- 1Department of Geography and Environmental Management, University of Waterloo, Waterloo, ON, Canada
- 2Department of Ecology and Evolutionary Biology, Cornell University, Ithaca, NY, United States
- 3Department of Renewable Resources, University of Alberta, Edmonton, AB, Canada
Industrial activities for resource extraction have led to a network of seismic lines across Canada’s boreal regions where peatlands often make up over 50% of the landscape. These clearings can have a significant influence on ecosystem functioning through vegetation removal, flattening of microtopography, altering hydrological pathways, and impacting biogeochemical processes. Recently, there has been a concerted effort to restore seismic lines to bring back the localized microtopography and encourage ecosystem recovery. A common restoration approach on seismic lines is mounding, which involves using machinery to recreate natural microtopography. Research is scarce on the impact on soil properties following both these disturbances and subsequent restoration on organic soils. The objectives of this study were to: (1) identify differences in soil physical and chemical characteristics between areas disturbed by seismic lines and adjacent natural areas and (2) determine changes to soil physical and chemical properties following the mounding restoration technique. Research was undertaken at two contrasting boreal ecosites (a poor mesic and a treed fen) near Fort McMurray, Alberta, Canada. In July 2018, we collected soil samples at 34 seismic line locations, both on the line and 20 m into the adjacent undisturbed area. Samples were analyzed for bulk density, volumetric water content (VWC), organic matter (OM) content, C:N ratios, and δ13C/δ15N isotope analysis. Seismic line disturbances had a significant impact on soil properties, with increased bulk density and VWC on the line at both ecosites. We found an almost 40% reduction in OM on the line compared to natural areas at the poor mesic site, implying changes to carbon cycling, increased mineralization rates, and carbon loss from the system. There was also δ13C/δ15N enrichment and narrower C:N ratios on the line, indicating increased decomposition. We found evidence of increased decomposition on the mounds created after restoration at the treed fen. Our results highlight a trade-off between restoration activities that may encourage recovery but also cause increased carbon losses from the system. This research is a first step in gaining a better understanding of these impacts in light of current restoration practices to ensure best management practices for improving ecosystem functioning.
Introduction
Industrial activities for resource extraction have led to an extensive network of exploration lines, known as seismic lines, across Canada’s boreal regions (Pasher et al., 2013; Dabros et al., 2018). The construction of these clearings involves vegetation removal and soil compaction, resulting in a network of linear disturbances that have the potential to impair forest cover (Filicetti et al., 2019), alter predator-prey dynamics (DeMars and Boutin, 2018), and increase greenhouse gas emissions (Strack et al., 2019). There is currently no mandate in Canada to restore these seismic line disturbances. According to standard practice in Canada, seismic lines were left for natural regeneration and although some natural tree regeneration does occur (Lieffers et al., 2017), most sites have limited recovery (van Rensen et al., 2015), even after decades (Lee and Boutin, 2006) although this can be dependent on ecosite type. Negative impacts of seismic lines on ecosystem structure, function, and lack of recovery have led to a decline in at-risk species such as woodland caribou (Rangifer tarandus caribou) (Dyer et al., 2001, 2002; Filicetti et al., 2019), leading to an increasing interest in restoring inactive seismic lines. In order to address the apparent ecological issues associated with failure to return to forest cover, the energy industry has voluntarily chosen to begin to actively restore seismic lines using a range of techniques including mechanical site preparation, tree planting, and recruitment of coarse woody material. Mounding treatments are a type of mechanical site preparation used to artificially recreate microtopography (Dabros et al., 2018; Filicetti et al., 2019) which is a key functional component of boreal ecosystems. Although application to treed peatlands is not well documented, mechanical site preparation and mounding specifically has a long history in industrial silviculture (Forest Resource Development Agreement [FRDA], 1989). Although it is well established that tree regeneration is largely dependent on soil conditions (Lieffers et al., 2017; Henneb et al., 2019), effects of seismic line disturbance and mounding treatments on organic soil physical and chemical properties are poorly understood. Here, we address this knowledge gap by assessing the effects of these disturbances and restoration techniques on soil physical and chemical properties.
There are two categories of seismic lines; legacy and low impact. Legacy lines (hereinafter referred to as wide lines) are typically 5–10 m wide, are straight and cleared using heavy machinery. Comparatively, low-impact lines (hereinafter referred to as narrow lines) are narrower (∼1–5 m), meandering, and cleared with a goal to limit ground disturbance (Lee and Boutin, 2006). Both of these disturbances result in the removal of vegetation (Filicetti et al., 2019), simplification of microtopography (Stevenson et al., 2019), alterations in microclimate (Stern et al., 2018), and hydrology (Williams et al., 2013; Braverman and Quinton, 2016), as well as changes in biogeochemical processes and soil characteristics (Lee and Boutin, 2006; Strack et al., 2019). Hydrological changes include altered and impeded surface and subsurface water flow (Braverman and Quinton, 2016). Increased compaction can decrease the saturated hydraulic conductivity of peat by 75% (Price et al., 2003). Decreased evapotranspiration can also occur due to the removal of the woody vegetation layer (Lee and Boutin, 2006). The flattening of the typical microtopography (hummocks; Stevenson et al., 2019) and compaction of the soil (Lovitt et al., 2018) leads to changes in soil morphology (Startsev and McNabb, 2009) and a reduction in microsites needed for successful tree germination (Lee and Boutin, 2006).
The compaction of the surface layer during disturbance can be measured by an increase in the bulk density of the soil. As organic particle and pore size decrease with increased decomposition (Boelter, 1969), bulk density can also be a useful indicator of soil decomposition extent. Disturbances can increase rates of mineralization and carbon (C) and nitrogen (N) cycling processes (Walbridge and Lockaby, 1994; Westbrook et al., 2006). C:N ratios have often been used to investigate the amount of soil decomposition (Kuhry and Vitt, 1996). Assuming that organic materials are consistent at the beginning and that volatile loss of N is negligible, this ratio is a measure of mass loss caused by mineralization based on the consumption of C-rich substances in relation to N (Damman, 1988; Drollinger et al., 2019) and subsequent release of carbon dioxide (CO2) or methane (CH4) through microbial respiration (Malmer and Holm, 1984). Disturbances such as these can also cause changes in the isotopic composition of the soil, including enrichment of both δ13C and δ15N. δ13C in plant litter and soil can be used to differentiate between isotopic effects in the early stages of decomposition (Nadelhoffer and Fry, 1988; Connin et al., 2001). δ15N can give us an insight into changes in N content within both the plant litter and soil layers (Asada et al., 2005; Goud and Sparks, 2018) and can also be used as an indicator of decomposition extent (Amundson et al., 2003). Nutrient availability may change post-disturbance, linked to warmer, more aerobic, or wetter conditions altering microbial activity that may ultimately change net mineralization rates (Bradford et al., 2008; Li et al., 2012).
Seismic lines can also cause a significant shift in vegetation community composition, altering competitive relationships and making it difficult for trees to recolonize. Dense graminoid cover, especially prevalent after disturbance can shade tree seedlings, whereas dense Sphagnum spp. communities can bury small seedlings (Roy et al., 2000; Camill et al., 2010). Furthermore, changes in vegetation community composition and litter inputs can alter microbial communities, changing microbial cycling and potentially enhancing mineralization or overall decomposition extent (Scheffer et al., 2001; Allison and Treseder, 2011).
In order to restore seismic lines and accelerate the recovery of these lines (including regeneration of the tree cover), mounding treatments are used (Dabros et al., 2018; Filicetti et al., 2019). This involved creation of artificial microtopography (hummocks) on which tree seedlings are planted to establish tree growth. The ground surface on the seismic line is often lower than the adjacent forested ecosystem due to compaction (Stevenson et al., 2019) and therefore often has higher soil moisture content. Artificial mounding creates drier conditions that encourage tree seedling establishment and success (Lieffers et al., 2017). Mounding has been successfully shown to enhance tree growth across seismic lines (Filicetti et al., 2019), yet where mounds are formed from well-decomposed soil they can eventually flatten out (Lieffers et al., 2017), in which case the microsite benefit may be lost. Furthermore, Takyi and Hillman (2000) found little improvement in tree growth on artificial mounding treatments compared to the flat ground in a drained peatland and Pacé et al. (2018) found that nutrient availability could be a limiting factor in tree regrowth. In fact, there is the potential that mounding may have increased rates of decomposition and soil respiration now that the soil is disturbed (Smolander and Heiskanen, 2007). Yet, despite this, the effects of seismic line disturbance and mounding restoration treatments on organic soil characteristics have not been investigated in detail. Therefore, the objectives of this study were: (1) to determine differences in soil physical and chemical characteristics between narrow and wide lines and the adjacent undisturbed areas and (2) document changes to soil physical and chemical characteristics following mounding compared to unmounded lines and adjacent reference sites. We hypothesized that narrow lines and mounded lines would have less compaction and faster rates of nutrient cycling, evidenced by lower bulk density, increased soil moisture, and C and N enrichment compared to wide lines.
