- 1Department of Earth and Environmental Sciences, Lehigh University, Bethlehem, PA, United States
- 2Institute for Peat and Mire Research, School of Geographical Sciences, Northeast Normal University, Changchun, China
The oxygen isotope composition (δ18O) of plant cellulose has been widely used to study ecohydrological processes of ecosystems as well as to reconstruct past climate conditions in terrestrial climate archives. These applications are grounded on a key assumption that the biochemical fractionation during cellulose synthesis is a constant around +27‰ and is not affected by environmental factors. Here we revisit the influence of temperature on biochemical fractionation factor during cellulose synthesis using a global compilation of Sphagnum cellulose δ18O data. Sphagnum (peat mosses) are known for inhabiting waterlogged peatlands and possessing unique physiological strategy in that their cellulose δ18O could closely reflect growing-season precipitation δ18O. Although within-site cellulose δ18O variability shows a median standard deviation of 0.7–0.8‰ resulting from different degrees of evaporative enrichment of 18O in metabolic leaf water, this evaporative enrichment should be a small quantity due to the external capillary “water buffer” in Sphagnum mosses. Using site-specific minimum cellulose δ18O data that most likely reflect the signal of unevaporated source water, we show that the apparent enrichment factor between cellulose and precipitation δ18O increases with decreasing air temperature. In particular, the apparent enrichment factor could reach values as high as +32‰ when growth temperature is below 5°C. This observational dataset extends the support for the temperature-dependent oxygen isotope fractionation in plant cellulose synthesis previously demonstrated in laboratory experiment, with implications for paleoclimate and plant physiology studies that employ cellulose δ18O measurements, particularly in alpine regions.
Introduction
The oxygen isotope ratio (18O/16O, expressed as δ18O) of plant cellulose (hereafter δ18Oc) is a valuable tracer to characterize the environmental conditions during tissue growth (Barbour, 2007; Sternberg, 2009). It has been used as a proxy to reconstruct past climate conditions in tree rings (Libby et al., 1976), lake sediments (Edwards and McAndrews, 1989), and peat deposits (Hong et al., 2000). Earlier studies established empirical relationships between δ18Oc and climatic parameters, such as temperature, relative humidity, and precipitation amount for a given region (Libby et al., 1976; Treydte et al., 2006; Managave et al., 2010). An improved understanding of physiological and biochemical processes enabled developing mechanistic models to quantitatively decipher the δ18Oc signal for paleoclimate reconstruction (Roden et al., 2000; Kahmen et al., 2011). It has been well recognized that the source water for vascular plants, in most cases precipitation, is taken up by roots for photosynthesis in leaves, while the leaf water is enriched in 18O relative to precipitation due to plant transpiration through stomata. The carbohydrate product (e.g., sucrose) that has been further enriched in 18O by biochemical fractionation is then translocated to the site of cellulose synthesis, such as stem, where additional biochemical exchange occurs with stem water. As such, plant δ18Oc signal essentially reflects precipitation δ18O (hereafter δ18Op) with an offset governed by both physiological and biochemical processes (Richter et al., 2008; Sternberg, 2009).
The current mechanistic models have made progress in depicting the physiological effects including how different climatic parameters affect the degree of 18O enrichment in leaf water and imprint the δ18Oc signal (Kahmen et al., 2011). However, less is known about the biochemical effects that are equally important in shaping the final δ18Oc signal. The combined physiological and biochemical effects could be described by the following equation (Barbour and Farquhar, 2000):
where δ18Oc and δ18Op are the oxygen isotope composition of cellulose and precipitation, respectively; Δleaf is the degree of 18O enrichment in leaf water known as the physiological effect; px is the proportion of unenriched water at the site of cellulose synthesis; pex is the proportion of exchangeable oxygen in carbohydrate substrates destined for cellulose synthesis; and εbio is the biochemical enrichment (or fractionation) factor associated with the exchange of oxygen atoms between carbonyl group and medium water. The px is expected to be unity for stem cellulose (Cernusak et al., 2005; Kahmen et al., 2011), but for leaf cellulose it is expected to be less than unity (Helliker and Ehleringer, 2002; Barbour, 2007; Kahmen et al., 2011; Song et al., 2014b; but see Liu et al., 2017), or even has been assumed to be zero (Tuthorn et al., 2014). The pex and εbio have been successfully constrained using different approaches to be 0.42 ± 0.3 and 27 ± 3‰, respectively (DeNiro and Epstein, 1981; Sternberg and DeNiro, 1983; Sternberg et al., 1986; Yakir and DeNiro, 1990; Roden et al., 2000). These biochemical factors are a net result of complex biochemical processes in cellulose synthesis (Waterhouse et al., 2013) and seem to contain less climatic information, thus these factors are almost always treated as constants in mechanistic modeling. For instance, Helliker and Richter (2008) incorporated global tree-ring δ18Oc data into mechanistic model and then inferred that boreal tree-canopy temperature must be tremendously higher than ambient temperature to explain large observed Δleaf from their data. They also found that the inferred tree-canopy temperature throughout a large range of latitudes converges to ∼21°C. This conclusion, however, was reached based on constant εbio and pex in their inverse modeling. Later, Sternberg and Ellsworth (2011) challenged this conclusion by showing that the εbio is also temperature-dependent and increases with decreasing temperature. They heterotrophically generated cellulose from starch substrate at different temperatures and found that the εbio could be as large as 31‰ when temperature is 5°C. However, Zech et al. (2014a, b) indicated that the temperature-dependence of εbio shown in that laboratory experiment might be an artifact from a variable pex which, albeit not statistically significant (Sternberg, 2014), is temperature-dependent instead.
Here we use a global compilation of Sphagnum (peat mosses) δ18Oc data to test the hypothesis that the εbio is temperature-dependent. Sphagnum mosses are the keystone bryophyte species in peat-forming ecosystems, and these mosses are adapted to cool, waterlogged, and nutrient-poor conditions. Almost half of the boreal peatland areas are covered and built by Sphagnum mosses (Rydin and Jeglum, 2013). There have been a number of studies exploring the potential of stable isotopes in Sphagnum as proxies for peat-based paleoclimate reconstruction (e.g., Ménot-Combes et al., 2002; Daley et al., 2010; Loader et al., 2016; Granath et al., 2018; Xia et al., 2020). In general, Sphagnum mosses have many unique physiological characteristics with which their tissue δ18Oc could closely track δ18Op with an offset dictated by εbio. First, Sphagnum mosses are important peat-forming plants in bogs where moisture is predominantly derived from precipitation. Second, Sphagnum mosses have no stomata and no root systems. They maintain a simple water use strategy without the ability to control evapotranspiration. The water loss on Sphagnum surface is balanced either by new precipitation input or by capillary movement of water from below (Rydin and Jeglum, 2013; McCarter and Price, 2014). Therefore, Sphagnum mosses passively utilize water stored in surface unsaturated and oxic peat known as the acrotelm without the ability to access water at depth as vascular plants do. Third, Sphagnum mosses have the extraordinary capacity to store water and, more importantly, could protect the metabolic water from evaporation loss (Xia et al., 2020). Sphagnum have specialized large hyaline (water-filling) cells on their leaves and outer cortex of stems, and in some species these hyaline cells completely enclose photosynthetic cells (Loisel et al., 2009). Most of the water is, however, external capillary water held in pore spaces between leaves (Murray et al., 1989; Rydin and Jeglum, 2013). As a result of these multiple layers of water storage in Sphagnum mosses, the metabolic water in photosynthetic cells of Sphagnum living in local wet habitats could be isolated and weakly impacted by evaporative enrichment of 18O (Xia et al., 2020). Fourth, Sphagnum mosses are opportunist photosynthesizers, growing and synthesizing new cellulose as long as conditions allow even in near-freezing temperature. This physiological trait allows us to explore the sensitivity of εbio to air temperatures that are too low to allow the growth of vascular plants (Clymo and Hayward, 1982). In summary, the newly compiled dataset and analysis presented in this study offer a simple but effective test of the relationship between εbio and temperature, while circumventing the complex physiology of vascular plants.
Data and Methods
Sphagnum Oxygen Isotope Data
We compiled all available Sphagnum tissue δ18O measurements reported in literature, supplemented with some new measurements to fill regional gaps. The final dataset includes 785 individual δ18O measurements from 173 peatland sites (Figure 1). Key site information and references are summarized in Table 1, while raw data and metadata are in the Supplementary Dataset 1. Most of these sites are bogs from boreal and sub-arctic regions, but a few bog sites are located in the tropical and subtropical regions or the Southern Hemisphere. A few fen sites were also included in the dataset. The main Sphagnum species are the common bog species, S. magellanicum, S. fuscum, S. cuspidatum, and S. capillifolium. In some studies, the local microtopography information (such as hummock or hollow), or water table depth (WTD) for collected Sphagnum samples were available.
