Triassic) (F,G) ichnotaxa. (A) Trackway of Amphisauropus kablikae (EMVG 2), Orobic Basin; arrows indicate the deepest parts of tracks (red) and the steepest parts of the tail impression (white); zoom of one of the manus-pes couples (white square) showing the deeper medial side of tracks. (B) Right manus-pes couple of Brontopus giganteus (KW-TR 1), Karoo Basin. (C) Left manus track of Dolomitipes accordii (BE 3), Karoo Basin. (D) Left manus-pes couple of Dolomitipes isp. (UG-NN 1), Hessian Basin. Asterisks (*) in (C,D) indicate equivalent anatomical part. (E) Left manus track of Characichnos isp. produced by a Limnopus-trackmaker, Pyrenean Basin. (F,G) Right manus tracks of an indeterminate ichnotaxon attributed to capitosaur stereospondyl trackmakers by Mujal and Schoch (2020); (F) is a track natural cast not recovered and (G) is preserved in SMNS 97003.
- The identification of footprint depth patterns was central in the track-trackmaker correlation of the Guadalupian–Lower Triassic therapsid tracks from South Africa carried out by Marchetti et al. (2019d). The very broad and more deeply impressed lateral part of the palm of the ichnogenus Brontopus (KW-TR 1; Figure 4B) suggests that trackmakers were dinocephalian therapsids, which have a broad metacarpal V. The more deeply impressed manus compared to pes imprints helped in the attribution of the ichnogenus Dolomitipes (BE-TR 1, AM 5744/5) (Figures 4C,D) to dicynodont therapsids, which are characterized by a marked postural dichotomy. The more deeply impressed lateral side of footprints helped Marchetti et al. (2019d) in the attribution of the ichnotaxa Karoopes (GF-TR1, 2, 3, SAM-NN 3) (Figure 2F) and Capitosauroides (GF-TR 9, GFTS 1, SAM-PK-K 7878a, b) to therapsid synapsids.
- In the tetrapod ichnoassemblages from the Lower–Middle Triassic of Port del Cantó and Tossal de Pollerini ichnosites (Pyrenean Basin), Mujal et al. (2017a) observed a similar depth pattern between different ichnotaxa: the posterior (proximal) part of digit I impression displays an elevated relief (Figure 3). This is related to the collapse of the footprint wall in its side (white arrows in Figure 3C–E). In other cases, this area is less impressed (red arrows in Figures 3A,B). Such features could be linked to the nature of the footprint producers, all of which are considered to be archosauromorphs (Mujal et al., 2017a). Alternatively, this could be the result of a similar gait of the trackmakers under similar substrate conditions. In any case, the impressions of digits II, III, and IV from both manus and pes tracks are usually the deepest (though exceptions exist: e.g., Mujal et al., 2017a), denoting a trend toward a tridactyl functional prevalence of autopodia. This is the case of chirotheriid ichnotaxa, as well as of other archosauromorph tracks, such as Prorotodactylus, Rotodactylus, and Rhynchosauroides (the later also referred to lepidosauromorph trackmakers) (Mujal et al., 2015, 2017a,2018b).
Locomotion and Behavior
Differential depths within a track, as well as subtle differences in steepness of impressions, are often indicative of the functional prevalence and progression direction of the trackmakers (Figures 4, 5). Footprints and trackways may record the whole set of limb movements (or at least partially) (e.g., Gatesy and Falkingham, 2017; Mujal and Schoch, 2020). In these cases, 3D models help to elucidate the timeline of footprints impression.
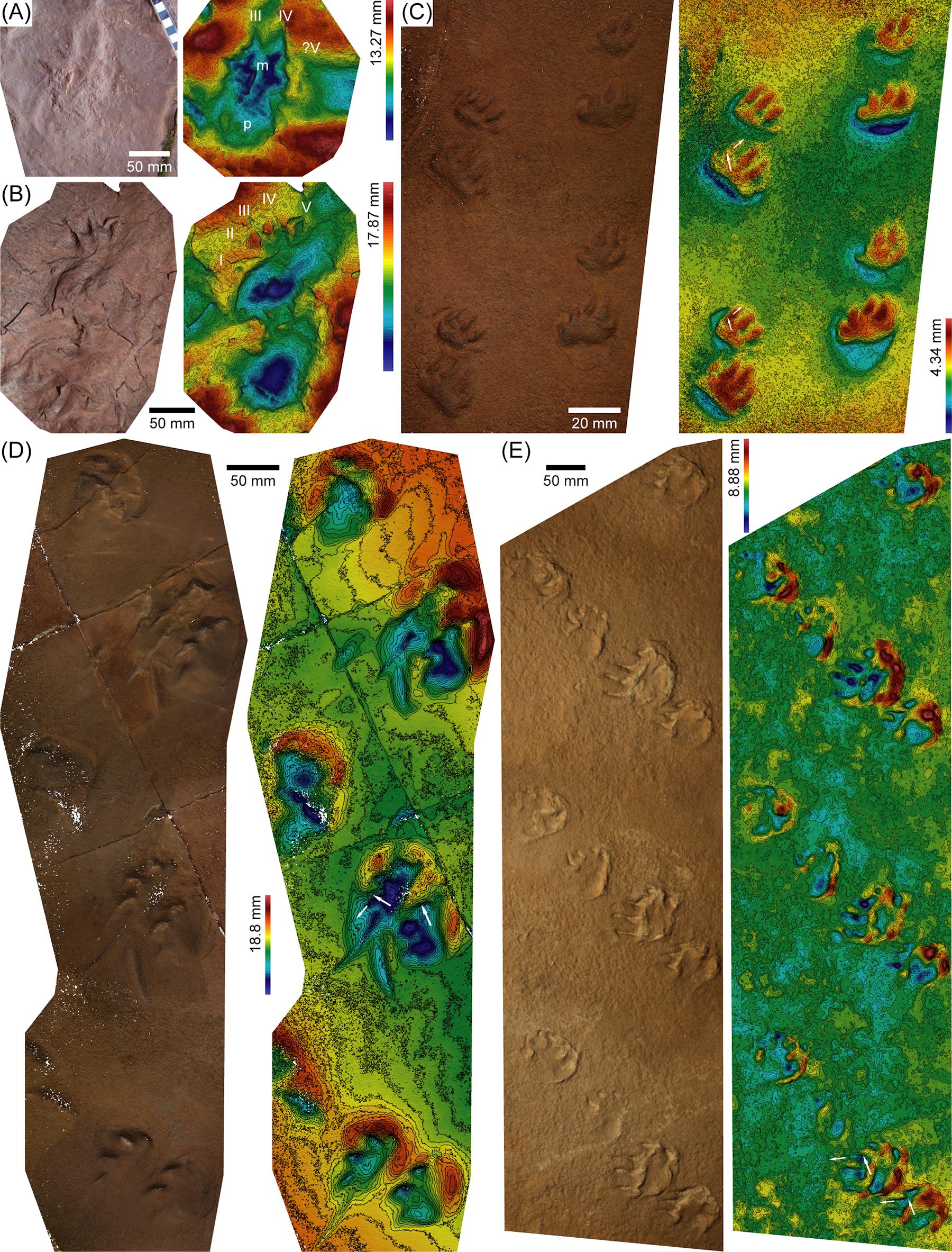
Figure 5. 3D models [except (A), which includes a photo instead] and corresponding depth maps of Cisuralian (A–D) and Lopingian (E) ichnotaxa. (A,B) Right manus-pes couples of Dimetropus leisnerianus, Pyrenean Basin; note that tracks in (A) are partially eroded. (C) Trackway in upslope progression of Varanopus curvidactylus (MNA-V 3327), Coconino Formation. (D) Trackway in downslope progression of Amphisauropus isp. (MNA-V 3442A), De Chelly Formation. (E) Trackway in upslope progression with significant lateral component of Dolomitipes isp. (NMK-PAL 183), Hessian Basin. Arrows in (C–E) indicate sliding and true orientation of digit impressions.
The 3D model of the Amphisauropus kablikae trackway in EMVG 2 was used in the identification of the locomotion pattern by Marchetti et al. (2017a) (Figure 4A): the tail impression displays different steepness of the lateral margins along the trackway (white arrows in Figure 4A). This, combined with the relative position of the tail impression respect to the tracks, indicates the direction of movement of the tail and the body of the trackmaker during locomotion. Moreover, the continuous digit drag traces indicate the precise position of the limbs during the entire step cycle. The 3D model provided additional clues to Marchetti et al. (2017a) on the digits that were closer to the substrate while lifted and thus generated deeper drag traces. It should be taken into account that the observed depth patterns and different steepness along the trackway are small, often indiscernible to the naked eye.
The Permian tetrapod footprints from eolian paleoenvironments were commonly preserved on the foreset dune surfaces (e.g., Gilmore, 1926, 1927; Schmidt, 1959; McKeever and Haubold, 1996). Since these surfaces were inclined and the sand was likely dry at the time of impression (as indicated by the sand avalanches and expulsion rims; the sand was probably dampened shortly after the impression or in the first phases of burial, allowing the preservation of tracks; Marchetti et al., 2019b; for further details on track preservation, see Mancuso et al., 2016, and references therein), the tetrapod locomotion could be difficult, and it was influenced by the force of gravity not perpendicular to the trampled surface. This caused asymmetric trackway pattern, footprint morphology, and footprint depth in trackways not perpendicular to the slope, which were caused by the trackmaker trying to oppose the force of gravity. While these effects are long known (e.g., Leonardi, 1987; Fornós et al., 2002; Hunt and Lucas, 2005; Loope, 2006; Krapovickas et al., 2015), only recently have they been thoroughly interpreted with the aid of the 3D models (Marchetti et al., 2019b, c). The most accurate tool to evaluate these asymmetries is certainly the study of false color depth maps and contours from 3D models. Commonly, the downslope side of the asymmetric trackway is more deeply impressed and shows higher expulsion rims. This was observed by Marchetti et al. (2019b, c) in several specimens, including trackways of Amphisauropus (MNA-V 3442A; Figure 5D), Ichniotherium (UCMP 42945), cf. Tambachichnium (USNM-V 11502), Dolomitipes (UG-NN 1; Figure 4D), and Pachypes (DUMFM-NN 2).
In all the 3D models of Prorotodactylus mesaxonichnus manus tracks from the Lower–Middle Triassic of the Catalan Pyrenees created by Mujal et al. (2017a), a broad and relatively high expulsion rim between the impressions of digits IV and V is observed (white arrow in Figures 3A,B). This feature, clearly visible in the false color maps (but often unseen at naked eye), indicates that the forelimbs applied a postero-lateral pressure during the taking off phase (Mujal et al., 2017a). Hence, the manus was slightly rotated outwards and/or the trackmaker performed a lateral movement of the trunk (as also suggested by the sinuous tail impressions of some trackways; Mujal et al., 2017a).