Materials and Methods
Study Site and Design
This study was undertaken in 2018 across seismic lines at two contrasting ecosites, a poor mesic site (seismic lines cleared in approximately 2006/2007) known as Tiger Sands [Figure 1A; defined as a combination of (c1) Laborador tea-mesic Pj-Sb and (g1) Laborador tea-subhygric Sb-Pj (Beckingham and Archibald, 1996) and a treed fen site (lines cleared roughly between 1998 and 2005) known as Kirby (Figure 1B; defined as (k1) treed rich fen; Beckingham and Archibald, 1996)] that was subject to a mounding restoration technique in 2015 (Figure 1C). The poor mesic site (56°21′11.38.8″ N, 111°35′13.2″ W) and treed fen site (55°22′11.17″ N, 111°09′15.4″ W) are located approximately 1 and 2 h south of the city of Fort McMurray, Alberta, respectively. Mean annual temperature (1981–2018) is 1°C and mean annual precipitation is ∼420 mm (Environment Canada, 2019). Both ecosite types were dominated by Picea mariana (Mill.) Britton (black spruce), with Pinus banksiana Lamb (jack pine) also present at the poor mesic site. The lines at the treed fen site were dominated by Carex spp. and Sphagnum spp., and the adjacent undisturbed areas had a much higher coverage of feather mosses.
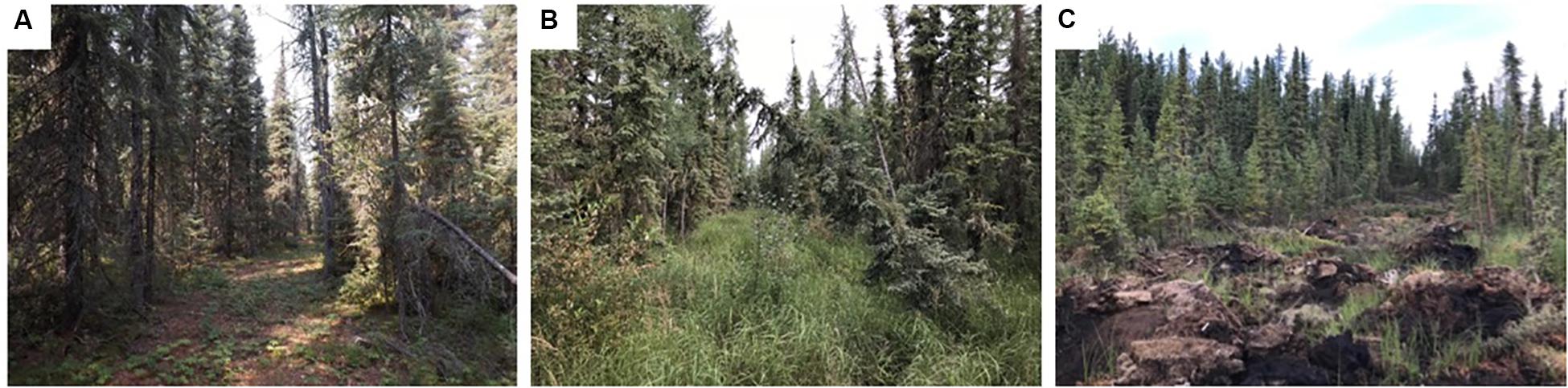
Figure 1. Example of seismic line disturbance at (A) poor mesic site, (B) treed fen site, and (C) example of the mounding technique.
At the poor mesic site, triplicate soil samples were collected near the center of the line, edge of the line and, 5 and 20 m into the adjacent undisturbed area (hereafter known as reference); this procedure was replicated for 24 lines (12 narrow and 12 wide) to a depth of 10 cm from the ground surface. The narrow lines are approximately 0.25 km apart and the wide lines are approximately 0.5 km apart. At the treed fen site, 10 narrow lines (five mounded and five unmounded) were chosen, with triplicate soil samples collected directly on the line and 20 m into the reference sites, at both high and low microtopographic positions at each sampling location. At the mounded line, the “low” samples were collected in locations as low as possible (lowest point on artificial mound or adjacent flat area) without being underwater. These lines were also approximately 0.25 km apart. A metal can (volume = 415 cm3) was used to collect the soil samples to a depth of 10 cm, taking care to avoid compaction during collection. Due to the difficulty of defining the transition from living moss to soil in organic soil layers, at both sites, the moss/lichen layer was not removed prior to sample being taken and was assumed to be the top layer of the soil. Although changes to physical and chemical properties may change throughout the soil column, we chose to focus on the near surface conditions as this is the layer in which tree seedlings will establish and the most likely to be disturbed by seismic exploration activities. This also allowed us to conduct a more extensive sampling design across many seismic lines in remote locations.
Soil Analysis
Soil Physical Characteristics
Bulk density (g/cm3) was determined by drying each sample in an oven at ∼60°C for 2 days or until the sample reached constant weight. Organic matter (OM) content (%) was calculated using the loss on ignition (LOI) method (Rowell, 1995). Approximately 2 g of each dried sample was weighed and then burned in a muffle furnace at 550°C for 3 h. After burning the samples, they were left to cool overnight and weighed post ignition. LOI is calculated as the difference between pre-ignition and post-ignition mass expressed as a percentage of pre-ignition mass. Volumetric water content (VWC) (%) was calculated by taking the wet weight minus the dry weight divided by the volume of the sample. Soil organic carbon (SOC; kg/m2) content in the top 10 cm was calculated by first multiplying the bulk density (converted to kg/m3) by the total carbon content (see next section) and then by 0.1 to account for the depth of the sample.
Soil Chemical Characteristics
Carbon and nitrogen percent element (C, N) and isotope ratios (δ13C, δ15N) were measured using a continuous flow isotope ratio mass spectrometer (Thermo Scientific Delta Plus XL) coupled to an elemental analyzer (Costech ECS 4010). Isotope ratios are expressed as δ values (per mil):
where Rsample and Rstandard are the ratios of heavy to light isotope of the sample relative to the international standards for C and N, Vienna-Pee-Dee Belemnite and atmospheric nitrogen gas (N2), respectively. A subset of the samples (approximately 90) was used for this analysis (0.01 mg for C and 0.02 mg for N). Samples were analyzed at the University of Waterloo Environmental Isotope Laboratory, Waterloo, ON, Canada.
Statistical Analyses
To determine potential differences in bulk density, OM content, VWC, C:N ratios, δ13C, and δ15N at the poor mesic site, we conducted a two-way ANOVA (Type III SS) with position (online, edge, 5 m and reference) and location (narrow or wide lines) as fixed factors. We conducted the same analyses for the treed fen site, with position (high or low) and treatment (mounded lines, unmounded lines, or reference) as fixed factors. To account for the nested experimental design, we averaged the measurements (n = 3) taken for bulk density, OM content, and VWC. If significant relationships were found, a Tukey HSD post hoc analysis was used (lsmeans package; Lenth, 2016). All analyses were performed in R 3.6.2 (R Core Team, 2013).
Results
Soil Physical Characteristics
Wider lines at the poor mesic site (created in approximately 2006/2007) demonstrated significantly greater soil compaction (ANOVA, F2,32 = 11.4; p = 0.0001), with a mean (±standard deviation) bulk density of 0.3 ± 0.2 g/cm3 compared to the narrow lines (0.04 ± 0.1 g/cm3) (Figure 2A). In comparison, the adjacent reference site had a bulk density of 0.05 ± 0.02 g/cm3 (Figure 2A). At the treed fen site (lines created between approximately 1998–2005), the unmounded lines (comparable narrow lines) had similar bulk density at 0.03 ± 0.02 g/cm3 to the narrower lines at the poor mesic site and the mounded lines had a bulk density of 0.06 ± 0.04 g/cm3 (Figure 3A).