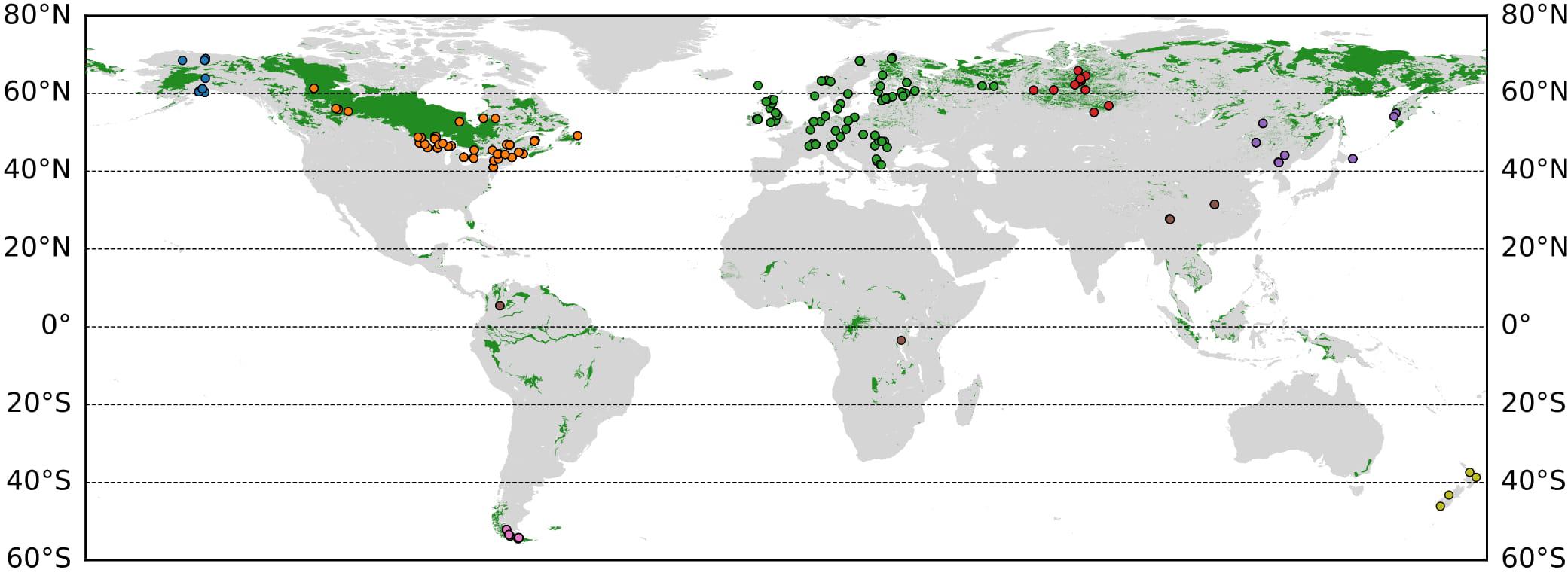
Figure 1. Global map of peatland regions (green areas; Yu et al., 2010) and sites with Sphagnum δ18Oc data presented in this study. Sites are color-coded to represent different regions: Alaska (blue), Canada and Contiguous US (orange), Europe (green), New Zealand (yellow), Patagonia (pink), Temperate East Asia (purple), Tropics and Subtropics (brown), and West Siberia (red).
The exact materials of Sphagnum mosses used for isotope analysis differ among studies and include “capitula” (the cluster of new branch leaves at the tip of moss), “capitula cellulose,” “newest 1-cm stem cellulose,” “leaf,” “leaf cellulose,” “stem cellulose,” “whole plant cellulose,” and “5-cm whole plant cellulose.” On the basis of our pilot experiments and literature data, we applied an offset correction to raw δ18O data that were measured from non-cellulose (“capitula” and “leaf”), or stems (“newest 1-cm stem cellulose” and “stem cellulose”), and re-scaled these raw δ18O data to their equivalent capitula or leaf δ18O values with the involved uncertainty propagated in the following analysis. Correction is not required for δ18O data that were measured from “whole plant cellulose” or “5-cm whole plant cellulose” because our pilot experiment (n = 6) found that leaf tissues represent 90.3 ± 5.0% (error is the standard deviation, 1σ) dry mass of Sphagnum whole plant and have a similar cellulose yield (45.6 ± 4.7%) as stem tissues (48.8 ± 8.0%). The details about our cellulose extraction method can be found in Supplementary Text 1.
Precipitation Oxygen Isotope Data and Climate Data
We used the Online Isotopes in Precipitation Calculator (OIPC; Bowen, 2020) to obtain monthly δ18Op for every Sphagnum sampling location in the dataset. In the OIPC, δ18Op at a given location is modeled based on latitude and altitude plus a residual term that is interpolated using existing δ18Op observations (Bowen et al., 2005). To our knowledge, this model provides the best estimate for the site-specific δ18Op for our synthesis as there is no on-site precipitation δ18O measurement prior to Sphagnum sample collection except at three sites. The uncertainty of OIPC is depicted as the confidence interval for mean annual δ18Op prediction and is large in regions where δ18Op observations are scarce (Bowen and Revenaugh, 2003), but the confidence interval for monthly δ18Op prediction is not available in the OIPC. In addition, the OIPC is for predicting long-term mean value for monthly and annual δ18Op at a given location but does not account for their inter-annual variability. This inter-annual variability is an important uncertainty source in determining εbio for Sphagnum as these samples were collected in different years and in some studies only their recent growth was harvested. To estimate this uncertainty, we used the archived outputs from three nudged isotope-enabled global circulation models (IsoGSM, LMDZ4, and GISS ModelE; accessible from https://data.giss.nasa.gov/swing2/swing2_mirror/; Risi et al., 2012) to calculate the multi-model mean standard deviation of monthly δ18Op for each sample location. Therefore, we used the OIPC model that had been validated against observational δ18Op data to derive the site-specific δ18Op but used the isotope-enabled global circulation models nudged on reanalysis data to estimate the uncertainty associated with its inter-annual variability (at least for 31 years). We acknowledge that the accuracy of monthly δ18Op generated in the OIPC is unknown, but we used the prediction error for mean annual δ18Op as a reference.
We used the NASA MERRA-2 monthly 2-m air temperature (T2M in M2IMNXASM; GMAO, 2015b) data to infer the relevant growth temperature for each Sphagnum sample that was collected in different year and month. The MERRA-2 is an atmospheric reanalysis product for satellite era (beginning in 1980) and has a resolution of 0.5° for latitude and 0.625° for longitude. The raw temperature data were corrected for the altitude difference between each sampling site and the corresponding MERRA-2 grid assuming a lapse rate of −0.0065°C/m. The MERRA-2 grid altitude is inferred from the constant model parameter surface geopotential height (PHIS in M2C0NXASM; GMAO, 2015a) as PHIS/g, where g is the standard gravity, 9.8 m/s2. Similarly, we also used the MERRA-2 monthly precipitation amount (PRECTOTCORR in M2TMNXFLX; GMAO, 2015c) data that were combined with OIPC δ18Op data to calculate the metrics of amount-weighted δ18Op that represent the isotopic composition of precipitation input for Sphagnum. However, the MERRA-2 products do not provide the uncertainty of reanalysis data other than the temporal variance of monthly mean.
Data Analysis
We first calculated the range and standard deviation of site-specific δ18Oc data in one-time sampling campaign to characterize the within-site variability and evaluated if measured WTD or microtopographical locations would explain the within-site δ18Oc variability. Then we calculated the apparent enrichment factor (εapp) for each δ18Oc datum as δ18Oc minus its corresponding δ18Op, with the latter value depending on the type of materials and sampling time. We also similarly determined the relevant growth temperature for each sample from the MERRA-2 dataset. For δ18Oc data derived from the recent growth of Sphagnum (capitula or the newest 1-cm stem), we used amount-weighted δ18Op of the sampling month and the previous month (δ18Op2) to pair with temperature of the sampling month (T1) for calculating the εapp. We assumed that the precipitation input in these recent 2 months was relevant to imprint the δ18Oc signal in the tissue of recent growth, but growth was only restricted to the course of recent 1 month. To evaluate the sensitivity of calculated εapp to the choice of relevant time span for δ18Op and temperature, we also used δ18Op of the recent 1 month or 3 months (amount-weighted), together with the arithmetic average temperature of the recent two or 3 months as a pair. For δ18Oc data derived from the whole plant of Sphagnum, we used the amount-weighted δ18Op of the entire recent 12 months (δ18Op12) to pair with the arithmetic average temperature of the most recent growing season defined as the months with MERRA-2 temperature above 0°C during the recent 12-month period. We assumed that precipitation input during all these months either as rainfall or snowfall were relevant to imprint the δ18Oc signal in whole plant, but growth itself was only restricted to the course of growing season. To minimize, but not necessarily eliminate the influence of evaporative enrichment that has caused the within-site variability in δ18Oc and εapp, we used the site-specific minimum δ18Oc to calculate the site-specific minimum εapp, i.e., εappmin, for each site as an approximation for εbio. The precision of εappmin was assessed using the Gaussian error propagation combining the analytical uncertainty in δ18Oc, the uncertainty related to offset correction from raw δ18O data, and the climatological uncertainty in amount-weighted δ18Op values. The accuracy of εappmin was assessed using the OIPC prediction error for mean annual δ18Op. Pairing the calculated εappmin and temperature data, we explored their relationship using a second order polynomial regression. Finally, we compared the result with previous study and discussed the implications for paleoclimate and plant physiology studies.
Results
Our compiled Sphagnum tissue δ18O data ranged from 11.5 to 26.6‰ (relative to VSMOW). The uncertainty of δ18O analysis was represented by either the long-term precision of measurements (1σ) in different laboratories or the standard deviation (1σ) of replicate measurements, which was less than 0.6 and 2.0‰, respectively. The difference in δ18O between extracted cellulose and untreated bulk tissue of the same Sphagnum material was small, and we determined the offset to be 0.69 ± 0.83‰ for capitula (error is the standard deviation, 1σ; Figure 2A). Kaislahti Tillman et al. (2010) reported that this offset is a negative value (Figure 2A), likely due to the fact that they measured Sphagnum materials from peat rather than from growing plants while untreated bulk tissues in peat probably had been contaminated by other chemical components in peat. The difference in δ18O between leaf cellulose (or capitula, which is formed by branch leaves) and stem cellulose of the same Sphagnum material was also small and well constrained in multiple studies as 0.62 ± 0.51‰ (Figure 2B). This tissue-specific offset might be due to different water pools at the site of leaf and stem cellulose synthesis (Moschen et al., 2009). The capitula medium water might be subtly more enriched in 18O than the matrix water below capitula where stem cellulose is synthesized. After offset correction, the median within-site ranges of δ18Oc data were 1.0, 1.5,1.8, and 2.3‰ for sites with two, three, four, and at least five measurements, respectively (Figure 3A). The within-site standard deviations (1σ) of δ18Oc data were consistently between 0.7 and 0.8‰, and are not influenced by the number of measurements per site (Figure 3B). There is a pattern that more sites have a positive correlation between δ18Oc and WTD (Figure 3C) and that Sphagnum collected from hummock locations have higher δ18Oc than from hollow locations (Figure 3D), but few are statistically significant.