The ichnogenus Characichnos is interpreted as the trace fossil of swimming tetrapods. Characichnos generally consists of digit drag traces on the substrate, therefore, not fully representing the anatomy of the trackmaker’s autopodia (except for number of digits and width) but rather its behavior. In fact, Characichnos tracks are associated with different ichnotaxa and are attributed to very different trackmakers, in a very long stratigraphic interval (e.g., Thomson and Droser, 2015; Vila et al., 2015; Mujal et al., 2016a). Nevertheless, in some cases Characichnos may show features similar to the associated ichntoaxa, possibly due to specific morphofunctional characteristics of the trackmaker. This was observed by Mujal et al. (2016b), who performed 3D models of Characichnos isp. (although not figured there) associated with Limnopus isp. tracks (mold and replica IPS82604) from the Artinskian of the Catalan Pyrenees. The 3D models reveal the same depth pattern in trace fossils assigned to both ichnogenera. In some cases, only impressions of digits I and II are preserved as scratches, and the false color depth map revealed that these scratches are associated with two digit tip imprints corresponding to digits III and IV (Figure 4E). This trait enhanced the correlation between the two track morphologies (Limnopus and Characichnos, walking and swimming tracks, respectively; see Mujal et al., 2016a). This provides additional support to the interpreted medial functional prevalence of the trackmakers’ forelimbs, with digits I and II more deeply impressed (or in this case, producing deeper drag traces) than digits III and IV (see section “Relative Depth Patterns on Permian to Triassic Tetrapod Footprints” below).
The set of tracks from the Ladinian of southern Germany, referred to temnospondyls producers by Mujal and Schoch (2020), could not be assigned to a specific ichnotaxon due to the paucity and preservation of the material. Nevertheless, the 3D models of footprints revealed a consistent depth pattern within footprints of apparently different shape due to their different size and preservation mode (Figures 4F,G). This allowed Mujal and Schoch (2020) to decipher a consistent locomotor pattern of the trackmakers (interpreted as a bottom-walking locomotion in subaqueous conditions), hence implying that they most probably correspond to the same trackmaker group.
Substrate Induced Effects
The 3D model of MNHN.F.LOD83 from the Asselian of Lodève, southern France (Mujal and Marchetti, 2020), reveals the presence of shallow and relatively wide expulsion rims around most of the tracks (white arrows in Figure 2A). Such structures remain difficult to identify by the naked eye due to their low relief: they were not reported by Heyler and Lessertisseur (1963), and Mujal and Marchetti (2020) noticed them only after the analysis of the 3D models. The identification of expulsion rims allowed for better inference of the substrate conditions, despite tracks preserved as plaster casts and the original substrate is lost. The expulsion rims, together with the relative depth patterns of the tracks, denote a peculiar fact: those from the pes tracks are higher (in this case, deeper, as they are casts) than those of the manus tracks, whereas manus tracks are much better impressed than pes tracks. This indicates an impression of the hindlimbs relatively stronger than of the forelimbs (but more focalized to a part of the autopodia in the case of the hindlimbs; see further discussion in Mujal and Marchetti, 2020).
A manus-pes set of Dimetropus leisnerianus from the Artinskian of the Catalan Pyrenees (not collected) was strongly eroded (smoothed) by the water flow probably soon after the impression of the footprints (Mujal et al., 2016a) (Figure 5A). Indeed, in the field, tracks were identified as shallow irregular impressions within a surface featured by unidirectional flow ripples. The identification of such impressions as D. leisnerianus was possible thanks to the 3D model: the false color depth map shows the depth pattern that characterizes this ichnospecies, with a broad and rounded sole impression and relatively thin digit impressions; the same can be observed in other manus-pes couples found by Mujal et al. (2016b) within the same stratigraphic succession (Figure 5B).
The morphology of Permian tracks from eolian paleoenvironments was strongly influenced by the substrate (Marchetti et al., 2019b, c). This is because the track-bearing surfaces were foreset dune surfaces; thus, they were relatively dry sand slopes that caused difficulty in trackmaker progression and very common digit sliding and sand avalanches. These digit drag traces were generally parallel to the slope and were generated when the trackmaker’s foot slid downslope with respect to the touch-down position. This was observed in trackways directed parallel, perpendicular, and transverse to the slope and masks the original orientation and morphology of the digits, causing problems in ichnotaxonomy (Marchetti et al., 2019b, c; Francischini et al., 2020). In order to correctly interpret this substrate-induced effect and recognize the actual digit impressions, the analysis of the 3D models is very helpful. Some notable examples of this are observed for trackways of Amphisauropus, Varanopus, and Dolomitipes with different orientations compared to the slope studied by Marchetti et al. (2019b, c) (RAM-NN 1, UCMP V 42944, MNA-V 3327, MNA-V 3442A, NMK-PAL 183, YPM 2143) (Figures 2E, 5C–E).
Preservation and Heritage
Collecting and/or excavating tetrapod footprints (and especially complete trackways) is often difficult or impossible. Therefore, an alternative solution in order to preserve the fossil record must be found. Traditionally, the use of molds and replicas of tracks and complete trackways has fulfilled this necessity (e.g., Heyler and Lessertisseur, 1963; Gand et al., 2000; Mujal et al., 2016a, b, 2017a; Marchetti et al., 2019d; Lucas and Harris, 2020). However, such molds and casts usually do not preserve the whole record of a site but a small part of it and even if adequately stored, can be deteriorated, depending on the lifetime of the mold/cast material nature, even becoming useless, as all material has a lifetime before degradation (mostly being of silicone, gypsum, acrylic resin, etc.).
The preservation of the tetrapod ichnofossil record may present some difficulties that should be considered. This is commonly due to difficult access to the localities (either because they are in private properties or due to orographic characteristics, i.e., remote areas) that may lead to the loss of specimens due to erosion and weathering. This problem has been faced by several of our works, in which photogrammetry has been a useful tool to overcome the impossibility to collect/cast/move specimens (e.g., Mujal et al., 2016a, b, 2017a,b; Marchetti et al., 2019b, d; Mujal and Marchetti, 2020):
- Manipulation of large casts can be harmful for their preservation, as they can be damaged during analysis. Nevertheless, 3D photogrammetric models facilitate the analysis, as tracks can be (virtually) moved and observed in different perspectives. This was indeed the case of MNHN.F.LOD83 (see Mujal and Marchetti, 2020).
- Photogrammetry is advantageous through time: previous non-optimal photogrammetric results may become useful thanks to newer versions of software. In fact, herein we have re-run a model using the same photos of a Limnopus isp. manus-pes set (IPS73724) and results show that the new 3D model is more detailed than the previous one (which due to its poor resolution was discarded by Mujal et al., 2016a) and also ichnologically informative (Figure 6A).
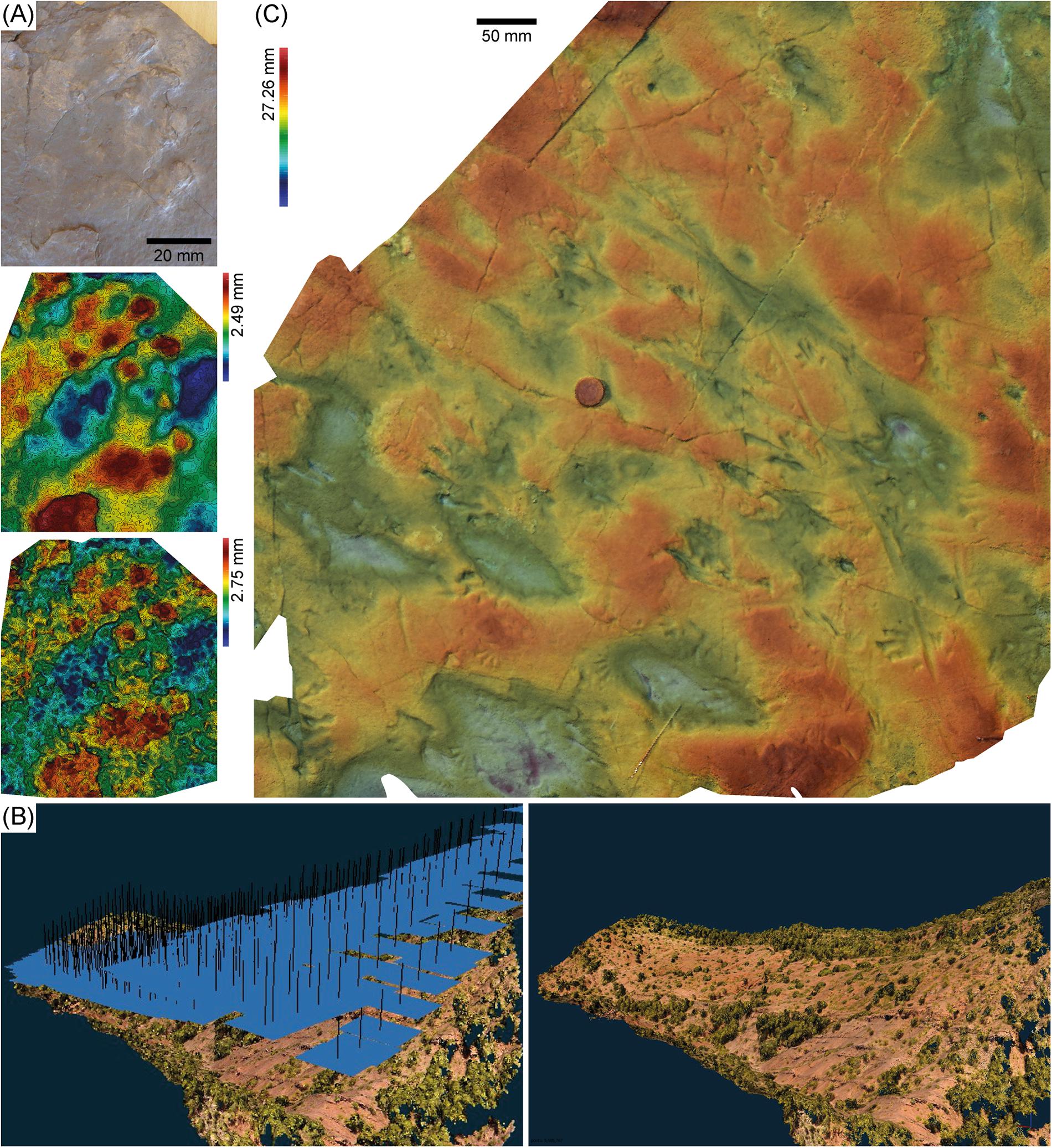
Figure 6. (A) Photo (top) and depth maps of the 3D models (new, middle; old, bottom) of a right manus-pes couple of Limnopus isp. (IPS73724), Pyrenean Basin, Cisuralian; note that the surface is much smoothed in the new model, allowing better elucidation of footprint features. (B) Captures of the 3D model obtained with drone of the Coll de Terrers outcrop, Pyrenean Basin, Guadalupian–Lopingian; left image contains the position of each photo. (C) 3D model with the depth map superimposed on transparency of a large surface containing abundant Prorotodactylus mesaxonichnus tracks, including the trackway paratype (mold and replica IPS93867), Pyrenean Basin, Early–Middle Triassic.
