- Institute of Geosciences and Geography, Martin Luther University Halle-Wittenberg, Halle, Germany
Thermodynamic coupling between atmosphere and ground yields increasing aquifer temperatures as a consequence of global warming. While this is expected to manifest as a gradual warming in groundwater temperature time series, such continuous long-term recordings are scarce. As an alternative, the present work examines the use of repeated temperature-depth profiles of 32 wells in southern Germany, that were logged during campaigns in the early 1990s and in 2019. It is revealed that the temperatures have increased in nearly all cases. We find a moderate to good depth-dependent correlation to trends in air temperature, which however is strongly influenced by local hydrogeological and climate conditions. While during the last three decades, air temperatures have increased by a rate of 0.35 K (10a)−1 on average, the temperature increase in the subsurface is decreasing with depth, with median values of 0.28 K (10a)−1 in 20 m and only of 0.09 K (10a)−1 in 60 m depth. Still, the slow and damped warming of the groundwater bodies are remarkable, especially considering naturally very minor temperature changes in pristine groundwater bodies and predictions of atmospheric temperatures. This entails implications for temperature-dependent ecological and hydro-chemical processes, and also for the heat stored in the shallow ground. Moreover, it is demonstrated that the annual heat gain in the groundwater bodies below 15 m due to climate change is in the range of one third of the state’s heat demand, which underlines the geothermal potential associated with the change in natural heat fluxes at the ground surface.
Introduction
Global warming is one of the most pressing challenges in the 21st century. Atmospheric climate and temperature variations are excessively studied, and a gigantic amount of climate data are continuously collected to delineate past warming trends and to predict future impacts of greenhouse gas accumulation. There is much less attention to the subsurface thermal regime, which is thermodynamically coupled with the atmosphere and thus also influenced by climate change. In fact, borehole temperature depth-profiles that deviate from the normal geothermal gradient served as early witnesses of regional atmospheric temperature increase (Wang and Lewis, 1992; Pollack and Chapman, 1993). This has in particular been studied in boreholes where vertical conduction is the dominant heat transport process and groundwater flow can be neglected. In contrast, temperature profiles recorded in wells are favored to infer the role and intensity of vertical groundwater flow (Bredehoeft and Papaopulos, 1965; Sorey, 1971; Taniguchi, 1993; Taniguchi et al., 1999; Bense et al., 2017; Kurylyk et al., 2019; Kurylyk and Irvine, 2019; Li et al., 2019). The use of natural temperature variations in aquifers has also been recognized as a precious tracer to understand groundwater flow systems, in particular when interacting with surface waters (Constantz, 2008; Rau et al., 2010; Molina-Giraldo et al., 2011; Saar, 2011; Coluccio and Morgan, 2019; Kaandorp et al., 2019).
Temperature profiles measured in wells reflect spatially and temporally varying heat inputs in aquifers from the surface and thus can be used to examine thermal coupling at the ground surface (Gunawardhana and Kazama, 2011; Kurylyk et al., 2013; Burns et al., 2016; Bense and Kurylyk, 2017). Especially when the shallow subsurface is dominated by horizontal flow, changes in atmospheric temperatures and land use represent thermal signals that are conduced to the aquifer and become visible in well-logs. These changes are pronounced in cities, where accelerated heat flux from urban warming, sealed ground, and buried infrastructures yields large scale subsurface urban heat islands (Ferguson and Woodbury, 2004; Menberg et al., 2013; Zhu et al., 2015; Benz et al., 2016; Epting et al., 2017; Bayer et al., 2019; Hemmerle et al., 2019), and urban, industrial and waste sites are revealed to cause the most prominent local heat anomalies in Central European aquifers (Tissen et al., 2019). In less-disturbed rural areas, groundwater temperatures are reported to slowly increase as well, which is obviously the response of the shallow ground to recent climate change (Maxwell and Kollet, 2008; Bloomfield et al., 2013; Kurylyk et al., 2014; Menberg et al., 2014; Colombani et al., 2016). In comparison to atmospheric temperature recordings, however, continuously monitored long-term time series of groundwater temperatures are scarce, often strongly superimposed by local effects and thus our current picture of subsurface warming due to climate change is not very clear. As alternative means, repeated transient temperature logs in wells can be used (Bense and Kurylyk, 2017; Benz et al., 2018a). Conductive heat transport through the ground and aquifer is slow, however, the time period between two measurements has to be in the order of years and ideally decades.
In this study, the main objective is to reveal subsurface temperature variations. We report the findings of rare well temperature profiles measured again after more than 25 years. Focus is set on a large spatial coverage with several wells in order to reveal regional groundwater warming and to minimize the fingerprint of local effects. The wells are all located in the country of Bavaria in southern Germany, where climate change effects on groundwater are broadly discussed. As in many other countries, aquifers represent the major source of freshwater and thus any factors that impair groundwater reservoirs are of prime hydrological interest. Therefore, related work and recent reviews of groundwater climate change focus mainly on change of groundwater recharge and availability (Earman and Dettinger, 2011; Green et al., 2011; Stoll et al., 2011; Alam et al., 2019; Bloomfield et al., 2019; Zhang et al., 2020). Potential hydrochemical effects from climate-driven shifts in groundwater levels on contaminant mobilization are stressed by Jarsjö et al. (2020). The consequences of ongoing shallow groundwater warming are only rarely discussed but manifold (Riedel, 2019). Among these are fundamental changes to microbial activity and ecosystems in groundwater that rely on long-term stable thermal conditions (Kløve et al., 2014; Griebler et al., 2019), as well as potential threats on spring and cave ecosystems (Jyväsjärvi et al., 2015; Mammola et al., 2019). Leonhardt et al. (2017) emphasize for the springs in the Bavarian National Parks that climate change effects are most critical, and just recently therefore a program of long-term monitoring of biotic and abiotic data including temperature at 15 of these springs has been initiated by the authorities.