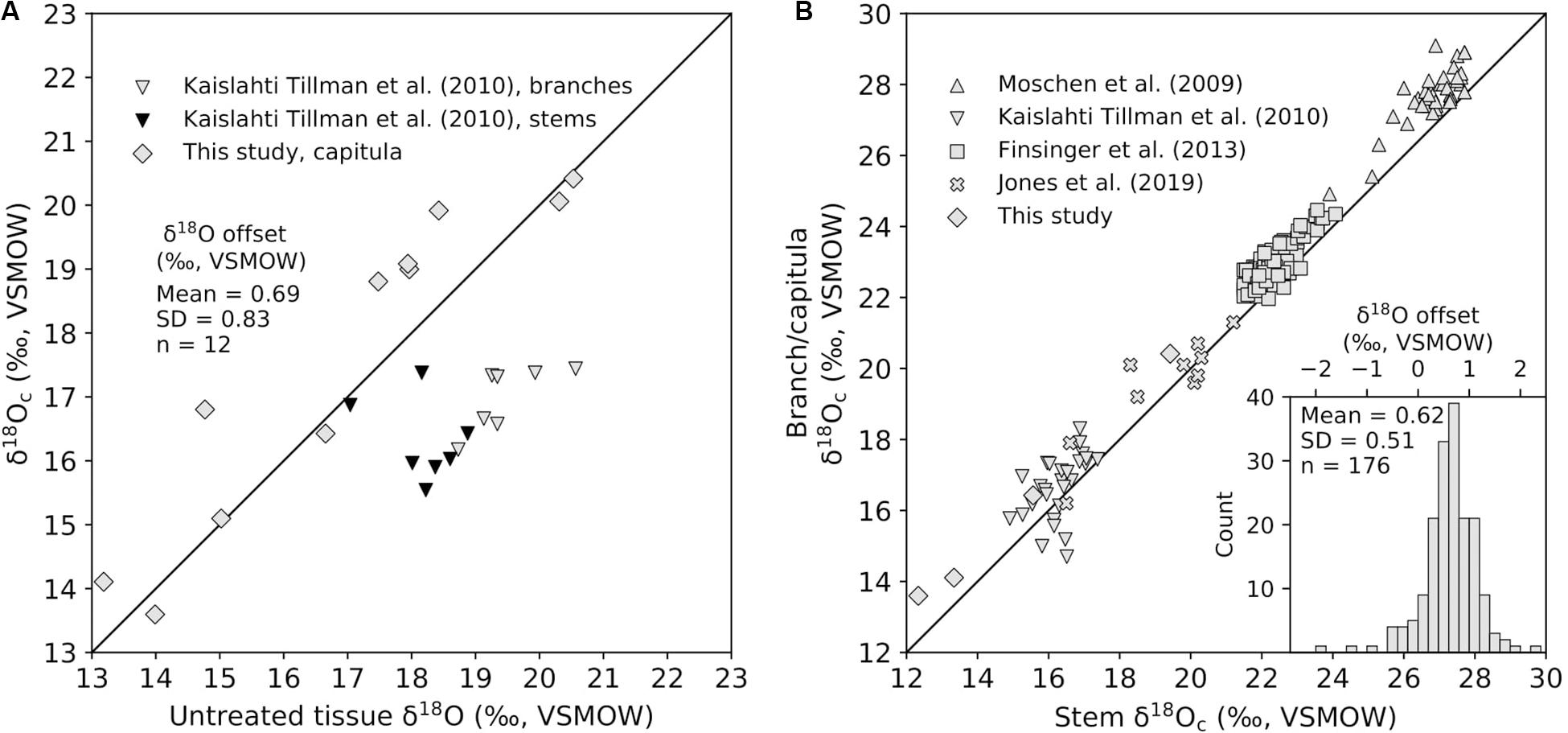
Figure 2. Oxygen isotope offset between different Sphagnum materials. (A) Biplot showing the relationship between δ18Oc and untreated bulk tissue δ18O. Data reported from Kaislahti Tillman et al. (2010) were derived from subfossil Sphagnum branches or stems in peat and are plotted below the 1:1 line, indicating that δ18Oc are lower than untreated bulk tissue δ18O in peat. Data from this study were derived from fresh capitula material and are plotted mostly above the 1:1 line, indicating that δ18Oc are higher than untreated fresh capitula δ18O. (B) Biplot showing the relationship between branch/capitula δ18Oc and corresponding stem δ18Oc. Data reported from Moschen et al. (2009), Kaislahti Tillman et al. (2010), and Finsinger et al. (2013) were derived from subfossil Sphagnum branches or stems in peat, while data from Jones et al. (2019) and this study were derived from fresh plants. The results tend to show that branch or capitula δ18Oc are higher than stem δ18Oc and the offset (branch/capitula δ18Oc minus stem δ18Oc) resembles a Gaussian distribution as shown in the inset histogram.
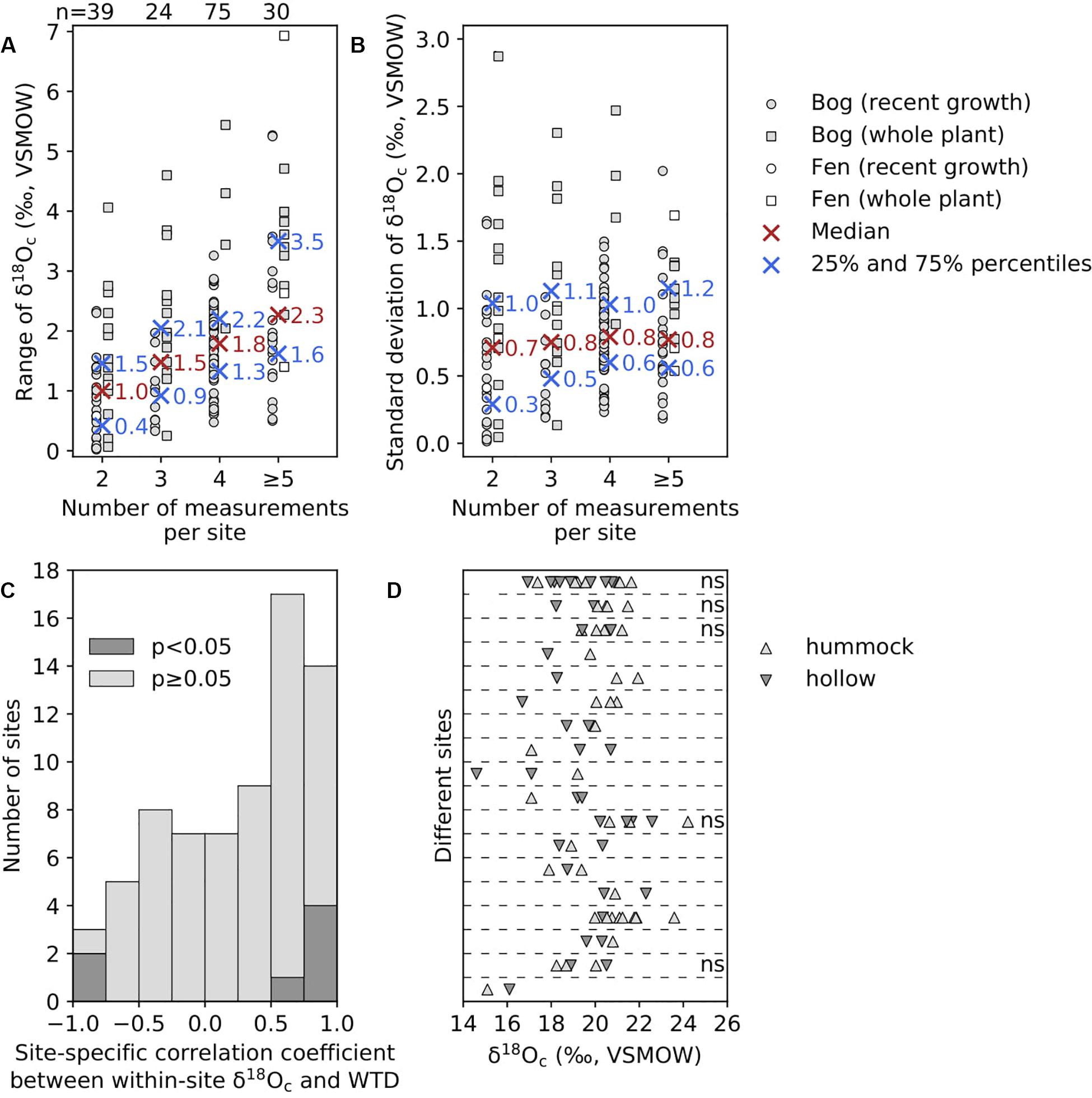
Figure 3. Within-site variability of Sphagnum δ18Oc data. (A) Range of within-site δ18Oc data for each site. (B) Standard deviation of within-site δ18Oc data for each site. In both (A,B), results are separated for the number of measurements per site. Individual site data are separated for bogs and fens and for recent growth and whole plant parts. Also shown are median and lower/upper quartile values of the range and the standard deviation as cross symbols. The numbers of sites are indicated on top of plot in (A). (C) Histogram of site-specific correlation coefficient between within-site δ18Oc and WTD data from studies by Loader et al. (2016), Granath et al. (2018), Jones et al. (2019), and Xia et al. (2020) in which WTD measurements are available and there are at least three data points. The significant correlations with p-value lower than 0.05 are shown as dark gray color. Positive correlation indicates that high δ18Oc correspond to deep WTD. (D) Within-site δ18Oc data from studies by Taylor (2008) and Brader (2013), plus a site from this study, in which samples were collected at microtopographical locations of hummock and hollow. Data from different sites are separated by horizontal lines. The “ns” denotes “not significant” difference at p < 0.05 level in Student’s t-test between hummock and hollow δ18Oc data for sites with at least two data points for both hummock and hollow.
For all data from the recent growth of Sphagnum, there was a clear trend that the εapp increases with decreasing temperature, especially when T1 is below 10°C (Figure 4A). The whole-plant data complemented the recent-growth data in the temperature range from 6 to 15°C (Figure 4B), but their εapp did not show a clear trend with temperature. In both recent-growth and whole-plant data synthesis, outliers were mainly those data collected from fens and their εapp could be either higher, or lower than the rest of dataset. Unlike bogs that are rain-fed, fens are mainly fed by groundwater and stream water that that could complicate the source water δ18O for Sphagnum. In addition, Sphagnum inhabiting fens are not under desiccation stress and could develop different physiological strategy compared to those in bogs. These fen data were thus not included in the following analysis.