- Creation of molds (and replicas) can be impossible due to difficult access to localities (e.g., remote areas only accessible by foot, crossing creeks and relatively dense forests) and to the situation of the track-bearing surfaces (in high slopes, being even vertically exposed), as is the case of Catalan Pyrenees localities. In the case of relatively small tracks (e.g., 2–5 cm long), molds may still be possible to create (e.g., Mujal et al., 2016b, a, 2017a), although those of relatively large trampled surfaces are often of difficult transport and are easily damaged, implying further careful lab work (EM and JF, pers. obs. 2016). Therefore, 3D photogrammetry is the optimal tool for the preservation of such record. Recently, during fieldtrips to Tossal de Pollerini and Coll de Terrers sites (Mujal et al., 2017a, b) an unnamed aerial vehicle was used to take photos for the creation of high-resolution 3D photogrammetric models of: (1) the whole outcrops (Figure 6B) (for similar documentation of tracksites, see Citton et al., 2017; Romilio et al., 2017; Petti et al., 2018) and (2) relatively large (∼2–5 m2) track-bearing surfaces (with sets of around 100 photos per surface) (Figure 6C). Besides providing new ichnological and sedimentological data (currently under analysis), these new models constitute a digital repository of this paleontological heritage.
- The most important Guadalupian–Lower Triassic tetrapod ichnosites from South Africa (Marchetti et al., 2019d) are surfaces left in place on private property. This is potentially harmful for the preservation of this heritage because of erosion and weathering while the tracksite remains unprotected. This is the case of Klein Waterval and Bethel sites, which are located in canyons/rills and covered by sediment at the time of field work (LM, pers. obs. 2018). Some latex casts of portions of these surfaces are preserved in repositories, but their quality is often not adequate for a new study. Therefore, the creation of 3D photogrammetric models of the most important trackways and surfaces from all these localities, as Marchetti et al. (2019d) did, is the ideal way to preserve such heritage for the scientific community (for further discussion see also Reolid et al., 2020).
Relative Depth Patterns on Permian to Triassic Tetrapod Footprints
Herein we present and discuss the relative depth patterns of tetrapod tracks and their implications for the functional prevalence of autopodia in a phylogenetic framework.
Substrates and Paleoenvironments
The localities of the herein reviewed tetrapod footprints encompass a wide range of substrates and paleoenvironments (see section “Tetrapod Ichnoassociations from the Lower Permian to the Middle Triassic” above and Supplementary Data S1). Most of the localities belong to siliciclastic successions commonly including red-beds, but also dark mudstones. Such successions usually represent alluvial, fluvial, and lacustrine paleoenvironments. Besides, a few of them represent coastal (e.g., sabkhas and tidal flats) and eolian paleoenvironments. In a few cases, the track-bearing substrate is a carbonate mudstone, usually of microbial origin, in coastal or restricted marine settings.
More specifically, the substrate preserving footprints, either in concave epirelief or convex hyporelief (or both, if part and counterpart strata/slabs are found) includes: (1) mudstones (claystones and siltstones), either massive or laminated; (2) sandstones, ranging from very fine-grained to coarse-grained, either massive or with parallel lamination and/or flow and wave ripples; (3) fine-grained and well-sorted (eolian) sandstones with large-scale cross lamination, wind ripples, and sand avalanches; (4) fine grained limestones (i.e., carbonate mudstones), either massive or laminated. Footprint surfaces are either flat or undulated; other structures, such as desiccation cracks, raindrops and wrinkles, as well as other vertebrate and/or invertebrate trace fossils, may also be present. In many cases, other trace fossils and structures cross, overprint, and/or mask the analyzed tetrapod footprints. In such cases, 3D models help to elucidate the sequence of events and, as a result, to correctly identify the footprint outline (see Marchetti et al., 2013; Mujal et al., 2015).
Although paleoenvironmental settings (and paleoclimatic conditions) constrain the distribution of tetrapod ichnotaxa (e.g., Mujal et al., 2016a), we observe that the same ichnotaxon can be present in a relatively wide range of substrates (e.g., Mujal et al., 2018a). Also, the absolute depth of footprints from the same ichnotaxon (or even along the same trackway) can vary consistently on different substrates, from very deep, to intermediate, and to very shallow. Nevertheless, all the diagnostic (anatomical) features of ichnotaxa (e.g., digit number, morphology and relative length, palm/sole impression shape and size, relative depth within a footprint) do not vary, even if their recognition relies on the effects caused by substrate rheology and behavior (see Gatesy and Falkingham, 2017; Mujal et al., 2018a; Marchetti et al., 2019a). The whole set of 3D photogrammetric models (i.e., both reviewed from previous publications and newly presented, see Figures 2–7) show a consistent relative depth pattern within pes and manus imprints of each ichnotaxon, independently from the absolute depth of each footprint. Therefore, the original substrate conditions (rheology) and environmental settings do not play a major role in the relative depth pattern (i.e., the diagnostic 3D features) observed within tracks of the same ichnotaxon, whereas their absolute depth may vary consistently (see also discussion in Mujal et al., 2018a).
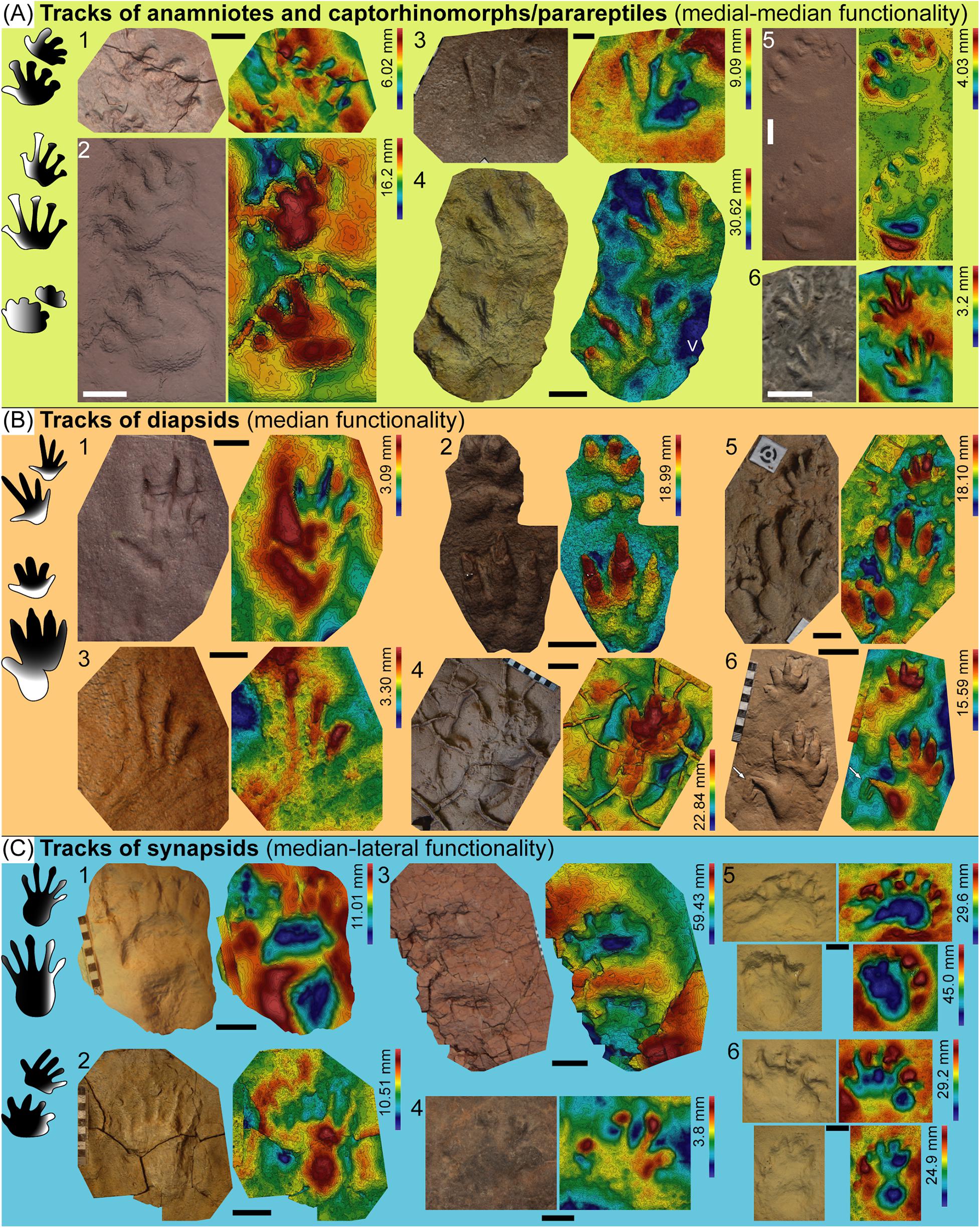
Figure 7. Depth patterns among Cisuralian to Middle Triassic tetrapod ichnotaxa shown by selected 3D models and corresponding depth maps. In the left part of each section, schematic unscaled left manus-pes couples showing the parts with functional prevalence (black areas) of each group are depicted. (A) 1 left couple (partial pes track) of Limnopus isp. (mold and replica IPS82606), Pyrenean Basin, Cisuralian; 2 left couple of Ichniotherium sphaerodactylum (UGKU 130), Tambach Formation (Germany), Cisuralian; 3 left couple (partial manus track) of Hyloidichnus isp. (MF-LOD 2001.1447.1), Lodève Basin, Cisuralian; 4 left couple of Merifontichnus thalerius (UM-LOD 16), Lodève Basin, Guadalupian; 5 right couple of Pachypes loxodactylus (DUMFM-NN 2), Dumfries Basin, Lopingian; 6 left couple of Procolophonichnium nopcsai (NHUMK R3173), Karoo Basin, Early Triassic. (B) 1 left manus track of Prorotodactylus mesaxonichnus (mold and replica IPS93869), Pyrenean Basin, Early–Middle Triassic; 2 left couple (partial pes track) of Chirotherium barthii (mold and replica IPS17899), Catalan Basin (Buntsandstein), Middle Triassic; 3 right pes track of Rhynchosauroides isp. (IPS73720), Catalan Basin (middle Muschelkalk), Middle Triassic; 4 right pes track of Isochirotherium isp. (IPS81873), Catalan Basin (middle Muschelkalk), Middle Triassic; 5,6 left couples of Chirotherium barthii (MF-LOD 2001.1438.1 and MF-LOD 2001.1437.1, respectively), and right pes track of Rotodactylus isp. (arrow in 6), Lodève Basin, Middle Triassic. (C) 1 and 2 pes tracks of Dimetropus leisnerianus (1, right imprint, MNHN.F.LOD59; 2, left imprint –undertrack–, UM-LOD 260), Lodève Basin, Cisuralian; 3 right couple of a Dicynodontipus-like morphotype, Pyrenean Basin, Lopingian; 4 left pes of cf. Capitosauroides (SAM-PT-K 7870), Karoo Basin, Lopingian; 5 right couple of Dolomitipes icelsi (AS-TR Q), Karoo Basin, Lopingian; 6 left couple of Dolomitipes accordii (AS-TR 2), Karoo Basin, Lopingian. Scale bars represent 10 mm [in 1 and 3 of (B)], 20 mm [in 1, 3, 5, and 6 of (A), and 4 of (C)], 50 mm [in 2 and 4 of (A), 2, 4, 5, and 6 of (B), and 1 and 2 of (C)] and 10 cm [in 3, 5, and 6 of (C)].
