- 1Inter Disciplinary Program (IDP) in Climate Studies, Indian Institute of Technology Bombay, Mumbai, India
- 2TCS Innovation Labs, Tata Consultancy Services Limited, Mumbai, India
- 3Hydro-Remote Sensing Applications (H-RSA) Group, Department of Civil Engineering, Indian Institute of Technology Bombay, Mumbai, India
Disappearance of mountain glaciers and formation/expansion of glacial lakes are among the most distinguishable and dynamic impacts of climate warming in the Himalayas. The present research focuses on the identification of potential sites for future lake formation in the 65 selected study glaciers of Chandra basin located in the western Himalayas. The study adopted stress-driven, physics-based GlabTop2_IITB model to obtain the ice-thickness distribution, which was then used to extract the bedrock topography. Based on the overdeepenings determined from the derived glacier bed topographies, a total of 350 potential future glacial lakes (PFGLs) were identified, and a detailed high-resolution database of these lakes was generated. The identified PFGLs were found to occupy an area of 49.56 km2, corresponding to 8.4% of the current total area of the 65 study glaciers (591 km2). The total storage volume of these PFGLs was estimated as 1.08 ± 0.16 km3. In our study region, 20 PFGLs were identified as potentially hazardous in the event of glacial lake outburst flood occurrence, and their combined storage volume was found to be more than 106 m3, which calls for continuous monitoring of the glaciers in this region.
Introduction
The Hindu Kush Himalaya (HKH) region covering a glacierized area of about 40,000 km2 (Bolch et al., 2012) is a huge source of freshwater to the major river systems in Asia. During the twentieth century, the temperature trend profiles of the northwest and western Himalayan regions of HKH have already shown significant warming (Bhutiyani et al., 2007; Dash et al., 2007). The annual mean temperature over the whole HKH region increased at a rate of 0.21 ± 0.08°C/decade with the maximum warming of 0.26 ± 0.09°C/decade localized over the western Himalayas (Gautam et al., 2010). In the recent decades, owing to increasing climatic warming, glaciers in many parts of the Himalayan region are thinning and retreating at high rates (Bolch et al., 2012). These changes could influence the development of unstable future glacial lakes at different locations of a glacier (Quincey et al., 2007; Frey et al., 2010).
Several studies covering various parts of the globe, including the HKH region, have attempted to detect and monitor the existing glacial lakes using remote sensing techniques (e.g., Huggel et al., 2002; Kääb et al., 2005; Quincey et al., 2007; Allen et al., 2009; Gardelle et al., 2011; Jain et al., 2012; Li and Sheng, 2012; Raj et al., 2012; Xin et al., 2012; Worni et al., 2013; Zhang et al., 2015; Nie et al., 2017) as well as through field investigations (e.g., Richardson and Reynolds, 2000; Haeberli et al., 2001). These studies have provided a critical database of existing glacial lakes. However, under the present climatic warming scenario, knowledge of potential sites of future lake formation is also essential to government agencies for preparing mitigation policies. The glacial lake outburst flood (GLOF) events originating from such proglacial lakes could be hazardous to the population and infrastructure situated in the valleys below (e.g., Vuichard and Zimmermann, 1987; Xu, 1988; Das et al., 2015; Allen et al., 2016a, b).
Among the currently available studies on identification of potential sites of future glacial lakes in any region, a detailed inventory of glacial lakes was first compiled by Reynolds (2000) for Bhutan, in the context of developing hydropower stations. Using a set of topographic maps as well as panchromatic and color SPOT images of the entire Bhutan, the study explored the formation of existing lakes on debris-covered glaciers and also provided insights into location of future lakes. In this study, a 2° slope gradient was identified as the critical threshold for formation of large supraglacial lakes on the debris-covered glaciers under negative mass balance condition in the Himalayas.
Quincey et al. (2007) identified the sites of supracglacial lake formation in the Nepali and Tibetan glaciers having a history of catastrophic outburst floods. These sites were determined based on a combined analysis of glacier surface velocity obtained from ERS-1/2 satellite data and slope gradient extracted from SPOT-5 high-resolution digital elevation model (DEM). The study highlighted that low flow velocity regions mainly constitute the potential sites for supraglacial lake formation. Further, the study found a strong correlation between glacier surface gradients below 2° and presence of large supraglacial lakes, which agrees with the conclusions of Reynolds (2000).
To date, only limited studies (Frey et al., 2010; Allen et al., 2016a; Linsbauer et al., 2016; Zhang et al., 2019) are available for detecting sites of potential future lake formation based on models and methods using remote sensing inputs. Among them, Frey et al. (2010) presented a three-level strategy for identifying the overdeepened parts of a glacier bed and thereby the sites of potential future lake formation. The first two levels of strategies are for preliminary and qualitative identification. The third level is for quantitative estimation of glacier bed topography using modeled ice-thickness distribution. This strategy was demonstrated for the Swiss Alps using high-quality DEM and historical maps, and the overdeepenings were identified based on ice thickness obtained from the Shallow-Ice Approximation (SIA)–based GlabTop model proposed by Linsbauer et al. (2009).
Linsbauer et al. (2016) investigated the formation of potential future lakes in the HKH region by modeling the bed topographies of about 28,000 glaciers (covering 40,775 km2) and producing DEMs “without glaciers.” These DEMs were produced using GlabTop2 model (Frey et al., 2014), wherein the labor-intensive manual digitization of flow lines was replaced with a fully automated process. The study identified about 16,000 overdeepenings that together account for about 5% of the current glacierized area and estimated the total volume of future lakes as 120 km3. Further, the study underlined the difficulties in accurately estimating the shape of overdeepenings due to limitations in model parameterizations and input data. However, the future appearance and location of these overdeepenings under the condition of continued glacier retreat were stated to be robust. Though this study provided information on the potential future lakes in the HKH region, the results were not validated.
Allen et al. (2016a) studied the current and future potential for GLOF across the Indian Himalayan state of Himachal Pradesh, and analyzed the flood impact on downstream areas and the societal vulnerability to GLOF disasters. The existing glacial lakes in the area were identified using Landsat 8 imagery, while the future glacial lakes expected to form due to glacier retreat were modeled using GlabTop2. However, the coarser resolution ASTER GDEM (30 m) and non-optimal model parameterization adopted in the study caused high uncertainty in glacier ice-thickness estimates and the corresponding bed topographies.
More recently, Zhang et al. (2019) conducted a study in the Poiqu River basin of the central Himalayas to identify the sites where new lakes might emerge and existing lakes could expand with projected glacial recession, following Linsbauer et al. (2016). In this study, ice thickness was modeled using the original version of the GlabTop model developed by Linsbauer et al. (2012), with the high-resolution TanDEM-X DEM as input. However, no glacier-specific model parameterization was implemented in the GlabTop model.
Among the studies discussed earlier (Frey et al., 2010; Allen et al., 2016a; Linsbauer et al., 2016), none except Zhang et al. (2019) has used a high-resolution DEM in GlabTop-based ice-thickness modeling. Further, glacier-wise shape factor parameterization has never been implemented in the modeling. Such shortcomings in these studies could give rise to higher uncertainties in the modeled glacier ice-thickness distribution and bed topographies, as reported in Ramsankaran et al. (2018). Moreover, to date, the robustness of the methods adopted for identifying overdeepening sites of future lake development and expansion of existing lakes in the Himalayas has not been validated.
In view of the aforementioned, the present research aims to provide a comprehensive, basin-scale, high-resolution database on potential future glacial lake sites in 65 selected glaciers of Chandra basin in the western Himalayas using high-resolution glacier bed topography. Here, the bedrock topography of each study glacier was extracted from our earlier study (Pandit and Ramsankaran, 2020), where the GlabTop2_IITB ice-thickness model was implemented with a high-resolution TanDEM-X DEM and glacier-specific optimal model parameterization. The western Himalayan region was specifically chosen as it has been experiencing more warming as compared with other HKH regions in recent decades (Gautam et al., 2010), and hence, requires more attention in the context of future lake formation under changing climate.
Study Area
This study was performed over 65 selected glaciers of Chandra basin located in the Lahaul-Spiti valley of Himachal Pradesh, India. The location map of Chandra basin is shown in Figure 1. In this research, glaciers less than 0.5 km2 were not considered because smaller glaciers are generally associated with large uncertainties in area estimates due to difficulty in glacier outline delineation. Chandra basin has a total area of 2381 km2 (Pandey et al., 2017) and ranges from 2400 to 6400 m a.s.l. The glaciers in this basin feed the Chandra River, a major tributary of the Chenab river system. The basin falls in the monsoon-arid transition zone and is alternately influenced by Indian Summer Monsoon during summer and mid-latitude westerlies during winter (Wagnon et al., 2007; Bookhagen and Burbank, 2010). High wet precipitation is recorded in the summer season (July–September) whereas winter season (November–February) experiences a significant amount of solid precipitation due to the influence of the westerlies (Sharma et al., 2013). This area is characterized by relatively cold temperatures (mean annual temperature of about 9°C) and heavy and dry snowfall (mean snowfall of about 5000 mm/year) with strong wind action (Sharma and Ganju, 2000; Negi et al., 2013).
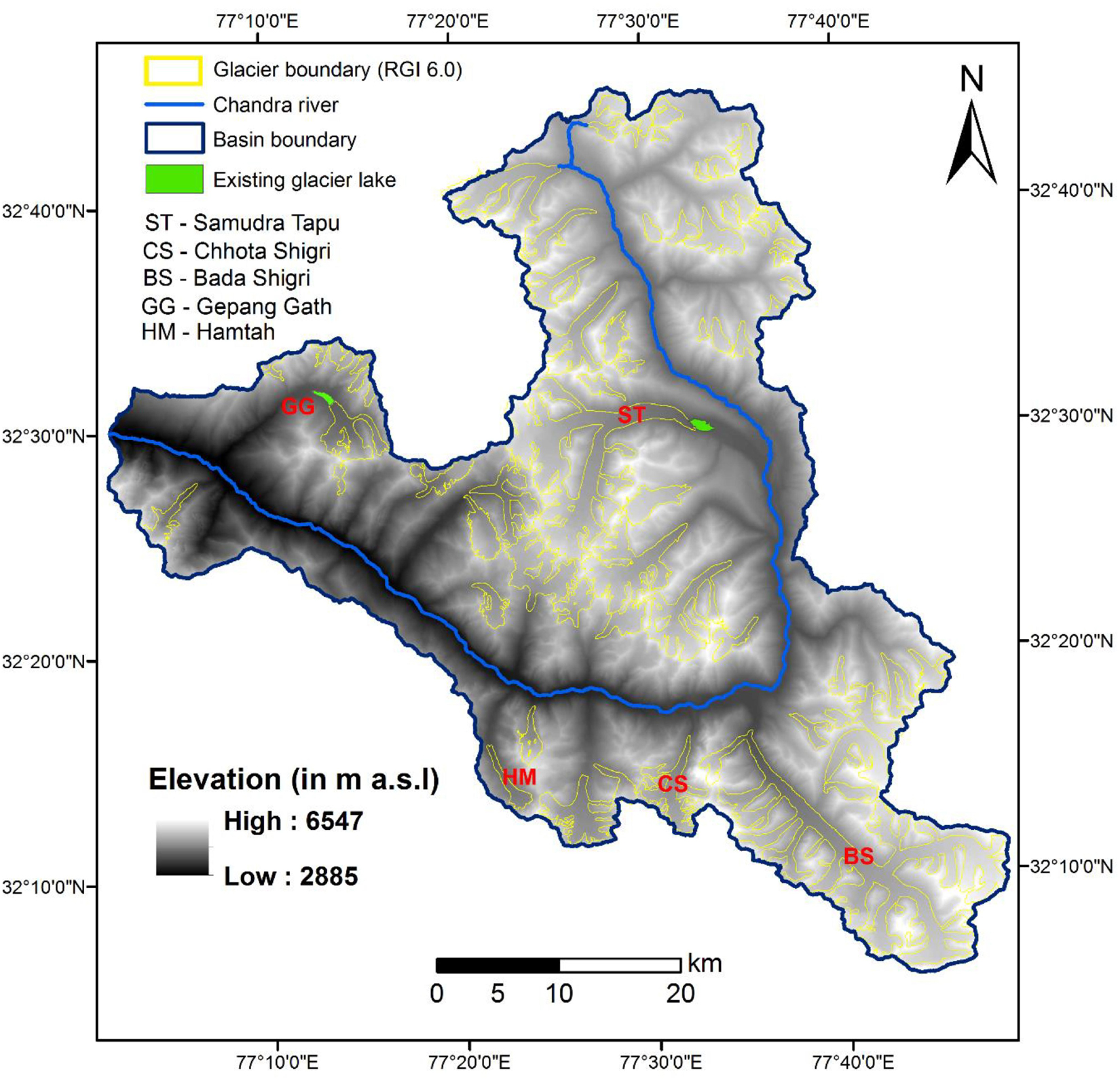
Figure 1. Map of the study area showing locations of the 65 study glaciers in Chandra river basin, Lahaul-Spiti valley, Himachal Pradesh, India. The background image is TDX DEM (10 m resolution) generated using SAR interferometry technique.