In the following, we describe the temperature profile measurements in Bavarian wells, which serve as the basis of this work. The presented data preparation is tailored to the purpose of this study, which is on quantifying the long-term thermal evolution of the aquifers on the country scale. This is examined by including available air temperature records and comparing the changes in the atmosphere with those found in the subsurface. Furthermore, in order to elucidate the heat accumulation associated with climate induced warming of the subsurface, also the change of the regional geothermal potential is assessed.
Methods and Data
Groundwater Temperature Data
Groundwater temperature data is recorded in Bavaria by logging temperatures in observation wells using a water level meter with a temperature sensor. Data was gathered during the period from 1992 to 1994 and in 2019. During the 1992–4 period, wells were sampled every 3 months on an irregular basis within one year, so that up to four measurements were obtained for each well. These measurements were performed by the Landesamt für Umwelt Bayern (LfU Bayern), which is the environmental state office of the federal state of Bavaria in Germany. In 2019, the same set of wells were measured in April and July/August field campaigns using a water level meter type 120 – LTC from HT Hydrotechnik GmbH. For the 2019 dataset, precision and accuracy of the temperature sensor is 0.1 K. For the 1992–4 sampling campaign, the precision is also 0.1 K. The accuracy for this dataset is assumed to be in the same magnitude, assuming the use of standard devices even though no detailed information on the applied loggers is preserved. The vertical resolution of sampling was highest close to the surface where temperature variations are commonly strongest. Temperatures were recorded in 1 m steps above 20 m, in 2 m steps between 20 and 40 m and in 10 m steps below 40 m for the 1992–4 campaign. In 2019, temperature was sensed at 0.5 m intervals above 10 m, at 1 m intervals between 10 and 40 m, at 2 m intervals between 40 and 60 m and at 5 m intervals below 60 m. For further analysis, all measured temperatures were then interpolated linearly at 1 m resolution.
Initially the data set measured in 1992–4 covered 347 temperature-depth profiles (209 of the wells are shallower than 10 m). From these, 95 wells had a complete seasonal record of four seasonal measurements. Temperature data from these 95 wells have been made available by the LfU Bayern for this study. Out of these, 46 wells were measured again in 2019. The other wells were either shallower than 15 m, have been dismissed from the observation well network (renaturalised), could not be found in the field or could not be accessed (no access permission for wells on private property).
For this study we applied a threshold for seasonal temperature variation of 0.5 K below 20 m. 12 wells that exceeded this threshold were cut for further analysis. These wells showed inconsistencies or clearly revealed the effect of disturbing processes either in the 1992–4 or in the 2019 measurements. In one of the wells abnormal changes of more than 4 K between the 1990s and 2019 period together with an inconsistent signal in 2019 were related to strong super positioning of local anthropogenic disturbances and thus these measurements were also excluded. At one well, the groundwater table was below 100 m depth, and in one well the probe got stuck in both 2019 measurements after less than 2 m below groundwater level. These two wells have also been excluded for further analysis.
The 32 remaining observation wells are located in different hydrogeological settings and distributed all over Bavaria. A map showing the location of the observation wells and air temperature stations is given in Figure 1A. Temperature-depth profiles are available as Supplementary Material to this article and are additionally archived in World Data Center PANGAEA.Temperature-depth profiles are also available at: https://doi.pangaea.de/10.1594/PANGAEA.923529.
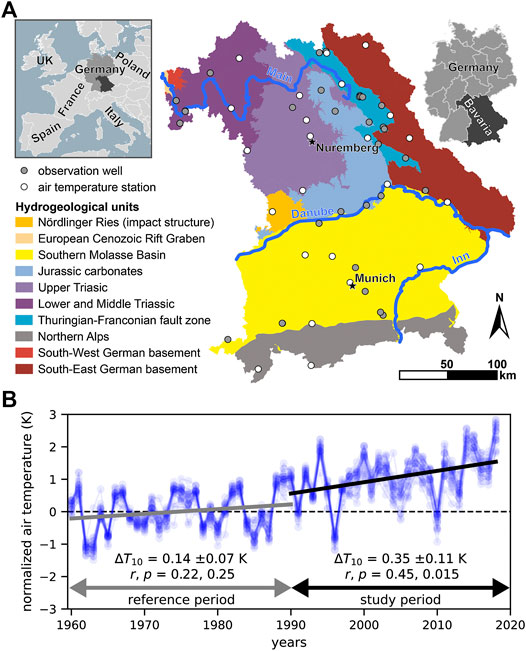
FIGURE 1. (A) Map of the main hydrogeological units and the location of the 32 groundwater temperature (GWT) observation wells and the 23 air temperature (AT) stations selected for this study. Major cities and rivers are indicated by black stars and blue lines, accordingly. (B) Arithmetic annual mean air temperatures from 1960 to 2018 for 23 stations in Bavaria (blue dots and lines). Temperatures are normalized to the respective mean temperature of the period from 1960 to 1989 for each station. ΔT10 is the decadal temperature change calculated by a linear least square regression with the Pearson correlation coefficient (r) and p-value (p).