Our qualitative interpretation of footprint relative depth patterns allows us to identify the functional prevalence of the autopodia, which results from specific morphofunctional features of the trackmaker, thus further linking the ichnotaxa to the potential trackmakers (see section “Relative Footprint Depth Patterns and Functional Prevalence of Autopodia” below). 3D models elucidate potentially biased observations related to the absolute depth of footprints: false color depth maps help to minimize the differences between shallowly and deeply impressed tracks of the same ichnotaxon (e.g., see Figures 5H,I of Mujal and Marchetti, 2020). As highlighted in the introduction of the present work, there is a major difference between upper Carboniferous to Triassic tetrapod ichnology and non-avian dinosaur ichnology. For instance, a deeply impressed theropod dinosaur track may show four digit impressions, whereas a shallow one would be just tridactyl, which could eventually lead to oversplitting and misinterpretation of ichnogenera (for a thorough study of such case, see Razzolini et al., 2014). On the contrary, within upper Carboniferous, Permian, and Triassic tetrapod tracks the anatomy-related morphological features are the same in deep and shallow imprints (only very shallow tracks may show less digit and/or palm/sole impressions) and/or in imprints impressed on mudstones, siltstones, carbonates, and dune sandstones. In fact, in different substrates or substrate rheological properties the relative depth pattern of tracks assigned to the same ichnotaxon is generally constant (especially if trackmakers maintain the same locomotion; e.g., Mujal et al., 2018a; Marchetti, 2019; Mujal and Schoch, 2020). Even on originally inclined surfaces, which caused additional asymmetries to the footprint depth, the relative depth pattern within footprints of the same ichnotaxon is still recognizable (e.g., Marchetti et al., 2019b). In fact, ichnotaxonomy is based on footprint outline morphology and relative depth patterns of the impressions (i.e., anatomical features of autopodia) (Voigt, 2005). Hence, relative depth patterns are recognizable in any substrate. In this sense, only footprints with substantial extramorphological variations (that completely mask/overprint anatomical features; see Peabody, 1948; Haubold, 1996) are excluded from such analyses (see section “Substrate Induced Effects” above).
Relative Footprint Depth Patterns and Functional Prevalence of Autopodia
As a whole, we preliminary identify three main groups of upper Carboniferous–Middle Triassic ichnotaxa (selected, representative specimens are shown in Figure 7) characterized by a different functional prevalence:
1) Anamniote and captorhinomorph/parareptile tracks: medial-median functional prevalence (Figures 2A–E, 4A,E–G, 5C,D, 6A, 7A).
2) Diapsid tracks: median functional prevalence (Figures 3, 6C, 7B).
3) Synapsid tracks: median-lateral functional prevalence (Figures 2F, 3B–D, 5A,B,E, 7C).
The first group of tracks (Figure 7A) includes ichnogenera produced by temnospondyl and lepospondyl amphibians (Batrachichnus, Limnopus, Matthewichnus), seymouriamorphs (Amphisauropus), diadectomorphs (Ichniotherium), and captorhinomorph/parareptiles (Erpetopus, Hyloidichnus, Merifontichnus, Notolacerta, Procolophonichnium, Pachypes, Varanopus). The medial (inner) part of these tracks (usually digits I and II, and the corresponding portion of the palm/sole) are generally the most deeply impressed parts. Depth patterns may vary between manus and pes tracks, the medial-lateral decrease in relief is usually more evident in the pes tracks. Besides, the trackways are often broad, with relatively low pace angulations (with the only exception of some captorhinomorph/parareptile tracks such as Erpetopus and Varanopus, which show also relatively high pace angulation and primary pes-manus overstep).
The second group of tracks (Figure 7B) includes ichnogenera produced by diapsid reptiles, being archosauromorph tracks the most representative group. It includes ichnogenera from the Chirotheriidae ichnofamily (e.g., Brachychirotherium, Chirotherium, Isochirotherium, Parachirotherium, Protochirotherium, Sphingopus, Synaptichnium), dinosauromorph tracks (e.g., Atreipus, Eubrontes, Evazoum, Grallator), relatively small-sized non-chirotheriid ichnotaxa such as Dromopus, Prorotodactylus, Rotodactylus, and Rhynchosauroides, as well as larger non-chirotheriid ichnotaxa such as Apatopus and Paradoxichnium. Of note, the Rhynchosauroides trackmakers may correspond to archosauromorphs or lepidosauromorphs, hence broadly corresponding to neodiapsid reptiles. Interestingly, also the varanopid ichnogenus Tambachichnium belongs to this group. Recently, varanopids have been interpreted as diapsids instead of synapsids (Laurin and Piñeiro, 2018; Ford and Benson, 2020; but see discussion in MacDougall et al., 2018). Based on the present analysis of relative depth patterns, different options could explain why varanopids are found within the group of diapsid tracks: (1) perhaps they reflect a very basal position of varanopids within synapsids; (2) a functional/convergent signal rather than a phylogenetic signal for this group. However, the latter option seems unfeasible as, in general terms, functional prevalence is correlated with phylogenetical signal. The first option is herein preferred; nonetheless, it should be remarked that the present analysis of depth patterns is preliminary, including only one ichnotaxon (Tambachichnium) correlated to varanopids, not precluding that further sampling could reveal differences across varanopid evolution.
Tracks of the second group are most commonly produced by autopodia with semidigitigrade to semiplantigrade postures, and digits II, III, and IV are usually the deepest, being in many cases the only impressed parts of the autopodia. Noteworthy, some chirotheriid tracks also display a relatively deep impression of the proximal pad of pedal digit V, being similar to that of digits II, III, and IV (see 5 and 6 in Figure 7B). Trackways are usually narrow, though most of the Dromopus and Rhynchosauroides specimens and some Prorotodactylus specimens display relatively low pace angulations (Mujal et al., 2017a). In the small-sized ichnotaxa, a certain degree of overstepping of the pes respect to the manus impressions is common, ranging from a slight lateral position of the pes respect to the manus to a complete overstepping of the pes, being positioned to the anterolateral side of the manus tracks. Generally, the tracks of this group show a trend toward a tridactyl functionality that can be mesaxonic (e.g., Chirotherium, Isochirotherium, Parachirotherium, Sphingopus, Atreipus), as observed in ornithopod and theropod dinosaur tracks (e.g., Castanera et al., 2013; Lallensack, 2019), or ectaxonic (e.g., Dromopus, Rhynchosauroides, Rotodactylus), as observed in most modern diapsids.
The third group of tracks (Figure 7C) is attributed to the synapsid group (i.e., including therapsids), with the exception of varanopid synapsid tracks (i.e., Tambachichnium; see discussion above). This group of tracks includes ichnogenera from the Pennsylvanian–Cisuralian (e.g., Dimetropus), the Guadalupian (e.g., Brontopus), the Lopingian (e.g., Capitosauroides, Dicynodontipus, Dolomitipes, Karoopes), and the Triassic (e.g., Capitosauroides, Dicynodontipus, Pentasauropus, Therapsipus) (see also Figure 9 by Marchetti et al., 2019d). These tracks often show a more deeply impressed digit IV, or in some cases even digit V, as well as the parts of palm/sole impressions proximal to digits IV and V. In addition, these tracks are generally suggestive of trackmakers with a plantigrade posture, with relatively broad sole and palm impressions and short digit imprints. Complete overstep of pes respect to manus tracks is uncommon and only observed in the ichnotaxa Dicynodontipus and Karoopes.
The relative depth patterns of footprints become more significant when mapped onto a cladogram of tetrapods (Figure 8). Although the discussion on the phylogeny at the amniote and reptile origin is currently debated (e.g., Laurin and Piñeiro, 2017, 2018; MacDougall et al., 2018; Ford and Benson, 2020), the three groups that we identify are very wide. We plotted our groups on two cladograms based on MacDougall et al. (2018) (see Figure 8A) and Ford and Benson (2020) (see Figure 8B), both of which including a possible new position of the varanopid group, being out of Synapsida. Group 1 apparently forms the primitive condition of upper Carboniferous to Middle Triassic tetrapods, as it occurs in both the putative lissamphibian stem-group (temnospondyls) as well as amniote stem lineages (e.g., seymouriamorphs and diadectomorphs), which are at the base of both cladograms represented (Figure 8). Interestingly, group 1 does not only encompass anamniotes and stem amniotes, but also extends to parareptiles and captorhinids, which form successive sister taxa of diapsid reptiles (Reisz et al., 2011; MacDougall et al., 2018) (Figure 8A). This suggests that the depth pattern of group 2 (diapsid tracks) derived from the depth pattern of group 1 (probably from captorhinomorphs), independently from the evolution of the synapsid footprint depth pattern (group 3), which forms a separate modification from the basal tetrapod footprint depth pattern (Figure 8). Further studies should focus on the question why the pattern of group 1 is so widespread across early tetrapods and what this means in functional and locomotory terms.
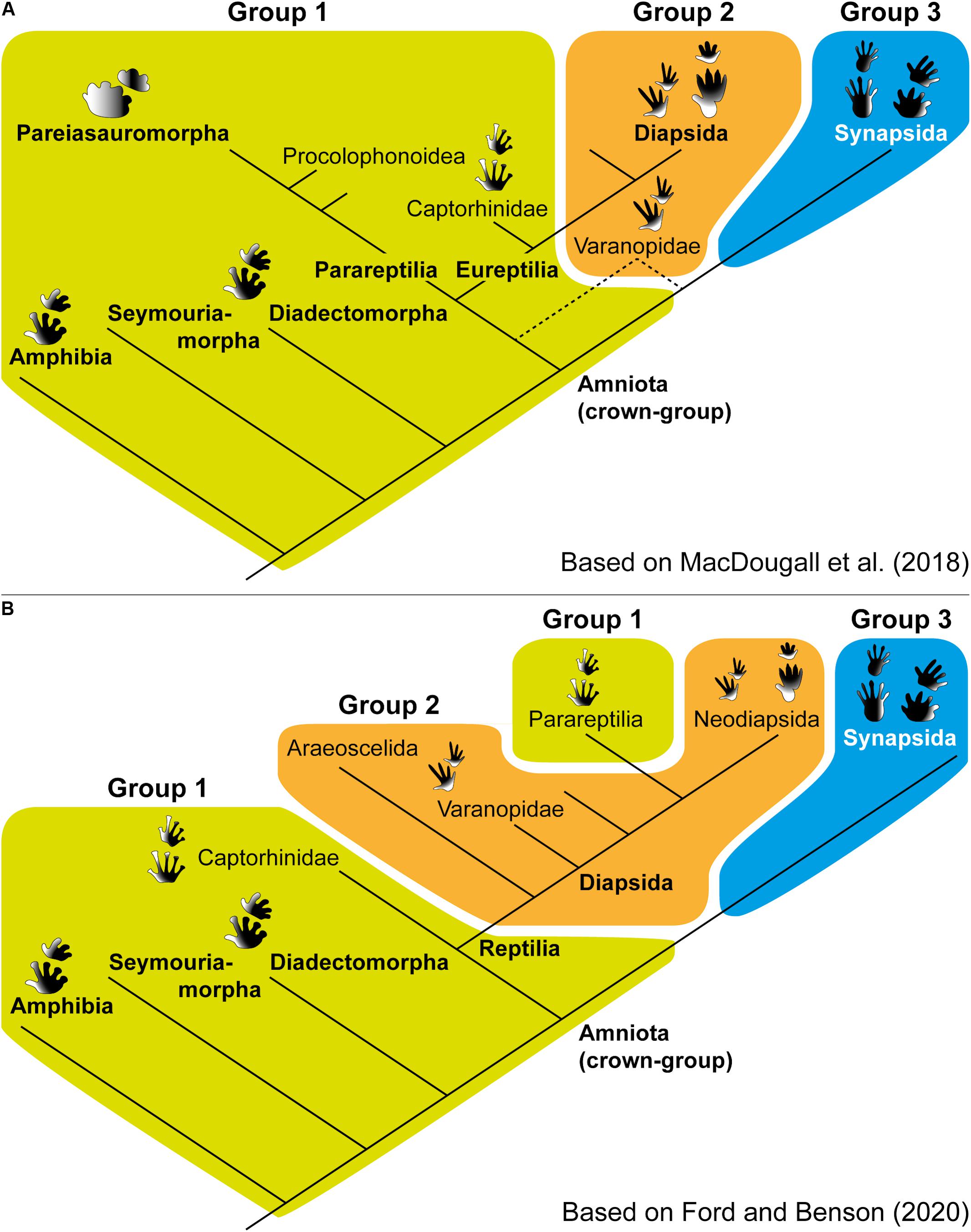
Figure 8. Simplified cladograms of tetrapods showing the relationship of the three footprint groups of relative depth patterns. (A) Tree based on MacDougall et al. (2018); note the dashed lines of varanopids: the line from the reptile lineage was recovered by these authors, the line from the synapsid lineage corresponds to the classical interpretation (here preferred; see text for discussion). (B) Tree based on Ford and Benson (2020); note the split of group 1, implying a less parsimonious chain of events.