Dataset Description
A brief description of the datasets used in this research such as the satellite remote sensing data and in situ data is provided in the following subsections.
Glacier Boundary
For this research, the boundaries of all 65 study glaciers (>0.5 km2) were obtained from Randolph Glacier Inventory (RGI 6.0)1. Because these boundary shapefiles belong to the year 2002, minor corrections were made using high-resolution Google Earth and Landsat-8 images of the years 2014 and 2015.
Elevation From TanDEM-X and SRTM
For the present research, high-resolution TerraSAR-X Add-on for Digital Elevation Measurements (TanDEM-X) satellite data was used for DEM generation. This raw satellite dataset was obtained under the science proposal XTI_GLAC7043 project. Specifications of each scene are given in Table 1.
Using the SAR interferometry technique available in SARScape software, a 10 × 10 m resolution DEM (referred as TDX DEM) was generated for all 65 study glaciers (Figure 1). The DEM generation process adopted here is the same as in Pandit et al. (2014), which involves interferogram generation, adaptive filter and coherence generation, phase unwrapping, and phase-to-height conversion. The overall root mean square error obtained in TDX DEM for the Chhota Shigri Glacier was 7.41 m (Ramsankaran et al., 2018) by comparing with differential global positioning system elevation measurements. For more details about the validation exercise, readers can refer to Ramsankaran et al. (2018).
Other Datasets
For validating and analyzing the results on future glacial lake outcomes based on past data, two datasets of the year 2000 pertaining to Gepang Gath and Samudra Tapu Glaciers were used for ice-thickness modeling and bottom topography extraction. They were (1) the 30 m C-band Shuttle Radar Topography Mission (SRTM) DEM of February 2000 (obtained from USGS Earth Explorer) and (2) the digitized glacier boundary (extracted using high-resolution Google Earth and Landsat-7 data of October 15, 2000; path 147 and row 37). Likewise, to estimate long-term average equilibrium line altitude (ELA) for these two glaciers, we adopted the approach given by Chandrasekharan et al. (2018). For this purpose, appropriate images, i.e., only cloud-free images, in the ablation season to the beginning of accumulation season (mid-June to mid-October) from the Landsat series, Sentinel, and Indian Remote Sensing Satellite (IRS) series archives between 1989 and 2018 were considered.
Methodology
The sites of potential future lake formation can be identified by detecting overdeepenings in a glacier bed. Figures 2A,B illustrates the formation of glacial lakes at a location due to glacier melt. Glaciers with a considerable amount of erosive power (due to steep slopes) form large depressions in the bed as shown in Figure 2A. In the future, when the glacier disappears (as shown in Figure 2B), such overdeepened parts of the glacier bed will be exposed and filled with meltwater (Figure 2B) as well as with some morainic materials, thus forming a proglacial lake (Boulton, 1967; Clague and Evans, 1994). GLOF events may be triggered in these proglacial lakes due to external factors such as ice avalanches, rockfall, heavy rainfall, and cloud burst.
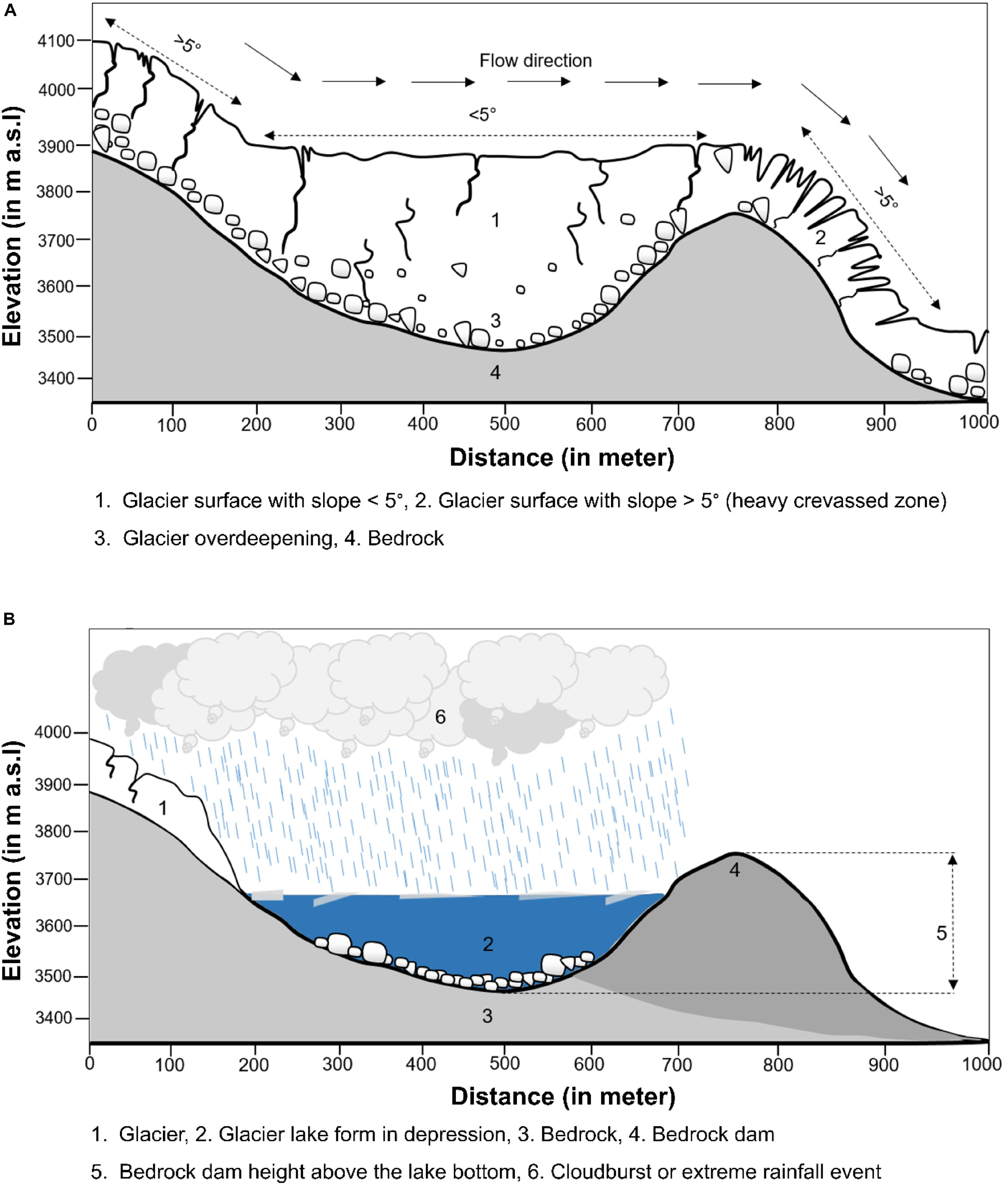
Figure 2. (A) Illustration of a glacier with an overdeepening formed due to glacier flow. (B) Glacier disappearance and formation of the future glacial lake.
In this study, for deriving bed topography profiles of the 65 selected study glaciers, we adopted the ice-thickness distribution obtained by Pandit and Ramsankaran (2020), which was estimated using the GlabTop2_IITB version model (referred to as the GlabTop2 model in Ramsankaran et al., 2018). The GlabTop2_IITB model is fully automated and requires only DEM, slope, and glacier outlines as model inputs. It is an independent implementation of the original GlabTop2 model with minor modifications as reported in Pandit and Ramsankaran (2020). This model is fundamentally based on the physical concept of SIA wherein glacier-specific basal shear stress is estimated from glacier elevation range following Haeberli and Hoelzle (1995) and then related with glacier slope and thickness. Ramsankaran et al. (2018) reported that shape factor is the only sensitive parameter in the GlabTop2_IITB model and recommended adopting glacier-specific shape factors. For estimating the near-optimal shape factor of a particular glacier, a new self-calibration approach was introduced by Ramsankaran et al. (2018), which is independent of ground observations of ice thickness. For implementing this approach, the model was allowed to run multiple times by varying shape factor values ranging between 0.6 and 0.9 (at an interval of 0.01) using TDX DEM as input. Based on the 31 obtained ice-thickness simulations, spatially distributed average ice thickness was calculated. Subsequently, the shape factors for different cross-sections located at various contours were estimated using Eq. 1 as follows. This equation was adopted from Nye (1965), where f is defined as a function of glacier width (w) and average ice thickness (hc) at the intersect of the central flow line and the transverse cross-section under consideration.
Then based on the shape factor thus derived at each cross-section, the glacier-wide average shape factor (considered as near-optimal shape factor) was calculated by simple averaging. Further details of the implementation and validation of the GlabTop2_IITB model along with the near-optimal shape factor estimation method carried out for Chhota Shigri Glacier can be obtained from our previous study (Ramsankaran et al., 2018).
Using this optimal-model parameterization approach, GlabTop2_IITB model was implemented to estimate ice-thickness distribution of the 65 study glaciers in Pandit and Ramsankaran (2020). Based on the estimated ice thickness and available DEMs, the glacier bed topographies of all study glaciers were derived, and quantitative information such as area, volume, maximum depth, and mean depth of the potential future lakes were determined. The depth distribution of the identified potential future glacial lakes (PFGLs) was extracted from the respective glacier bed topography assuming the overdeepenings to be filled with water.
Uncertainty Analysis
In this study, the uncertainty in future lake volume was estimated using Eq. 2. Here, V is the volume of a glacier, ΔV is the uncertainty in the volume, A is glacier area, ΔA is the uncertainty in glacier area, hf is the thickness of the glacier at specific f, and Δhf is the uncertainty in the estimated ice thickness/depth of overdeepenings.