Air Temperature Data
To derive atmospheric temperature trends, we used arithmetic annual mean values from 23 air temperature stations in Bavaria. These data are provided by the Climate Data Center (CDC) run by the Deutscher Wetterdienst (DWD). According to recommendation of the World Meteorological Organization (World Meteorological Organization, 2017) air temperature data was split into two 30-year periods. Herein after referred to as reference period 1960–1989, and study period 1990–2019. Air temperature stations were selected for having consistent, gap-free records from 1960 onwards. Detailed descriptions and metadata for each weather station can be found on the accessed FTP server by the DWD.
Theoretical Geothermal Potential
Temperature variations in the subsurface also change the total thermal energy stored in the subsurface. From the perspective of shallow geothermal energy utilization, this energy determines the theoretical geothermal potential (Bayer et al., 2019). This represents the heat in place and can be calculated based on the caloric equation of state for a water saturated homogeneous solid:
where n is the porosity of the solid, cw and cs in kJ (m3K)−1 are the volumetric heat capacities of water and solid, V (m3) is the reservoir volume and T (K) is the temperature of the reservoir. This equation can be condensed by distinction of a component related to material properties,
We also tested a different approach where the arithmetic mean difference profile is linearly fitted to each observation profile. This approach is based on standard statistical measures, as related spatial temperature profile analysis is scarce, there is no earlier work known following the same approach. In this model, profiles are calibrated for the lowest root-mean squared error (RMSE) between the reference profile and the measured ΔT profiles at each station. This allows for the possibility to get both a spatial and a total depth coverage at each observation well. The resulting profiles were integrated over the depths between 15 and 100 m and afterward interpolated via inverse distance weighting on a 1 km × 1 km grid with a total of 70,610 grid cells for the state of Bavaria.
Results and Discussion
Recent Variations in Air Temperature
Recent trends in air temperature are calculated from annual mean values of 23 air temperature stations, uniformly distributed over Bavaria (Figure 1A). Figure 1B shows the temperature record since 1960 normalized by the corresponding mean temperature of the reference period from 1960 to 1989 for each station. Annual mean air temperature records for individual stations exhibit a high year-to-year variability of up to 3 K, but also a high consistency of the trendlines among each other. This indicates that relative air temperature changes behave uniformly within the studied area and are forced by a large-scale climatic regime. Calculating a linear regression over longer periods allows to deduce the decadal temperature change rate (ΔT10), which is the slope of the linear regression per decade. For the study period of 1990–2019, the linear regression of these air temperature stations yields a slope of 0.35 ± 0.11 K (10a)−1. Compared to the linear regression slope of the previous 30 years period of 0.14 ± 0.07 K (10a)−1, the rate of temperature change for the study period is significantly elevated. The change in slope of the linear regression is consistent with the median temperature change of 1.06 K between 1990 and 2019 and the subsequent 30 years (1960–1989), which results in a decadal temperature change of also 0.35 K (10a)−1.
Recent Variations in Groundwater Temperature
To reveal subsurface temperature variations, we will first describe recent groundwater temperature variations in the wells individually. Recorded temperature profiles reflect a limited section of the subsurface. This section is limited by the groundwater table toward the top and the drilling depth of the observation well to the bottom. From the seasonal measurements 1992–4 period, we can confer seasonal temperature variation by depth. For depths shallower than 15 m, seasonal variations are higher than 0.1 K and thus measurable. Supplementary Figure S1 shows the mean values of the ranges (min-max differences) per depth at the selected 32 stations.
Vertical temperature changes below 15 m commonly follow the local geothermal gradient according to the basal heat flux from the interior of the Earth. They also conserve thermal signatures over a longer time period, e.g., from land-surface temperature changes (e.g., in urban areas (Banks et al., 2009) as well as from changes in regional groundwater temperatures – which closely reflects mean annual air temperatures in the recharge area (Burns et al., 2017)). Changes in repeated temperature profiles measured over timeframes of more than a year can therefore reveal long-term temperature variations, such as those related to global warming. The temperature difference, ΔT(z), between the 1992–4 and the 2019 period is calculated from mean temperature profiles for each time period (cf., Supplementary Figure S2). This yields ΔT-profiles for each individual station depicted in Figure 2A. To deal with alternating sampling dates in the 1992–4 period and for better comparability, the temperature change is normalized to the decadal temperature change rate (ΔT10) with respect to the time span between the periods. The decadal temperature change rates per depth are depicted as boxplots in Figure 2B. At a depth of 20 m, ΔT10 varies between −0.01 and 0.49 with a mean of 0.28 K (10a)−1. Toward greater depths, the mean ΔT10 decreases to 0.16, 0.09, 0.07, 0.05 K (10a)−1 at 40, 60, 80 and 100 m depth, respectively.
