- 1Istituto Nazionale di Geofisica e Vulcanologia, Palermo, Italy
- 2Northwest Institute of Eco-Environment and Resources, Chinese Academy of Sciences (CAS), Lanzhou, China
- 3Key Laboratory of Petroleum Resource Research, Lanzhou, China
- 4Consiglio Nazionale delle Ricerche (CNR), Istituto di Geoscienze e Georisorse (IGG), Pisa, Italy
- 5School of Engineering, University of Basilicata, Potenza, Italy
- 6Consiglio Nazionale delle Ricerche (CNR), Istituto di Metodologie per Analisi Ambientale (IMAA), Tito Scalo (Potenza), Italy
Central Apennines are one of the highest seismic risk regions in Italy. A number of energetic events (
Introduction
Central Italy is affected by intense crustal deformation processes driven by the relative motion of the African and the Eurasian plates. In this region, active extension occurs along the axial and western zones of the Apennine mountainous belt (backarc extension area, characterized by crustal thinning and active volcanism toward the Tyrrhenian Sea). Conversely, compression mostly occurs along the frontal part of the belt, toward the Adriatic Sea (contraction area, associated with the eastward propagation of the Apennine accretionary prism). Strain values of 1.5–2 cm/year are currently estimated all over the extensive belt. An array of northwest-striking (i.e., northeast- and southwest-dipping) normal and normal-oblique faults control regional seismicity, so that earthquake foci are prevalently distributed along the Apennines axis (e.g., Doglioni, 1991; Patacca and Scandone, 2001; Patacca et al., 2008; Montone and Mariucci, 2016).
Since the late 1970s, carbon dioxide emissions have been recognized to be associated on a global scale with areas of intense tectonic activity and/or extensive seismicity (e.g., Barnes et al., 1978; Irwin and Barnes, 1980; Tamburello et al., 2018). Large stresses associated with earthquakes and crustal deformations are known to cause measurable changes in the physical properties of rocks and significant hydrologic perturbations in the connected aquifers (e.g., Rojstaczer et al., 1995). Major hydrologic effects observed worldwide include spring and stream flow variations, groundwater level oscillations, triggered eruptions of mud volcanoes, gas emissions, modifications of the geochemical signature of spring and/or well waters (e.g., among many others, Wakita, 1975; King et al., 1981; Sato et al., 1986; Roeloffs, 1988; Roeloffs et al., 1989; Toutain and Baubron, 1999; King et al., 2006; Pierotti et al., 2015). Martinelli and Dadomo (2018) recently evidenced that a large part of possible geochemical precursory phenomena recorded worldwide have been found in areas characterized by i) shallow seismicity, ii) relatively high heat flux, and iii) significant crustal deformation rates. All these features are characteristics of the study area (e.g., Chiodini et al., 2004; Chiodini et al., 2011; Chiodini et al., 2020).
Moderate-magnitude earthquakes (4.0 <
Significant variations in the chemical composition of dissolved gases (e.g., Italiano et al., 2004), and in water level, temperature and chemical composition of water (Lapenna et al., 2004) were detected in thermal waters of Umbria region, Central Italy, during the 1997–1998 seismic sequence (Colfiorito
Variations of the geomagnetic field (from satellites and observatories), and of atmospheric climatological data recorded during the 2015–2016 period have been retrospectively investigated to search for possible lithosphere-atmosphere-ionosphere coupled effects during the preparatory phase of the Central Italy seismic sequence 2016–2017, and a few anomalies in electromagnetic parameters were identified over the year preceding the Amatrice earthquake of August 24th, 2016 (Marchetti et al., 2019). Satellite-based techniques (TIR index) captured possible intense and large scale degassing phenomena that occurred in concomitance with the 1997–1998 seismic sequence (Aliano et al., 2008). Further pieces of evidence of degassing during the 2009 seismic sequence were recorded by satellite techniques (TIR index; Genzano et al., 2009; Bonfanti et al., 2012; and references therein). Seismic sequences in Central Italy have been accompanied by crustal deformation processes (e.g., Cheloni et al., 2017). In some cases, the beginning of the crustal deformation processes was detected before the start of the sequences (Bella et al., 1995; Sgrigna and Malvezzi, 2003; Moro et al., 2017). Under these conditions, any geochemical precursor possibly detected by ground and/or satellite techniques, could be theoretically attributable to CO2–driven crustal permeability variations able to induce volume and shape changes in CO2 reservoirs. These flux variations have the potential to modify the chemical composition of fluids hosted in deep and shallow aquifers. This mechanism is particularly effective in areas where natural manifestations (mostly mofettes) with high-CO2 discharge rate occur (e.g., Chiodini et al., 2008; Figure 1).