Uncertainty in the ice thickness is retrieved from Ramsankaran et al. (2018). Because the future lake depth was extracted by differencing the ice thickness and surface elevation, the same uncertainty of ± 14% was assumed in the depth of overdeepenings neglecting the thickness of deposited materials. When lake area was extracted, often a few pixels are found disconnected from the main lake. These pixels were considered as uncertainty in the lake identification, which corresponds to almost 5% of the total area of the lakes.
It is important to note that many other processes can induce uncertainty in volume estimations of potential future lakes, such as, for instance, deposit of materials in the overdeepenings. However, as quantifying the amount of material getting deposited at the bottom of future lakes is difficult, the associated uncertainty was not considered in the analysis.
Results and Discussion
This section is divided into four subsections. The first subsection discusses the sites of PFGLs identified in the Gepang Gath Glacier. The second subsection deals with the qualitative validation exercise undertaken to determine whether the formation/expansion of lakes in the Gepang Gath Glacier at present could have been anticipated in the past. The third subsection reports the sites of potential future lakes in the Samudra Tapu Glacier. The final subsection provides a comprehensive database of PFGLs identified in all 65 study glaciers of Chandra basin. To facilitate easier identification, each glacial lake was uniquely labeled in the format “xx_lake_yy,” where xx and yy represent glacier ID and lake number, respectively. The location of all study glaciers is provided in Supplementary Table A1.
Potential Future Lake Sites in the Gepang Gath Glacier
Figures 3A–C illustrates the modeled surface and bed topographic profiles along the central flow line of the right tributary, left tributary, and the main trunk (Figure 3D) of the Gepang Gath Glacier. These results clearly show the overdeepenings on the glacier bed surface. In Figure 3C, a relatively large overdeepening is observed in the bed topography of the glacier until 4000 m from the snout between the surface elevations 4100 and 4300 m a.s.l. This observed overdeepening may combine with the proglacial lake present adjacent to the glacier snout and give rise to a larger proglacial lake in the future, if the current glacier retreat trend persists. Note that these overdeepenings are observed at different elevations in the left/right tributaries and the main trunk of the glacier. Distinct overdeepenings are observed at the surface elevation of about 4400 and 4600 m a.s.l. for the right tributary (Figure 3A). On the other hand, for the left tributary, overdeepening is observed at around 4400 m a.s.l. (Figure 3B). All the overdeepenings observed are below the long-term average ELA of the Gepang Gath Glacier, which is at around a surface elevation of 4700 m a.s.l. It shall be noted that the glacier region above the ELA is smaller in area and steeper than other regions, thus preventing the formation of overdeepenings on the glacier bed.
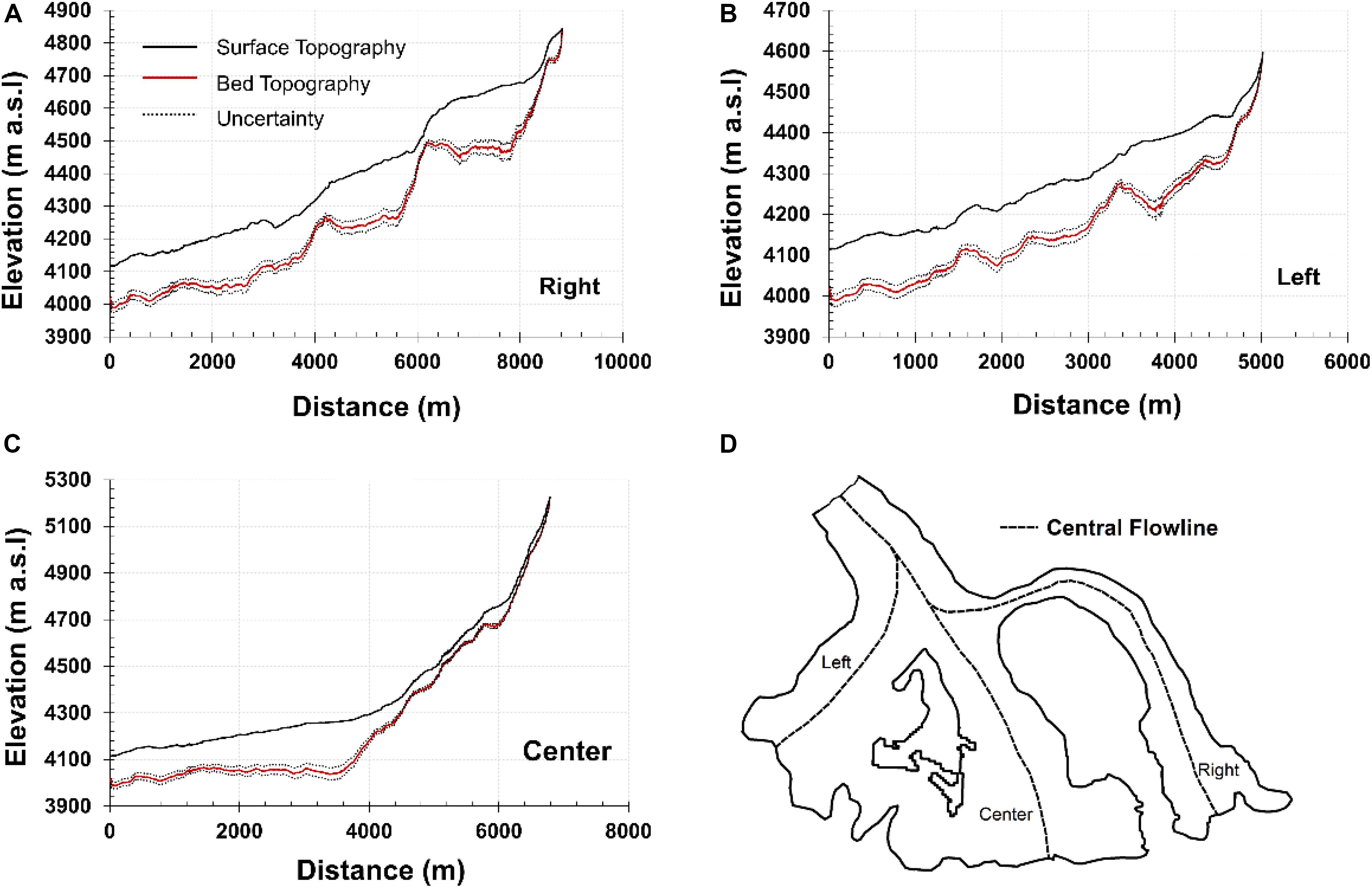
Figure 3. Glacier surface and bed topographic profiles of the Gepang Gath Glacier along the central flowline of (A) right tributary, (B) left tributary, (C) the main trunk, and (D) glacier boundary demarcated with left and right trunk of the glacier. The location of confluence of all three parts of the glacier (i.e., A–C) is between 4200 and 4300 m a.s.l.
Figure 4 illustrates the spatial distribution of the sites identified as potential future glacier lakes (PFGLs). In total, seven PFGL sites situated at different elevations ranging from 4100 to 4700 m a.s.l. were identified in the Gepang Gath Glacier (Table 2).
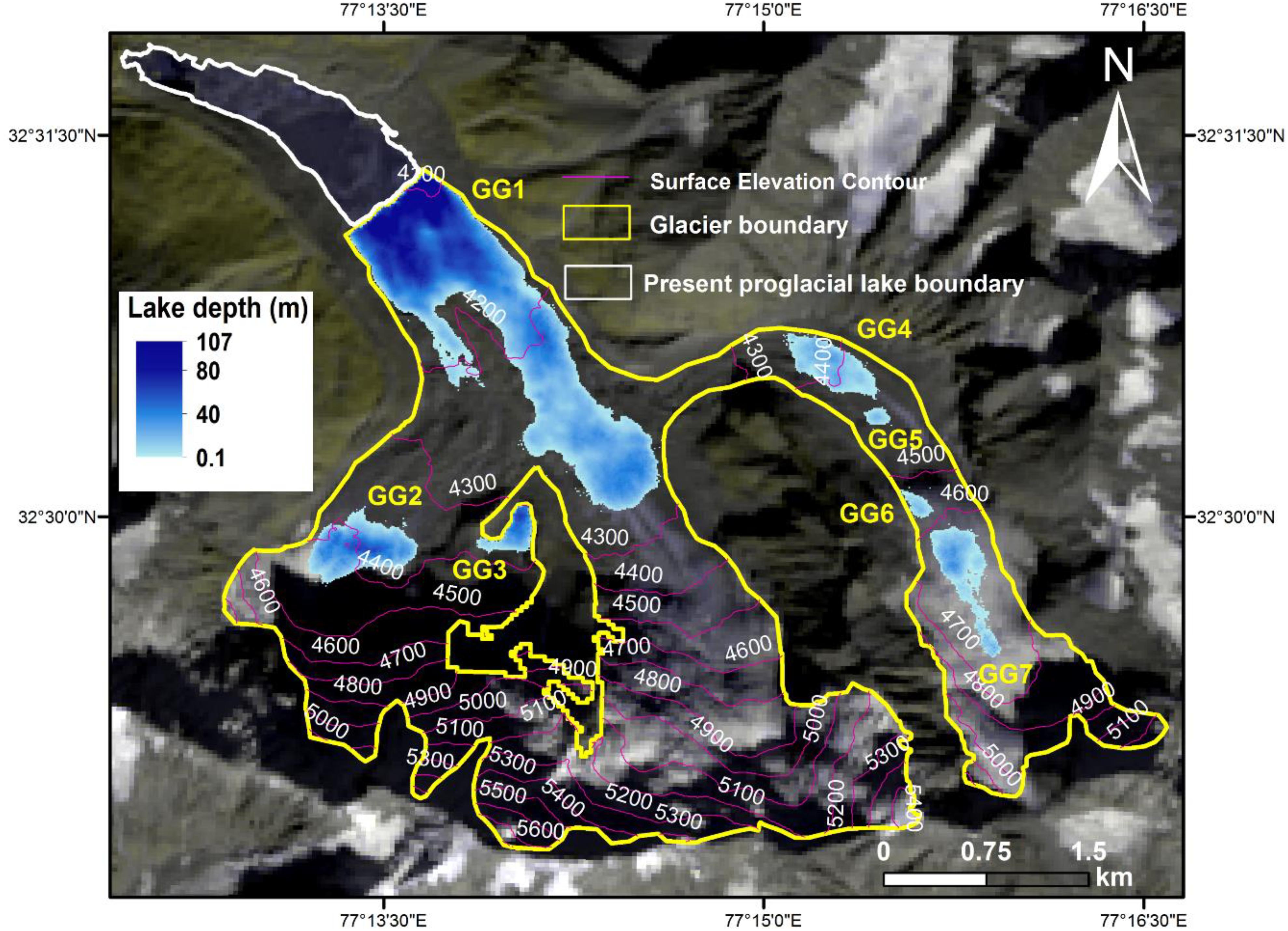
Figure 4. Spatial distribution of the potential sites for future lake formation in the Gepang Gath Glacier obtained based on TDX DEM.