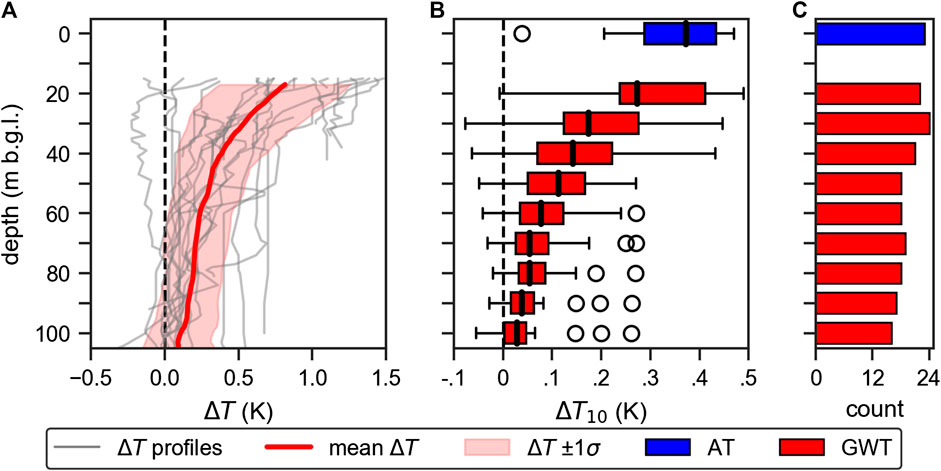
FIGURE 2. (A) Differences (ΔT) between the temperature profiles measured in 2019 vs. the mean signal of the time period from 1992 to 1994 (gray lines). The arithmetic mean difference (red line) is calculated as rolling mean with a window of 5 m and is displayed as red line along with the standard deviation (1σ) as red transparent area. (B) Decadal temperature change rate (ΔT10) for air temperature (AT, blue box) and groundwater temperature at different depths (GWT, red boxes). AT represent the slopes of ordinary least squared regression on annual mean values from 1990 to 2018. GWT rates are calculated from temperature-depth profiles measured in 2019 and 1992–1994. Whiskers are drawn for values smaller than 1.5 magnitudes of the interquartile range. Outliers are shown as dots. (C) Count of measurements at respective depths.
The proposed rates are in line with recent single depth groundwater observations from the western adjacent federal state of Baden-Württemberg and observations in Austria in the south. For Baden-Württemberg, Riedel (2019) reported ΔT10 rates of 0.1–0.4 K (10a)−1 based on a state-wide groundwater quality dataset for the period from 2000 to 2015. Riedel (2019) also found a quasi-depth dependency with temperatures in spring waters averaging at a higher ΔT10 rate of 0.3 K (10a)−1 over a rate of 0.2 K (10a)−1 in groundwater. In Austria, Benz et al. (2018b) observed a slightly higher temperature change rate of 0.7 ± 0.8 K over the 20-year period from 1994 to 2013 (ΔT10 ∼ 0.37 ± 0.42 K (10a)−1). In both studies, temperatures change rates are not linked to depths. Despite this it has to be noted that the average measurement depth in the Austrian wells is relatively shallow at 7 ± 4 m below ground surface.
Correlation Between Air and Groundwater Temperature Change Rates
To infer the correlation between air and groundwater temperature variations, the air temperature at each observation well is calculated by inverse distance weighting of the five nearest air temperature stations. Figure 3 shows the temperature change per decade for each of the 32 observation wells for air temperature and the mean value of 10 m increments of the individual temperature profiles. For the respective depths vs. air temperatures, Pearson correlation coefficients (r) indicate a moderate but robust correlation with values between 0.4 and 0.6. P-values exhibit that statistical significance is increasing with depth despite a high-variability. In general, higher temperature change rates in air temperature are reflected by higher temperature change rates in the subsurface. However, local variations are high both in the penetration depth of the signal and in absolute values. Note that air temperature change rates have a low standard deviation of 0.8 K, which makes it hard to identify distinct variations between temperature stations, as the atmospheric temperature signal is more homogeneous than that in the subsurface. The diffuse signal present in the subsurface is hereby hard to infer with the minor variations in air temperatures (Figure 3A). The correlation metrics are highest for the ΔT10 at 100 m and inferred air temperature. Assuming a process where the thermal field of the subsurface is mainly altered by a change in ground surface conditions (e.g., for shallow and unconfined aquifers), we would expect the contrary with decreasing metrics at greater depth induced by changing ground surface conditions. Obviously, correlating surface or air temperatures directly to subsurface temperatures presumes that changes in the temperature profile are a result of downward vertical heat transfer (advective or diffusive). If this is not the case, temperatures especially at greater depths could be altered by lateral heat transfer associated with the regional groundwater flow regime (Taniguchi et al., 1999; Zhu et al., 2015; Burns et al., 2016; Bense et al., 2020). In this case the temperature profile would carry an integrated signal dominated by annual mean temperatures of the recharge area. However, despite the potential influence of local hydrogeological conditions, it is also possible that the observed discrepancy is an artifact of the statistical analysis as the number of observations decreases with depth (from 24 to 16).
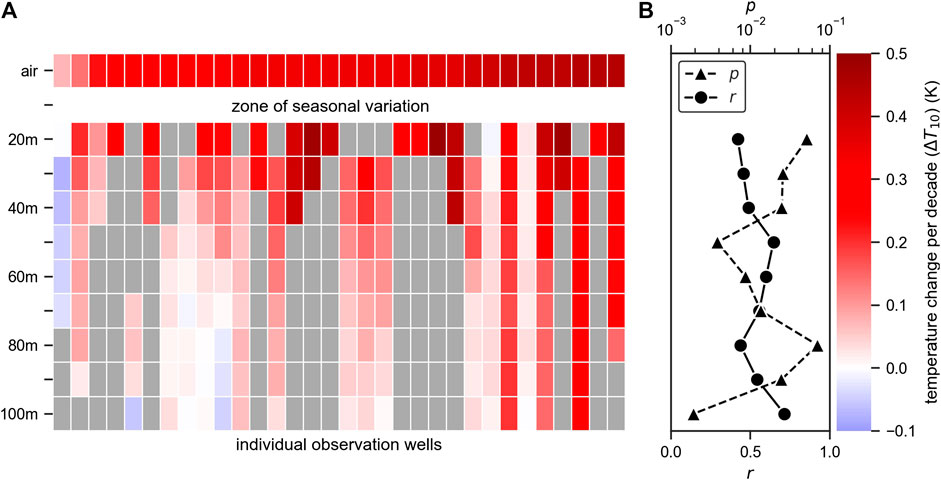
FIGURE 3. (A) Heatmap showing the temperature change per decade (ΔT10) for air temperature (AT) of the period from 1990 to 2018 and for groundwater temperature (GWT) calculated from temperature-depth profiles measured in 2019 and 1992–1994. Air temperature is inferred for each observation well location. Values at 10 m depth have been masked for showing high seasonal variability. Observation wells are sorted by their AT lapse rates. Empty cells are displayed in gray. (B) Pearson correlation coefficient (r) and p-value (p) for GWT at each depths.