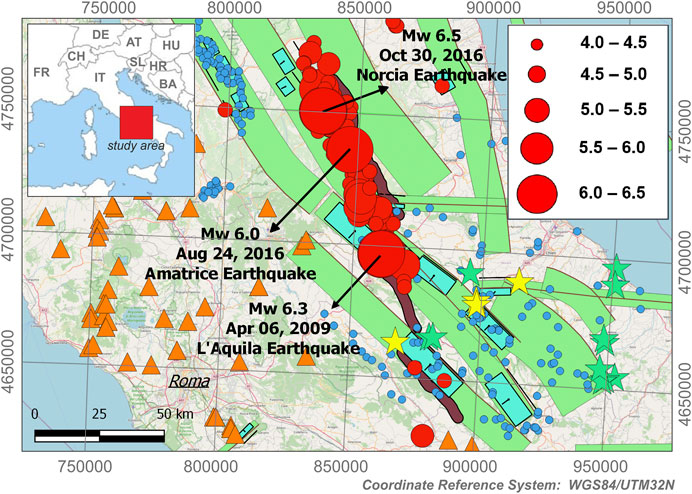
FIGURE 1. Location of earthquakes with
In order to better understand the possible relations between local seismicity, groundwater chemistry, and CO2 degassing activity, geochemical data recorded by several Regional Agencies for the Environmental Protection (ARPA) located in Central Italy were analyzed (the geographic coordinates of the water points of the ARPA network are given in Table 1 of the Supplementary Material). Furthermore, Thermal InfraRed spectral range radiations (TIR) attributable to fluctuations in CO2 degassing rate (e.g., Tramutoli et al., 2013) were recorded by satellite techniques, and compared with other fluid-related parameters (pH, chemical composition of major constituents,
Geological and Seismological Setting
The study area is located in the Central Apennines. The Apennine chain is the result of the opening of the Tyrrhenian sea that induced the eastward migration of a compressive front (e.g., Vai and Martini, 2001). Areas previously controlled by compressive tectonics were affected by back-arc extension due to the migration of the compressive front eastwards since the early Miocene. Crustal thinning due to extension in the Tyrrhenian area induced volcanic activities along the western margin of Italy. This region is characterized by high heat flow, positive gravity anomalies and shallow earthquakes due to the presence of a relatively thin crust (0–25 km). The eastern compressive Adriatic area is characterized by relatively low heat flow values, negative gravity anomalies and relatively high crustal thickness (30–35 km). Seismicity follows tectonic boundaries of distensive and compressive domains. All seismic sequences occurred in Central Apennines in past twenty years have been characterized by the occurrence of multiple events represented by
The main shock of the L'Aquila seismic event was preceded by a foreshock sequence that lasted four months, and was followed by an aftershock sequence that lasted eight months (Valoroso et al., 2013). The hypocenter of this earthquake was found along the Paganica-San Demetrio fault, a southwestward dipping fault segment intermediate between the eastern and the western major fault systems recognized in the Abruzzo sector of Central Apennines. The L'Aquila earthquake broke a ∼15–18 km long NW–SE trending fault, mainly confined to the upper 10 km of the crust (Serpelloni et al., 2012, and references therein). The area is characterized by a baseline heat flux of about 60
The Amatrice earthquake started on August 24th, 2016, with a
Methods
For the present study, we propose a novel approach (see Cicerone et al., 2009, for a review of previous monitoring techniques) that combines groundwater monitoring and satellite techniques to indirectly evaluate variations in the stress field, and possibly examine the effects due to the crustal deformation processes associated with such variations. Groundwater monitoring techniques were applied to detect hydrogeochemical anomalies (e.g., Gherardi and Pierotti, 2018; Martinelli and Dadomo, 2018), whereas satellite techniques were applied to detect time-space variations in thermal ground emissions (e.g., Tramutoli et al., 2013). The main advantage of the proposed approach is that it allows for i) identifying possible anomalies over large areas, thanks to the application of satellite techniques, and ii) for independently verifying these anomalies on a local scale, by ground-based inspection of the relevant geochemical parameters, with beneficial results for diagnostic accuracy.