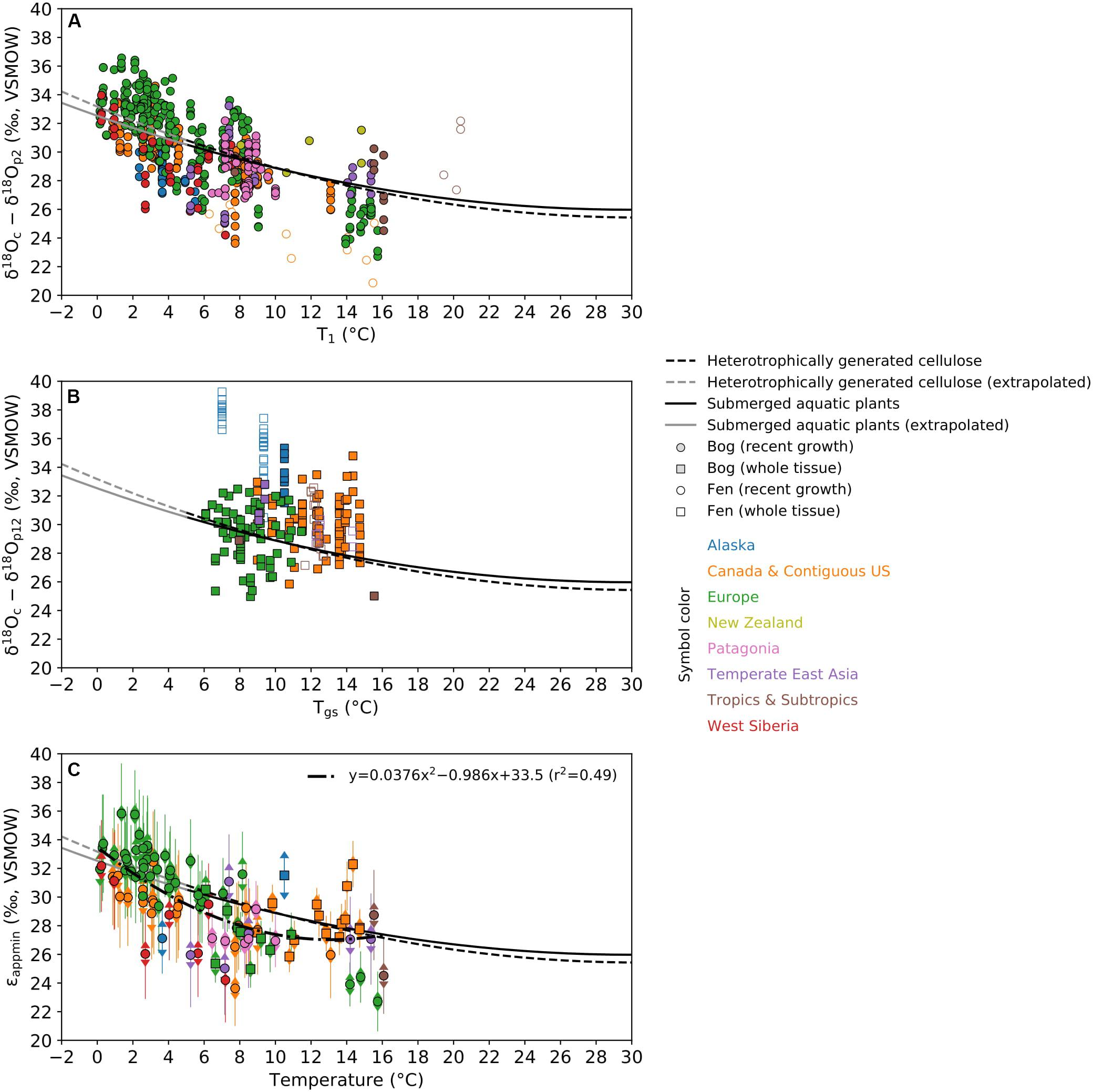
Figure 4. The relationship between εapp and temperature. Symbols are color-coded to represent different regions as in Figure 1. (A) All data for the recent growth of Sphagnum are plotted using the pair of δ18Oc–δ18Op2 and T1. (B) All data for the whole plant of Sphagnum are plotted using the pair of δ18Oc–δ18Op12 and Tgs. (C) The biplot between the site-specific εappmin and growth temperature for bog sites with at least three measurements. The 95% prediction interval of OIPC-based mean annual δ18Op at each sampling location is shown as error bar with arrow caps. The precision (2σ) of εappmin is denoted as error bar without caps. These data were fitted by a second order polynomial regression shown by the dash-dotted line. Also shown in these figures are the second order polynomial regressions fitted by data from heterotrophic generation of cellulose and data from submerged aquatic plants by Sternberg and Ellsworth (2011) as well as their extrapolations. Note that the full range of temperature (to 30°C) in laboratory experiments by Sternberg and Ellsworth (2011) is shown to illustrate that the sensitivity of εapp to temperature is very weak at high temperature.
Merging both recent-growth and whole-plant data from bogs and plotting the site-specific εappmin for sites with at least three data points versus corresponding growth temperature, the overall relationship that the εappmin increases with decreasing temperature was slightly different from the regression models by Sternberg and Ellsworth (2011; Figure 4C). The second order polynomial regression from our data indeed corroborated the trend that the increase in εappmin was steeper when temperature was lower, but the sensitivity was very weak for temperature range from 10 to 15°C. Our additional analysis indicated that this pattern was not affected by pairing different time spans of precipitation input and temperature for recent-growth dataset (Supplementary Figure 1). The εappmin data points were considerably scattering relative to the trend line, but the deviations were acceptable considering the propagated uncertainty range. Notably, the accuracy of εappmin was robust compared to their precision, except in a few cases, as shown by comparing the two types of error bars (Figure 4C).
Discussion
Within-Site Variability in Sphagnum Cellulose Oxygen Isotopes
The δ18Oc variability for a specific site reflects differential evaporative enrichment of 18O in metabolic leaf water for Sphagnum inhabiting different microtopographical locations in peatlands, as samples from the same site should receive the same precipitation input (Xia et al., 2020). This evaporation component—which could theoretically be expressed as Δleaf (1–pxpex) in Eq. (1)—has modified the δ18Op signal before the precipitation source water was incorporated into cellulose synthesis. For most sites, the δ18Oc data were not measured from the same Sphagnum species, but recent studies suggested that the species effect of leaf anatomy or physiology (biotic factor) does not directly cause the within-site δ18Oc variability (El Bilali and Patterson, 2012; Xia et al., 2020). Instead, different Sphagnum species favor different microhabitats (abiotic factor) such as drier hummocks, or wetter hollows that indirectly control the degree of evaporative enrichment of 18O in metabolic leaf water and thus the δ18Oc signal (Xia et al., 2020).
The result that most sites, if WTD data were available, displayed positive correlations between δ18Oc and WTD suggests that Sphagnum samples associated with a deeper water table would register a higher degree of evaporative enrichment, although few of these correlations were statistically significant (Figure 3C). Similar result was also found for data collected at hummock and hollow locations within a site (Figure 3D), despite that the actual WTD measurements and the size of these microtopographical features were unknown. The mechanism behind the connection between evaporative enrichment of 18O in leaf water and WTD is more complex than a simple corollary that drier habitats with deeper water table cause a higher evaporation rate of leaf water and a higher enrichment of 18O. In fact, the metabolic leaf water is technically difficult to collect as Sphagnum and other bryophytes store a large proportion of water in external capillary spaces (Dilks and Proctor, 1979). Through measuring Sphagnum water content and “bulk” leaf water δ18O, our previous study proposed that water stored in external capillary spaces of Sphagnum would evaporate first, thereby indirectly protecting internal metabolic leaf water from evaporative loss. As such, drier Sphagnum mosses would have a smaller proportion of external capillary water and as a consequence the metabolic leaf water is also subject to some degree of evaporation (Xia et al., 2020). That being said, the negative correlations between δ18Oc and WTD also existed in the dataset (Figure 3C), although in at least a few cases these negative correlations were probably an artifact from a narrow range of WTD that did not represent a well-defined moisture gradient (Supplementary Figure 2). In addition, at a few sites Sphagnum inhabiting hollow could have higher δ18Oc than hummock (Figure 3D). These opposite observations might be due to the decoupling between Sphagnum water content and WTD. Hollow-inhabiting Sphagnum have a higher collective surface area and these Sphagnum are more often susceptible to desiccation (Turetsky et al., 2008; Rydin and Jeglum, 2013) and could have a lower water content despite a shallower WTD, in particular after a sustained period of lacking precipitation. Studies that reported these data did not measure the Sphagnum water content nor had any description of their microhabitats, thus this specific hypothesis could not be directly evaluated.
Unlike vascular plants for which Δleaf is governed by leaf-air interaction through stomata and is highly dependent on relative humidity and transpiration rate (Zech et al., 2014a), there is no appropriate physiological model to mechanistically derive the Δleaf in Eq. (1) for Sphagnum mosses. Instead, the expression of Δleaf in Sphagnum should be considered in terms of the partitioning between internal and external leaf water rather than the atmospheric humidity. This water storage partitioning could explain why Sphagnum δ18Oc data are less variable at site-specific scale (Figure 3B) compared to their measured “bulk” leaf water δ18O that could be highly variable or highly enriched in 18O (Ménot-Combes et al., 2002; Price et al., 2009; Loader et al., 2016; Xia et al., 2020). This “water buffer” mechanism might be unique for Sphagnum mosses adapted to waterlogged peatlands, but not for other bryophytes (Xia et al., 2020). For example, the moss species (Polytrichum strictum and Chorisodontium aciphyllum) from aerobic moss peatbanks in the Antarctic Peninsula showed very high and highly variable δ18Oc values (Royles et al., 2013, 2016), which are only possible with an unbuffered metabolic leaf water. Due to the presence of external water buffer for Sphagnum mosses, we propose that the evaporative enrichment of 18O in their metabolic leaf water, despite being difficult to be directly measured or modeled, is a fairly small quantity compared to εbio. From a process-based understanding, the evaporative enrichment should approximate to zero for Sphagnum inhabiting very wet habitats or having persistent external water layer, in which case the metabolic leaf water is close to being completely protected by external capillary water from evaporation. For individual sites where Sphagnum δ18Oc data displayed a considerable variability, the site-specific minimum δ18Oc value represents the case that the metabolic leaf water is least modified by evaporative enrichment. Therefore, we hereby justify that the corresponding εapp, i.e., εappmin, serves as an alternative measure of εbio for each of those sites. Future studies should aim to further refine the processes in how the isotopic signals of diverse Sphagnum water pools (e.g., Ooki et al., 2018) are modified evaporatively or transferred into newly formed cellulose in controlled laboratory experiments (Price et al., 2009; Brader et al., 2010).