The substantial number of wells included in this study allows robust average estimates. Compared with the corresponding aboveground air temperatures, as expected, the decadal temperature increase is dampened in the subsurface. The median values for Bavaria are ΔT10 = 0.35 K for the atmosphere, 0.28 K at 20 m and 0.09 K at 60 m depth. This means that at the deepest level, the changes, however, are close to measurement accuracy. Temperatures in air and subsurface show a moderate to good correlation, with higher scattering of groundwater than air temperatures. Major tendencies of air temperature are also reflected in the subsurface temperature record. This supports a good statistical basis of the measured data, even without detailed analysis of the site-specific heat transport processes.
Shallow Geothermal Potential of Recent Temperature Variations
The changes in subsurface temperatures also constitute an increase in thermal energy stored in the subsurface in response to recent shifts in the thermal regime of surface conditions. This additional heat can be accessed via shallow geothermal systems and thus increases the theoretical geothermal potential of the shallow subsurface. To accurately quantify this additional heat, both geological material properties and the thermal field have to be characterized at a high level. However, thermal properties such as heat capacity do not vary over a broad range in geological media (Stauffer et al., 2013). If we assume bulk material properties and superimpose an arithmetic mean ΔT profile over the depth interval between 15 and 100 m below surface, we can calculate a rough estimate of the magnitude of the heat flow into the subsurface. Table 1 lists the energy stored per year for a minimum, a maximum and median (50%) assumption of porosity (0.5–0.15) and volumetric heat capacity of the solid (1900–2,500 kJ (m3K)−1). The energy stored per year varies between 155 (±125) and 212 (±171) PJ between the minimum and maximum scenarios (±1σ). For the median scenario the thermal energy stored by climate change in the subsurface per year equals 184 (±149) PJ. Compared to the primary energy demand (1944 PJ) and the heating demand (669.7 PJ) in 2017 (Ebert and Voigtländer, 2019), the energy stored per year in the subsurface for the median scenario equals 9.5% of the annual primary energy demand or 27.5% of the heating demand of Bavaria. This simplistic calculation, however, does not consider spatial variations and relies on the arithmetic mean differences of all profiles.
For an alternative approach where the arithmetic mean difference profile is linearly fitted to each observation profile, stored energies for the minimum and maximum scenario are 208 and 285 PJ with RMSEs of 40 and 54 PJ, accordingly. The median scenario yields a thermal energy input of 248 PJ with an RMSE of 47 PJ, which would equal 12.8% of the Bavarian primary energy demand or 37% of the heating demand. Supplementary Figures S3, S4 display the profile fitting and the spatial distribution of interpolated groundwater and air temperatures. Note that the spatial coverage of the observation wells with respect to the area of Bavaria is insufficient to produce fully reliable numbers, but it provides a first rough estimate of the order of magnitude of energy stored in the subsurface annually. Still, the presented spatial approach offers the opportunity to employ more precise regionalized material parameters for heterogeneous hydrogeological facies. In essence, we found the thermal energy stored by climate change in the subsurface per year to be in the magnitude of 10% of the total Bavarian primary energy demand, or one third of the heating demand. This continuous heat transfer represents an enormous replenishing resource that is fueled by climate change. The results from this local study are expected be applicable in similar magnitudes on a global scale as observed atmospheric temperature trends in Bavaria are in line with the global trend and the thermal connection between the surface and shallow subsurface has been proven to be robust also on a global scale (Benz et al., 2017).
Conclusion
The scope of this study was to extract regional long-term trends of groundwater temperature, and for this purpose, well profiles re-measured after up to 27 years were compared to each other. In order to remove as much as possible momentary, local variability, we compared profiles that were obtained as averaged logs from repeated campaigns throughout each year. The total number of suitable wells was 32 which cover broadly the entire state of Bavaria, Germany, and these offered a substantial insight into the shallow thermal regime in the subsurface. Clearly, each profile was different, influenced by the local hydrogeological, climatic and potentially anthropogenic conditions. However, short term variability and seasonality was shown to be marginal for these wells beneath 15 m, and this is where long-term climate change left an observable thermal imprint. Nearly all wells revealed increasing groundwater temperatures. The increase and the discrepancy among the well profiles was largest at shallow depth, and a maximum warming of 1.5 K was observed. Only one well showed a slight cooling by 0.2 K, but on average, 0.7 K warmer groundwater is found at 15 m depth which declines with depth along the profile.
Over the past ∼30 years, the warming climate transferred heat in the subsurface at a rate that is estimated roughly more than one quarter of the state’s annual heating demand. Rising ground (water) temperatures increase shallow geothermal system efficiency, and accelerated ground heat flux represents a form of thermal recharge of shallow geothermal reservoirs. This ground heat gain thus can be considered as favourable for geothermal use. In contrast, groundwater ecosystems used to nearly static thermal conditions need to adjust and may be under increasing stress. Even if the changes are considered marginal so far, and impacts are considered acceptable, the groundwater temperature will further increase as delayed response to the past temperature changes, and are likely to rise further in response to future atmospheric warming.