Hydrogeochemical monitoring consisted of the discrete monitoring of dissolved CO2 on a large number of water points belonging to the ARPA (Regional Environmental Protection Agency of Italy) network (see Table 1 of the Supplementary Material). In particular, we processed a subset of 114 chemical analyses collected from springs and wells roughly distributed within and around the area of greatest damage caused by the 2016 seismic events, in the Umbria and Abruzzo regions. Finally, the MSG-SEVIRI (Meteosat Second Generation - Spinning Enhanced Visible and Infrared Imager) night-time TIR images collected over the Italian region during the 2004–2017 period were analyzed with the RST (Robust Satellite Techniques) methodology (Tramutoli, 1998; Tramutoli, 2005).
Robust Satellite Techniques Methodology and Robust Estimator of Thermal InfraRed Anomalies Index
The Earth's thermally emitted radiation measured by satellite sensors operating in the Thermal InfraRed (8–14 μm) spectral range (TIR) was considered in its apparent relation with the seismic events. The most recent literature (e.g., Tramutoli et al., 2013) now supports the hypothesis that a relationship could exist between positive TIR anomalies, and the release of volatiles originally stored at depth in rocks, as a result of stress field variations. Along with Rn, optically active gases like CO2 and CH4 are considered the gases most likely involved in this process. The areal extension and the geometric distribution of the thermal anomalies detected by the satellite likely also depends on the relative density of the emitted gas: the larger the amount of heavier-than-air gases present, the smaller the effect of atmospheric dispersion, and the more focused the detected thermal anomaly. On the reverse, the predominant release of gases lighter than air would produce diffusion-like patterns of the thermal anomalies. The RST (Robust Satellite Techniques) approach proposed by Tramutoli (1998), Tramutoli (2005), and Tramutoli (2007) was adopted to isolate possible TIR anomalies from other signal variations related to natural and/or observational factors that can be responsible of “false” proliferations. The methodology was already used to study the different seismic phases of earthquakes with a wide range of magnitudes (from 4.0 to 7.9), and occurred in different geotectonic settings (compressive, extensional and transcurrent). More details can be found in Tramutoli et al. (2015); Tables 3 and 4 of the Supplementary Material).
The RST methodology identifies space-time anomalies with respect to a preliminarily defined “normal” (i.e., under unperturbed conditions) signal behaviour, which is achievable by the analysis of long-term series of satellite records. TIR transients normally affect areas of several thousand square kilometers and can be detected up to several hundred kilometers far from the epicenter zone, some days to almost one month prior to a seismic event, and can last for a few days after the seismic event.