Some recent studies nested the parareptiles within diapsids (Ford and Benson, 2020). This would imply a separation of group 1 in two sub-groups: (1) anamniotes, stem amniotes, and captorhinids and (2) parareptiles (Figure 8B). A possible explanation for this is a morphological reversal between group 2 (Diapsida) and group 1 (Parareptilia) to the ancestral depth pattern condition. However, this would represent a less parsimonious chain of events. All in all, based on available interpretations of early amniotes, we concur in general terms with the interpretation of MacDougall et al. (2018).
The present work is the first step to (1) create a general framework to analyze upper Paleozoic to Triassic tetrapod trace fossils and (2) evaluate how the three identified groups evolved through time and space. Of note, these analyses also have to consider the exceptions/differences observed within the groups, as well as differences between ecosystems (i.e., different paleoenvironments in which the footprints are preserved and the related biases, as not all the paleoenvironments are equally preserved in the fossil record). In addition, future studies of tetrapod tracks from any age interval and (ichno-) taxonomic group should also test this analysis. For instance, in a preliminary overview, we observed that dinosaur tracks from different lineages (see examples in Castanera et al., 2013; Salisbury et al., 2016; Lallensack et al., 2017) appear to fit with group 2, which encompasses diapsids. Furthermore, the identification of these groups, when possible, should be accompanied with additional data regarding the ichnotaxa considered, including general footprints’ morphologies, relative proportions, and trackway parameters. In fact, depth patterns are considered ichnotaxobases (e.g., Voigt, 2005; Marchetti et al., 2017a). Also, such classification must be based on a representative sample, avoiding assumptions or interpretations derived from the study of a small number of specimens.
Of interest, despite the different types of substrates (even for the same ichnotaxon) and the wide age range of each of the three identified groups, a substantially consistent relative depth pattern of tracks is observed within the groups (Figure 7). Indeed, all these groups cross major biotic crises, such as the end-Guadalupian and end-Permian mass extinctions. This would indicate that the functional prevalence of each group is preserved and inherited despite environmental and climatic (catastrophic) changes. Henceforth, evidences of such events may be found in broader context analyses, including quantitative studies of the functional prevalence of autopodia, encompassing a relatively wide stratigraphic range.
A further question to explore is the relationship of these groups with the postcranial skeleton record of the supposed producers, which may help to identify the anatomic and functional reasons of these depth patterns. Also, it should be checked if the herein proposed groups of footprints according to their functional prevalence keep a relationship and/or present a correlation with the morphofunctional categories of temporal skull fenestra in amniotes (Wernerburg, 2019; Ford and Benson, 2020). Indeed, in a general point of view, a similar distribution of high-rank taxonomic groups exists between these two different grouping.
Conclusion
Photogrammetry has been proved to be an extremely useful tool in the study of upper Paleozoic and Triassic tetrapod ichnofossils. Nevertheless, this technique has not been commonly applied in this ichnological record, especially when compared with dinosaur footprints. Through the present work, we review and highlight the benefits of using photogrammetry. Indeed, it is proved that 3D models can be key elements in ichnotaxonomy. Footprint relative depth patterns, when appropriately compared with other tracks and skeletal remains, refine the correlation of tracks with the potential trackmakers. False color depth maps also help to unravel the formation and superimposition of structures that can mask footprints. Such structures may result from both the locomotion of the same (or other) trackmakers and from sedimentary processes (e.g., ripples, collapse of the substrate in inclined surfaces), as well as from weathering of the footprints. Furthermore, 3D models are a suitable solution to preserve the ichnological record, especially when original specimens cannot be collected and thus are subjected to disappearance.
A review of the available 3D data of Permian and Triassic (and upper Carboniferous to a lesser degree) tetrapod footprints allowed us to preliminary identify three main groups of tracks linked to specific relative depth patterns derived from the functional prevalence of autopodia: (1) anamniote and captorhinomorph/parareptile tracks (medial-median functional prevalence); (2) diapsid tracks (median functional prevalence); and (3) synapsid tracks (median-lateral functional prevalence). This qualitative analysis shows a substantially consistent depth pattern within each group, despite being preserved in different substrates and corresponding to a wide stratigraphic interval. All in all, this indicates that 3D models may show features linked to the potential trackmakers that remain unseen by the naked eye.
Data Availability Statement
All datasets generated for this study are included in the article/Supplementary Material.
Author Contributions
JF, EM, LM, and RS contributed on the conception and design of the study and performed the ichnological analysis. EM and LM organized the database. EM wrote the first draft of the manuscript and prepared the figures, with comments from all authors. LM, RS, and JF wrote sections of the manuscript. All the authors contributed to manuscript revision, read, and approved the submitted version.
Conflict of Interest
The authors declare that the research was conducted in the absence of any commercial or financial relationships that could be construed as a potential conflict of interest.
Funding
EM received funding from the SYNTHESYS Project http://www.synthesys.info/ (FR-TAF-3621 and FR-TAF-4808) which is financed by European Community Research Infrastructure Action under the FP7 “Capacities” Program; Secretaria d’Universitats i Recerca (Departament d’Economia, Generalitat de Catalunya; grant number 2013 CTP 00013); the Erasmus+ program of the Universitat Autònoma de Barcelona. LM was funded by the Alexander von Humboldt Foundation. JF was supported by the Spanish Agencia Estatal de Investigación and the European Regional Development Fund of the European Union (AEI/FEDER EU, project CGL2017-82654-P). JF is member of the consolidated research group 2017 SGR 86 GRC of the Generalitat de Catalunya. EM and JF are members of the project “Evolució dels ecosistemes durant la transició Paleozoic–Mesozoic a Catalunya” (ref. CLT009/18/00066), financially supported by the Departament de Cultura (Generalitat de Catalunya). This research received support from the CERCA program (ICP) from the Generalitat de Catalunya.
Acknowledgments
We thank all curators and researchers for allowing access to specimens presented in this work, stored in their corresponding institutions, which include Muséum National d’Histoire Naturelle (Paris), Institut des Sciencies de l’Evolution de Montpellier (at Université de Montpellier, Montpellier), Musée Fleury (Lodève). We thank all the land owners who granted access to the specimens in situ. We especially thank Sergio Llácer (ICP) for the elaboration of several 3D models for this work. We also thank Rafel Matamales-Andreu (ICP) for the help in acquisition of photos and elaboration of 3D models from Erillcastell, and Jaume Balagué (Terra Dron) for the acquisition of aerial and close-range photos, and data processing from Erillcastell and Coll de Terrers. We acknowledge three reviewers who helped to substantially improve an earlier version of the manuscript, as well as Martin D. Ezcurra for editorial handling.
Supplementary Material
The Supplementary Material for this article can be found online at: https://www.frontiersin.org/articles/10.3389/feart.2020.00248/full#supplementary-material
DATA SHEET S1 | Reviewed localities.
Footnotes
References
Adams, T. L., Strganac, C., Polcyn, M. J., and Jacobs, L. L. (2010). High resolution three-dimensional laser-scanning of the type specimen of Eubrontes (?) glenrosensis Shuler, 1935, from the Comanchean (Lower Cretaceous) of Texas: implications for digital archiving and preservation. Palaeontol. Electron. 13, 1–11.
Avanzini, M., Neri, C., Nicosia, U., and Conti, M. A. (2008). A new Early Permian ichnocoenosis from the “Grupo vulcanico atesino” (Mt. Luco, Southern Alps, Italy). Stud. Trent. Sci. Natl. Acta Geol. 83, 231–236.
Baird, D. (1957). Triassic reptile footprint faunules from Mildford, New Jersey. Bull. Mus. Comp. Zool. 117, 449–520.