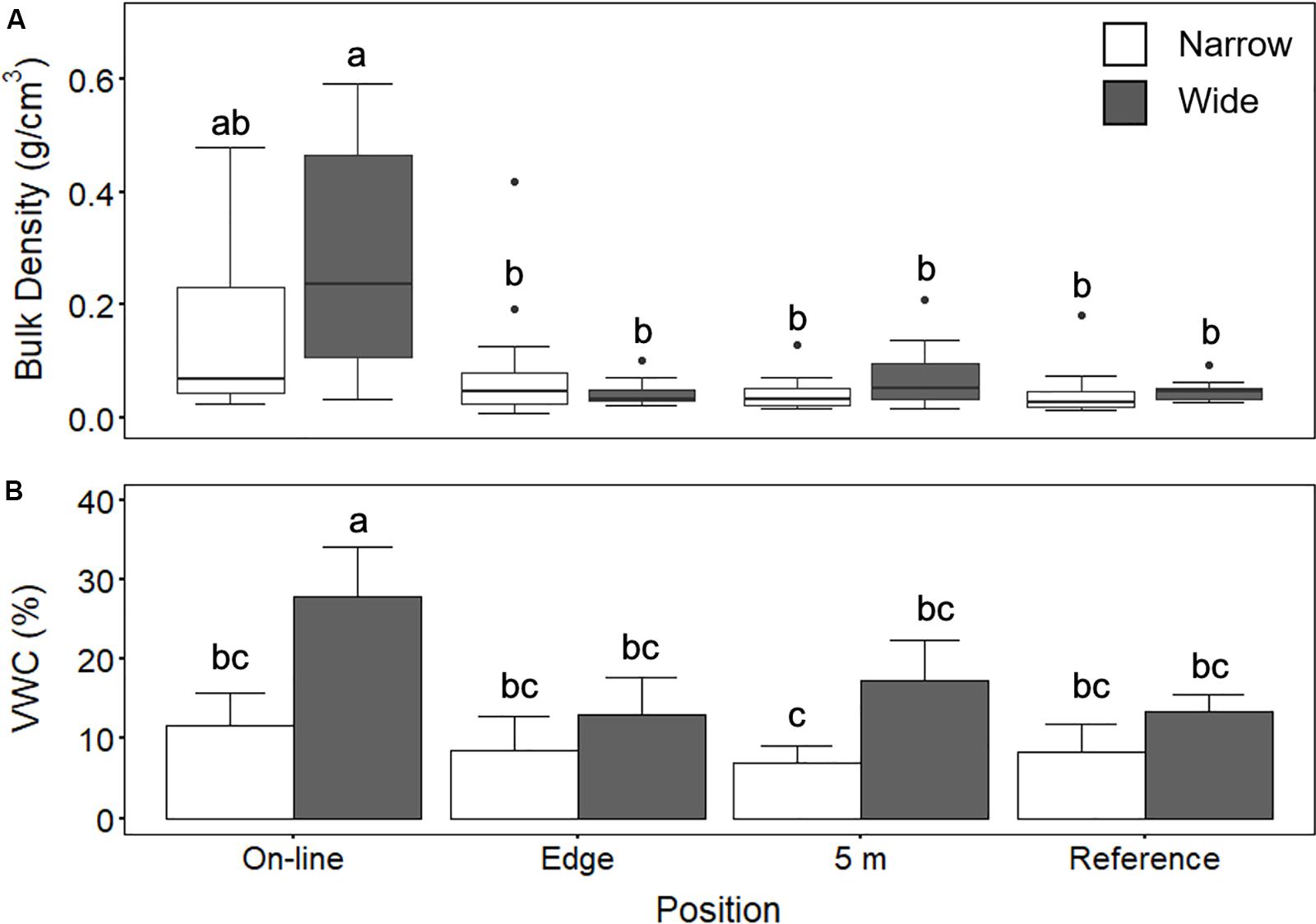
Figure 2. (A) Bulk density (g/cm3) and (B) volumetric water content (VWC; %) on wide and narrow lines at positions Online (wide n = 12, narrow n = 16), Edge (wide n = 16, narrow n = 16), 5 m (wide n = 16, narrow n = 16), and 20 m into the undisturbed site (Reference; wide n = 16, narrow n = 18) at the poor mesic site. Two-way ANOVA followed by a Tukey HSD (p < 0.05). Groups sharing a letter are not significantly different.
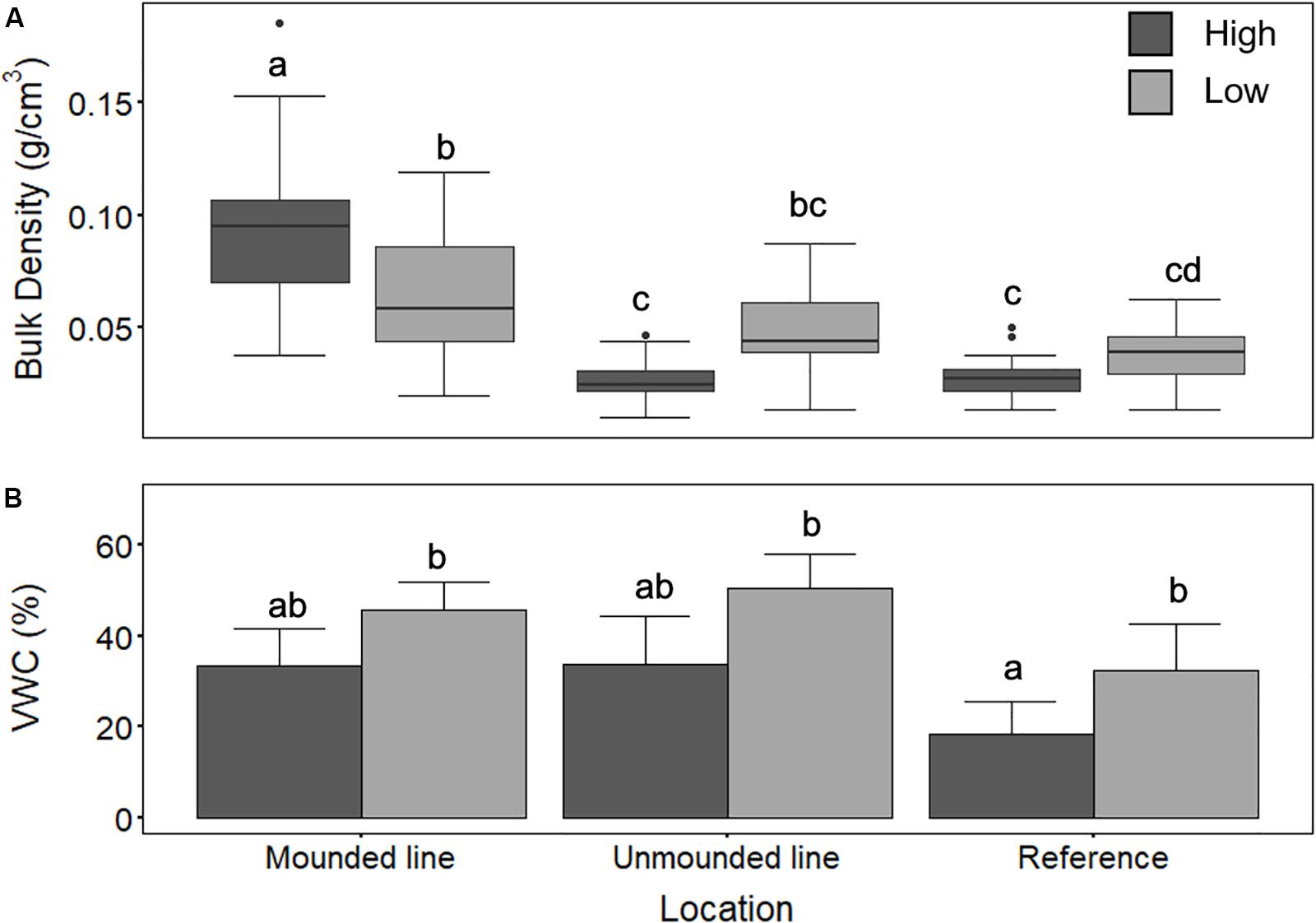
Figure 3. (A) Bulk density (g/cm3) and (B) volumetric water content (VWC; %) for high and low positions at the treed fen site across Mounded line (high n = 16, low n = 15), Unmounded lines (high n = 18, low n = 17), and 20 m into the undisturbed site (Reference; high n = 30, low n = 29). Two-way ANOVA followed by a Tukey HSD (p < 0.05). Groups sharing a letter are not significantly different.