Data Availability Statement
The datasets presented in this study can be found in online repositories. The names of the repository/repositories and accession number(s) can be found in the article/Supplementary Material.
Author Contributions
All authors contributed equally to this work.
Funding
This work is funded by the German Research Foundation, DFG (grant number BA2850/3-1).
Conflict of Interest
The authors declare that the research was conducted in the absence of any commercial or financial relationships that could be construed as a potential conflict of interest.
Acknowledgments
We thank Jakob Michael for language editing. We would also like to thank Leonhard Zimmerer and Rafael Meinhardt for their great support during the field measurements and Tanja Mast (TH Ingolstadt) for her valuable insights and advice on energy demand statistics of Bavaria. Additionally, we thank Thies Rixen and Christian Albrecht (WWA Aschaffenburg), Simon Kirner (WWA Bad Kissingen), Siegfried Brunner (WWA Deggendorf), Verena Bagehorn and Georg Lindner (WWA Hof), Ralf Ottmann (WWA Ingolstadt), Angelika Babl (WWA Kempten), Max Pöhlmann (WWA Kronach), Franz Schröger (WWA Landshut), Patrick Bahner (WWA München), Norbert Galambos (WWA Nürnberg), Klaus Moritz and Michael Gottwald (WWA Rosenheim), Jürgen Festbaum (WWA Weiden), Katrin Knief and Ralf Ostertag (WWA Weilheim) for help with locating wells, granting access and sharing (meta-)data on the observation wells.
Supplementary Material
The Supplementary Material for this article can be found online at: https://www.frontiersin.org/articles/10.3389/feart.2020.575894/full#supplementary-material Temperature-depth profiles are also available at: https://doi.pangaea.de/10.1594/PANGAEA.923529
References
Alam, S., Gebremichael, M., Li, R., Dozier, J., and Lettenmaier, D. P. (2019). Climate change impacts on groundwater storage in the Central Valley, California. Climatic Change 157, 387–406. doi:10.1007/s10584-019-02585-5
Banks, D., Gandy, C. J., Younger, P. L., Withers, J., and Underwood, C. (2009). Anthropogenic thermogeological 'anomaly' in Gateshead, Tyne and Wear, UK. Q. J. Eng. Geol. Hydrogeol. 42, 307–312. doi:10.1144/1470-9236/08-024
Bayer, P., Attard, G., Blum, P., and Menberg, K. (2019). The geothermal potential of cities. Renew. Sustain. Energy Rev. 106, 17–30. doi:10.1016/j.rser.2019.02.019
Bense, V. F., and Kurylyk, B. L. (2017). Tracking the subsurface signal of decadal climate warming to quantify vertical groundwater flow rates. Geophys. Res. Lett. 44 (12), 244–253. doi:10.1002/2017gl076015
Bense, V. F., Kurylyk, B. L., van Daal, J., van der Ploeg, M. J., and Carey, S. K. (2017). Interpreting repeated temperature-depth profiles for groundwater flow. Water Resour. Res. 53, 8639–8647. doi:10.1002/2017wr021496
Bense, V., Kurylyk, B., de Bruin, J., and Visser, P. (2020). Repeated subsurface thermal profiling to reveal temporal variability in deep groundwater flow conditions. Water Resour. Res. 56, e2019WR026913. doi:10.1029/2019wr026913
Benz, S. A., Bayer, P., Goettsche, F. M., Olesen, F. S., and Blum, P. (2016). Linking surface urban heat islands with groundwater temperatures. Environ. Sci. Technol. 50, 70–78. doi:10.1021/acs.est.5b03672
Benz, S. A., Bayer, P., and Blum, P. (2017). Global patterns of shallow groundwater temperatures. Environ. Res. Lett. 12, 034005. doi:10.1088/1748-9326/aa5fb0
Benz, S. A., Bayer, P., Blum, P., Hamamoto, H., Arimoto, H., and Taniguchi, M. (2018a). Comparing anthropogenic heat input and heat accumulation in the subsurface of Osaka, Japan. Sci. Total Environ. 643, 1127–1136. doi:10.1016/j.scitotenv.2018.06.253
Benz, S. A., Bayer, P., Winkler, G., and Blum, P. (2018b). Recent trends of groundwater temperatures in Austria. Hydrol. Earth Syst. Sci. 22, 3143. doi:10.5194/hess-22-3143-2018
Bloomfield, J. P., Jackson, C. R., and Stuart, M. E. (2013). Changes in groundwater levels, temperature and quality in the UK over the 20th century: an assessment of evidence of impacts from climate change.