For seismic monitoring prone areas, TIR anomalies are identified by using the specific index RETIRA (Robust Estimator of TIR Anomalies; Filizzola et al., 2004; Tramutoli et al., 2005). The RETIRA index is computed on the image at hand only over locations not affected by cloudiness1, using the following equation:
where:
The RETIRA index is intrinsically not protected by the proliferation of signal outliers, and TIR anomalies are subject to a space-time persistence analysis (e.g., Tramutoli et al., 2015). Such analysis is devoted to discriminating significant anomalies from well-known spurious effects (e.g., local warming due to cloud passages, error in image navigation/co-location process; see Aliano et al., 2008; Eleftheriou et al., 2016; Genzano et al., 2015).
Finally, it should be noted that by using the RETIRA index, the effects of natural (e.g., topography and land cover) and observational (e.g., solar illumination and satellite view angle) conditions are minimized, and the occasional warming due to daily variations and/or season time-drifts (i.e., inter-annual changes) are also reduced (see Tramutoli et al., 2005, for more details).
Results and Discussion
Discrete Monitoring of CO2 in Selected Cold Aquifers of Central Italy
Two major areas of CO2 earth degassing have been identified in Central-Southern Italy (Chiodini et al., 2004): i) the Tyrrhenian hinterland, where CO2 is directly released into the atmosphere predominantly by two degassing structures, the so-called Tuscan-Roman and Campanian degassing structure, respectively; ii) the Adriatic foreland, where CO2 is predominantly dissolved into groundwaters circulating through major carbonate aquifers hosted along the Apennine mountainous range. The central-southern part of Apennines is a highly active seismic area, and some authors (e.g., Chiodini et al., 2004; Chiodini et al., 2018) have speculated that the existence of high-pressure, CO2-rich fluid pockets at depth may play a major role in the generation of Apennine earthquakes. Groundwater circulation patterns in Central Italy have been described by Boni et al., (1986) and Petitta et al. (2018), and references therein), among others. From these studies, it emerged that groundwater hosted in the vadose zone is affected by fast vertical flow, while significant groundwater flow toward local springs with relatively high discharge values is generally driven by the relatively high thickness of the saturated zone.