There was significantly higher soil moisture content on the lines compared to the reference sites at the treed fen site (ANOVA, F2,34 = 11:00; p = 0.0002) (Figure 3B). There was no significant difference between high and low positions at both the mounded and unmounded lines. Wider lines at the poor mesic site also had significantly higher soil moisture content (ANOVA, F3,32 = 3.36; p = 0.03) (∼30%), compared with the adjacent reference site at the poor mesic site (Figure 2B).
We found a significant decrease in OM content (between 30 and 40%) from the Reference area and edge to on-line at the poor mesic site (ANOVA, F3,34 = 8.3, p = 0.0001) (Table 1). However, there was no significant difference in OM content between the line (both mounded and unmounded) and adjacent undisturbed locations at the treed fen site (Table 2). SOC in the top 10 cm was greatest on the wide (3.92 ± 1.6 kg/m2) and mounded (3.76 ± 1.4 kg/m2) lines, respectively (Table 3), which both had the greatest rates of compaction (Figures 2A, 3A).
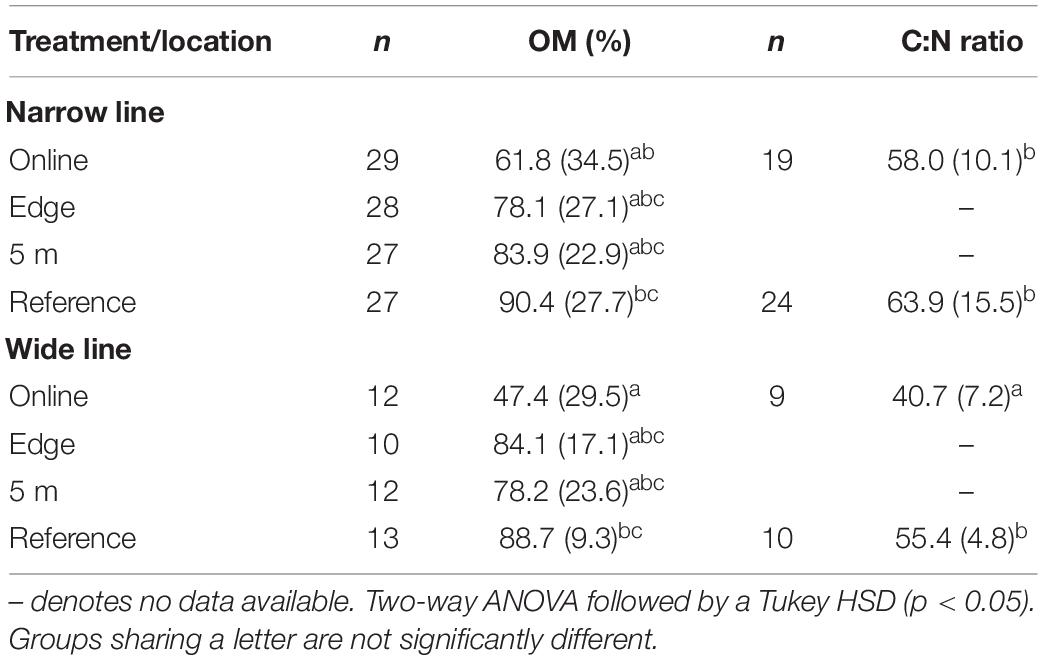
Table 1. Mean (± standard deviation) organic matter content (OM%), C:N ratio, δ13C and δ15N values for poor mesic site.
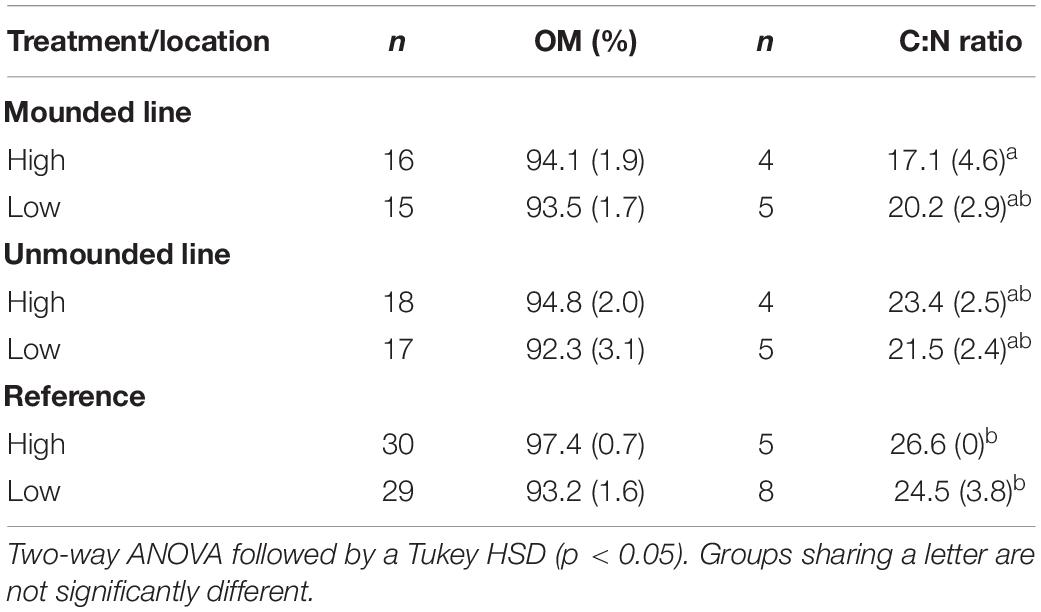
Table 2. Mean (±standard deviation) organic matter content (OM%), C:N ratio, δ13C and δ15N values for treed fen site.
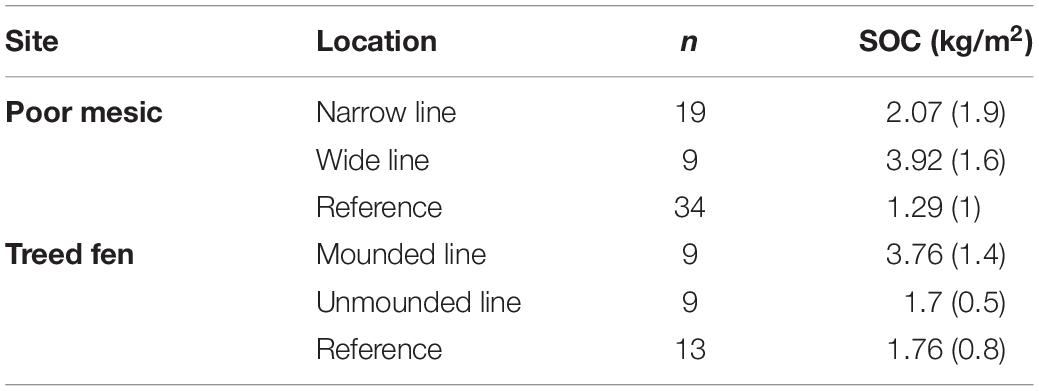
Table 3. Mean (±standard deviation) soil organic carbon (SOC; kg/m2) in the top 10 cm across each location.
Soil Chemical Characteristics
Mean (±SD) C:N ratios ranged from 40.7 ± 7.2 (online) and 63.9 ± 15.5 (reference) at the poor mesic site to 17.1 ± 4.6 (online) and 26.6 ± 0 (reference) at the treed fen site (Tables 1, 2). C:N ratios were significantly narrower on the wide lines at the poor mesic site and on the mounded line at the treed fen compared to the adjacent reference areas (Tables 1, 2).
We observed significantly higher δ13C values on the wider lines compared to both the narrow lines and the reference sites (ANOVA, F2,25 = 14.73, p = 0.0003) (Figure 4A). Similarly, there was significantly higher δ15N enrichment in online samples compared to reference locations (ANOVA, F2,25 = 61.02, p < 0.0001) (Figure 4B). The mounded lines at the treed fen sites were significantly enriched in both δ13C (ANOVA, F2,24 = 7.01, p = 0.004) and δ15N (ANOVA, F2,25 = 7.8, p = 0.002) in comparison to the reference sites (Figure 5). Overall, δ13C values between both the poor mesic site and the treed fen were similar, but the treed fen was more enriched in δ15N than the poor mesic site.