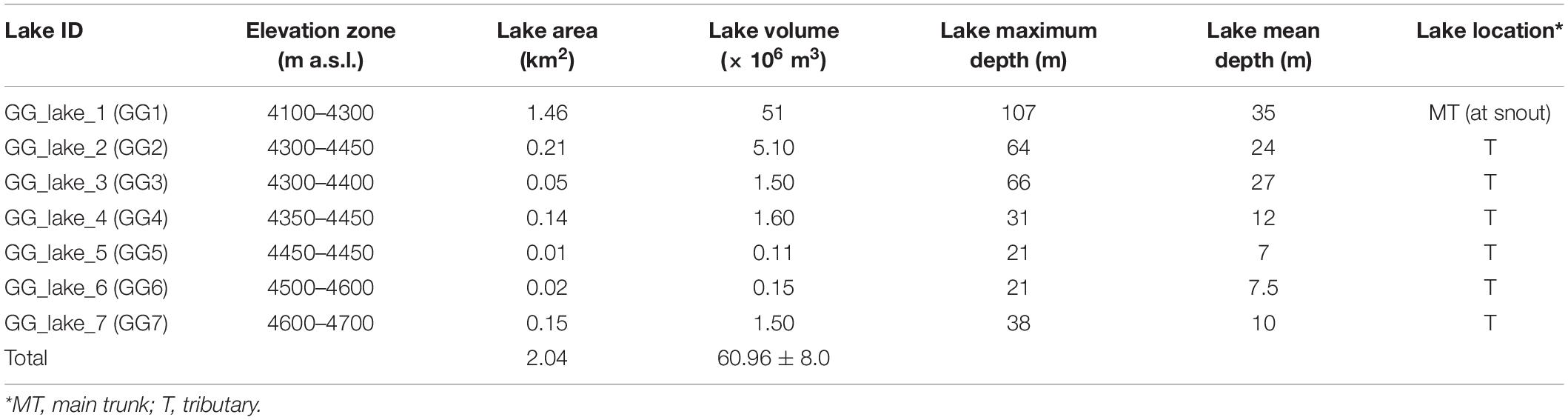
Table 2. Physical attributes of the identified potential sites of future lake formation in the Gepang Gath Glacier.
The area of these PFGLs range from 0.01 to 1.46 km2, and their storage volume vary between 0.11 and 51 × 106 m3. Out of these seven PFGLs, only GG_lake_1 would be formed at the main trunk while the others would be formed at the glacier tributaries. In terms of both area and volume, GG_lake_1 would be the largest and GG_lake_5 would be the smallest. The identified PFGLs in the Gepang Gath Glacier would occupy a total area of 2.04 km2, which constitutes 18.61% of the existing glacier area. The combined lake storage volume was estimated as 60.96 ± 8.0 × 106 m3, which is 6% of the existing glacier volume (1.08 km3) as obtained from the GlabTop2_IITB model simulations.
Currently, the Gepang Gath Glacier has one proglacial lake of around 0.8 km2 lying between the surface elevation of 4050 and 4100 m a.s.l. (Figure 4). Between 1971 and 2004, this proglacial lake had expanded from 0.1 to 0.8 km2 (Patel et al., 2017). Over the past 10 years, the glacier behind the proglacial lake has retreated by a distance of 300 m, and the lake may further expand in the future (Worni et al., 2013). As per our estimates, the lake would continue to grow in the face of continuous glacier retreat until it eventually attains the combined size of the current glacial lake and the PFGL GG_lake_1 to create a bigger proglacial lake (referred as Combined PFGL GG_lake_1) with an area of 2.06 km2. Similar conclusions regarding the expansion of existing proglacial lake of the Gepang Gath Glacier were also drawn by Allen et al. (2016a) in which the future overdeepenings were identified using the GlabTop2 model (Frey et al., 2014).
It is important to highlight that the present moraine dammed proglacial lake at the Gepang Gath Glacier (Randhawa et al., 2005) could expand in the future due to the calving of glacier ice (Thakuri et al., 2016). Such continuous calving activities at the massive glacier tongue could possibly trigger the dam breach process (Benn and Evans, 2010; Iturrizaga, 2011; Worni et al., 2013). Therefore, if collapsed, even the existing Gepang Gath proglacial lake has a high damage potential (Patel et al., 2017). Further, when the existing proglacial lake and PFGL GG_lake_1 combine, the resultant larger proglacial lake would present an even higher damage potential in terms of GLOF.
Validation Exercise on Anticipation of Current Glacial Lake Extent Using Historical Data
In view of the difficulty in validating the predictions of future conditions, an attempt was made to know whether the formation/expansion glacier lake in the present state could have been anticipated in the past using historical data. A similar exercise was conducted by Frey et al. (2010) on the Swiss Alps where the three-level approach was applied to historical data to allow a comparison with the present situation. In this study, we considered the Gepang Gath Glacier, where a proglacial lake had formed during in the 1970s (Prakash and Nagarajan, 2018). The bed topography of Gepang Gath Glacier was derived from the ice-thickness distribution obtained from the GlabTop2_IITB model simulations based on the glacier boundary of the year 2000 and the archived historical DEM (i.e., SRTM 30 m) captured during February 2000. Later, the extracted glacier bed topography was used to identify the overdeepenings.
Figures 5A–C illustrates the change in the extent of Gepang Gath Glacier between the years 2000 and 2017. Because the exercise was performed for identifying the extent of present glacier lakes using historical data, only the PFGL predicted near the snout location is shown in Figure 5A. Based on the analysis performed using the data of the year 2000, the modeled PFGL (blue region in Figure 5A) would have been the extension of the proglacial lake existing in the year 2000 (cross-hatch region in Figure 5A).
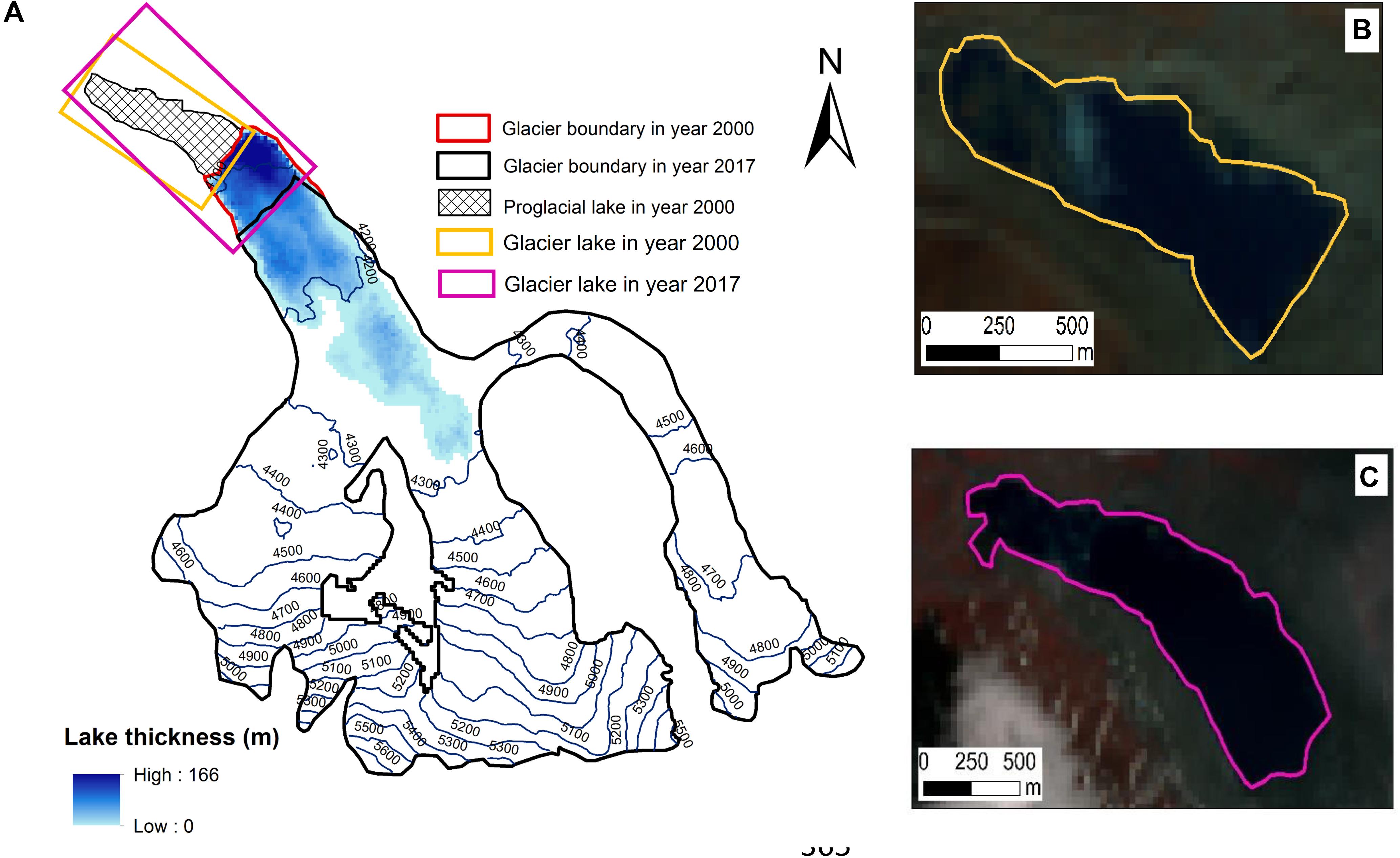
Figure 5. (A) Gepang Gath Glacier boundary in the year 2000 and 2017. Blue region shows the extent of potential future glacial lake observed from the year 2000 data. (B) Proglacial lake in the year 2000 (Image Source: Landsat 7—15 Oct 2000) and (C) proglacial lake in the year 2017 (Image Source: Landsat 8—16 June 2017).
In 2017, a segment of the PFGL (the region between the red and black glacier boundaries in Figure 5A) identified based on the data for the year 2000 had emerged, and would be expanding further due to glacier retreat and calving process, as mentioned by Thakuri et al. (2016). It is clearly visible that within a span of 17 years, a large glaciated area has been vacated and turned into a proglacial lake, as predicted in the present study (Figures 5B,C). Overall, this exercise proves that the obtained overdeepenings at glacier bed topography aid predicting PFGL sites, and thus the approach could be reliably applied to the rest of the study glaciers. However, because of the unavailability of in situ lake bathymetric data, it is not possible to directly compare the modeled results with the lake bottom topography for validation. Therefore, the discussion mainly focuses on the extent to which the existing proglacial lake would expand in the face of continuous glacier retreat.
Potential Future Lake Sites in the Samudra Tapu Glacier
Figures 6A,B shows the overdeepenings at different elevations in both the right and left trunks (Figure 6C) of the Samudra Tapu Glacier. For this glacier, most overdeepenings are observed below the long-term average ELA of 5200 m a.s.l. However, a few overdeepenings are also observed close to or above the ELA. Based on the spatial distribution of all the observed overdeepenings, a total of 26 PFGLs were identified in this glacier (Table 3). The area of these PFGLs range from 0.03 to 1.21 km2 and their storage volume vary between 0.18 and 40 (× 106) m3. Out of the 26 PFGLs, 12 would appear at the main trunk while the remaining 14 would be formed at the tributaries (Figure 7A). In terms of lake area and volume, ST_lake_1 will be the largest while ST_lake_10 will be the smallest. The total area of PFGLs in the Samudra Tapu Glacier was estimated as 7.91 km2, which represents 8% of the existing glacier area. The total storage volume of the PFGLs was calculated as 225 ± 30 × 106 m3, which is equivalent to 2.4% of the current glacier volume.