A number of springs, and wells 100 to 200 m deep, are currently monitored for environmental purposes by the Regional Environmental Protection Agencies (ARPA) of Central Italy. These manifestations are sampled for chemical analyses two to four times per year, according to the needs of local governments. All the samples have a calcium-bicarbonate chemical composition, low salinity, and outlet temperatures close to the average annual air temperature. The goal of the survey was to quantify the contents of CO2 dissolved in the waters, and to compare any possible anomaly in CO2 contents with seismic activity over time. Carbon dioxide fugacity values were calculated with the PHREEQC code (Parkhurst and Appelo, 2013) from the analytical concentration of total inorganic dissolved carbon and pH values measured in the field. Noteworthy, a quite high
Time series of recalculated
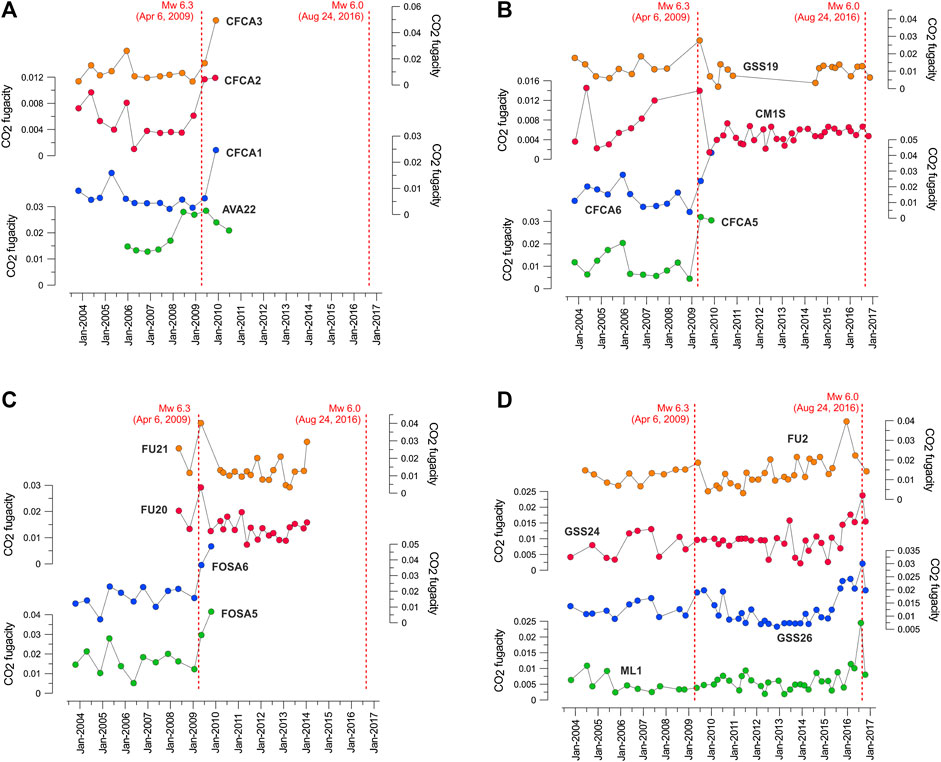
FIGURE 2. Temporal trends (2004–2017) of
Thermal InfraRed Anomalies
Daily TIR images collected at night-time2 (time slot 00:00÷00:15 GMT) by MSG-SEVIRI satellite (sensor with spatial resolution of ∼3.5 × 3.5 km) over the Italian region in the period 2004–2017 were analyzed by means RST methodology and RETIRA index, with the aim to discern TIR anomalies possibly related to seismic process (Figures 3, 4).
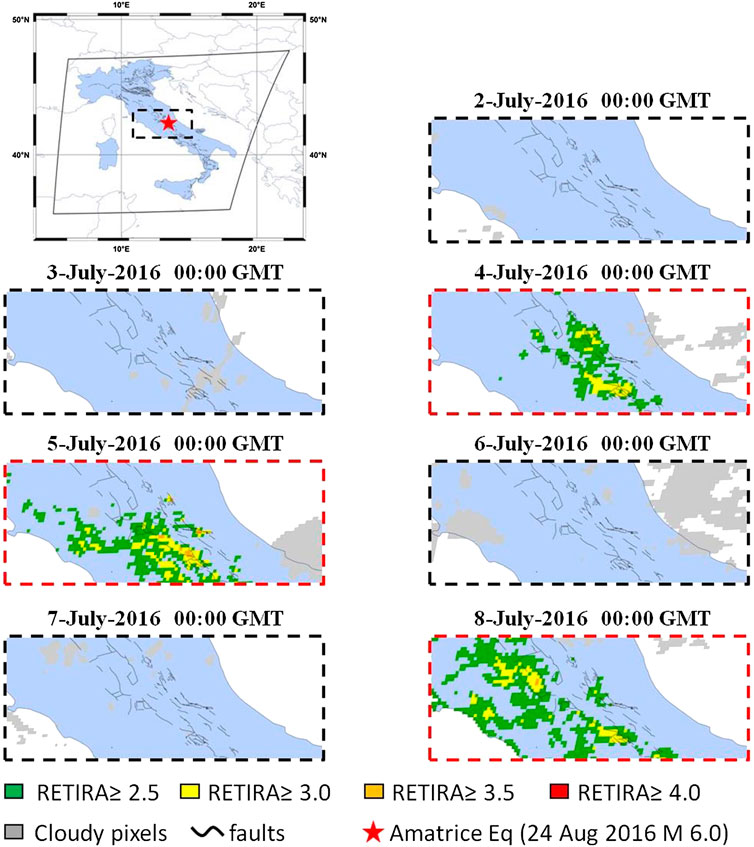
FIGURE 3. Example of sequence of TIR anomalies. The RETIRA index over Central Italy at the time of Amatrice earthquake (August 24, 2016,
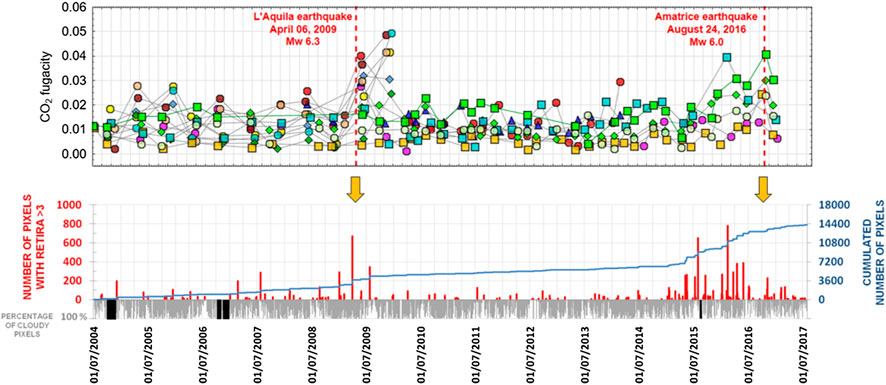
FIGURE 4. Comparison between time variations of
In order to discriminate significant anomalies from residual well-known spurious effects (outliers, geo-location errors, night-time warm cloud passages and asymmetric distribution over the scene; see Eleftheriou et al., 2016, and references therein), in this work we considered to be significant anomalies those with pixels with
An additional example of TIR anomaly sequence in Central Italy is reported in Figure 3. Here, the RETIRA index at the time of the Amatrice earthquake (August 24, 2009,
Cross-Checking of Independent Signals
A qualitative cross-check of the different signals considered in this study was finally done in Figure 4 by graphically superimposing geochemical (i.e., CO2) patterns and TIR anomalies.