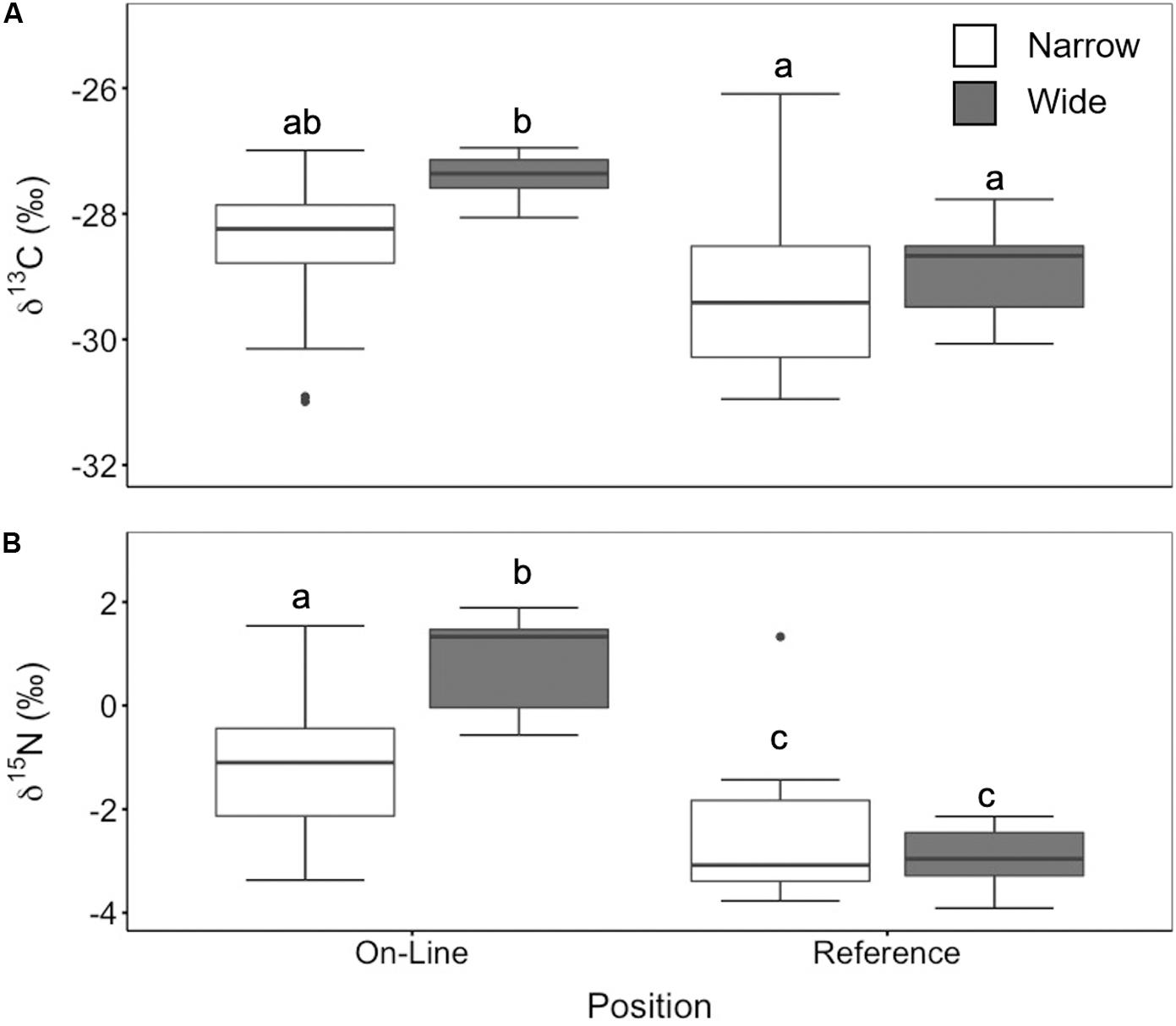
Figure 4. (A) δ13C (‰) and (B) δ15N (‰) for online (wide n = 9, narrow n = 19) and reference sites (wide n = 10, narrow = 24) at both narrow and wide lines. Two-way ANOVA followed by a Tukey HSD (p < 0.05). Groups sharing a letter are not significantly different.
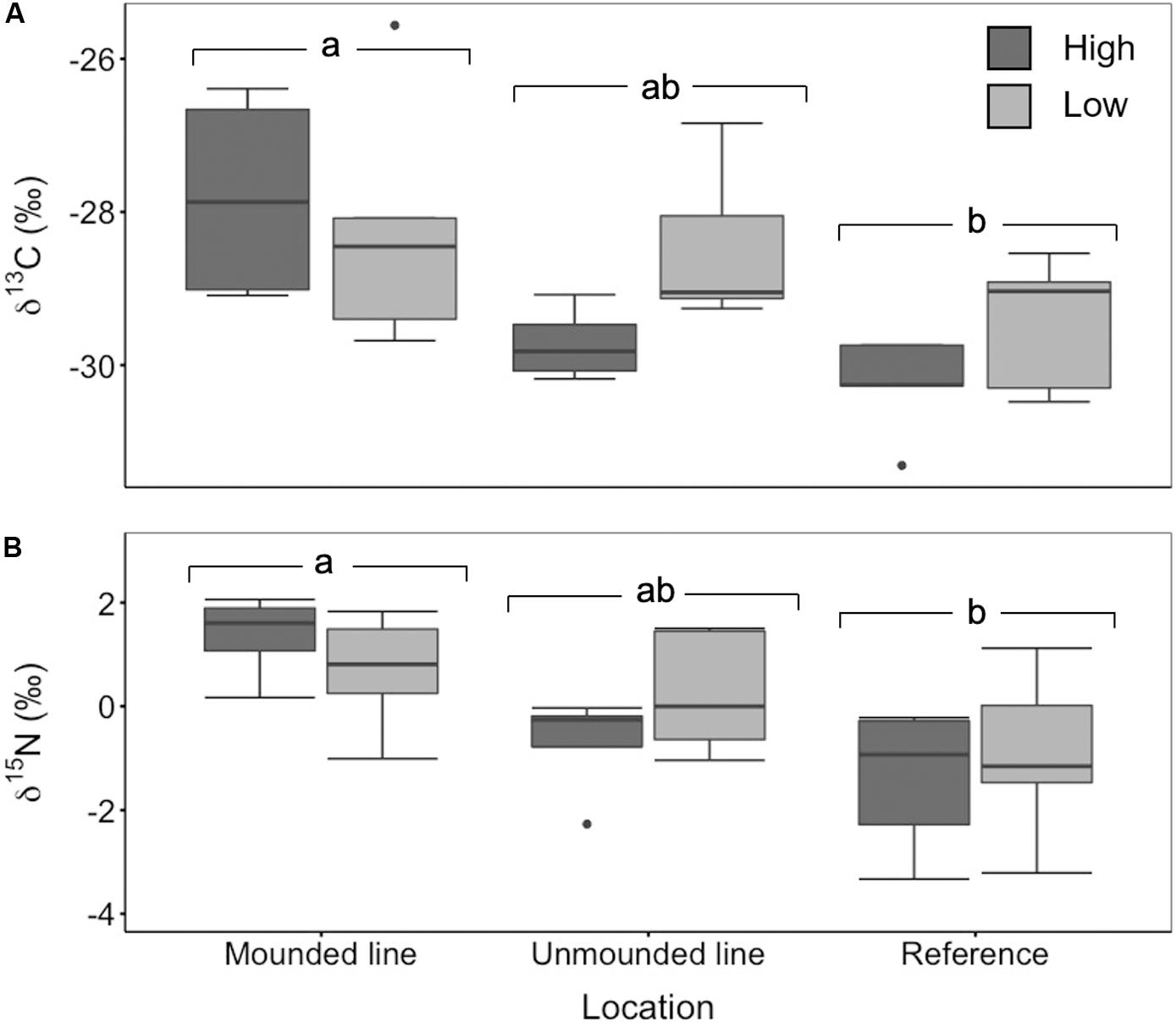
Figure 5. (A) δ13C (‰) and (B) δ15N (‰) for mounded (high n = 4, low n = 5) and unmounded lines (high n = 4, low n = 5) and reference sites (high n = 5, low n = 8). Two-way ANOVA followed by a Tukey HSD (p < 0.05). Groups sharing a letter are not significantly different.