Uncertainty in Constraining the Time Span of Precipitation Input and Sphagnum Growth
Defining the time span of growth and precipitation input for collected Sphagnum samples presents a main challenge in refining the relationship between εapp and temperature. The whole plant of Sphagnum likely integrated the growth for the time span of entire growing season, which is best defined as those unfrozen months (above 0°C). The threshold value of 0°C has been used to define bioclimatic variables such as the photosynthetically active radiation during the growing season (Gallego-Sala et al., 2010; Loisel et al., 2012; Charman et al., 2013). We thus used the Tgs, the arithmetic average temperature of the months above 0°C, as the growth temperature for the whole plant of Sphagnum. In fact, Sphagnum growth during the months of freezing temperature does occur if snow cover was thick and persistent enough to insulate Sphagnum from winter frost (Dorrepaal et al., 2004; Genet et al., 2013), as snow cover shading is not is not a major limiting factor for Sphagnum growth (Clymo and Hayward, 1982; Küttim et al., 2020). That being said, biomass production of Sphagnum during freezing months is much reduced. Considering this, using the Tgs rather than the average temperature of every month of a year, could avoid underestimating the growth temperature for Sphagnum if the freezing season was very long or cold. However, the cumulative temperature—such that is defined as growing degree days above zero in bioclimatic studies (Charman et al., 2013)—might be a more accurate measure of the total warmth Sphagnum have experienced. Studies found that Sphagnum productivity at a given site is enhanced during the warmest season (Krebs et al., 2016; Küttim et al., 2020), although a few studies suggested that Sphagnum growth could be limited by summer desiccation stress and photoinhibition (Lindholm, 1990; Dorrepaal et al., 2004; Genet et al., 2013). Certainly, there is a tendency that Tgs would underestimate the mass-weighted growth temperature for the whole plant of Sphagnum.
Although the freezing season is not considered relevant for Sphagnum growth, the accumulation of winter precipitation as snowpack during the freezing season could be an important component of source water for Sphagnum for the following snowmelt season. Indeed, direct water sampling of peatland water table showed δ18O values close to amount-weighted mean annual δ18Op (Ménot-Combes et al., 2002; Xia et al., 2020). For δ18Oc data measured from the whole plant of Sphagnum, the amount-weighted δ18Op12, which should be the most relevant representation for precipitation input, was used to derive the εapp to pair with the Tgs in our analysis.
Unlike the whole plant, the capitula and the newest 1-cm stem only represent the recent growth of Sphagnum right before sample collection, and these tissues could register the recent input of δ18Op in cellulose. For example, Daley et al. (2010) collected Sphagnum capitula every two to three months and found that the δ18Oc signals showed variations of more than 4‰ that were in general tracking the variations of δ18Op over seasonal scale at two of three sites in northwestern Europe, but at another site the variations were less than 2.5‰, despite similar δ18Op seasonality at these locations according to the OIPC model. These observations imply that Sphagnum capitula regenerate and refresh their δ18Oc signal at bimonthly and quarterly timescales, but the rate of δ18Oc renewal for capitula appears to be variable. This isotopic renewal rate may depend on biotic factors such as Sphagnum productivity, which has been routinely determined by measuring Sphagnum stem height growth (Clymo, 1970). Field measurements indicate that stem height growth is enhanced with a higher photosynthetically active radiation and a longer growing season length (Loisel et al., 2012) but is also influenced by local ecohydrological factors (Gunnarsson, 2005; Loisel and Yu, 2013). The capitula turnover rate might be proportional to the stem increment rate as the capitula density is lower when stem growth is faster (Breeuwer et al., 2008; Loisel et al., 2012). Aldous (2002) used the ratio of number of branches per capitulum to number of branches per 1-cm of stem to normalize the turnover of capitula to stem increment. This method showed that capitula could replace themselves completely with every 1.65-cm stem increment for a case study of S. capillifolium from peat bogs in the northeastern United States (Aldous, 2002). Nevertheless, the exact time spans for the capitula replacement and the growth of newest 1-cm stem in the dataset are unknown. Other than the physical replacement, capitula δ18Oc renewal rate may also depend on abiotic factors such as the residence time of metabolic leaf water δ18O, which in principle should be governed by both the size of acrotelm water reservoir and the rate of precipitation and evaporation. The role of residence time has been proposed to explain the smaller range of along-stem δ18Oc variation compared to δ18Op variation in a case study of S. magellanicum collected from a southern Patagonian peat bog (Xia et al., 2020). The δ18Oc renewal not only influences how many months of precipitation input are relevant for Sphagnum cellulose synthesis but also the average growth temperature, which are two important but potentially site-specific metrics for our analysis on the temperature-dependence of εapp.
In our analysis of δ18Oc data measured from the recent growth of Sphagnum, we used the δ18Op2 as the precipitation input to derive the εapp that was paired with the T1 as the relevant growth temperature. This choice assumed that the sampling month temperature was the most relevant for capitula growth and the newest increment of stem, while metabolic leaf water not only derived from the precipitation of the sampling month but also partly inherited the precipitation input in the previous month. We notice that changing the definition of these time spans does not affect the overall trend between εappmin and temperature, except in the case that the relationship between εappmin and temperature became more like linear when the recent 3-month amount-weighted δ18Op was paired with the recent 3-month average temperature (Supplementary Figure 1). Another possible complexity is that Sphagnum from the colder regions could have a slower capitulum turnover or stem increment rate (Loisel et al., 2012). If so, a longer time span of both δ18Op and temperature should be used specifically for these data. However, we argue that the site-specific local ecohydrological factors are equally important to climate factors to affect Sphagnum growth. For example, the growth rate of Sphagnum in cold arctic regions with very short summer could be remarkably high (Sonesson et al., 1980). That being said, when the recent 3-month amount-weighted δ18Op and the recent 2-month average temperature were used instead for samples with T1 lower than 6°C, we found that the overall trend between εappmin and temperature was not affected (Supplementary Figure 3).
The Effect of Temperature on Biochemical Enrichment Factor
The demonstrated relationship between Sphagnum εappmin and growth temperature supports the previous finding that the εbio is temperature-dependent in wheat seedling experiment by Sternberg and Ellsworth (2011). The relationship between εbio and growth temperature in that experiment was established by assuming a known value of substrate δ18O and a constant of pex, while the second order polynomial regression based on their experimental data was almost identical to that based on a compilation of aquatic plant δ18Oc data (Sternberg and Ellsworth, 2011). From both their and our studies, the influence of temperature on εbio is mainly manifested over the range of low temperature. Another previous study showing that the εbio is insensitive to temperature (Roden and Ehleringer, 2000) could be reconciled by the fact that the growth temperature for their plants was above 20°C, at which the temperature sensitivity of εbio is too weak to be convincingly detected.
Our results imply that the temperature-dependence of εbio shown in heterotrophic generation of cellulose is not an artifact from a variable pex as previously suggested (Zech et al., 2014a, b), because we used the site-specific minimum Sphagnum δ18Oc data by which the term Δleaf was minimized and temperature-driven variation in pex, if any, was too small to introduce variation in δ18Oc in Eq. (1). The Δleaf is usually a large quantity for vascular plants with stomata and increases substantially with decreasing relative humidity (Tuthorn et al., 2014; Zech et al., 2014a). Due to the large contrast between evaporated leaf water δ18O and unevaporated stem water δ18O in vascular plants, the pex that could vary with plant types and in response to different growth conditions could play an important role in shaping the tree-ring δ18Oc (Barbour and Farquhar, 2000; Ellsworth and Sternberg, 2014; Song et al., 2014a). Controversy has arisen from determining whether the pex is dependent on temperature or not in laboratory experiments (Sternberg, 2014; Zech et al., 2014b), which in turn affects the determination of the corresponding εbio. However, our study focusing on the field-collected Sphagnum mosses has avoided the complexity brought by the pex. Finally, any variation in pex could be further dampened by the factor of px, which is less than unity for leaf tissue.
The second order polynomial regression from our dataset is slightly different from that by Sternberg and Ellsworth (2011) in that our regression line is flatter when temperature is above 10°C and steeper when temperature is below 5°C than their extrapolated line (Figure 4C). We recognize that the growth temperature we used is essentially lower than the true temperature for moss growth that only occurs in warmer daytime. By comparison, both the temperatures for seedling growth and of aquarium medium water were controlled as experimental invariables and are more comparable to the daytime temperature for terrestrial plants in field conditions. Future studies should further investigate the discrepancy between mean temperature and photosynthetic temperature for naturally growing mosses and vascular plants.
Implications for Paleoclimate and Plant Physiology Studies
Plant δ18Oc is a valuable paleoclimate proxy in a range of terrestrial climate archives, but studies employing plant δ18Oc data should incorporate the influence of temperature on εbio into the process-based proxy system model framework for interpreting proxy data (Dee et al., 2015; Keel et al., 2016; Okazaki and Yoshimura, 2019). The temperature effect might be small for Holocene-scale paleoclimate reconstruction, as temperature variability is in general less than 1°C (Liu et al., 2014). However, this effect would be important for high-latitude and alpine regions as the εbio exhibited the strongest sensitivity at low temperature and long-term temperature changes at these locations could be amplified due to various feedback mechanisms (Ballantyne et al., 2010; Feng et al., 2019). Furthermore, this temperature effect could be important in cold season, potentially resulting in varying biochemical enrichments in intra-annual tree-ring δ18Oc records that could be used to reconstruct past climate seasonality (Schubert and Jahren, 2015; Zeng et al., 2016).