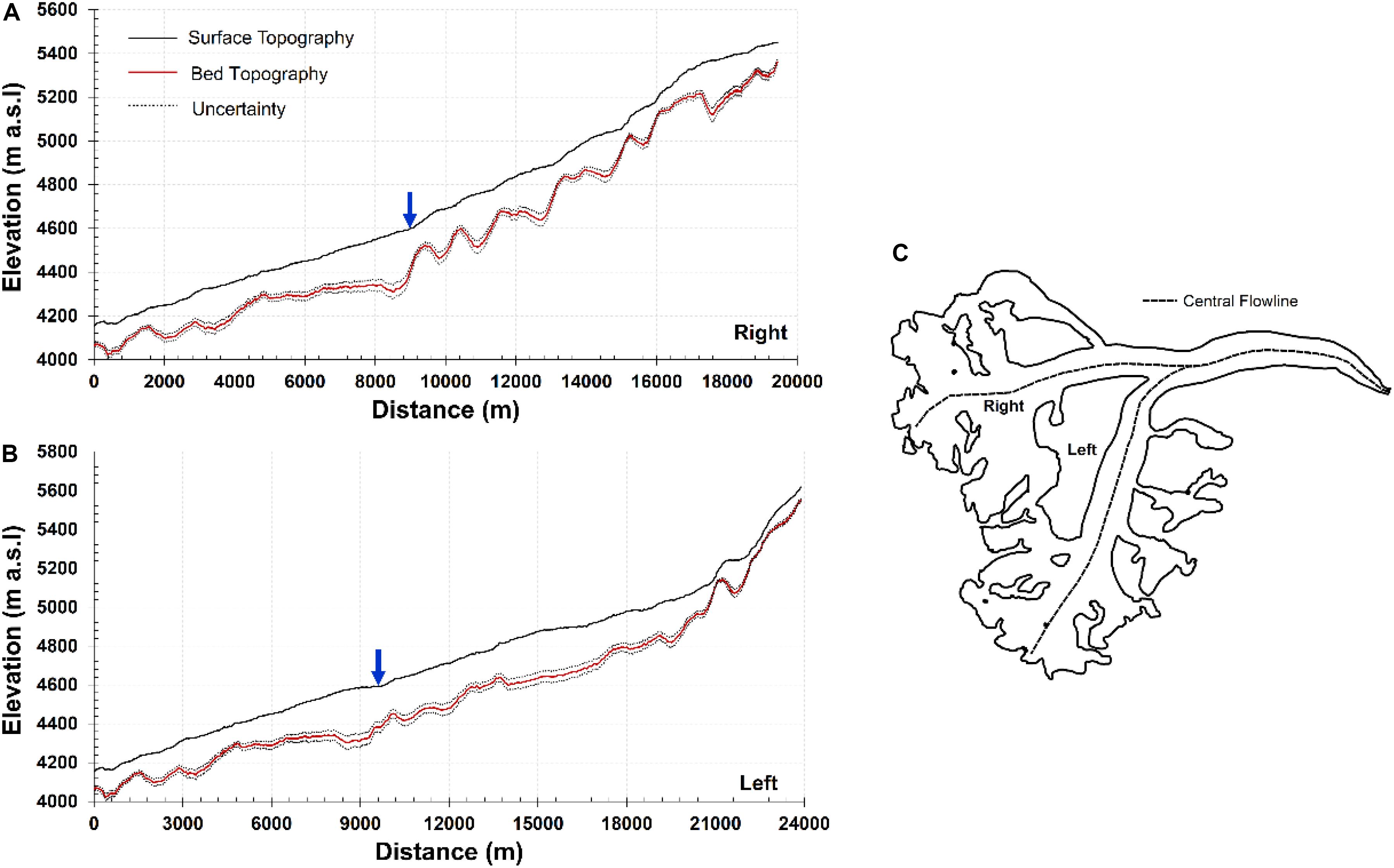
Figure 6. Glacier surface and bed topographic profiles along the central flow line of (A) right and (B) left trunk of the Samudra Tapu Glacier. (C) Glacier boundary demarcated with left and right trunk of the glacier. The location of confluence (blue arrow) of the right and left trunks of the glacier is about 4600 m a.s.l.
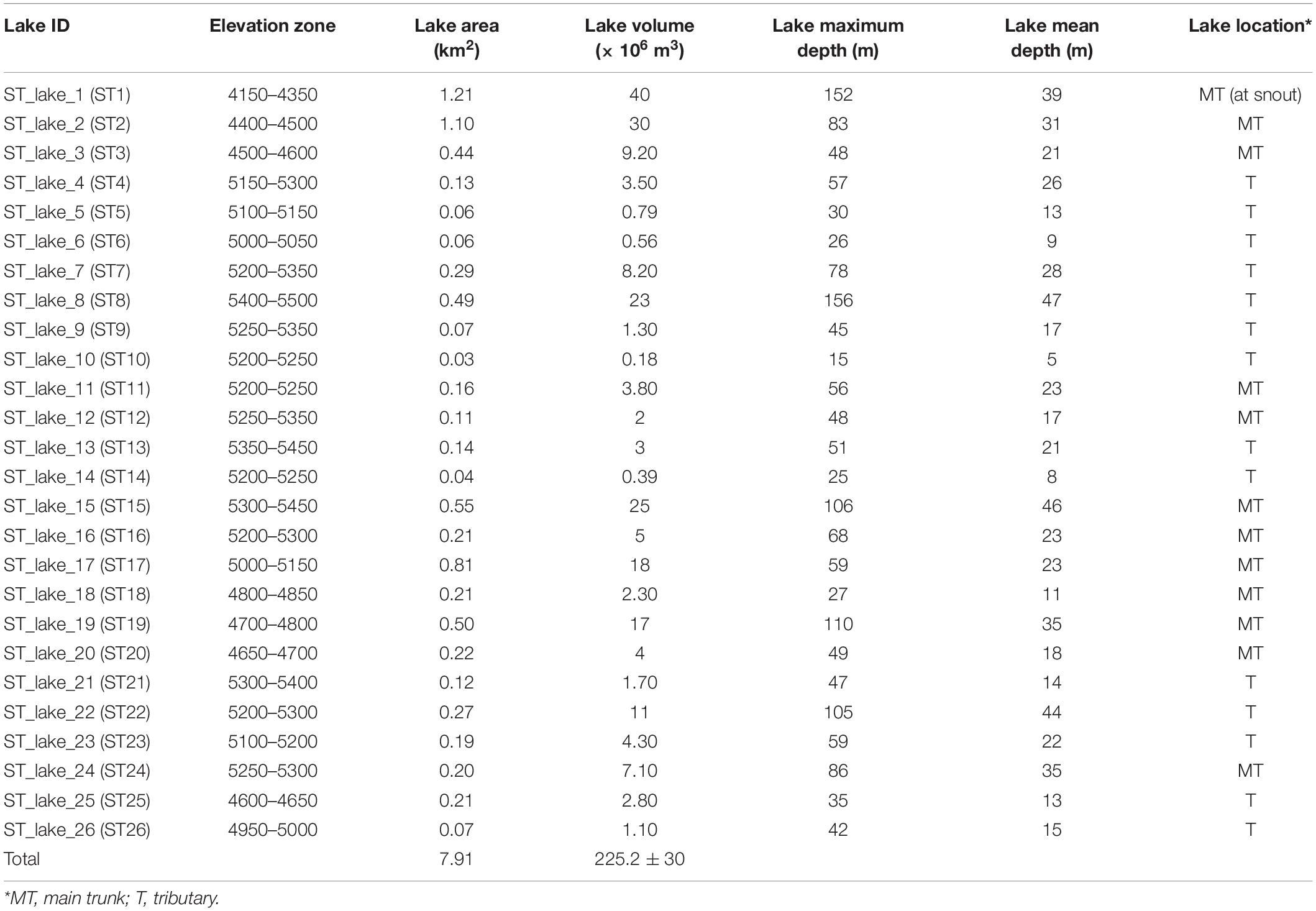
Table 3. Physical attributes of the identified potential sites of future lake formation in the Samudra Tapu Glacier.
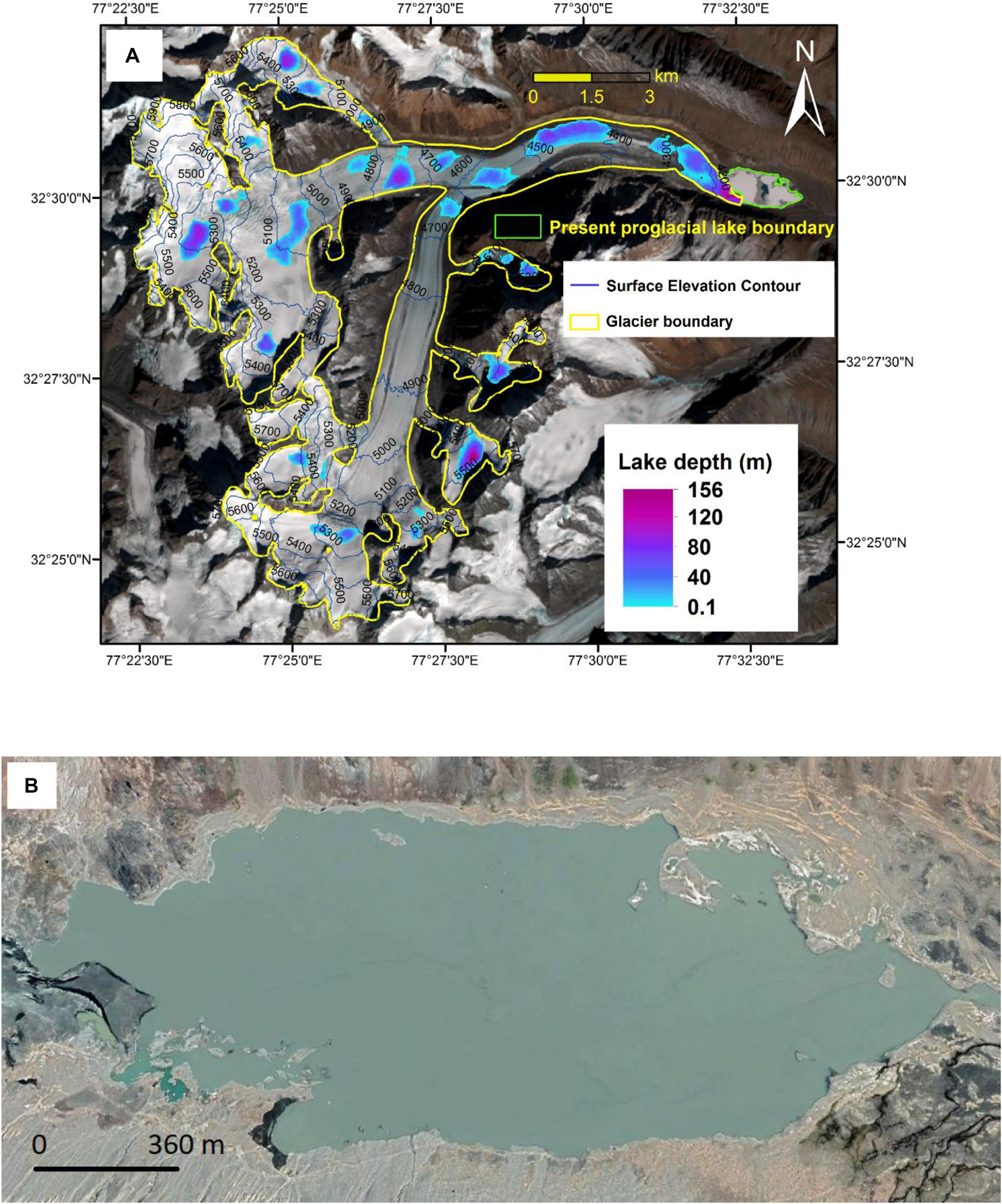
Figure 7. (A) Potential sites for future lake formation in the Samudra Tapu Glacier. Background image: standard FCC of Landsat 8 image acquired on 17 March 2017. (B) Enlarged image of the existing proglacial lake at the Samudra Tapu Glacier (Image source: Google Earth, Sep 2019).