Discussion
Across Alberta, it is estimated that seismic line disturbances from resource extraction operations account for more than 80% of boreal anthropogenic disturbances (Pasher et al., 2013), covering an area of approximately 1200 km2 (Strack et al., 2019). Our aim was to investigate the impact of these disturbances and subsequent restoration methods on soil physical and chemical properties across contrasting boreal ecosites. We found a significant effect of seismic line disturbances on soil characteristics at both the poor mesic and treed fen sites. There was a greater impact to soils on the wide lines at the poor mesic site, with greater increases in bulk density and a higher moisture content due to the lowering of the surface (reducing water table depth) following a potential combination of both compaction and loss of OM. Bulk density at the treed fen was similar to an undisturbed boreal peatland by Waddington and Roulet (2000) (between 0.05 and 0.15 g/cm–3) and to a study by Strack et al. (2018) on a boreal peatland impacted by winter road construction (0.04–0.16 g/cm–3), whereas on the wide lines at the poor mesic site, the bulk density was more than five times this. This is due to both higher mineral soil content at this ecosite, but also a combination of increased compaction, mineralization, and decomposition on the line. Soil compaction can cause a decrease in soil aeration, leading to changes in soil morphology and impacting productivity of the system (Startsev and McNabb, 2009). A likely cause of this compaction is the use of heavy machinery to clear the wide lines but may also be related to removal of the more porous surface soil layer during the disturbance, or continued mineralization post-disturbance resulting in continued compaction. At both sites, the increased soil compaction on the lines also led to increases in VWC, likely due to the soil surface now being closer to the water table. Higher bulk density may also result in smaller pore sizes that have greater moisture retention, even with a water table drawdown during drier conditions, further contributing to higher soil moisture (Rezanezhad et al., 2016; Golubev and Whittington, 2018). The compaction of the soil and homogenizing of the landscape through the removal of localized microtopographic features may lead to pooling of water near the surface, as water cannot easily infiltrate highly compacted soil (Dabros et al., 2018; Golubev and Whittington, 2018). However, consistent increased moisture levels could also reduce respiration rates through reduction of the aerobic zone, and this will likely lower soil OM decomposition (Ise et al., 2008). Removal of the woody vegetation from the lines can also contribute to increases in soil moisture, through a reduction in water intake and decreased rates of evapotranspiration (Vitt et al., 1975; Dabros et al., 2018).
There was a significant difference in OM content from the reference site to the line in poor mesic sites. This could be attributed to mineralization of OM following disturbance (Mallik and Hu, 1997), either at the time of seismic line construction, or persisting in the new environmental conditions present on the line, or both. It could also result in a change in the net carbon balance of the system due to shifting inputs through lack of tree cover and changing ground layer vegetation. It is possible that seismic line construction and subsequent mounding in the treed fen also caused losses of OM through increased decomposition rates and subsequent respiration. When the soil is disturbed, inverted to create the mound and exposed to the air, higher amounts of decomposition and soil respiration through increased microbial activity would be expected (Mallik and Hu, 1997). Although not significantly different, we did observe consistently lower mean OM content on the line than in the reference ecosystem at the treed fen site. However, given that approximately the entire upper 1 m of the soil column is partially decomposed OM, these losses would likely not be measurable by investigating OM content alone. In fact, the amount of CO2 lost from the system following this disturbance is poorly known and is the subject of further study.
The poor mesic site had higher C:N ratios, attributed to larger influence of litterfall from adjacent trees and woody vegetation and potentially slower decomposition (Kuhry and Vitt, 1996) compared to the treed fen site. During line clearing, there are a variety of practices for removing tree cover, including removal from site, creation of windrows, and mulching of woody debris. Given the high C:N ratio of wood, this may have an impact on measured soil C:N values, but this would be difficult to account for, given that specific actions at any given site are difficult to obtain. The low C:N ratios found at the mounded site on the treed fen indicate that decomposition has been enhanced on these artificial hummocks (Kuhry and Vitt, 1996; Drollinger et al., 2019) and support the hypotheses of an increase in respiration rates and lower OM content expected in these areas. This is further supported by the enriched δ13C values found at these sites. This indicates enhanced decomposition post-disturbance and may be a useful indicator of carbon loss given we cannot easily capture a loss in OM at these wetland sites.
Removal of the woody vegetation would lead to differences in N-inputs between lines and undisturbed areas, resulting from changes in the vegetation and microbial community, and the microclimate/abiotic conditions following disturbance. Enrichment occurs when there are more arbutoid or monotropoid mycorrhizae that give enriched N to their plant partners relative to ericoid or ecto-mycorrhizae that give depleted N (Hobbie and Högberg, 2012; Goud and Sparks, 2018). Higher δ15N can also be attributed to warmer soils and soils with more ammonium (NH4+) relative to nitrate (NO3–) (Craine et al., 2015), the latter likely when soil moisture content is high (Macrae et al., 2013; Wood et al., 2016). Perhaps most importantly, and intimately connected to the previous points, are rates of the various N cycling components, especially increasing mineralization and immobilization given the alterations of oxic-anoxic conditions following disturbance (Amundson et al., 2003; Kramer et al., 2003; Asada et al., 2005). Regardless of ecosystem or disturbance type, enrichment of δ15N in these boreal systems is most likely driven by different N sources, particularly the relative availability of organic vs. mineral/mineralized N (Högberg, 1997) which can have implications for vegetation community change and/or recovery.
The changes in ecohydrological dynamics following disturbance will over time lead to changes in the vegetation composition (Dabros et al., 2018), including increases in graminoid cover (for example, an increase in sedge cover across wetter disturbed peatland areas; van Rensen et al., 2015). The removal of woody vegetation and the opening of the tree canopy allow Sphagnum species to rapidly colonize these disturbed sites and outcompete feather moss (Bisbee et al., 2001). This can become an issue where slow growing tree-saplings that are successful in naturally regenerating on the lines are outcompeted by the faster growing moss species (Ohlson and Zackrisson, 1992; Roy et al., 2000). The lines at the treed fen have a substantial cover of Sphagnum in comparison to the feather dominated reference areas. We also saw colonization of tree saplings on the artificial mounds at the treed fen site. However, the increase in Sphagnum cover could lead to an increase in C uptake and decrease respiration rates as Sphagnum is typically highly productive and recalcitrant (Turetsky et al., 2008). In fact, similar carbon exchange function can arise in peatland plant communities despite species turnover (Robroek et al., 2017), suggesting that new communities could offer similar C storage function despite the lack of trees.
Conclusion
This work has provided an insight into changes in soil properties following the creation and subsequent restoration of seismic lines across two contrasting ecosites in northern Alberta. Overall, seismic line disturbances significantly impact soil physical and chemical characteristics. Specifically, seismic lines increase bulk density and moisture content, decrease OM content, and enhance decomposition. The large reduction in OM found in poor mesic sites has major implications for carbon cycling across these sites, indicating increased rates of mineralization. This is also highlighted by the increased δ13C and δ15N values found on the line, also pointing to increases in decomposition. The mounding technique used for restoration of these lines (Lieffers et al., 2017) also causes some disturbance to soil properties through increased decomposition and higher bulk density. Although seismic lines only make up a relatively small part of the landscape, it has to be questioned whether mounding these lines is a trade-off between disturbing the landscape to encourage tree regeneration and enhancing OM decomposition leading to increased carbon losses from the system. As mounding has been successfully used to improve tree regeneration in other ecosite types, future work should involve investigating alternative mounding techniques to ensure both tree recovery and minimal impact to the surrounding landscape in wetland systems.
Author’s Note
This research was undertaken within the boundaries of Treaty 8, traditional lands of the Dene and Cree, as well as the traditional lands of the Métis of northeastern Alberta. We would also like to acknowledge that the University of Waterloo is on the traditional territory of the Neutral, Anishnaabeg, and Hausenosaunee Peoples. The University of Waterloo is situated on the Haldimand Tract, land promised to Six Nations, which includes six miles on each side of the Grand River.
Data Availability Statement
The datasets generated for this study are available on request to the corresponding author.
Author Contributions
All authors contributed to the article and approved the submitted version. SD and MS designed the study. SD and CF performed the research. SD analyzed the data input from EG and MS. SD, EM, CF, SN, and MS wrote the manuscript.