Bates, K. T., Manning, P. L., Margetts, L., and Sellers, W. I. (2010). Sensitivity analysis in evolutionary robotic simulation of bipedal dinosaur running. J. Vert. Paleontol. 30, 458–466. doi: 10.1080/02724630903409329
Bates, K. T., Manning, P. L., Vila, B., and Hodgetts, D. (2008a). Three-dimensional modelling and analysis of dinosaur trackways. Palaeontology 51, 999–1010. doi: 10.1111/j.1475-4983.2008.00789.x
Bates, K. T., Rarity, F., Manning, P. L., Hodgetts, D., Vila, B., Oms, O., et al. (2008b). High-resolution LiDAR and photogrammetric survey of the Fumanya dinosaur tracksites (Catalonia): implications for the conservation and interpretation of geological heritage sites. J. Geol. Soc. 165, 115–127. doi: 10.1144/0016-76492007-033
Belvedere, M., Bennett, M. R., Marty, D., Budka, M., Reynolds, S. C., and Bakirov, R. (2018). Stat-tracks and mediotypes: powerful tools for modern ichnology based on 3D models. PeerJ 6:e4247. doi: 10.7717/peerj.4247
Bertling, M., Braddy, S. J., Bromley, R. G., Demathieu, G. R., Genise, J., Mikuláš, R., et al. (2006). Names for trace fossils: a uniform approach. Lethaia 39, 265–286. doi: 10.1080/00241160600787890
Carrano, M. T., and Wilson, J. A. (2001). Taxon distributions and the tetrapod track record. Paleobiology 27, 564–582. doi: 10.1666/0094-8373(2001)027<0564:tdattt>2.0.co;2
Castanera, D., Pascual, C., Razzolini, N. L., Vila, B., Barco, J. L., and Canudo, J. I. (2013). Discriminating between medium-sized tridactyl trackmakers: tracking ornithopod tracks in the base of the Cretaceous (Berriasian. Spain). PLoS One 8:e81830. doi: 10.1371/journal.pone.0081830
Cavin, L., and Piuz, A. (2020). A several-kilometer-long archosaur route in the triassic of the swiss alps. Front. Earth Sci. 8:4. doi: 10.3389/feart.2020.00004
Cisneros, J. C., Day, M. O., Groenewald, J., and Rubidge, B. S. (2020). Small footprints expand middle Permian amphibian diversity in the South African Karoo. Palaios 35, 1–11. doi: 10.2110/palo.2018.098
Citton, P., Carluccio, R., Nicolosi, I., and Nicosia, U. (2019a). Re-evaluation of Chelichnus tazelwürmi, a non mammalian therapsid-grade track from the Upper Permian Arenaria di Val Gardena. Hist. Biol. 31, 322–340. doi: 10.1080/08912963.2017.1370586
Citton, P., Ronchi, A., Maganuco, S., Caratelli, M., Nicosia, U., Sacchi, E., et al. (2019b). First tetrapod footprints from the Permian of Sardinia and their palaeontological and stratigraphical significance. Geol. J. 54, 2084–2098. doi: 10.1002/gj.3285
Citton, P., Díaz-Martínez, I., de Valais, S., and Cónsole-Gonella, C. (2018). Triassic pentadactyl tracks from the Los Menucos Group (Río Negro province, Patagonia Argentina): possible constraints on the autopodial posture of Gondwanan trackmakers. PeerJ 6:e5358. doi: 10.7717/peerj.5358
Citton, P., Nicolosi, I., Carluccio, R., and Nicosia, U. (2016). Unveiling trampling history through trackway interferences and track preservational features: a case study from the Bletterbach gorge (Redagno, Western Dolomites, Italy). Palaeontol. Electron. 19.2. 20A, 1–20. doi: 10.26879/611
Citton, P., Romano, M., Carluccio, R., D’Ajello Caracciolo, F., Nicolosi, I., Nicosia, U., et al. (2017). The first dinosaur tracksite from Abruzzi (Monte Cagno, Central Apennines, Italy. Cretaceous Res. 73, 47–59. doi: 10.1016/j.cretres.2017.01.002
Citton, P., Ronchi, A., Nicosia, U., Sacchi, E., Maganuco, S., Cipriani, A., et al. (2020). Tetrapod tracks from the Middle Triassic of NW Sardinia (Nurra region. Italy). Italian J. Geosci. 139, 309–320. doi: 10.3301/ijg.2020.07
Curioni, G. (1870). Osservazioni geologiche sulla Val Trompia. Rend. Ist. Lomb. Sci. Lett. Arti Mem. 3, 1–60.
de Klerk, W. J. (2002). A dicynodont trackway from the Cistecephalus assemblage zone in the Karoo, east of Graaff-Reinet. South Africa. Palaeontol. Afr. 91, 73–91.
Demathieu, G. (1985). Trace fossil assemblages in middle Triassic marginal marine deposits, Eastern border of the Massif Central, France. SEPM Spec. Publ. 35, 53–66. doi: 10.2110/pec.85.35.0053
Demathieu, G., and Demathieu, P. (2004). Chirotheria and other ichnotaxa of the European Triassic. Ichnos 11, 79–88. doi: 10.1080/10420940490444898
Falcon-Lang, H. J., Gibling, M. R., Benton, M. J., Miller, R. F., and Bashforth, A. R. (2010). Diverse tetrapod trackways in the Lower Pennsylvanian Tynemouth Creek Formation, near St. Martins, southern New Brunswick, Canada. Palaeogeogr. Palaeoclimatol. Palaeoecol. 296, 1–13. doi: 10.1016/j.palaeo.2010.06.020
Falkingham, P. L. (2012). Acquisition of high resolution three-dimensional models using free, open-source, photogrammetric software. Palaeontol. Electron. 15.1. 1T, 1–15.
Falkingham, P. L. (2014). Interpreting ecology and behaviour from the vertebrate fossil track record. J. Zool. 292, 222–228. doi: 10.1111/jzo.12110
Falkingham, P. L., Bates, K. T., Avanzini, M., Bennett, M., Brody, E. M., Breithaupt, B. H., et al. (2018). A standard protocol for documenting modern and fossil ichnological data. Palaeontology 61, 469–480. doi: 10.1111/pala.12373
Falkingham, P. L., Bates, K. T., and Farlow, J. O. (2014). Historical photogrammetry: Bird’s Paluxy River dinosaur chase sequence digitally reconstructed as it was prior to excavation 70 years ago. PLoS One 9:e93247. doi: 10.1371/journal.pone.0093247
Falkingham, P. L., and Gatesy, S. M. (2014). The birth of a dinosaur footprint: subsurface 3D motion reconstruction and discrete element simulation reveal track ontogeny. Proc. Natl. Acad. Sci. U.S.A. 111, 18279–18284. doi: 10.1073/pnas.1416252111
Farman, R. M., and Bell, P. R. (2020). Australia’s earliest tetrapod swimming traces from the Hawkesbury Sandstone (Middle Triassic) of the Sydney Basin. J. Paleontol. 13, 1–13. doi: 10.1017/jpa.2020.22
Ford, D. P., and Benson, R. B. J. (2020). The phylogeny of early amniotes and the affinities of Parareptilia and Varanopidae. Nat. Ecol. Evol. 4, 57–65. doi: 10.1038/s41559-019-1047-3
Fornós, J. J., Bromley, R. G., Clemmensen, L. B., and Rodriguez-Perea, A. (2002). Tracks and trackways of Myotragusbalearicus Bate (Artiodactyla, Caprinae) in Pleistocene aeolianites from Mallorca (Balearic Islands, Western Mediterranean). Palaeogeogr. Palaeoclimatol. Palaeoecol. 180, 277–313. doi: 10.1016/s0031-0182(01)00431-x
Fortuny, J., Bolet, A., Sellés, A. G., Cartanyà, J., and Galobart, À (2011). New insights on the Permian and Triassic vertebrates from the Iberian Peninsula with emphasis on the Pyrenean and Catalonian basins. J. Iber. Geol. 37, 65–86. doi: 10.5209/rev_JIGE.2011.v37.n1.5
Francischini, H., Dentzien-Dias, P., Lucas, S. G., and Schultz, C. L. (2018). Tetrapod tracks in Permo–Triassic eolian beds of southern Brazil (Paraná Basin). PeerJ 6:e4764. doi: 10.7717/peerj.4764
Francischini, H., Lucas, S. G., Voigt, S., Marchetti, L., Santucci, V. L., Knight, C. L., et al. (2020). On the presence of Ichniotherium in the Coconino Sandstone (Cisuralian) of the Grand Canyon and remarks on the occupation of deserts by non-amniote tetrapods. Pal. Z. 94, 207–225. doi: 10.1007/s12542-019-00450-5
Gand, G. (1987). Les traces de Vertébrés tétrapodes du Permien français (paléontologie, stratigraphie, paléoenvironnements). Dissertation thesis, Université de Bourgogne, Dijon.
Gand, G., Demathieu, G., and Montenat, C. (2007). Les traces de pas d’amphibiens, de dinosaures et autres reptiles du Mésozoïque français: inventaire et interprétations. Palaeovertebrata 1- 4, 1–149.
Gand, G., and Durand, M. (2006). “Tetrapod footprint ichno-associations from French Permian basins. Comparisons with other Euramerican ichnofaunas,” in Non-Marine Permian Biostratigraphy and Biochronology, eds S. G. Lucas, G. Cassinis, and J. W. Schneider (London: Geological Society of London Special Publication 265), 157–177. doi: 10.1144/gsl.sp.2006.265.01.07
Gand, G., Garric, J., Demathieu, G., and Ellenberger, P. (2000). La palichnofaune de vertébrés tétrapodes du Permien supérieur du Bassin de Lodève (Languedoc-France). Palaeovertebrata 29, 1–82.
Gatesy, S. M., and Falkingham, P. L. (2017). Neither bones nor feet: track morphological variation and ‘preservation quality’. J. Vert. Paleontol. 37:e1314298. doi: 10.1080/02724634.2017.1314298
Gatesy, S. M., Middleton, K. M., Jenkins, F. A. Jr., and Shubin, N. H. (1999). Three-dimensional preservation of foot movements in Triassic theropod dinosaurs. Nature 399, 141–144. doi: 10.1038/20167
Geinitz, H. B. (1861). Dyas oder die Zechsteinformation und des Rotliegende. Die Animalischen ueberreste der Dyas, vol. I. Leipzig: William Engelmann.
Geinitz, H. B. (1863). Beiträge zur Kenntnis der organischen Überreste in der Dyas. N. Jb. Min. Geol. Paläont. 1863, 385–398.
Geinitz, H. B., and Deichmüller, J. V. (1882). Die Saurier der unteren Dyas von Sachsen. Palaeontographica 29, 1–4.
Gilmore, C. W. (1927). Fossil footprints from the Grand Canyon. II. Smithson. Miscell. Coll. 80, 1–78.
Gisbert, J. (1981). Estudio geológico-petrológico del Estefaniense-Pérmico de la Sierra del Cadí (Pirineo de Lérida): Diagénesis y sedimentología. Dissertation thesis, Universdad de Zaragoza, Zaragoza.
Haubold, H. (1970). Versuch der Revision der Amphibien-Fährten des Karbon und Perm. Freib. Forsch. C 260, 83–117.
Haubold, H. (1971a). Die Tetrapodenfährten des Buntsandsteins in der Deutschen Demokratischen Republik und in Westdeutschland und ihre Äquivalente in der gesamten Trias. Paläntologische Abhandlungen A 4, 395–548.
Haubold, H. (1971b). Encyclopedia of Palaeoherpetology, Part 18: Ichnia amphibiorum et Reptiliorum fossilium. Stuttgart: Gustav Fischer Verlag, 1–124.
Haubold, H. (1996). Ichnotaxonomie und Klassifikation von Tetrapodenfährten aus dem Perm. Hallesch. Jahr. Geowiss. B 18, 23–88.
Haubold, H., Hunt, A. P., Lucas, S. G., and Lockley, M. G. (1995a). Wolfcampian (early Permian) vertebrate tracksfrom Arizona and New Mexico. N. M. Mus. Nat. Hist. Sci. Bull. 6, 135–165.
Haubold, H., Lockley, M. G., Hunt, A. P., and Lucas, S. G. (1995b). Lacertoid footprints from Permian dune sandstones, Cornberg and De Chelly Sandstones. N. M. Mus. Nat. Hist. Sci. Bull. 6, 235–244.
Heyler, D., and Gand, G. (2000). Pistes et traces du Permien du sud de la France dans les collections du Muséum National d’Histoire Naturelle (Paris), première partie: les bassin de Lodève. Bull. Soc. Hist. Nat. Autun. 176, 7–50.