Plant δ18Oc has also been used to understand the ecohydrological processes of plants such as tracing the water source for plants (Sargeant and Singer, 2016) or inversely modeling their growth temperature (Helliker and Richter, 2008; Song et al., 2011). Assuming a constant value of εbio, Helliker and Richter (2008) showed that boreal tree-canopy temperature must be substantially higher than ambient temperature. Further analysis by Helliker et al. (2018) found that their previously inferred high tree-canopy temperature for boreal biome is essentially the result of a similarly warm daytime air temperature during growing season but tree-canopy itself could not reach such a high temperature. In fact, the temperature-dependence of εbio is more important for subalpine and alpine regions where trees have a cool growing-season temperature (below 10°C) and thus a considerably elevated εbio. Indeed, existing tree-ring data from the Swiss Alps (Keel et al., 2016) and the Rocky Mountains in the western United States (Helliker et al., 2018) have shown that incorporating a temperature-dependent εbio into mechanistic modeling would produce a better fit for observed δ18Oc. Furthermore, in a study from the trans-Himalayan region (Chhumuja at an elevation of 4400 m above sea level) where growing season temperature is lower than 10°C, a tree-ring δ18Oc record was found to be best fitted with a relative humidity of 64%, despite the nearby station-observed values between 70% and 100% during the monsoon season (Brunello et al., 2019). This discrepancy could be alternatively explained by a higher εbio at a low growing-season temperature.
Summary
This study focused on Sphagnum mosses as a model plant and used an observational dataset of Sphagnum oxygen isotope measurements as a “natural experiment” to provide insights into the relationship between εbio and growth temperature. After a careful consideration of Sphagnum physiology relevant for understanding the degree of 18O enrichment in leaf water and the time span of precipitation input and plant growth, we demonstrated the temperature-dependence of εbio for Sphagnum mosses comparable to what was determined experimentally in heterotrophic generation of cellulose. Although the relationship between photosynthetic temperature for field-growing mosses and mean air temperature remains to be refined and perhaps explains the discrepancy in two regression equations, both approaches suggest that the factor of temperature becomes increasingly important to increase the εbio from the often assumed 27‰ when growth temperature is lower and thus should be considered in future paleoclimate and plant physiology studies wherever δ18Oc data are measured, in particular for trees in high-elevation areas. Additionally, but not discussed here, our data compilation further constrained the within-site variability in Sphagnum δ18Oc and implied that their δ18Oc variations in peat core might reflect past changes in evaporative enrichment possibly linked to peatland surface patterning rather than δ18Op.
Data Availability Statement
The datasets presented in this study can be found in online Supplementary Material.
Author Contributions
ZX conceived the research, performed the laboratory and data analyses, and wrote the manuscript with input from ZY. ZY provided some samples and contributed to the discussion. Both authors contributed to the article and approved the submitted version.
Funding
This study was supported by United States National Science Foundation (ERA-1502891 and DEB-1802810) and National Natural Science Foundation of China (41877458).
Conflict of Interest
The authors declare that the research was conducted in the absence of any commercial or financial relationships that could be construed as a potential conflict of interest.
Acknowledgments
We thank Yuwen Fu, Jingjing Sun, Zuo Wang, Yingfan Xia, and other for providing samples from China, and two reviewers for constructive comments.
Supplementary Material
The Supplementary Material for this article can be found online at: https://www.frontiersin.org/articles/10.3389/feart.2020.00307/full#supplementary-material
References
Aldous, A. R. (2002). Nitrogen translocation in Sphagnum mosses: effects of atmospheric nitrogen deposition. New Phytol. 156, 241–253. doi: 10.1046/j.1469-8137.2002.00518.x
Amesbury, M. J., Charman, D. J., Newnham, R. M., Loader, N. J., Goodrich, J., Royles, J., et al. (2015). Can oxygen stable isotopes be used to track precipitation moisture source in vascular plant-dominated peatlands? Earth Planet. Sci. Lett. 430, 149–159. doi: 10.1016/j.epsl.2015.08.015
Aravena, R., and Warner, B. G. (1992). Oxygen-18 composition of Sphagnum, and microenvironmental water relations. Bryologist 95, 445–448. doi: 10.2307/3243570
Aucour, A. M., Hillaire-Marcel, C., and Bonnefille, R. (1996). Oxygen isotopes in cellulose from modern and quaternary intertropical peatbogs: implications for palaeohydrology. Chem. Geol. 129, 341–359. doi: 10.1016/0009-2541(95)00179-4
Ballantyne, A. P., Greenwood, D. R., Sinninghe Damsté, J. S., Csank, A. Z., Eberle, J. J., and Rybczynski, N. (2010). Significantly warmer Arctic surface temperatures during the Pliocene indicated by multiple independent proxies. Geology 38, 603–606. doi: 10.1130/G30815.1
Barbour, M. M. (2007). Stable oxygen isotope composition of plant tissue: a review. Funct. Plant Biol. 34, 83–94. doi: 10.1071/FP06228
Barbour, M. M., and Farquhar, G. D. (2000). Relative humidity- and ABA-induced variation in carbon and oxygen isotope ratios of cotton leaves. Plant Cell Environ. 23, 473–485. doi: 10.1046/j.1365-3040.2000.00575.x
Bowen, G. J. (2020). ). The Online Isotopes in Precipitation Calculator, version 3.1. Available: http://wateriso.utah.edu/waterisotopes/pages/data_access/oipc.html (accessed January 2020).
Bowen, G. J., and Revenaugh, J. (2003). Interpolating the isotopic composition of modern meteoric precipitation. Water Resour. Res. 39:1299. doi: 10.1029/2003wr002086
Bowen, G. J., Wassenaar, L. I., and Hobson, K. A. (2005). Global application of stable hydrogen and oxygen isotopes to wildlife forensics. Oecologia 143, 337–348. doi: 10.1007/s00442-004-1813-y
Brader, A. V. (2013). Precipitation in Peatlands: The Stable Isotope Record of Sphagnum Mosses as a Proxy for Environmental Change. Doctoral dissertation, Netherlands: Vrije Universiteit, Amsterdam.
Brader, A. V., van Winden, J. F., Bohncke, S. J. P., Beets, C. J., Reichart, G.-J., and de Leeuw, J. W. (2010). Fractionation of hydrogen, oxygen and carbon isotopes in n-alkanes and cellulose of three Sphagnum species. Org. Geochem. 41, 1277–1284. doi: 10.1016/j.orggeochem.2010.09.006
Breeuwer, A., Heijmans, M. M. P. D., Robroek, B. J. M., and Berendse, F. (2008). The effect of temperature on growth and competition between Sphagnum species. Oecologia 156, 155–167. doi: 10.1007/s00442-008-0963-8
Brenninkmeijer, C. A. M., van Geel, B., and Mook, W. G. (1982). Variations in the D/H and 18O/16O ratios in cellulose extracted from a peat bog core. Earth Planet. Sci. Lett. 61, 283–290. doi: 10.1016/0012-821X(82)90059-0
Brunello, C. F., Andermann, C., Helle, G., Comiti, F., Tonon, G., Tiwari, A., et al. (2019). Hydroclimatic seasonality recorded by tree ring δ18O signature across a Himalayan altitudinal transect. Earth Planet. Sci. Lett. 518, 148–159. doi: 10.1016/j.epsl.2019.04.030
Cernusak, L. A., Farquhar, G. D., and Pate, J. S. (2005). Environmental and physiological controls over oxygen and carbon isotope composition of Tasmanian blue gum, Eucalyptus globulus. Tree Physiol. 25, 129–146. doi: 10.1093/treephys/25.2.129
Charman, D. J., Beilman, D. W., Blaauw, M., Booth, R. K., Brewer, S., Chambers, F. M., et al. (2013). Climate-related changes in peatland carbon accumulation during the last millennium. Biogeosciences 10, 929–944. doi: 10.5194/bg-10-929-2013
Clymo, R. S. (1970). The growth of Sphagnum: methods of measurement. J. Ecol. 58, 13–49. doi: 10.2307/2258168
Clymo, R. S., and Hayward, P. M. (1982). “The Ecology of Sphagnum,” in Bryophyte Ecology, ed. A. J. E. Smith (Dordrecht: Springer Netherlands), 229–289. doi: 10.1007/978-94-009-5891-3_8
Daley, T. J. (2007). Tracking Holocene Climate Change Using Peat Bog Stable Isotopes. Doctoral dissertation, University of Southampton, Southampton.
Daley, T. J., Barber, K. E., Street-Perrott, F. A., Loader, N. J., Marshall, J. D., Crowley, S. F., et al. (2010). Holocene climate variability revealed by oxygen isotope analysis of Sphagnum cellulose from Walton Moss, northern England. Quat. Sci. Rev. 29, 1590–1601. doi: 10.1016/j.quascirev.2009.09.017
Dee, S., Emile-Geay, J., Evans, M. N., Allam, A., Steig, E. J., and Thompson, D. M. (2015). PRYSM: an open-source framework for PRoxY System Modeling, with applications to oxygen-isotope systems. J. Adv. Model Earth Syst. 7, 1220–1247. doi: 10.1002/2015ms000447
DeNiro, M. J., and Epstein, S. (1981). Isotopic composition of cellulose from aquatic organisms. Geochim. Cosmochim. Acta 45, 1885–1894. doi: 10.1016/0016-7037(81)90018-1
Dilks, T. J. K., and Proctor, M. C. F. (1979). Photosynthesis, respiration and water content in bryophytes. New Phytol. 82, 97–114. doi: 10.1111/j.1469-8137.1979.tb07564.x
Dorrepaal, E., Aerts, R., Cornelissen, J. H. C., Callaghan, T. V., and Van Logtestijn, R. S. P. (2004). Summer warming and increased winter snow cover affect Sphagnum fuscum growth, structure and production in a sub-arctic bog. Glob. Change Biol. 10, 93–104. doi: 10.1111/j.1365-2486.2003.00718.x
Edwards, T. W. D., and McAndrews, J. H. (1989). Paleohydrology of a Canadian Shield lake inferred from 18O in sediment cellulose. Can. J. Earth. Sci. 26, 1850–1859. doi: 10.1139/e89-158
El Bilali, H., and Patterson, R. T. (2012). Influence of cellulose oxygen isotope variability in sub-fossil Sphagnum and plant macrofossil components on the reliability of paleoclimate records at the Mer Bleue Bog. Ottawa, Ontario, Canada. Org. Geochem. 43, 39–49. doi: 10.1016/j.orggeochem.2011.11.003
Ellsworth, P. V., and Sternberg, L. S. L. (2014). Biochemical effects of salinity on oxygen isotope fractionation during cellulose synthesis. New Phytol. 202, 784–789. doi: 10.1111/nph.12696
Feng, X., Zhao, C., D’Andrea, W. J., Liang, J., Zhou, A., and Shen, J. (2019). Temperature fluctuations during the Common Era in subtropical southwestern China inferred from brGDGTs in a remote alpine lake. Earth Planet. Sci. Lett. 510, 26–36. doi: 10.1016/j.epsl.2018.12.028
Finsinger, W., Schoning, K., Hicks, S., Lücke, A., Goslar, T., Wagner-cremer, F., et al. (2013). Climate change during the past 1000 years: a high-temporal-resolution multiproxy record from a mire in northern Finland. J. Quatern. Sci. 28, 152–164. doi: 10.1002/jqs.2598
Gallego-Sala, A. V., Clark, J. M., House, J. I., Orr, H. G., Prentice, I. C., Smith, P., et al. (2010). Bioclimatic envelope model of climate change impacts on blanket peatland distribution in Great Britain. Clim. Res. 45, 151–162. doi: 10.3354/cr00911
Genet, H., Oberbauer, S. F., Colby, S. J., Staudhammer, C. L., and Starr, G. (2013). Growth responses of Sphagnum hollows to a growing season lengthening manipulation in Alaskan Arctic tundra. Polar Biol. 36, 41–50. doi: 10.1007/s00300-012-1236-x
GMAO (2015b). MERRA-2 instM_2d_asm_Nx: 2d, Monthly Mean, Single-Level, Assimilation, Single-Level Diagnostics V5.12.4. Greenbelt, MD: GES DISC.