Funding
This research is part of the Boreal Ecosystem Recovery and Assessment (BERA) project (www.bera-project.org), and was supported by a Natural Sciences and Engineering Research Council of Canada Collaborative Research and Development Grant (CRDPJ 469943-14) in conjunction with Alberta-Pacific Forest Industries, Cenovus Energy, ConocoPhillips Canada, and Canadian Natural Resources.
Conflict of Interest
The authors declare that the research was conducted in the absence of any commercial or financial relationships that could be construed as a potential conflict of interest.
Acknowledgments
We would like to thank Lucas Schmaus, Duncan Forbes, and Angelo Filicetti for assistance in the field and Matt Coulas and Olivia Trudeau for their help with the soil sample processing. We also thank Sophie Wilkinson for earlier comments on the manuscript and to the two reviewers whose reviews really strengthened this research.
Supplementary Material
The Supplementary Material for this article can be found online at: https://www.frontiersin.org/articles/10.3389/feart.2020.00281/full#supplementary-material
References
Allison, S. D., and Treseder, K. K. (2011). Climate change feedbacks to microbial decomposition in boreal soils. Fungal Ecol. 4, 362–374. doi: 10.1016/j.funeco.2011.01.003
Amundson, R., Austin, A. T., Schuur, E. A. G., Yoo, K., Matzek, V., Kendall, C., et al. (2003). Global patterns of isotopic composition of soil and plant nitrogen. Glob. Biogeochem. Cycles 17:1031. doi: 10.1029/2002GB001903
Asada, T., Warner, B., and Aravena, R. (2005). Effects of the early stage of decomposition on change in carbon and nitrogen isotopes in Sphagnum litter. J. Plant Interact. 1, 229–237. doi: 10.1080/17429140601056766
Beckingham, J. D., and Archibald, J. H. (1996). Field Guide to Ecosites of Northern Alberta. Natural Resources Canada. Edmonton: Canadian Forest Service.
Bisbee, K. E., Gower, S. T., Norman, J. M., and Nordheim, E. V. (2001). Environmental controls on ground cover species composition and productivity in a boreal black spruce forest. Oecologia 129, 261–270. doi: 10.1007/s004420100719
Boelter, D. H. (1969). Physical properties of peats as related to degree of decomposition. Soil Sci. Soc. Am. J. 33, 606–609. doi: 10.2136/sssaj1969.03615995003300040033x
Bradford, M. A., Davies, C. A., Frey, S. D., Maddox, T. R., Melillo, J. M., Mohan, J. E., et al. (2008). Thermal adaptation of soil microbial respiration to elevated temperatures. Ecol. Lett. 11, 1316–1327. doi: 10.1111/j.1461-0248.2008.01251.x
Braverman, M., and Quinton, W. I. (2016). Hydrological impacts of seismic lines in the wetland-dominated zone of thawing, discontinous permafrost, Northwest Territories, Canada. Hydrol. Process. 30, 2617–2627. doi: 10.1002/hyp.10695
Camill, P., Chihara, L., Adams, B., Andreassi, A., Barry, A., Kalim, S., et al. (2010). Early life history transitions and recruitment of Picea mariana in thawed boreal permafrost peatlands. Ecology 91, 448–459. doi: 10.1890/08-1839.1
Connin, S. L., Feng, X., and Virginia, R. A. (2001). Isotopic discrimination during long term decomposition in an arid land ecosystem. Soil Biol. Biochem. 31, 41–51. doi: 10.1016/s0038-0717(00)00113-9
Craine, J. M., Brookshire, E. N. J., Cramer, M. D., Hasselquist, N. J., Koba, K., Marin-spiota, E., et al. (2015). Ecological interpretations of nitrogen isotope ratios of terrestrial plants and soils. Plant Soil 396, 1–26. doi: 10.1007/s11104-015-2542-1
Dabros, A., Pyper, M., and Castilla, G. (2018). Seismic lines in the boreal and arctic ecosystems of North America: environmental impacts, challenges, and opportunities. Environ. Rev. 26, 214–229. doi: 10.1139/er-2017-0080
Damman, A. W. (1988). Regulation of nitrogen removal and retention in Sphagnum bogs and other peatlands. Oikos 51, 291–305.
DeMars, C. A., and Boutin, S. (2018). Nowhere to hide: effects of linear features on predator-prey dynamics in a large mammal system. J. Anim. Ecol. 87, 274–284. doi: 10.1111/1365-2656.12760
Drollinger, A., Kuzyakov, Y., and Glatzel, S. (2019). Effects of peat decomposition on δ13C and δ15N depth profiles of Alpine bogs. Catena 178, 1–10. doi: 10.1016/j.catena.2019.02.027
Dyer, S. J., O’Neill, J. P., Wasel, S. M., and Boutin, S. (2001). Avoidance of industrial development by woodland caribou. J. Wildl. Manag. 65, 531–542.
Dyer, S. J., O’Neill, J. P., Wasel, S. M., and Boutin, S. (2002). Quanitifying barrier effects of roads and seismic lines on movements of female woodland caribou in northeastern Alberta. Can. J. Zool. 80, 839–845. doi: 10.1139/z02-060
Environment Canada (2019). National Climate Data and Information Archive. Available at: http://www.climate.weatheroffice.ec.gc.ca/climateData/monthlydata_e.html?timeframe=3&Prov=CA&StationID=4977&Year=1951&Month=2&Day=26 (accessed August 1, 2019).
Filicetti, A. T., Cody, M., and Nielsen, S. E. (2019). Caribou conservation: restoring trees in seismic lines in Alberta, Canada. Forests 10:185. doi: 10.3390/f10020185
Forest Resource Development Agreement [FRDA] (1989). Mounding for Site Preparation. FRDA Memo No. 100. Available online: https://www.for.gov.bc.ca/hfd/pubs/Docs/Frm/Frm100.pdf (accessed November 1, 2019).
Golubev, V., and Whittington, P. (2018). Effects of volume change on the unsaturated hydraulic conductivity of Sphagnum moss. J. Hydrol. 559, 884–894. doi: 10.1016/j.jhydrol.2018.02.083
Goud, E. M., and Sparks, J. P. (2018). Leaf stable isotopes suggest shared ancestory is an important driver of functional diversity. Oecologia 187, 967–975. doi: 10.1007/s00442-018-4186-3
Henneb, M., Valeria, O., Thiffault, N., Fenton, N. J., and Bergeron, Y. (2019). Effects of mechanical site preparation on microsite availability and growth of planted black spuce in canadian paludified forests. Forests 10:670. doi: 10.3390/f10080670
Hobbie, E. A., and Högberg, P. (2012). Tansley review: nitrogen isotopes link mycorrhizal fungi and plants to nitrogen dynamics. New Phytol. 196, 367–382. doi: 10.1111/j.1469-8137.2012.04300.x
Högberg, P. (1997). Tansely review No. 96: 15N natural abundance in soil-plant systems. New Phytol. 137, 179–203. doi: 10.1046/j.1469-8137.1997.00808.x
Ise, T., Dunn, A. L., Wofsy, S. C., and Moorcroft, P. R. (2008). High sensitivity of peat decomposition to climate change through water table feedback. Nat. Geosci. 1, 763–766. doi: 10.1038/ngeo331
Kramer, M. G., Sollins, P., Sletten, R. S., and Swart, P. K. (2003). N isotope fractionation and measures of organic matter alteration during decomposition. Ecology 84, 2021–2025. doi: 10.1890/02-3097
Kuhry, P., and Vitt, D. H. (1996). Fossil carbon/nitrogen ratios as a measure of peart decomposition. Ecology 77, 271–275. doi: 10.2307/2265676
Lee, P., and Boutin, S. (2006). Persistence and developmental transition of wide seismic lines in the western Boreal Plains of Canada. J. Environ. Manage. 78, 240–250. doi: 10.1016/j.jenvman.2005.03.016
Lenth, R. V. (2016). Least-squares means: the R package lsmeans. J. Stat. Softw. 69, 1–33. doi: 10.18637/jss.v069.i01
Li, J., Ziegler, S., Lane, C. S., and Billings, S. A. (2012). Warming-enhanced preferential microbial mineralization of humified boreal forest soil organic matter: interpretation of soil profiles along a climate transect using laboratory incubations. J. Geophys. Res. 17:G02008. doi: 10.1029/2011JG001769
Lieffers, V. J., Caners, R. T., and Ge, H. (2017). Re-establishment of hummock topography promotes tree regeneration on highly disturbed moderate-rich fens. J. Environ. Manage. 197, 258–264. doi: 10.1016/j.jenvman.2017.04.002
Lovitt, J., Rahman, M. M., Saraswati, S., McDermid, G. J., Strack, M., and Xu, B. (2018). UAV remote sensing can reveal the effects of low-impact seismic lines on surface morphology, hydrology and methane (CH4) release in a boreal treed bog. J. Geophys. Res. Biogeosci. 123, 1117–1129. doi: 10.1002/2017JG004232
Macrae, M. L., Devito, K. J., Strack, M., and Waddington, J. M. (2013). Effect of water table drawdown on peatland nutrient dynamics: implications for climate change. Biogeochemistry 112, 661–676. doi: 10.1007/s10533-012-9730-3
Mallik, A. U., and Hu, D. (1997). Soil respiration following site preparation treatments in boreal mixedwood forest. For. Ecol. Manag. 97, 265–275. doi: 10.1016/s0378-1127(97)00067-4
Malmer, N., and Holm, E. (1984). Variation in the C/N-quotient of peat in relaction to decomposition rate and age determination with 210Pb. Oikos 43, 171–182.