Bloomfield, J. P., Marchant, B. P., and McKenzie, A. A. (2019). Changes in groundwater drought associated with anthropogenic warming. Hydrol. Earth Syst. Sci. 23, 1393–1408. doi:10.5194/hess-23-1393-2019
Bredehoeft, J. D., and Papaopulos, I. S. (1965). Rates of vertical groundwater movement estimated from the Earth’s thermal profile. Water Resour. Res. 1, 325–328. doi:10.1029/wr001i002p00325
Burns, E. R., Ingebritsen, S. E., Manga, M., and Williams, C. F. (2016). Evaluating geothermal and hydrogeologic controls on regional groundwater temperature distribution. Water Resour. Res. 52, 1328–1344. doi:10.1002/2015wr018204
Burns, E. R., Zhu, Y., Zhan, H., Manga, M., Williams, C. F., Ingebritsen, S. E., et al. (2017). Thermal effect of climate change on groundwater-fed ecosystems. Water Resour. Res. 53, 3341–3351. doi:10.1002/2016wr020007
Colombani, N., Giambastiani, B. M. S., and Mastrocicco, M. (2016). Use of shallow groundwater temperature profiles to infer climate and land use change: interpretation and measurement challenges. Hydrol. Process. 30, 2512–2524. doi:10.1002/hyp.10805
Coluccio, K., and Morgan, L. K. (2019). A review of methods for measuring groundwater-surface water exchange in braided rivers. Hydrol. Earth Syst. Sci. 23, 4397–4417. doi:10.5194/hess-23-4397-2019
Constantz, J. (2008). Heat as a tracer to determine streambed water exchanges. Water Resour. Res. 44, W00D10. doi:10.1029/2008wr006996
Earman, S., and Dettinger, M. (2011). Potential impacts of climate change on groundwater resources - a global review. J. Water Clim. Change 2, 213–229. doi:10.2166/wcc.2011.034
Ebert, M., and Voigtländer, C. (2019). Aktuelle Zahlen zur Energieversorgung in Bayern - prognose für das Jahr 2017. Bayerisches Staatsministerium für Wirtschaft, Landesentwicklung und Energie.
Epting, J., Scheidler, S., Affolter, A., Borer, P., Mueller, M. H., Egli, L., et al. (2017). The thermal impact of subsurface building structures on urban groundwater resources–a paradigmatic example. Sci. Total Environ. 596-597, 87–96. doi:10.1016/j.scitotenv.2017.03.296
Ferguson, G., and Woodbury, A. D. (2004). Subsurface heat flow in an urban environment. J. Geophys. Res. Solid Earth 109, B02402 doi:10.1029/2003jb002715
Green, T. R., Taniguchi, M., Kooi, H., Gurdak, J. J., Allen, D. M., Hiscock, K. M., et al. (2011). Beneath the surface of global change: impacts of climate change on groundwater. J. Hydrol. 405, 532–560. doi:10.1016/j.jhydrol.2011.05.002
Griebler, C., Avramov, M., and Hose, G. (2019). “Groundwater ecosystems and their services: current status and potential risks,” in Atlas of Ecosystem Services. New York, NY: Springer, 197–203.
Gunawardhana, L. N., and Kazama, S. (2011). Climate change impacts on groundwater temperature change in the Sendai plain, Japan. Hydrol. Process. 25, 2665–2678. doi:10.1002/hyp.8008
Hemmerle, H., Hale, S., Dressel, I., Benz, S. A., Attard, G., Blum, P., et al. (2019). Estimation of Groundwater Temperatures in Paris, France. Geofluids 2019, 1–11. doi:10.1155%2F2019%2F5246307
Jarsjö, J., Andersson-Sköld, Y., Fröberg, M., Pietroń, J., Borgström, R., Löv, Å., et al. (2020). Projecting impacts of climate change on metal mobilization at contaminated sites: controls by the groundwater level. Sci. Total Environ. 712, 135560. doi:10.1016/j.scitotenv.2019.135560
Jyväsjärvi, J., Marttila, H., Rossi, P. M., Ala-Aho, P., Olofsson, B., Nisell, J., et al. (2015). Climate-induced warming imposes a threat to north European spring ecosystems. Global Change Biol. 21, 4561–4569. doi:10.1111/gcb.13067
Kløve, V. P., Doornenbal, P. J., Kooi, H., Peter Broers, H., and de Louw, P. G. B. (2019). Temperature buffering by groundwater in ecologically valuable lowland streams under current and future climate conditions. J. Hydrol. 3, 100031. doi:10.1016/j.hydroa.2019.100031
Kløve, B., Ala-Aho, P., Bertrand, G., Gurdak, J. J., Kupfersberger, H., Kværner, J., et al. (2014). Climate change impacts on groundwater and dependent ecosystems. J. Hydrol. 518, 250–266. doi:10.1016%2Fj.jhydrol.2013.06.037
Kurylyk, B. L., and Irvine, D. J. (2019). Heat: An overlooked tool in the practicing hydrogeologist's toolbox. Groundwater 57, 517–524. doi:10.1111/gwat.12910
Kurylyk, B. L., Bourque, C., and MacQuarrie, K. T. (2013). Potential surface temperature and shallow groundwater temperature response to climate change: an example from a small forested catchment in east-central New Brunswick (Canada). Hydrol. Earth Syst. Sci. 10, 2701–2716. doi:10.5194%2Fhessd-10-3283-2013
Kurylyk, B. L., MacQuarrie, K. T. B., and McKenzie, J. M. (2014). Climate change impacts on groundwater and soil temperatures in cold and temperate regions: Implications, mathematical theory, and emerging simulation tools. Earth Sci. Rev. 138, 313–334. doi:10.1016/j.earscirev.2014.06.006
Kurylyk, B. L., Irvine, D. J., and Bense, V. F. (2019). Theory, tools, and multidisciplinary applications for tracing groundwater fluxes from temperature profiles. WIREs Water 6, e1329. doi:10.1002/wat2.1329
Leonhardt, G., Seifert, L., Gerecke, R., Müller, J., Hotzy, R., and Lotz, A. (2017). “Springs in the Bavarian National Parks as indicators for climate change,” in 6th Symposium for Research in Protected Areas, Salzburg, AT, November 2–3, 2017, 369–372.