The proglacial lake currently existing at the snout is shown in Figure 7B. Between the years 2000 and 2017, no significant variation in the proglacial lake area was detected, unlike the case of Gepang Gath Glacier lake. Between 1971 and 2014, the area of this lake had increased from 0.2 to 1.2 km2 (Patel et al., 2017), and its area in 2017 was calculated as 1.26 km2. In the future, this proglacial lake (Figure 7B) would expand further in the face of continuous glacier retreat to attain an area of 2.47 km2 (referred to as Combined PFGL ST_lake_1). As per the results of the present study, this combined lake is expected between the surface elevation ranges of 4160 and 4350 m a.s.l., and would have a large discharge potential in case of a lake outburst.
Potential Future Lake Sites in all the Study Glaciers of the Chandra Basin
As in the cases of Gepang Gath and Samudra Tapu Glacier, the extracted overdeepenings were analyzed to identify PFGL sites for the remaining 63 study glaciers in Chandra basin. Figure 8 shows the location and depth variations of the identified PFGL for all 65 study glaciers.
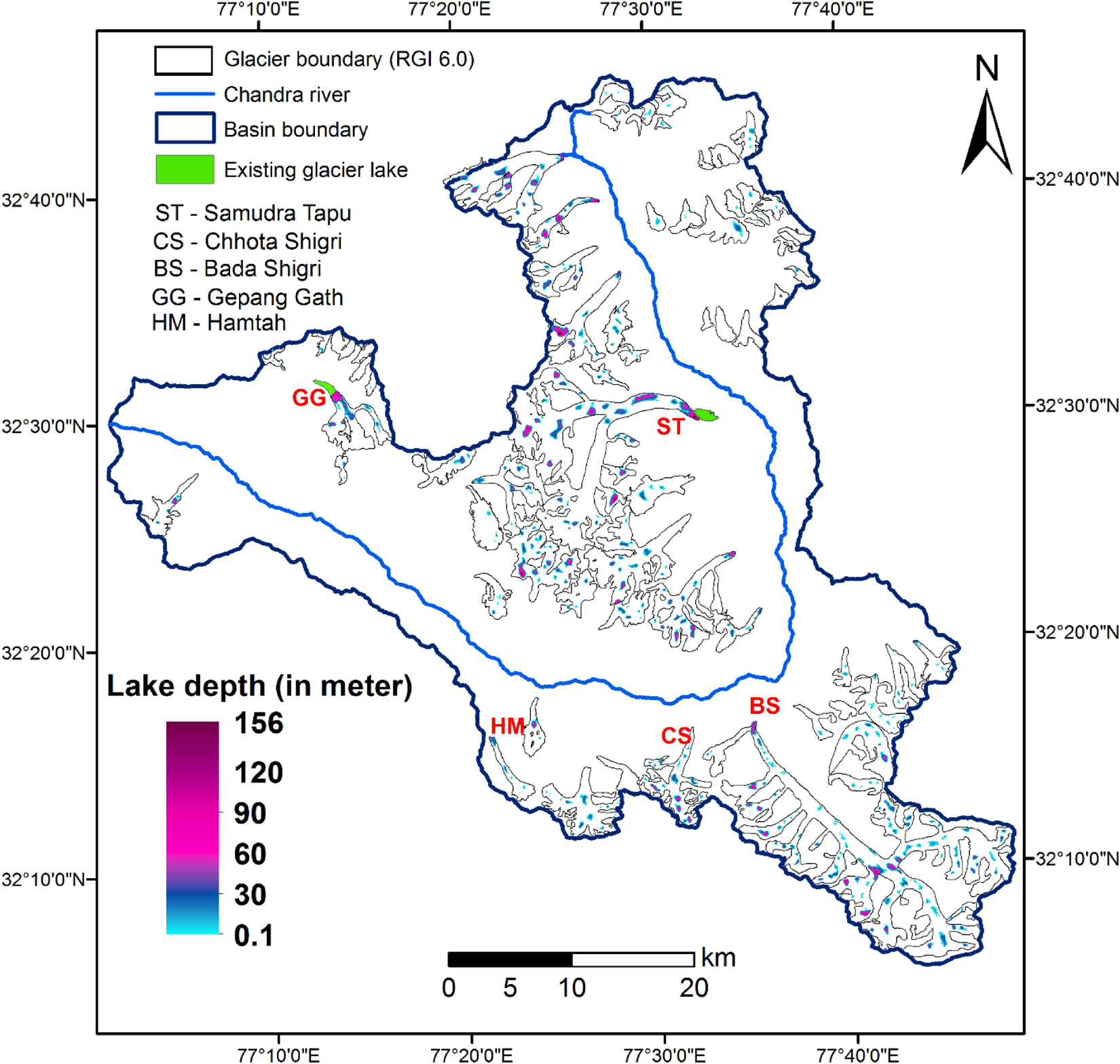
Figure 8. Potential sites for future glacial lake formation in all 65 study glaciers of the Chandra basin.
Based on the modeled PFGL distribution, glacier-wise future lake information such as total lake area, total lake volume, and the number of future lakes are compiled in Figure 9 (see the Supplementary Table A1 for the location of glaciers). From Figure 9, it was found that 360 PFGLs would emerge in this region. These PFGLs were estimated to occupy an area of 49.56 km2, which corresponds to 8.4% of the total area of all 65 study glaciers currently (591 km2). The total storage volume of these PFGLs was assessed as 1.08 ± 0.15 km3.
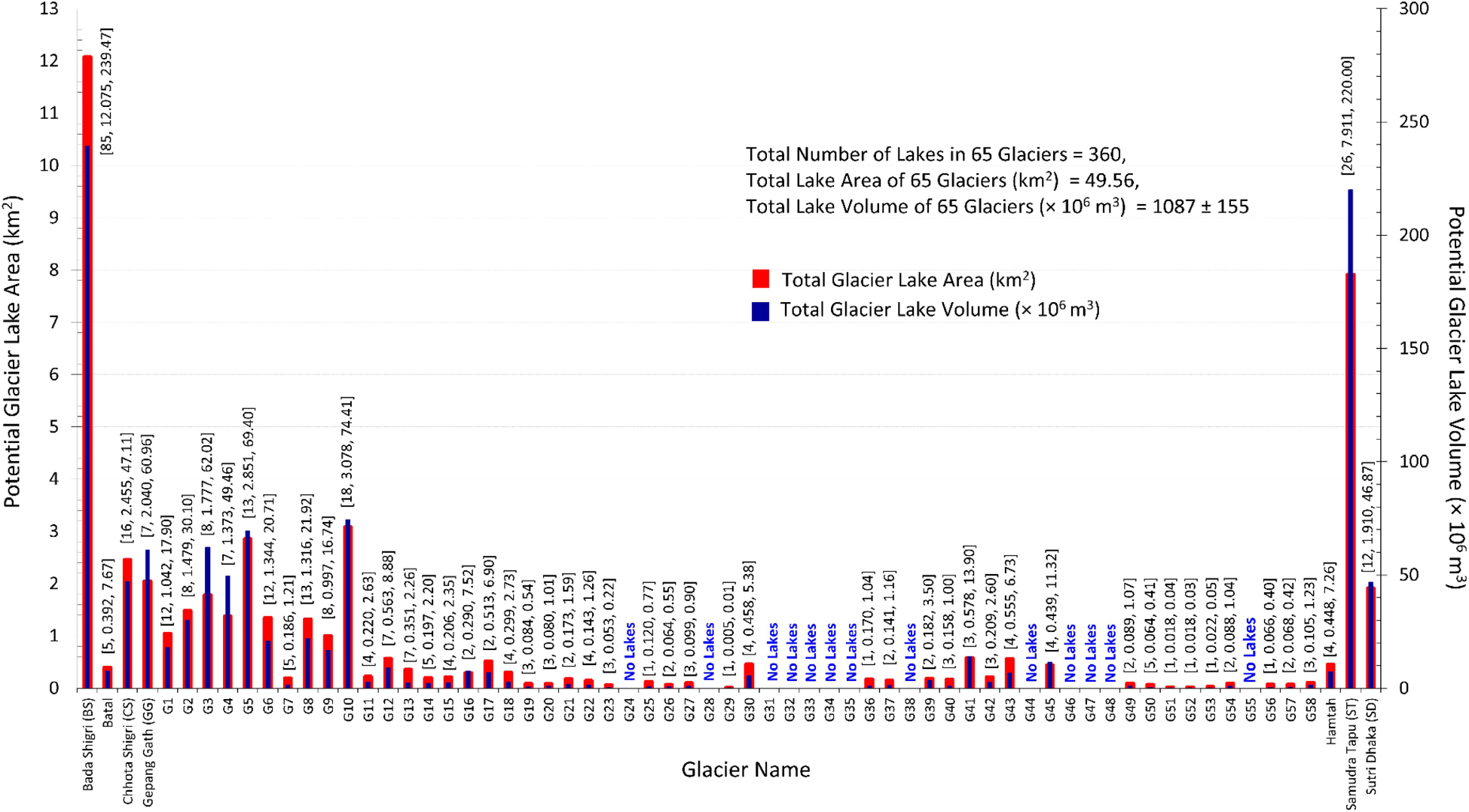
Figure 9. Glacier-wise number, total area, and volume of PFGLs in all 65 study glaciers. Information associated with each bar is arranged as [number of lakes, total lake area (km2), total lake volume (km3)] Table 5 provides the area and storage volume of the largest PFGL in each elevation zone. Such elevation-based knowledge of lakes would greatly benefit the policymakers and disaster management authorities in preparing mitigation policies well in advance. In the context of GLOF hazards associated with future lake development, the authorities can devise strategies to continuously monitor the locations of PFGLs, especially in Gepang Gath, Bada Shigri, G41, and G6 Glaciers (Table 5).
Out of 360 PFGLs, 203 lakes (56%) were found to have an area less than 0.1 km2 (“small size”), 154 PFGLs to have an area between 0.1 and 1 km2 (“medium size”), and only three to have an area more than 1 km2 (“large size”). The total volume of the PFGLs falling in small, medium, and large size categories was estimated as 0.10, 0.86, and 0.12 km3, respectively. This indicates that the medium-size lakes would combinedly hold almost 80% of the total storage volume of all PFGLs. The analysis also showed that 65% of the lakes would be formed in the main trunk of the glaciers while the remaining would be at the glacier tributaries.