GMAO (2015c). MERRA-2 tavgM_2d_flx_Nx: 2d, Monthly Mean, Time-Averaged, Single-Level, Assimilation, Surface Flux Diagnostics V5.12.4. Greenbelt, MD: GES DISC.
Granath, G., Rydin, H., Baltzer, J. L., Bengtsson, F., Boncek, N., Bragazza, L., et al. (2018). Environmental and taxonomic controls of carbon and oxygen stable isotope composition in Sphagnum across broad climatic and geographic ranges. Biogeosciences 15, 5189–5202. doi: 10.5194/bg-15-5189-2018
Gunnarsson, U. (2005). Global patterns of Sphagnum productivity. J. Bryol. 27, 269–279. doi: 10.1179/174328205X70029
Helliker, B. R., and Ehleringer, J. R. (2002). Differential 18O enrichment of leaf cellulose in C3 versus C4 grasses. Funct. Plant Biol. 29, 435–442. doi: 10.1071/PP01122
Helliker, B. R., and Richter, S. L. (2008). Subtropical to boreal convergence of tree-leaf temperatures. Nature 454, 511–514. doi: 10.1038/nature07031
Helliker, B. R., Song, X., Goulden, M. L., Clark, K., Bolstad, P., Munger, J. W., et al. (2018). Assessing the interplay between canopy energy balance and photosynthesis with cellulose δ18O: large-scale patterns and independent ground-truthing. Oecologia 187, 995–1007. doi: 10.1007/s00442-018-4198-z
Hong, B., Liu, C., Lin, Q., Yasuyuki, S., Leng, X., Wang, Y., et al. (2009). Temperature evolution from the δ18O record of Hani peat, Northeast China, in the last 14000 years. Sci. China Earth Sci. 52, 952–964. doi: 10.1007/s11430-009-0086-z
Hong, Y. T., Jiang, H. B., Liu, T. S., Zhou, L. P., Beer, J., Li, H. D., et al. (2000). Response of climate to solar forcing recorded in a 6000-year δ18O time-series of Chinese peat cellulose. Holocene 10, 1–7. doi: 10.1191/095968300669856361
Jones, M. C., Anderson, L., Keller, K., Nash, B., Littell, V., Wooller, M., et al. (2019). An assessment of plant species differences on cellulose oxygen isotopes from two Kenai Peninsula, Alaska peatlands: implications for hydroclimatic reconstructions. Front. Earth Sci. 7:25. doi: 10.3389/feart.2019.00025
Kahmen, A., Sachse, D., Arndt, S. K., Tu, K. P., Farrington, H., Vitousek, P. M., et al. (2011). Cellulose δ18O is an index of leaf-to-air vapor pressure difference (VPD) in tropical plants. Proc. Natl. Acad. Sci. U.S.A. 108, 1981–1986. doi: 10.1073/pnas.1018906108
Kaislahti Tillman, P., Holzkämper, S., Kuhry, P., Sannel, A. B. K., Loader, N. J., and Robertson, I. (2010). Stable carbon and oxygen isotopes in Sphagnum fuscum peat from subarctic Canada: implications for palaeoclimate studies. Chem. Geol. 270, 216–226. doi: 10.1016/j.chemgeo.2009.12.001
Keel, S. G., Joos, F., Spahni, R., Saurer, M., Weigt, R. B., and Klesse, S. (2016). Simulating oxygen isotope ratios in tree ring cellulose using a dynamic global vegetation model. Biogeosciences 13, 3869–3886. doi: 10.5194/bg-13-3869-2016
Krebs, M., Gaudig, G., and Joosten, H. (2016). Record growth of Sphagnum papillosum in Georgia (Transcaucasus): rain frequency, temperature and microhabitat as key drivers in natural bogs. Mires Peat 18, 1–16. doi: 10.19189/MaP.2015.OMB.190
Küttim, M., Küttim, L., Ilomets, M., and Laine, A. M. (2020). Controls of Sphagnum growth and the role of winter. Ecol. Res. 35, 219–234. doi: 10.1111/1440-1703.12074
Libby, L. M., Pandolfi, L. J., Payton, P. H., Marshall, J., Becker, B., and Giertz-Sienbenlist, V. (1976). Isotopic tree thermometers. Nature 261, 284–288. doi: 10.1038/261284a0
Lin, Q., Hong, Y., Zhu, Y., Dong, L., Wang, Y., Leng, X., et al. (2001). The carbon and oxygen isotope composition of modern plants from typical peat bogs in China and its significance on the palaeoclimatic study. Bull. Mineral. Petrol. Geochem. 20, 93–97.
Lindholm, T. (1990). Growth dynamics of the peat moss Sphagnum fuscum on hummocks on a raised bog in southern Finland. Ann. Bot. Fenn. 27, 67–78.
Liu, H. T., Schäufele, R., Gong, X. Y., and Schnyder, H. (2017). The δ18O and δ2H of water in the leaf growth-and-differentiation zone of grasses is close to source water in both humid and dry atmospheres. New Phytol. 214, 1423–1431. doi: 10.1111/nph.14549
Liu, J. (2017). The Oxygen Isotope Composition of Modern Plants in Dajiuhu Peat Bog and its Climate Significance. Master’s thesis, Nanjing Normal University, Nanjing.
Liu, Z., Zhu, J., Rosenthal, Y., Zhang, X., Otto-Bliesner, B. L., Timmermann, A., et al. (2014). The Holocene temperature conundrum. Proc. Natl. Acad. Sci. U.S.A. 111, E3501–E3505. doi: 10.1073/pnas.1407229111
Loader, N. J., Street-Perrott, F. A., Mauquoy, D., Roland, T. P., van Bellen, S., Daley, T. J., et al. (2016). Measurements of hydrogen, oxygen and carbon isotope variability in Sphagnum moss along a micro-topographical gradient in a southern Patagonian peatland. J. Quatern. Sci. 31, 426–435. doi: 10.1002/jqs.2871
Loisel, J., Gallego-Sala, A. V., and Yu, Z. (2012). Global-scale pattern of peatland Sphagnum growth driven by photosynthetically active radiation and growing season length. Biogeosciences 9, 2737–2746. doi: 10.5194/bg-9-2737-2012
Loisel, J., Garneau, M., and Hélie, J. F. (2009). Modern Sphagnum δ13C signatures follow a surface moisture gradient in two boreal peat bogs, James Bay lowlands, Québec. J. Quatern. Sci. 24, 209–214. doi: 10.1002/jqs.1221
Loisel, J., and Yu, Z. (2013). Surface vegetation patterning controls carbon accumulation in peatlands. Geophys. Res. Lett. 40, 5508–5513. doi: 10.1002/grl.50744
Managave, S. R., Sheshshayee, M. S., Borgaonkar, H. P., and Ramesh, R. (2010). Past break-monsoon conditions detectable by high resolution intra-annual δ18O analysis of teak rings. Geophys. Res. Lett. 37:L05702. doi: 10.1029/2009GL041172
McCarter, C. P. R., and Price, J. S. (2014). Ecohydrology of Sphagnum moss hummocks: mechanisms of capitula water supply and simulated effects of evaporation. Ecohydrology 7, 33–44. doi: 10.1002/eco.1313
Ménot-Combes, G., Burns, S. J., and Leuenberger, M. (2002). Variations of 18O/16O in plants from temperate peat bogs (Switzerland): implications for paleoclimatic studies. Earth Planet. Sci. Lett. 202, 419–434. doi: 10.1016/S0012-821X(02)00794-X
Mora, G., and Zanazzi, A. (2017). Hydrogen isotope ratios of moss cellulose and source water in wetlands of Lake Superior, United States reveal their potential for quantitative paleoclimatic reconstructions. Chem. Geol. 468, 75–83. doi: 10.1016/j.chemgeo.2017.08.017
Moschen, R., Kühl, N., Rehberger, I., and Lücke, A. (2009). Stable carbon and oxygen isotopes in sub-fossil Sphagnum: assessment of their applicability for palaeoclimatology. Chem. Geol. 259, 262–272. doi: 10.1016/j.chemgeo.2008.11.009
Murray, K. J., Harley, P. C., Beyers, J., Walz, H., and Tenhunen, J. D. (1989). Water content effects on photosynthetic response of Sphagnum mosses from the foothills of the Philip Smith Mountains, Alaska. Oecologia 79, 244–250. doi: 10.1007/bf00388484
Okazaki, A., and Yoshimura, K. (2019). Global evaluation of proxy system models for stable water isotopes with realistic atmospheric forcing. J. Geophys. Res. Atmos. 124, 8972–8993. doi: 10.1029/2018jd029463
Ooki, S., Akagi, T., Jinno, H., Franzén, L. G., and Newton, J. (2018). Hydrological study of Lyngmossen bog, Sweden: isotopic tracers (3H, δ2H and δ18O) imply three waters with different mobilities. Quat. Sci. Rev. 199, 97–107. doi: 10.1016/j.quascirev.2018.09.014
Price, J. S., Edwards, T. W. D., Yi, Y., and Whittington, P. N. (2009). Physical and isotopic characterization of evaporation from Sphagnum moss. J. Hydrol. 369, 175–182. doi: 10.1016/j.jhydrol.2009.02.044
Richter, S. L., Johnson, A. H., Dranoff, M. M., and Taylor, K. D. (2008). Continental-scale patterns in modern wood cellulose δ18O: implications for interpreting paleo-wood cellulose δ18O. Geochim. Cosmochim. Acta 72, 2735–2743. doi: 10.1016/j.gca.2008.01.030
Risi, C., Noone, D., Worden, J., Frankenberg, C., Stiller, G., Kiefer, M., et al. (2012). Process-evaluation of tropospheric humidity simulated by general circulation models using water vapor isotopologues: 1. Comparison between models and observations. J. Geophys. Res. Atmos. 117:D05303. doi: 10.1029/2011jd016621
Roden, J. S., and Ehleringer, J. R. (2000). There is no temperature dependence of net biochemical fractionation of hydrogen and oxygen isotopes in tree-ring cellulose. Isot. Environ. Healt. S. 36, 303–317. doi: 10.1080/10256010008036389
Roden, J. S., Lin, G., and Ehleringer, J. R. (2000). A mechanistic model for interpretation of hydrogen and oxygen isotope ratios in tree-ring cellulose. Geochim. Cosmochim. Acta 64, 21–35. doi: 10.1016/S0016-7037(99)00195-7
Royles, J., Amesbury, M. J., Roland, T. P., Jones, G. D., Convey, P., Griffiths, H., et al. (2016). Moss stable isotopes (carbon-13, oxygen-18) and testate amoebae reflect environmental inputs and microclimate along a latitudinal gradient on the Antarctic Peninsula. Oecologia 181, 931–945. doi: 10.1007/s00442-016-3608-3
Royles, J., Sime, L. C., Hodgson, D. A., Convey, P., and Griffiths, H. (2013). Differing source water inputs, moderated by evaporative enrichment, determine the contrasting δ18OCELLULOSE signals in maritime Antarctic moss peat banks. J. Geophys. Res. Biogeo. 118, 184–194. doi: 10.1002/jgrg.20021
Sargeant, C. I., and Singer, M. B. (2016). Sub-annual variability in historical water source use by Mediterranean riparian trees. Ecohydrology 9, 1328–1345. doi: 10.1002/eco.1730
Schubert, B. A., and Jahren, A. H. (2015). Seasonal temperature and precipitation recorded in the intra-annual oxygen isotope pattern of meteoric water and tree-ring cellulose. Quat. Sci. Rev. 125, 1–14. doi: 10.1016/j.quascirev.2015.07.024
Sonesson, M., Persson, S., Basilier, K., and Stenström, T. A. (1980). Growth of Sphagnum riparium Ångstr. in relation to some environmental factors in the Stordalen mire. Ecol. Bull. 30, 191–207.