Nadelhoffer, K., and Fry, B. (1988). Controls on natural nitrogen-15 and carbon-13 abundances in forest soil organic matter. Soil Sci. Soc. Am. J. 52, 1633–1640. doi: 10.2136/sssaj1988.03615995005200060024x
Ohlson, M., and Zackrisson, O. (1992). Tree establishment and microhabitat relationships in north Swedish peatlands. Can. J. For. Res. 22, 1869–1877. doi: 10.1139/x92-244
Pacé, M., Fenton, N. J., Paré, D., and Bergeron, Y. (2018). Differential effects of feather and Sphagnum spp. mosses on black spruce germination and growth. For. Ecol. Manag. 415-416, 10–18. doi: 10.1016/j.foreco.2018.02.020
Pasher, J., Seed, E., and Duffe, J. (2013). Development of boreal ecosystem anthropogenic disturbance layers for Canada based on 2008-2010 Landsat imagery. Can. J. Rem. Sen. 39, 42–58. doi: 10.5589/m13-007
Price, J. S., Heathwaite, A. L., and Baird, A. J. (2003). Hydrological processes in abandoned and restored peatlands: an overview of management approaches. Wetlands Ecol. Manag. 11, 65–83.
R Core Team (2013). R: A Language and Environment for Statistical Computing. Vienna: R Foundation for Statistical Computing.
Rezanezhad, F., Price, J. S., Quinton, W. L., Lennartz, B., Milojevic, T., and Van Cappellen, P. (2016). Structure of peat soils and implications for water storage, flow and solute transport: a review update for geochemists. Chem. Geol. 429, 75–84. doi: 10.1016/j.chemgeo.2016.03.010
Robroek, B. J. M., Jassey, V. E., Payne, R. J., Marti, M., Bragazza, L., Bleeker, A., et al. (2017). Taxonomic and functional turnover are decoupled in European peat bogs. Nat. Commun. 8:1161. doi: 10.1038/s41467-017-01350-5
Roy, V., Ruel, J.-C., and Plamondon, A. P. (2000). Establishment, growth and survival of natural regeneration after clearcutting and drainage on forested wetlands. For. Ecol. Manag. 19, 253–267. doi: 10.1016/s0378-1127(99)00170-x
Scheffer, R. A., van Logestijn, R. S. P., and Verhoeven, J. T. A. (2001). Decomposition of Carex and Sphagnum litter in two mesotrophic fens differing in dominant plant species. Oikos 92, 44–54. doi: 10.1034/j.1600-0706.2001.920106.x
Smolander, A., and Heiskanen, J. (2007). Soil N and C transformations in two forest clear-cuts during three year after mounding and inverting. Can. J. Soil. Sci. 87, 251–258. doi: 10.4141/s06-028
Startsev, A. D., and McNabb, D. H. (2009). Effects of compaction on aeration and morphology of boreal forest soils in Alberta, Canada. Can. J. Soil. Sci. 89, 45–56. doi: 10.4141/cjss06037
Stern, E. R., Riva, F., and Nielsen, S. E. (2018). Effects of narrow linear disturbances on light and wind patterns in fragmented boreal forests in northeastern Alberta. Forests 9:486. doi: 10.3390/f9080486
Stevenson, C. J., Fillicetti, A. T., and Nielsen, S. E. (2019). High precision altimeter demonstrates simplification and depression of microtopography on seismic lines in treed peatlands. Forests 10:295. doi: 10.3390/f10040295
Strack, M., Hayne, S., Lovitt, S., McDermid, G. J., Rahman, M. M., Saraswati, S., et al. (2019). Petroleum exploration increases methane emissions from northern peatlands. Nat. Commun. 10:2804. doi: 10.1038/s41467-019-10762-4
Strack, M., Softa, D., Bird, M., and Xu, B. (2018). Impact of winter roads on boreal peatland carbon exchange. Glob. Chang. Biol. 24, 1–12.
Takyi, S. K., and Hillman, G. R. (2000). Growth of coniferous seedlings on a drained and mounded peatland in Central Alberta. N. J. Appl. For. 17, 71–79. doi: 10.1093/njaf/17.2.71
Turetsky, M. R., Crow, S. E., Evans, R. J., Vitt, D. H., and Wieder, R. K. (2008). Trade-offs in resource allocation among moss species control decomposition in boreal peatlands. J. Ecol. 96, 1297–1305. doi: 10.1111/j.1365-2745.2008.01438.x
van Rensen, C. K., Nielsen, S. E., White, B., Vinge, T., and Lieffers, V. J. (2015). Natural regeneration of forest vegetation on legacy seismic lines in boreal habitats in Alberta’s oil sands region. Biol. Conserv. 184, 127–135. doi: 10.1016/j.biocon.2015.01.020
Vitt, D. H., Achuff, P., and Andrus, R. E. (1975). The vegetation and chemical properties of patterned fens in the Swan Hills, north central Alberta. Can. J. Bot. 53, 2776–2795. doi: 10.1139/b75-306
Waddington, J. M., and Roulet, N. T. (2000). Carbon balance of a boreal patterned peatland. Glob. Change Biol. 6, 87–98.
Walbridge, M. R., and Lockaby, B. G. (1994). Effects of forest management on biogeochemical functioning in southern forested wetlands. Wetlands 14, 10–17. doi: 10.1007/bf03160617
Westbrook, C. J., Devito, K. J., and Allan, C. J. (2006). Soil N cycling in harvested and pristine Boreal forests and peatlands. For. Ecol. Manag. 234, 227–237. doi: 10.1016/j.foreco.2006.07.004
Williams, T. J., Quinton, W. L., and Baltzer, J. L. (2013). Linear disturbances on discontinuous permafrost: implications for thaw-induced changes to land cover and drainage patterns. Environ. Res. Lett. 8:025006. doi: 10.1088/1748-9326/8/2/025006
Keywords: linear disturbance, boreal forest, bulk density, decomposition, stable isotopes, restoration, artificial microtopography
Citation: Davidson SJ, Goud EM, Franklin C, Nielsen SE and Strack M (2020) Seismic Line Disturbance Alters Soil Physical and Chemical Properties Across Boreal Forest and Peatland Soils. Front. Earth Sci. 8:281. doi: 10.3389/feart.2020.00281
Received: 25 February 2020; Accepted: 17 June 2020;
Published: 07 July 2020.
Edited by:
Jianghua Wu, Memorial University of Newfoundland, CanadaReviewed by:
David Cooper, Colorado State University System, United StatesGustaf Granath, Uppsala University, Sweden
Copyright © 2020 Davidson, Goud, Franklin, Nielsen and Strack. This is an open-access article distributed under the terms of the Creative Commons Attribution License (CC BY). The use, distribution or reproduction in other forums is permitted, provided the original author(s) and the copyright owner(s) are credited and that the original publication in this journal is cited, in accordance with accepted academic practice. No use, distribution or reproduction is permitted which does not comply with these terms.
*Correspondence: Scott J. Davidson, czdkYXZpZHNvbkB1d2F0ZXJsb28uY2E=