Li, S., Dong, L., Chen, J., Li, R., Yang, Z., and Liang, Z. (2019). Vertical groundwater flux estimation from borehole temperature profiles by a numerical model, RFLUX. Hydrol. Process. 33, 1542–1552. doi:10.1002/hyp.13420
Mammola, S., Piano, E., Cardoso, P., Vernon, P., Domínguez-Villar, D., Culver, D. C., et al. (2019). Climate change going deep: the effects of global climatic alterations on cave ecosystems. Anthr. Rev. 6, 98–116. doi:10.1177/2053019619851594
Maxwell, R. M., and Kollet, S. J. (2008). Interdependence of groundwater dynamics and land-energy feedbacks under climate change. Nat. Geosci. 1, 665–669. doi:10.1038/ngeo315
Menberg, K., Bayer, P., Zosseder, K., Rumohr, S., and Blum, P. (2013). Subsurface urban heat islands in German cities. Sci. Total Environ. 442, 123–133. doi:10.1016/j.scitotenv.2012.10.043
Menberg, K., Blum, P., Kurylyk, B. L., and Bayer, P. (2014). Observed groundwater temperature response to recent climate change. Hydrol. Earth Sys. Sci. 18, 4453–4466. doi:10.5194/hess-18-4453-2014
Molina-Giraldo, N., Bayer, P., Blum, P., and Cirpka, O. A. (2011). Propagation of seasonal temperature signals into an aquifer upon bank infiltration. Ground Water 49, 491–502. doi:10.1111/j.1745-6584.2010.00745.x
Pollack, H. N., and Chapman, D. S. (1993). Underground records of changing climate. Sci. Am. 268, 44–50. doi:10.1038/scientificamerican0693-44
Rau, G. C., Andersen, M. S., McCallum, A. M., and Acworth, R. I. (2010). Analytical methods that use natural heat as a tracer to quantify surface water-groundwater exchange, evaluated using field temperature records. Hydrogeol. J. 18, 1093–1110. doi:10.1007/s10040-010-0586-0
Riedel, T. (2019). Temperature-associated changes in groundwater quality. J. Hydrol. 572, 206–212. doi:10.1016/j.jhydrol.2019.02.059
Saar, M. O. (2011). Review: Geothermal heat as a tracer of large-scale groundwater flow and as a means to determine permeability fields. Hydrogeol. J. 19, 31–52. doi:10.1007/s10040-010-0657-2
Sorey, M. L. (1971). Measurement of vertical groundwater velocity from temperature profiles in wells. Water Resour. Res. 7, 963–970. doi:10.1029/wr007i004p00963
Stauffer, F., Bayer, P., Blum, P., Giraldo, N. M., and Kinzelbach, W. (2013). Thermal use of shallow groundwater: Boca Raton, FL:CRC Press.
Stoll, S., Hendricks Franssen, H. J., Butts, M., and Kinzelbach, W. (2011). Analysis of the impact of climate change on groundwater related hydrological fluxes: a multi-model approach including different downscaling methods. Hydrol. Earth Syst. Sci. 15, 21–38. doi:10.5194/hess-15-21-2011
Taniguchi, M. (1993). Evaluation of vertical groundwater fluxes and thermal properties of aquifers based on transient temperature-depth profiles. Water Resour. Res. 29, 2021–2026. doi:10.1029/93wr00541
Taniguchi, M., Shimada, J., Tanaka, T., Kayane, I., Sakura, Y., Shimano, Y., et al. (1999). Disturbances of temperature-depth profiles due to surface climate change and subsurface water flow: 1. An effect of linear increase in surface temperature caused by global warming and urbanization in the Tokyo Metropolitan Area, Japan. Water Resour. Res. 35, 1507–1517. doi:10.1029/1999wr900009
Tissen, C., Benz, S. A., Menberg, K., Bayer, P., and Blum, P. (2019). Groundwater temperature anomalies in central Europe. Environ. Res. Lett. 14, 104012. doi:10.1088/1748-9326/ab4240
Wang, K., and Lewis, T. J. (1992). Geothermal evidence from Canada for a cold period before recent climatic warming. Science 256, 1003–1005. doi:10.1126/science.256.5059.1003
World Meteorological Organization (2017). WMO guidelines on the calculation of climate normals. Geneva, Switzerland; World Meteorological Organization.
Zhang, Z., Li, Y., Barlage, M., Chen, F., Miguez-Macho, G., Ireson, A., et al. (2020). Modeling groundwater responses to climate change in the Prairie Pothole Region. Hydrol. Earth Syst. Sci. 24, 655–672. doi:10.5194/hess-24-655-2020
Keywords: groundwater temperature, subsurface, climate change, water resources, shallow geothermal
Citation: Hemmerle H and Bayer P (2020) Climate Change Yields Groundwater Warming in Bavaria, Germany. Front. Earth Sci. 8:575894. doi: 10.3389/feart.2020.575894
Received: 24 June 2020; Accepted: 14 October 2020;
Published: 13 November 2020.
Edited by:
Nils Moosdorf, Leibniz Centre for Tropical Marine Research (LG), GermanyReviewed by:
Dylan Irvine, Flinders University, AustraliaCorinna Abesser, British Geological Survey (BGS), United Kingdom
Copyright © Hemmerle and Bayer. This is an open-access article distributed under the terms of the Creative Commons Attribution License (CC BY). The use, distribution or reproduction in other forums is permitted, provided the original author(s) and the copyright owner(s) are credited and that the original publication in this journal is cited, in accordance with accepted academic practice. No use, distribution or reproduction is permitted which does not comply with these terms.
*Correspondence: Hannes Hemmerle, aGFubmVzLmhlbW1lcmxlQGdlby51bmktaGFsbGUuZGU=