Bada Shigri, the largest of all 65 study glaciers, was observed to contain 85 PFGLs, the most for any glacier in this region. Samudra Tapu was ranked second with 26 PFGLs. The total storage volume of the Bada Shigri and Samudra Tapu glacial lakes was calculated to be 239 and 225 × 106 m3, respectively. Apart from these two glaciers, only four would have PFGLs with a total storage volume between 50 and 100 × 106 m3, while 46 would have PFGLs with a total storage volume below 50 × 106 m3. The remaining 13 glaciers did not show any sign of overdeepenings and thus indicated no future lake development. These 13 glaciers are generally smaller in size with an area ranging from 1 to 4 km2. Ashraf et al. (2012), which studied the GLOF hazards in the Hindukush, Karakoram, and Himalayan Ranges of Pakistan, suggested that the valley lakes larger than 0.1 km2 are potentially dangerous. Accordingly, in our study region, 20 PFGLs were identified as potentially hazardous in the event of GLOF occurrence, and their combined storage volume was found to be more than 0.001 km3. The formation of these future lakes would also accelerate the ice calving process. There are many examples of serious GLOF disasters caused by smaller lakes too. For instance, the Kedarnath disaster in the year 2013 originated from a lake with a volume less than 0.5 × 106 m3 (Das et al., 2015; Allen et al., 2016b).
A maximum depth of around 156 m was observed for the future lake identified in Samudra Tapu Glacier. Almost 70% of the identified PFGLs would have a maximum depth lower than 50 m, whereas the rest would have a maximum depth ranging from 50 to 156 m. The mean depth of all 65 study glaciers would vary between 1.5 and 51 m.
To investigate the elevation-wise distribution, the identified PFGLs were grouped into different elevation zones having an interval of 500 m, based on the current surface elevation levels (Table 4). Hypsometric analysis revealed that the elevation zone 5000–5500 m a.s.l. would consist of the highest number of PFGLs (208), which would totally occupy an area of 26.81 km2 (i.e., 54.09% of combined area of all PFGLs). Also, the total storage volume of PFGLs in this elevation zone was estimated as 0.57 km3 (i.e., 52.29% of the combined volume of all PFGLs). On the other hand, only one PFGL would be formed at the elevation zone 3500–4000 m a.s.l. Further, among the 65 study glaciers, 35 would have future proglacial lakes at their current snout locations, mostly in the elevation zones 4500–5000 and 5000–5500 m a.s.l.
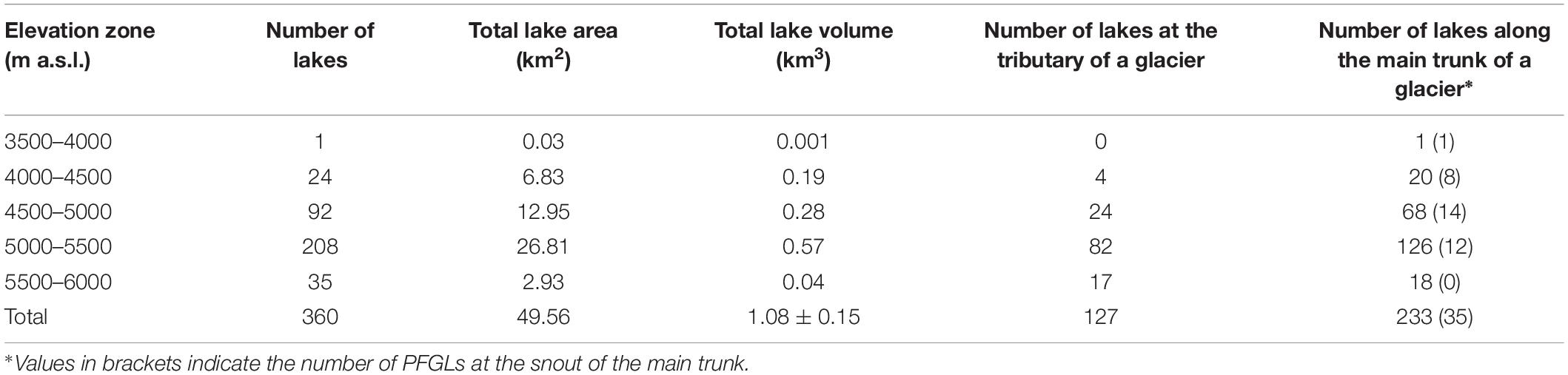
Table 4. Elevation zone-wise potential future glacial lake information for all the 65 study glaciers.
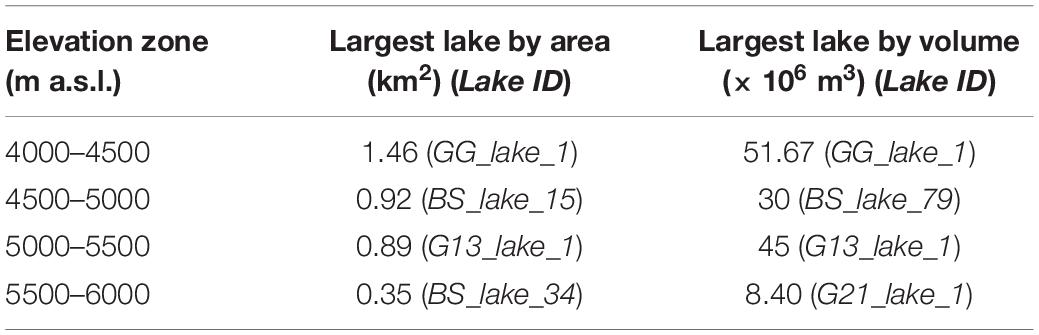
Table 5. Elevation zone-wise largest potential future glacial lake information based on their area and storage volume.
The ice-thickness estimates used for extracting glacier bed topographies in this study are associated with relatively low uncertainty (Ramsankaran et al., 2018) compared with previous studies such as Frey et al. (2014) and Linsbauer et al. (2016). This lower uncertainty was achieved through optimal parameterization of Glabtop2_IITB model and use of high-resolution TDX DEM, as reported in Ramsankaran et al. (2018) and Pandit and Ramsankaran (2020). Hence, the spatial and dimensional characteristics/attributes of the PFGLs reported could be considered reliable.
Conclusion
In the present research, a detailed high-resolution database of 360 potential future glacial lakes (with a total area and volume of 49.56 km2 and 1.08 km3, respectively) was developed for 65 glaciers in Chandra basin, located in western Himalayas. The location of the lakes was determined based on the overdeepenings identified in the glacier bed topographies derived using an optimally parameterized GlabTop2_IITB model. With the use of high-resolution bed topographic information, the study captured the PFGLs having an area greater than 0.005 km2. The developed database includes the lakes’ geographical distribution across the elevation zones, mean depth, maximum depth, location, its area, and volume. This information shall greatly benefit the policymakers, disaster management officials, and local administrative officials in preparing mitigation policies against GLOF events in the future.
Data Availability Statement
The modelled ice thickness information for all the study glaciers can be obtained from http://doi.org/10.5281/zenodo.3694001. The identified potential sites of future glacial lakes and their characteristics can be retrieved from http://doi.org/10.5281/zenodo.3727617 as well as from the Supplementary Material.
Author Contributions
AP and RR designed the study. AP conducted the analysis and wrote the manuscript. RR contributed to discussions and revisions of the manuscript, providing important feedback, comments, and suggestions. Both authors contributed to the article and approved the submitted version.
Conflict of Interest
AP is employed by Tata Consultancy Services Limited.
The remaining author declares that the research was conducted in the absence of any commercial or financial relationships that could be construed as a potential conflict of interest.
Acknowledgments
We acknowledge the support provided by the Indian Institute of Technology Bombay, Center of Excellence in Climate Studies (IITB-CECS) project of the Department of Science and Technology (DST), New Delhi, India. This study was partially funded by Ministry of Earth Sciences (MOES) and Ministry of Human Resource Development (MHRD), Government of India under the IMPacting Research INnovation and Technology (IMPRINT) scheme (Project Number: 4096). We are thankful to the German Aerospace Agency (DLR) for providing TanDEM-X CoSSC products under the TanDEM-X Science proposal XTI_GLAC7043. We are also thankful to the editor for providing valuable suggestions during the pre-review stage of the article and to the two referees for their constructive comments which helped to improve the article. We are also grateful to the publishers of Frontiers journals for providing waiver in publication fee.
Supplementary Material
The Supplementary Material for this article can be found online at: https://www.frontiersin.org/articles/10.3389/feart.2020.500116/full#supplementary-material
Footnotes
References
Allen, S., Schneider, D., and Owens, I. F. (2009). First approaches towards modelling glacial hazards in the Mount Cook region of New Zealand’s Southern Alps. Nat. Hazards Earth Syst. Sci. 9, 481–499. doi: 10.5194/nhess-9-481-2009
Allen, S. K., Linsbauer, A., Randhawa, S. S., Huggel, C., Rana, P., and Kumari, A. (2016a). Glacial lake outburst flood risk in Himachal Pradesh, India: an integrative and anticipatory approach considering current and future threats. Nat. Hazards 84, 1741–1763. doi: 10.1007/s11069-016-2511-x
Allen, S. K., Rastner, P., Arora, M., Huggel, C., and Stoffel, M. (2016b). Lake outburst and debris flow disaster at Kedarnath, June 2013: hydrometeorological triggering and topographic predisposition. Landslides 13, 1479–1491. doi: 10.1007/s10346-015-0584-3
Ashraf, A., Naz, R., and Roohi, R. (2012). Glacial lake outburst flood hazards in Hindukush, Karakoram and Himalayan Ranges of Pakistan: implications and risk analysis. Geomat. Nat. Hazards Risk 3, 113–132. doi: 10.1080/19475705.2011.615344
Bhutiyani, M. R., Kale, V. S., and Pawar, N. J. (2007). Long-term trends in maximum, minimum and mean annual air temperatures across the Northwestern Himalaya during the twentieth century. Clim. Change 85, 159–177. doi: 10.1007/s10584-006-9196-1
Bolch, T., Kulkarni, A., Kääb, A., Huggel, C., Paul, F., Cogley, J. G., et al. (2012). The state and fate of Himalayan glaciers. Science 336, 310–314. doi: 10.1126/science.1215828
Bookhagen, B., and Burbank, D. W. (2010). Toward a complete Himalayan hydrological budget: spatiotemporal distribution of snowmelt and rainfall and their impact on river discharge. J. Geophys. Res. Earth Surface 115, 1–25. doi: 10.1029/2009JF001426
Boulton, G. S. (1967). The development of a complex supraglacial moraine at the margin of Sørbreen, Ny Friesland, Vestspitsbergen. J. Glaciol. 6, 717–735. doi: 10.3189/s0022143000019961
Chandrasekharan, A., Ramsankaran, R., Pandit, A., and Rabatel, A. (2018). Quantification of annual glacier surface mass balance for the chhota shigri glacier, Western Himalayas, India using an equilibrium-line altitude (ELA) based approach. Int. J. Remote Sens. 39, 9092–9112. doi: 10.1080/01431161.2018.1506182
Clague, J. J., and Evans, S. G. (1994). Formation and Failure of Natural Dams in the Canadian Cordillera 464. Ottawa: Geological Survey of Canada.