Heyler, D., and Lessertisseur, J. (1963). Piste de tétrapodes permiens dans la région de Lodève (Hérault). Mém. Mus. Natl. Hist. Nat. Série C Sci. de la Terre 11, 125–221.
Hunt, A. P., and Lucas, S. G. (2005). “Tetrapod Ichnofacies and their utility in the Paleozoic,” in Pennsylvanian Footprints in the Black Warrior Basin of Alabama, eds R. J. Buta, A. K. Rindsberg, and D. Kopaska-Merkel (Alabama: Alabama Paleontological Society), 113–119.
International Commission on Zoological Nomenclature (1999). International Code of Zoological Nomenclature, 4th Edn. London: International Trust for Zoological Nomenclature.
Klein, H., and Lucas, S. G. (2010). Review of the tetrapod ichnofauna of the Moenkopi Formation/Group (Early–Middle Triassic) of the American Southwest. N. M. Mus. Nat. Hist. Sci. Bull. 50, 1–167.
Klein, H., Lucas, S. G., and Voigt, S. (2015). Revision of the ?Permian-Triassic tetrapod ichnogenus Procolophonichnium Nopcsa, 1923 with description of the new ichnospecies P. lockleyi. Ichnos 22, 155–176. doi: 10.1080/10420940.2015.1063490
Krapovickas, V., Marsicano, C. A., Mancuso, A. C., de la Fuente, M. S., and Ottone, E. G. (2015). Tetrapod and invertebrate trace fossils from aeolian deposits of the lower Permian of central-western Argentina. Hist. Biol. 27, 827–842. doi: 10.1080/08912963.2014.904857
Lagnaoui, A., Melchor, R. N., Bellosi, E. S., Villegas, P. M., Espinoza, N., and Umazano, A. M. (2019). Middle Triassic Pentasauropus-dominated ichnofauna from western Gondwana: Ichnotaxonomy, palaeoenvironment, biostratigraphy and palaeobiogeography. Palaeoegeogr. Palaeoclim. Palaeoecol. 524, 41–61. doi: 10.1016/j.palaeo.2019.03.020
Lallensack, J. N. (2019). Automatic generation of objective footprint outlines. PeerJ 7:e7203. doi: 10.7717/peerj.7203
Lallensack, J. N., Klein, H., Milàn, J., Wings, O., Mateus, O., and Clemmensen, L. B. (2017). Sauropodomorph dinosaur trackways from the Fleming Fjord Formation of East Greenland: evidence for Late Triassic sauropods. Acta Palaeontol. Pol. 62, 833–843.
Lallensack, J. N., Sander, P. M., Knötschke, N., and Wings, O. (2015). Dinosaur tracks from the Langenberg Quarry (Late Jurassic, Germany) reconstructed with historical photogrammetry: evidence for large theropods soon after insular dwarfism. Palaeontol. Electron. 18, 1–34.
Laurin, M., and Piñeiro, G. H. (2017). A reassessment of the taxonomic position of Mesosaurs, and a surprising phylogeny or early Amniotes. Front. Earth Sci. 5:88. doi: 10.3389/feart.2017.00088
Laurin, M., and Piñeiro, G. H. (2018). Response: commentary: a reassessment of the taxonomic position of Mesosaurs, and a surprising phylogeny or early Amniotes. Front. Earth Sci. 6:200. doi: 10.3389/feart.2018.00220
Leonardi, G. (1987). Glossary and Manual of Tetrapod Palaeoichnology. Brasilia: Departamento Nacional da Produção Mineral.
Leonardi, G., and Mietto, P. (2000). Dinosauri in Italia: Le orme giurassiche dei Lavini di Marco (Trentino) e gli altri resti fossili italiani. Pisa-Roma: Accademia Editoriale.
Lockley, M. G., Cawthra, H. C., De Vynck, J. C., Helm, C. W., McCrea, R. T., and Nel, R. (2019). New fossil sea turtle trackway morphotypes from the Pleistocene of South Africa highlight role of ichnology in turtle paleobiology. Q. Res. 92, 626–640. doi: 10.1017/qua.2019.40
Loope, D. B. (2006). Dry-season tracks in dinosaur-triggered grainflows. Palaios 21, 132–142. doi: 10.2110/palo.2005.p05-55
Lucas, S. G. (2019). An ichnological perspective on some major events of Paleozoic tetrapod evolution. Boll. Soc. Paleont. It. 58, 223–266.
Lucas, S. G., and Harris, J. D. (2020). The “plastotype problem” in ichnological taxonomy. Ichnos 27, 107–110. doi: 10.1080/10420940.2019.1688802
MacDougall, M. J., Modesto, S. P., Brocklehurst, N., Verrière, A., Reisz, R. R., and Fröbisch, J. (2018). Response: a reassessment of the taxonomic position of mesosaurs, and a surprising phylogeny of early Amniotes. Front. Earth Sci. 6:99. doi: 10.3389/feart.2018.00099
Maidwell, F. T. (1911). Notes on footprints from the Keuper of Runcorn Hill. Proc. Liverpool Geol. Soc. 11, 140–152.
Mallison, H., and Wings, O. (2014). Photogrammetry in paleontology – A practical guide. J. Paleontol. Tech. 12, 1–31.
Mancuso, A. C., Krapovickas, V., Marsicano, C., Benavente, C., Benedito, D., de la Fuente, M., et al. (2016). Tetrapod tracks taphonomy in eolian facies from the Permian of Argentina. Palaios 31, 374–388. doi: 10.2110/palo.2015.077
Marchetti, L. (2016). New occurrences of tetrapod ichnotaxa from the Permian Orobic Basin (Northern Italy) and critical discussion of the age of the ichnoassociation. Papers Palaeontol. 2, 363–386. doi: 10.1002/spp2.1045
Marchetti, L. (2019). Can undertracks show higher morphologic quality than surface tracks? Remarks on large amphibian tracks from the Early Permian of France. J. Iber. Geol. 45, 353–363. doi: 10.1007/s41513-018-0080-4
Marchetti, L., Belvedere, M., Voigt, S., Klein, H., Castanera, D., Díaz-Martínez, I., et al. (2019a). Defining the morphological quality of fossil footprints. Problems and principles of preservation in tetrapod ichnology with examples from the Palaeozoic to the present. Earth Sci. Rev. 193, 109–145. doi: 10.1016/j.earscirev.2019.04.008
Marchetti, L., Klein, H., Buchwitz, M., Ronchi, A., Smith, R. M., De Klerk, W. J., et al. (2019d). Permian-Triassic vertebrate footprints from South Africa: ichnotaxonomy, producers and biostratigraphy through two major faunal crises. Gondw. Res. 72, 139–168. doi: 10.1016/j.gr.2019.03.009
Marchetti, L., Voigt, S., and Lucas, S. G. (2019c). An anatomy-based study of the Lopingian eolian tracks of Germany and Scotland reveals the first evidence of the end-Guadalupian mass extinction at low paleolatitudes of Pangea. Gondw. Res. 73, 32–53. doi: 10.1016/j.gr.2019.03.013
Marchetti, L., Voigt, S., Lucas, S. G., Francischini, H., Dentzien-Dias, P., Sacchi, R., et al. (2019b). Tetrapod ichnotaxonomy in eolian paleoenvironments (Coconino and De Chelly formations, Arizona) and late Cisuralian (Permian) sauropsid radiation. Earth Sci. Rev. 190, 148–170. doi: 10.1016/j.earscirev.2018.12.011
Marchetti, L., Bernardi, M., and Avanzini, M. (2013). Some insights on well-preserved Amphisauropus and Erpetopus trackways from the Eastern Collio Basin (Trentino-Alto Adige NE Italy). Boll. Soc. Paleont. It. 52, 55–62.
Marchetti, L., Mujal, E., and Bernardi, M. (2017a). An unusual Amphisauropus trackway and its implication for understanding seymouriamorph locomotion. Lethaia 50, 162–174. doi: 10.1111/let.12184
Marchetti, L., Belvedere, M., and Mietto, P. (2017b). Lopingian tetrapod footprints from the Venetian Prealps, Italy: new discoveries in a largely incomplete panorama. Acta Palaeontol. Pol. 62, 801–817.
Marchetti, L., Petti, F. M., Bernardi, M., Citton, P., Rossi, R., and Schirolli, P. (2018a). On the first description of tetrapod footprints from Italy: re-analysis of the original specimen after 150 years. Rend. Online Soc. Geol. It. 44, 112–118. doi: 10.3301/rol.2018.16
Marchetti, L., Voigt, S., and Santi, G. (2018b). A rare occurrence of Permian tetrapod footprints: Ichniotherium cottae and Ichniotherium sphaerodactylum on the same stratigraphic surface. Ichnos 25, 106–118. doi: 10.1080/10420940.2017.1380005
Marchetti, L., Ronchi, A., Santi, G., and Voigt, S. (2015). The Gerola Valley site (Orobic Basin, Northern Italy): a key for understanding late Early Permian tetrapod ichnofaunas. Palaeoegeogr. Palaeoclim. Palaeoecol. 439, 97–116. doi: 10.1016/j.palaeo.2015.02.032
Marsh, O. C. (1894). Footprints of vertebrates in the Coal Measures of Kansas. Am. J. Sci. Ser. 3, 81–84. doi: 10.2475/ajs.s3-48.283.81
Marty, D., Strasser, A., and Meyer, C. A. (2009). Formation and taphonomy of human footprints in microbial mats of present-day tidal-flat environments: implications for the study of fossil footprints. Ichnos 16, 127–142. doi: 10.1080/10420940802471027
Matthews, N. A. (2008). Aerial and Close-Range Photogrammetric Technology: Providing Resource Documentation, Interpretation, and Preservation. Technical Note 428. Denver: U.S. Department of the Interior, Bureau of Land Management, National Operations Center.
McKeever, P. J., and Haubold, H. (1996). Reclassification of vertebrate trackways from the Permian of Scotland and related forms from Arizona and Germany. J. Paleontol. 70, 1011–1022. doi: 10.1017/s0022336000038713
Meade, L. E., Jones, A. S., and Butler, R. J. (2016). A revision of tetrapod footprints from the late Carboniferous of the West Midlands. UK. PeerJ 4:e2718. doi: 10.7717/peerj.2718
Michel, L. A., Tabor, N. J., Montañez, I. P., Schmitz, M. D., and Davydov, V. I. (2015). Chronostratigraphy and Paleoclimatology of the Lodève Basin, France: evidence for a pan-tropical aridification event across the Carboniferous–Permian boundary. Palaeoegeogr. Palaeoclim. Palaeoecol. 430, 118–131. doi: 10.1016/j.palaeo.2015.03.020
Milàn, J., Klein, H., Voigt, S., and Stemmerik, L. (2016). First record of tetrapod footprints from the Carboniferous Mesters Vig Formation in East Greenland. Bull. Geol. Soc. Denmark 64, 69–76.
Moodie, R. L. (1929). Vertebrate footprints from the red beds of Texas. J. Geol. 38, 548–565. doi: 10.1086/623755
Moodie, R. L. (1930). Vertebrate footprints from the Red Beds of Texas II. Amer. J. Sci. 97, 352–368. doi: 10.2475/ajs.s5-17.100.352
Mujal, E. (2017). Registre sedimentari i icnològic del fini-Carbonífer, Permià i Triàsic continentals dels Pirineus Catalans: Evolució i crisis paleoambientals a l’equador de Pangea. Dissertation thesis, Universitat Autònoma de Barcelona, Cerdanyola del Vallès.