It emerged that a quite large number of springs/wells (14 water points in total, with 22 variations larger than
Conversely, the Amatrice earthquake (2016) was largely anticipated by TIR anomalies observed up to several months before the event. In this case, the water points from the ARPA network registered an early increase in CO2 concentration. The occurrence of possible precursory signals in Central Italy is not limited to degassing activity. Terakawa et al. (2017) reported that variations in local seismicity possibly induced by CO2 pressure fluctuations at depth were observed before the 2009 L'Aquila mainshock, while crustal deformation processes were evidenced by GNSS before the 2016 mainshock (Panza et al., 2018). Furthermore, a possible precursory seismic pattern was observed in the same period and in the same area by Montuori et al. (2016) and by Gentili et al. (2017). Further possible precursory geophysical anomalies were also observed by Marchetti et al. (2019), Crespi et al. (2020) and Nardò et al. (2020).
The multidisciplinary approach presented in this manuscript has the potential to be applied to investigate seismic precursors outside the region considered here, in areas undergoing significant degassing, and characterized by the presence of surface water sampling points sensitive to crustal deformation processes (e.g., Martinelli and Albarello, 1997; Cioni et al., 2007; Gherardi and Pierotti, 2018). The key point to advance from a condition of mere a posteriori recognition of possible anomalies to the analysis of possible seismic precursors foresees the capability to detect the real temporal variability of the natural system under observation, and requires that relevant geochemical parameters should be strictly monitored continuously, as done elsewhere (e.g., in Tuscany, Italy; Pierotti et al., 2015; Pierotti et al., 2017).
Conclusion
Geochemical effects of crustal deformation processes on geofluids in Central Italy have been evidenced by ground and satellite techniques. Geochemical sampling in thermal springs and in gaseous emissions has allowed us to identify large-scale degassing phenomena in concomitance with the seismic sequence that occurred in Central Italy in 2009. The time series analysis of
Data Availability Statement
The original contributions presented in the study are included in the article/Supplementary Materials, further inquiries can be directed to the corresponding author/s.