Das, S., Kar, N. S., and Bandyopadhyay, S. (2015). Glacial lake outburst flood at Kedarnath, Indian Himalaya: a study using digital elevation models and satellite images. Nat. Hazards 77, 769–786. doi: 10.1007/s11069-015-1629-6
Dash, S. K., Jenamani, R. K., Kalsi, S. R., and Panda, S. K. (2007). Some evidence of climate change in twentieth-century India. Clim. Change 85, 299–321. doi: 10.1007/s10584-007-9305-9
Frey, H., Haeberli, W., Linsbauer, A., Huggel, C., and Paul, F. (2010). A multi-level strategy for anticipating future glacier lake formation and associated hazard potentials. Nat. Hazards Earth Syst. Sci. 10, 339–352. doi: 10.5194/nhess-10-339-2010
Frey, H., Machguth, H., Huss, M., Huggel, C., Bajracharya, S., Bolch, T., et al. (2014). Estimating the volume of glaciers in the Himalayan–Karakoram region using different methods. Cryosphere 8, 2313–2333. doi: 10.5194/tc-8-2313-2014
Gardelle, J., Arnaud, Y., and Berthier, E. (2011). Contrasted evolution of glacial lakes along the Hindu Kush Himalaya mountain range between 1990 and 2009. Glob. Planet. Change 75, 47–55. doi: 10.1016/j.gloplacha.2010.10.003
Gautam, R., Hsu, N. C., and Lau, K. M. (2010). Premonsoon aerosol characterization and radiative effects over the Indo-Gangetic Plains: implications for regional climate warming. J. Geophys. Res. Atmos. 115, 1–15. doi: 10.1029/2010JD013819
Haeberli, W., and Hoelzle, M. (1995). Application of inventory data for estimating characteristics of and regional climate-change effects on mountain glaciers: a pilot study with the European Alps. Ann. Glaciol. 21, 206–212. doi: 10.1017/S0260305500015834
Haeberli, W., Kääb, A., Mühll, D. V., and Teysseire, P. (2001). Prevention of outburst floods from periglacial lakes at Grubengletscher, Valais, Swiss Alps. J. Glaciol. 47, 111–122. doi: 10.3189/172756501781832575
Huggel, C., Kääb, A., Haeberli, W., Teysseire, P., and Paul, F. (2002). Remote sensing based assessment of hazards from glacier lake outbursts: a case study in the Swiss Alps. Can. Geotech. J. 39, 316–330. doi: 10.1139/T01-099
Iturrizaga, L. (2011). “Glacier lake outburst floods,” in Encyclopedia of Snow, Ice and Glaciers, eds H. Oerlemans, V. P. Singh, and W. Haeberli (Cham: Springer), 381–399. doi: 10.1007/978-90-481-2642-2_196
Jain, S. K., Lohani, A. K., Singh, R. D., Chaudhary, A., and Thakural, L. N. (2012). Glacial lakes and glacial lake outburst flood in a Himalayan basin using remote sensing and GIS. Nat. Hazards 62, 887–899. doi: 10.1007/s11069-012-0120-x
Kääb, A., Huggel, C., Fischer, L., Guex, S., Paul, F., Roer, I., et al. (2005). Remote sensing of glacier and permafrostrelated hazards in high mountains: an overview. Nat. Hazards Earth Syst. Sci. 5, 527–554. doi: 10.5194/nhess-5-527-2005
Li, J., and Sheng, Y. (2012). An automated scheme for glacial lake dynamics mapping using Landsat imagery and digital elevation models: a case study in the Himalayas. Int. J. Remote Sens. 33, 5194–5213. doi: 10.1080/01431161.2012.657370
Linsbauer, A., Frey, H., Haeberli, W., Machguth, H., Azam, M. F., and Allen, S. (2016). Modelling glacier-bed overdeepenings and possible future lakes for the glaciers in the Himalaya—Karakoram region. Ann. Glaciol. 57, 119–130. doi: 10.3189/2016AoG71A627
Linsbauer, A., Paul, F., and Haeberli, W. (2012). Modeling glacier thickness distribution and bed topography over entire mountain ranges with GlabTop: application of a fast and robust approach. J. Geophys. Res. Earth Surf. 117, 1–17. doi: 10.1029/2011JF002313
Linsbauer, A., Paul, F., Hoelzle, M., Frey, H., and Haeberli, W. (2009). “The Swiss Alps without glaciers: a GIS-based modelling approach for reconstruction of glacier beds,” in Proceedings of Geomorphometry, (Zurich, CH: University of Zurich), 243–247.
Negi, H. S., Saravana, G., Rout, R., and Snehmani (2013). Monitoring of great Himalayan glaciers in Patsio region, India using remote sensing and climatic observations. Curr. Sci. 105, 1383–1392.
Nie, Y., Sheng, Y., Liu, Q., Liu, L., Liu, S., Zhang, Y., et al. (2017). A regional-scale assessment of Himalayan glacial lake changes using satellite observations from 1990 to 2015. Remote Sens. Environ. 189, 1–13. doi: 10.1016/j.rse.2016.11.008
Nye, J. F. (1965). The flow of a glacier in a channel of rectangular, elliptic or parabolic cross-section. J. Glaciol. 5, 661–690. doi: 10.1017/S0022143000018670
Pandey, P., Ali, S. N., Ramanathan, A. L., and Venkataraman, G. (2017). Regional representation of glaciers in Chandra Basin region, western Himalaya, India. Geosci. Front. 8, 841–850. doi: 10.1016/j.gsf.2016.06.006
Pandit, A., and Ramsankaran, R. (2020). Modeling ice-thickness distribution and storage volume of glaciers in the chandra basin, India. J. Mountain Sci. 17, 2011–2022. doi: 10.1007/s11629-019-5718-y
Pandit, A., Ramsankaran, R., and Rao, Y. S. (2014). Generation and validation of the interferometric SAR DEMs from TanDEM-X data for gangotri and hamtah glaciers of Indian Himalayas. Proc. Technol. 16, 793–805. doi: 10.1016/j.protcy.2014.10.029
Patel, L. K., Sharma, P., Laluraj, C. M., Thamban, M., Singh, A., and Ravindra, R. (2017). A geospatial analysis of Samudra Tapu and Gepang Gath glacial lakes in the Chandra Basin, Western Himalaya. Nat. Hazards 86, 1275–1290. doi: 10.1007/s11069-017-2743-4
Prakash, C., and Nagarajan, R. (2018). Glacial lake changes and outburst flood hazard in Chandra basin, North-Western Indian Himalaya. Geomatics Nat. Hazards Risk 9, 337–355. doi: 10.1080/19475705.2018.1445663
Quincey, D. J., Richardson, S. D., Luckman, A., Lucas, R. M., Reynolds, J. M., Hambrey, M. J., et al. (2007). Early recognition of glacial lake hazards in the Himalaya using remote sensing datasets. Glob. Planet. Change 56, 137–152. doi: 10.1016/j.gloplacha.2006.07.013
Raj, B. K. G., Kumar, V. K., and Remya, S. N. (2012). Remote sensing-based inventory of glacial lakes in Sikkim Himalaya: semi-automated approach using satellite data. Geomatics Nat. Hazards Risk 4, 241–253. doi: 10.1080/19475705.2012.707153
Ramsankaran, R., Pandit, A., and Azam, M. F. (2018). Spatially distributed ice-thickness modelling for chhota shigri glacier in western Himalayas, India. Int. J. Remote Sens. 39, 3320–3343. doi: 10.1080/01431161.2018.1441563
Randhawa, S. S., Sood, R. K., Rathore, B. P., and Kulkarni, A. V. (2005). Moraine-dammed lakes study in the Chenab and the Satluj river basins using IRS data. J. Indian Soc. Remote Sens. 33, 285–290. doi: 10.1007/bf02990047
Reynolds, J. M. (2000). On the Formation of Supraglacial Lakes on Debris-Covered Glaciers. Wallingford: IAHS publication, 153–164.
Richardson, S., and Reynolds, J. (2000). An overview of glacial hazards in the Himalayas. Q. Int. 6, 31–47. doi: 10.1016/S1040-6182(99)00035-X
Sharma, P., Ramanathan, A. L., and Pottakkal, J. (2013). Study of solute sources and evolution of hydrogeochemical processes of the Chhota Shigri Glacier meltwaters, Himachal Himalaya, India. Hydrol. Sci. J. 58, 1128–1143. doi: 10.1080/02626667.2013.802092
Sharma, S. S., and Ganju, A. (2000). Complexities of avalanche forecasting in western Himalaya-an overview. Cold Regions Sci. Technol. 31, 95–102. doi: 10.1016/S0165-232X(99)00034-8
Thakuri, S., Salerno, F., Bolch, T., Guyennon, N., and Tartari, G. (2016). Factors controlling the accelerated expansion of Imja Lake, Mount Everest region, Nepal. Ann. Glaciol. 57, 245–257. doi: 10.3189/2016AoG71A063
Vuichard, D., and Zimmermann, M. (1987). The 1985 catastrophic drainage of a moraine-dammed lake, Khumbu Himal, Nepal: cause and consequences. Mountain Res. Dev. 7, 91–110. doi: 10.2307/3673305
Wagnon, P., Linda, A., Arnaud, Y., Kumar, R., Sharma, P., Vincent, C., et al. (2007). Four years of mass balance on Chhota Shigri Glacier, Himachal Pradesh, India, a new benchmark glacier in the western Himalaya. J. Glaciol. 53, 603–611. doi: 10.3189/002214307784409306
Worni, R., Huggel, C., and Stoffel, M. (2013). Glacial lakes in the Indian Himalayas-From an area-wide glacial lake inventory to on-site and modeling based risk assessment of critical glacial lakes. Sci. Tot. Environ. 468, S71–S84. doi: 10.1016/j.scitotenv.2012.11.043
Xin, W., Shiyin, L., Wanqin, G., Xiaojun, Y., Zongli, J., and Yongshun, H. (2012). Using remote sensing data to quantify changes in glacial lakes in the Chinese Himalaya. Mountain Res. Dev. 32, 203–212. doi: 10.1659/MRD-JOURNAL-D-11-00044.1
Xu, D. (1988). Characteristics of debris flow caused by outburst of glacial lake in Boqu river, Xizang, China. GeoJournal 17, 569–580. doi: 10.1007/BF00209443
Zhang, G., Bolch, T., Allen, S., Linsbauer, A., Chen, W., and Wang, W. (2019). Glacial lake evolution and glacier–lake interactions in the Poiqu River basin, central Himalaya, 1964–2017. J. Glaciol. 65, 347–365. doi: 10.1017/jog.2019.13
Keywords: potential future glacial lakes, TanDEM-X, GlabTop2_IITB, chandra basin, western Himalayas
Citation: Pandit A and Ramsankaran R (2020) Identification of Potential Sites for Future Lake Formation and Expansion of Existing Lakes in Glaciers of Chandra Basin, Western Himalayas, India. Front. Earth Sci. 8:500116. doi: 10.3389/feart.2020.500116
Received: 16 November 2019; Accepted: 14 August 2020;
Published: 16 September 2020.
Edited by:
Shin Sugiyama, Hokkaido University, JapanReviewed by:
Guoqing Zhang, Institute of Tibetan Plateau Research (CAS), ChinaHolger Frey, University of Zurich, Switzerland
Copyright © 2020 Pandit and Ramsankaran. This is an open-access article distributed under the terms of the Creative Commons Attribution License (CC BY). The use, distribution or reproduction in other forums is permitted, provided the original author(s) and the copyright owner(s) are credited and that the original publication in this journal is cited, in accordance with accepted academic practice. No use, distribution or reproduction is permitted which does not comply with these terms.
*Correspondence: RAAJ Ramsankaran, cmFtc2Fua2FyYW5AY2l2aWwuaWl0Yi5hYy5pbg==