Mujal, E., Fortuny, J., Bolet, A., Oms, O., and López, J. Á (2017a). An archosauromorph dominated ichnoassemblage in fluvial settings from the late early Triassic of the Catalan Pyrenees (NE Iberian Peninsula). PLoS one 12:e0174693. doi: 10.1371/journal.pone.0174693
Mujal, E., Fortuny, J., Pérez-Cano, J., Dinarès-Turell, J., Ibáñez-Insa, J., Oms, O., et al. (2017b). Integrated multi-stratigraphic study of the Coll de Terrers late Permian–early Triassic continental succession from the Catalan Pyrenees (NE Iberian Peninsula): a geologic reference record for equatorial Pangaea. Glob. Planet. Chang. 159, 46–60. doi: 10.1016/j.gloplacha.2017.10.004
Mujal, E., Gretter, N., Ronchi, A., López-Gómez, J., Falconnet, J., Diez, J. B., et al. (2016b). Constraining the Permian/Triassic transition in continental environments: stratigraphic and paleontological record from the Catalan Pyrenees (NE Iberian Peninsula). Palaeogeogr. Palaeoclimatol. Palaeoecol. 445, 18–37. doi: 10.1016/j.palaeo.2015.12.008
Mujal, E., Fortuny, J., Oms, O., Bolet, A., Galobart, À, and Anadón, P. (2016a). Palaeoenvironmental reconstruction and early Permian ichnoassemblage from the NE Iberian Peninsula (Pyrenean Basin). Geol. Mag. 153, 578–600. doi: 10.1017/s0016756815000576
Mujal, E., Fortuny, J., Rodríguez-Salgado, P., Diviu, M., Oms, O., and Galobart, À (2015). First footprints occurrence from the Muschelkalk detritical unit of the Catalan Basin: 3D analyses and palaeoichnological implications. Span. J. Palaeontol. 30, 97–108.
Mujal, E., Fortuny, J., Marmi, J., Dinarès-Turell, J., Bolet, A., and Oms, O. (2018b). Aridification across the Carboniferous–Permian transition in central equatorial Pangea: the Catalan Pyrenean succession (NE Iberian Peninsula). Sediment. Geol. 363, 48–68. doi: 10.1016/j.sedgeo.2017.11.005
Mujal, E., Iglesias, G., Oms, O., Fortuny, J., Bolet, A., and Méndez, J. M. (2018a). Rhynchosauroides footprint variability in a Muschelkalk detrital interval (late Anisian–middle Ladinian) from the Catalan Basin (NE Iberian Peninsula). Ichnos 25, 150–161. doi: 10.1080/10420940.2017.1337571
Mujal, E., and Marchetti, L. (2020). Ichniotherium tracks from the Permian of France, and their implications for understanding the locomotion and palaeobiogeography of large diadectomorphs. Palaeogeogr. Palaeoclimatol. Palaeoecol. 547:109698. doi: 10.1016/j.palaeo.2020.109698
Mujal, E., and Schoch, R. R. (2020). Middle Triassic (Ladinian) amphibian tracks from the Lower Keuper succession of southern Germany: implications for temnospondyl locomotion and track preservation. Palaeogeogr. Palaeoclimatol. Palaeoecol. 543:109625. doi: 10.1016/j.palaeo.2020.109625
Olsen, P. E. (1995). A new approach for recognizing track makers. Geol. Soc. Am. Abst. Progr. 27:72.
Pabst, W. (1895). Thierfährten aus dem Rothliegenden von Friedrichroda, Tambach und Kabarz in Thüringen. Z. Dtsch. geol. Ges. 47, 570–576.
Pabst, W. (1908). Die Tierfährten in dem Rotliegenden, Deutschlands’. Nova Acta Leopold 89, 315–481.
Peabody, F. E. (1948). Reptile and amphibian trackways from the Moenkopi Formation of Arizona and Utah. Univ. California Publ. Bull. Dept. Geol. Sci. 27, 295–468.
Petti, F. M., Avanzini, M., Belvedere, M., De Gasperi, M., Ferretti, P., Girardi, S., et al. (2008). Digital 3D modelling of dinosaur footprints by photogrammetry and laser scanning techniques: integrated approach at the Coste dell’Anglone tracksite (Lower Jurassic, Southern Alps, Northern Italy). Stud. Trent. Sci. Nat., Acta Geol. 83, 303–315.
Petti, F. M., Avanzini, M., Nicosia, U., Girardi, S., Bernardi, M., Ferretti, P., et al. (2009). Late Triassic (early-middle Carnian) chirotherian tracks from the Val Sabbia Sandstone (eastern Lombardy, Brescian Prealps, northern Italy). Riv. It. Paleont. Strat. 115, 277–290.
Petti, F. M., Petruzzelli, M., Conti, J., Spalluto, L., Conti, J., Wagensommer, A., et al. (2018). The use of aerial and close-range photogrammetry in the study of dinosaur tracksites: lower Cretaceous (upper Aptian/lower Albia) Molfetta ichnosite (Apulia, southern Italy). Palaeontol. Electron. 21, 1–18.
Razzolini, N. L., Vila, B., Castanera, D., Falkingham, P. L., Barco, J. L., Canudo, J. I., et al. (2014). Intra-trackway morphological variations due to substrate consistency: the El Frontal dinosaur tracksite (lower Cretaceous Spain). PLoS One 9:e93708. doi: 10.1371/journal.pone.0093708
Reisz, R. R., Modesto, S. P., and Scott, D. M. (2011). A new Early Permian reptile and its significance in early diapsid evolution. Proc. R. Soc. B 278, 3731–3737. doi: 10.1098/rspb.2011.0439
Remondino, F., Rizzi, A., Girardi, S., Petti, F. M., and Avanzini, M. (2010). 3D ichnology – Recovering digital 3D models of dinosaur footprints. Photogrammetr. Record 25, 266–282. doi: 10.1111/j.1477-9730.2010.00587.x
Reolid, J., Cardenal, F. J., Reolid, M., and Mata, E. (2020). 3D Imaging of southernmost Triassic archosaur footprints from Europe (Southern Spain) J. Iberian Geol. 46, 145–161. doi: 10.1007/s41513-020-00125-0
Romilio, A., Hacker, J. M., Zlot, R., Poropat, G., Bosse, M., and Salisbury, S. W. (2017). A multidisciplinary approach to digital mapping of dinosaurian tracksites in the Lower Cretaceous (Valanginian–Barremian) Broome Sandstone of the Dampier Peninsula. Western Australia. PeerJ 5:e3013. doi: 10.7717/peerj.3013
Salisbury, S. W., Romilio, A., Herne, M. C., Tucker, R. T., and Nair, J. P. (2016). The dinosaurian ichnofauna of the Lower Cretaceous (Valanginian–Barremian) Broome Sandstone of the Walmadany Area (James Price Point), Dampier Peninsula, Western Australia. J. Vert. Paleontol. 36, 1–152. doi: 10.1080/02724634.2016.1269539
Schmidt, H. (1959). Die Cornberger Fährten im Rahmen der Vierfüßler-Entwicklung. Abh. Hess. Landesamt Bodenforsch. 28, 1–137.
Schoch, R. R., and Seegis, D. (2016). A Middle Triassic palaeontological gold mine: the vertebrate deposits of Vellberg (Germany). Palaeogeogr. Palaeoclimatol. Palaeoecol. 459, 249–267. doi: 10.1016/j.palaeo.2016.07.002
Smith, R. M. H., and Botha-Brink, J. (2014). Anatomy of a mass extinction: sedimentological and taphonomic evidence for drought-induced die-offs at the Permo-Triassic boundary in the main Karoo Basin, South Africa. Palaeogeogr. Palaeoclimatol. Palaeoecol. 396, 99–118. doi: 10.1016/j.palaeo.2014.01.002
Thomson, T. J., and Droser, M. L. (2015). Swimming reptiles make their mark in the Early Triassic: delayed ecologic recovery increased the preservation potential of vertebrate swim tracks. Geology 43, 215–218. doi: 10.1130/g36332.1
Vila, B., Castanera, D., Marmi, J., Canudo, J. I, and Galobart, À (2015). Crocodile swim tracks from the latest Cretaceous of Europe. Lethaia 48, 256–266. doi: 10.1111/let.12103
Voigt, S. (2005). Die Tetrapoden ichnofauna des kontinentalen Oberkarbon und Perm im Thüringer Wald - Ichnotaxonomie, Paläoökologie und Biostratigraphie. Dissertation thesis, Martin-Luther-Universität Halle-Wittenberg, Göttingen.
Voigt, S., Berman, D. S., and Henrici, A. C. (2007). First well-established track-trackmaker association of Paleozoic tetrapods based on Ichniotherium trackways and diadectid skeletons from the Lower Permian of Germany. J. Vert. Paleontol. 27, 553–570. doi: 10.1671/0272-4634(2007)27[553:fwtaop]2.0.co;2
Voigt, S., and Haubold, H. (2015). Permian tetrapod footprints from the Spanish Pyrenees. Palaeogeogr. Palaeoclimatol. Palaeoecol. 417, 112–120. doi: 10.1016/j.palaeo.2014.10.038
Keywords: photogrammetry, tetrapod ichnology, ichnotaxonomy, locomotion, preservation, Permian, Triassic
Citation: Mujal E, Marchetti L, Schoch RR and Fortuny J (2020) Upper Paleozoic to Lower Mesozoic Tetrapod Ichnology Revisited: Photogrammetry and Relative Depth Pattern Inferences on Functional Prevalence of Autopodia. Front. Earth Sci. 8:248. doi: 10.3389/feart.2020.00248
Received: 20 December 2019; Accepted: 05 June 2020;
Published: 24 July 2020.
Edited by:
Martin Daniel Ezcurra, Museo Argentino de Ciencias Naturales Bernardino Rivadavia, ArgentinaReviewed by:
Silvina De Valais, CONICET Research Institute in Paleobiology and Geology (IIPG), ArgentinaSteven W. Salisbury, The University of Queensland, Australia
Verónica Krapovickas, Consejo Nacional de Investigaciones Científicas y Técnicas (CONICET), Argentina
Copyright © 2020 Mujal, Marchetti, Schoch and Fortuny. This is an open-access article distributed under the terms of the Creative Commons Attribution License (CC BY). The use, distribution or reproduction in other forums is permitted, provided the original author(s) and the copyright owner(s) are credited and that the original publication in this journal is cited, in accordance with accepted academic practice. No use, distribution or reproduction is permitted which does not comply with these terms.
*Correspondence: Josep Fortuny, josep.fortuny@icp.cat
†ORCID: Eudald Mujal, orcid.org/0000-0002-6310-323X; Lorenzo Marchetti, orcid.org/0000-0002-1047-7887; Rainer R. Schoch, orcid.org/0000-0002-0312-2877; Josep Fortuny, orcid.org/0000-0003-4282-1619