Song, X., Barbour, M. M., Saurer, M., and Helliker, B. R. (2011). Examining the large-scale convergence of photosynthesis-weighted tree leaf temperatures through stable oxygen isotope analysis of multiple data sets. New Phytol. 192, 912–924. doi: 10.1111/j.1469-8137.2011.03851.x
Song, X., Clark, K. S., and Helliker, B. R. (2014a). Interpreting species-specific variation in tree-ring oxygen isotope ratios among three temperate forest trees. Plant Cell Environ. 37, 2169–2182. doi: 10.1111/pce.12317
Song, X., Farquhar, G. D., Gessler, A., and Barbour, M. M. (2014b). Turnover time of the non-structural carbohydrate pool influences δ18O of leaf cellulose. Plant Cell Environ. 37, 2500–2507. doi: 10.1111/pce.12309
Sternberg, L., and Ellsworth, P. F. V. (2011). Divergent biochemical fractionation, not convergent temperature, explains cellulose oxygen isotope enrichment across latitudes. PLoS One 6:e28040. doi: 10.1371/journal.pone.0028040
Sternberg, L. D. S., and DeNiro, M. J. D. (1983). Biogeochemical implications of the isotopic equilibrium fractionation factor between the oxygen atoms of acetone and water. Geochim. Cosmochim. Acta 47, 2271–2274. doi: 10.1016/0016-7037(83)90049-2
Sternberg, L. D. S. L. (2014). Comment on “Oxygen isotope ratios (18O/16O) of hemicellulose-derived sugar biomarkers in plants, soils and sediments as paleoclimate proxy I: insight from a climate chamber experiment” by Zech et al. (2014). Geochim. Cosmochim. Acta 141, 677–679. doi: 10.1016/j.gca.2014.04.051
Sternberg, L. D. S. L., Deniro, M. J., and Savidge, R. A. (1986). Oxygen isotope exchange between metabolites and water during biochemical reactions leading to cellulose synthesis. Plant Physiol. 82, 423–427. doi: 10.1104/pp.82.2.423
Sternberg, L. S. L. (2009). Oxygen stable isotope ratios of tree-ring cellulose: the next phase of understanding. New Phytol. 181, 553–562. doi: 10.1111/j.1469-8137.2008.02661.x
Taylor, M. (2008). Continental-Scale Validation of the Temperature Signal in Oxygen Isotopes of Sphagnum Cellulose and Its Application as a Paleoclimate Proxy. Master’s thesis, University of Wyoming, Laramie, WY.
Treydte, K. S., Schleser, G. H., Helle, G., Frank, D. C., Winiger, M., Haug, G. H., et al. (2006). The twentieth century was the wettest period in northern Pakistan over the past millennium. Nature 440, 1179–1182. doi: 10.1038/nature04743
Turetsky, M. R., Crow, S. E., Evans, R. J., Vitt, D. H., and Wieder, R. K. (2008). Trade-offs in resource allocation among moss species control decomposition in boreal peatlands. J. Ecol. 96, 1297–1305. doi: 10.1111/j.1365-2745.2008.01438.x
Tuthorn, M., Zech, M., Ruppenthal, M., Oelmann, Y., Kahmen, A., Valle, H. F. D., et al. (2014). Oxygen isotope ratios (18O/16O) of hemicellulose-derived sugar biomarkers in plants, soils and sediments as paleoclimate proxy II: insight from a climate transect study. Geochim. Cosmochim. Acta 126, 624–634. doi: 10.1016/j.gca.2013.11.002
Waterhouse, J. S., Cheng, S., Juchelka, D., Loader, N. J., McCarroll, D., Switsur, V. R., et al. (2013). Position-specific measurement of oxygen isotope ratios in cellulose: isotopic exchange during heterotrophic cellulose synthesis. Geochim. Cosmochim. Acta 112, 178–191. doi: 10.1016/j.gca.2013.02.021
Xia, Z., Zheng, Y., Stelling, J. M., Loisel, J., Huang, Y., and Yu, Z. (2020). Environmental controls on the carbon and water (H and O) isotopes in peatland Sphagnum mosses. Geochim. Cosmochim. Acta 277, 265–284. doi: 10.1016/j.gca.2020.03.034
Yakir, D., and DeNiro, M. J. (1990). Oxygen and hydrogen isotope fractionation during cellulose metabolism in Lemna gibba L. Plant Physiol. 93, 325–332. doi: 10.1104/pp.93.1.325
Yu, Z., Loisel, J., Brosseau, D. P., Beilman, D. W., and Hunt, S. J. (2010). Global peatland dynamics since the Last Glacial Maximum. Geophys. Res. Lett. 37:L13402. doi: 10.1029/2010GL043584
Zanazzi, A., and Mora, G. (2005). Paleoclimatic implications of the relationship between oxygen isotope ratios of moss cellulose and source water in wetlands of Lake Superior. Chem. Geol. 222, 281–291. doi: 10.1016/j.chemgeo.2005.08.006
Zech, M., Mayr, C., Tuthorn, M., Leiber-Sauheitl, K., and Glaser, B. (2014a). Oxygen isotope ratios (18O/16O) of hemicellulose-derived sugar biomarkers in plants, soils and sediments as paleoclimate proxy I: insight from a climate chamber experiment. Geochim. Cosmochim. Acta 126, 614–623. doi: 10.1016/j.gca.2013.10.048
Zech, M., Mayr, C., Tuthorn, M., Leiber-Sauheitl, K., and Glaser, B. (2014b). Reply to the comment of Sternberg on “Zech et al. (2014) Oxygen isotope ratios (18O/16O) of hemicellulose-derived sugar biomarkers in plants, soils and sediments as paleoclimate proxy I: insight from a climate chamber experiment. GCA 126, 614–623.”. Geochim. Cosmochim. Acta 141, 680–682. doi: 10.1016/j.gca.2014.05.048
Zeng, X., Liu, X., Evans, M. N., Wang, W., An, W., Xu, G., et al. (2016). Seasonal incursion of Indian Monsoon humidity and precipitation into the southeastern Qinghai–Tibetan plateau inferred from tree ring δ18O values with intra-seasonal resolution. Earth Planet. Sci. Lett. 443, 9–19. doi: 10.1016/j.epsl.2016.03.011
Keywords: Sphagnum, cellulose, oxygen isotopes, biochemical fractionation, temperature, peat bogs
Citation: Xia Z and Yu Z (2020) Temperature-Dependent Oxygen Isotope Fractionation in Plant Cellulose Biosynthesis Revealed by a Global Dataset of Peat Mosses. Front. Earth Sci. 8:307. doi: 10.3389/feart.2020.00307
Received: 04 April 2020; Accepted: 29 June 2020;
Published: 24 July 2020.
Edited by:
Alexandra V. Turchyn, University of Cambridge, United KingdomReviewed by:
Patrick Z. Ellsworth, USDA-ARS, United StatesRegina Theresia Hirl, Technical University of Munich, Germany
Copyright © 2020 Xia and Yu. This is an open-access article distributed under the terms of the Creative Commons Attribution License (CC BY). The use, distribution or reproduction in other forums is permitted, provided the original author(s) and the copyright owner(s) are credited and that the original publication in this journal is cited, in accordance with accepted academic practice. No use, distribution or reproduction is permitted which does not comply with these terms.
*Correspondence: Zhengyu Xia, emh4MjE1QGxlaGlnaC5lZHU=