Author Contributions
GM, FG, LP, and GF contributed to the collection and interpretation of the geochemical results. NG, ML, and VT contributed to the collection and interpretation of the geophysical results. All authors provided critical feedback and helped shape the research, analysis and manuscript.
Funding
Preliminary scientific communications related to present paper were done during the 14th and the 15th International Conference in Gas Geochemistry. This study benefited from funding provided by the Presidenza del Consiglio dei Ministri – Dipartimento della Protezione Civile (DPC), Project S3-2012. This paper does not necessarily represent official DPC opinion and policies. GM was partially supported by the Chinese Academy of Sciences Visiting Professorship for Senior International Scientists (2018VMA0007).
Acknowledgments
The authors are indebted with Aeronautica Militare Italiana for their support and for granting the access to MS-SEVIRI satellite data, and with the Regional Agencies for Environmental Protection of Umbria, Lazio and Abruzzo Regions for sharing groundwater chemical data. The authors dearly thank two reviewers and Gaetano De Luca (INGV, L'Aquila) for their constructive comments, which have significantly contributed to improving the quality of the manuscript.
Conflict of Interest
The authors declare that the research was conducted in the absence of any commercial or financial relationships that could be construed as a potential conflict of interest.
Supplementary Material
The Supplementary Material for this article can be found online at: https://www.frontiersin.org/articles/10.3389/feart.2020.584716/full#supplementary-material
Footnotes
1Cloudy pixels are identified by means the One channel Cloudy radiance detection Approach (OCA; Cuomo et al., 2004).
2Night-time imagery is less influenced by effects related to soil-air temperature differences (normally higher during other hours of the day) and is less sensitive to local solar exposure.
References
Aliano, C., Corrado, R., Filizzola, C., Genzano, N., Pergola, N., and Tramutoli, V. (2008). Robust TIR satellite techniques for monitoring earthquake active regions: limits, main achievements and perspectives. Ann. Geophys. 51 (1), 303–317. doi:10.4401/ag-3050
Barberio, M. D., Barbieri, M., Billi, A., Doglioni, C., and Petitta, M. (2017). Hydrogeochemical changes before and during the 2016 Amatrice-Norcia seismic sequence (central Italy). Sci. Rep. 7, 11735. doi:10.1038/s41598-017-11990-8
Barnes, I., Irwin, W. P., and White, D. E. (1978). Global distribution of carbon-dioxide discharges, and major zones of seismicity, scale 1:40000,000, in Water Resour. Invest., 78-39, open-file report, 11 pp., U.S. Geol. Surv., Washington, DC.
Bella, F., Biagi, P. F., Caputo, M., Della Monica, G., Ermini, A., Manjgaladze, , et al. (1995). Possible creep-related tilt precursors obtained in the central Apennines (Italy) and in the southern Caucasus (Georgia). Pure Appl. Geophys. 144, 277–300. doi:10.1007/BF00878635
Bonfanti, P., Genzano, N., Heinicke, J., Italiano, F., Martinelli, G., Pergola, N., et al. (2012). Evidence of CO2-gas emission variations in the central Apennines (Italy) during the L’Aquila seismic sequence (March-April 2009). B. Geofis. Teor. Appl. 53, 147–168. doi:10.4430/bgta0043
Boni, C. F., Bono, P., and Capelli, G. (1986). Schema idrogeologico dell’Italia centrale. Memorie Società Geologica Italiana, 35, 991–1012 [in Italian].
Boschetti, T., Barbieri, M., Barberio, M. D., Billi, A., Frondini, S., and Petitta, M. (2019). CO2 inflow and elements desorption prior to a seismic sequence, Amatrice-Norcia 2016, Italy. Geochem. Geophys. 20, 2303–2317. doi:10.1029/2018GC008117
Cheloni, D., De Novellis, V., Albano, M., Antonioli, A., Anzidei, M., Atzori, S., et al. (2017). Geodetic model of the 2016 Central Italy earthquake sequence inferred from InSAR and GPS data. Geophys. Res. Lett. 44 (13), 6778–6787. doi:10.1002/2017GL073580
Chiaraluce, L., Di Stefano, R., Tinti, E., Scognamiglio, L., Michele, M., Casarotti, E., et al. (2017). The 2016 central Italy seismic sequence: a first look at the mainshocks, aftershocks and source models. Seismol. Res. Lett. 88, 757–771. doi:10.1785/0220160221
Chiodini, G., Caliro, S., Cardellini, C., Frondini, F., Inguaggiato, S., and Matteucci, F. (2011). Geochemical evidence for and characterization of CO2 rich gas sources in the epicentral area of the Abruzzo 2009 earthquakes. Earth Planet. Sci. Lett. 304, 389–398. doi:10.1016/j.epsl.2011.02.016
Chiodini, G., Cardellini, C., Amato, A., Boschi, E., and Caliro, S. (2004). Carbon dioxide Earth degassing and seismogenesis in central and southern Italy. Geophys. Res. Lett. 31, L07615. doi:10.1029/2004GL019480
Chiodini, G., Cardellini, C., Di Luccio, F., Selva, G., Frondini, F., Caliro, S., et al. (2020). Correlation between tectonic CO2 Earth degassing and seismicity is revealed by a 10-year record in the Apennines, Italy. Sci. Adv. 6, eabc2938. doi:10.1126/sciadv.abc2938
Chiodini, G., Frondini, F., Cardellini, C., Caliro, S., Beddini, G., and Rosiello, A. (2018). Measuring and interpreting CO2 fluxes at regional scale: the case of Apennines, Italy. J. Geol. Soc. doi:10.1144/jgs2017-169.
Chiodini, G., Valenza, M., Cardellini, C., and Frigeri, A. (2008). A new web-based catalog of earth degassing sites in Italy. EOS 89, 341–342.
Cicerone, R. D., Ebel, J. E., and Britton, J. (2009). A systematic compilation of earthquake precursors. Tectonophysics 476, 371–396. doi:10.1016/j.tecto.2009.06.008
Cioni, R., Guidi, M., Pierotti, L., and Scozzari, A. (2007). An automatic monitoring network installed in Tuscany (Italy) for studying possible geochemical precursory phenomena. Nat. Hazards Earth Syst. Sci. 7, 405–416. doi:10.5194/nhess-7-405-2007
Crespi, M., Kossobokov, V., Panza, G. F., and Peresan, A. (2020). Space-time precursory features within ground velocities and seismicity in North-Central Italy. Pure Appl. Geophys. 177, 369–386. doi:10.1007/s00024-019-02297-y
Cuomo, V., Filizzola, C., Pergola, N., Pietrapertosa, C., and Tramutoli, V. (2004). A self-sufficient approach for GERB cloudy radiance detection. Atmos. Res., 72, 39–56. doi:10.1016/j.atmosres.2004.03.030
De Luca, G., Di Carlo, G., and Tallini, M. (2018). A record of changes in the Gran Sasso groundwater before, during and after the 2016 Amatrice earthquake, central Italy. Sci. Rep. 8, 15982. doi:10.1038/s41598-018-34444-1
DISS Working Group (2018). Database of Individual Seismogenic Sources (DISS), Version 3.2.1: a compilation of potential sources for earthquakes larger than M 5.5 in Italy and surrounding areas. http://diss.rm.ingv.it/diss/ (Istituto Nazionale di Geofisica e Vulcanologia) (Accessed on July 16, 2020). doi:10.6092/INGV.IT-DISS3.2.1
Doglioni, C. (1991). A proposal for the kinematic modelling of W-dipping subductions-possible applications to the Tyrrhenian-Apennines system. Terra. Nova 3, 423–434.
Eleftheriou, A., Filizzola, C., Genzano, N., Lacava, T., Lisi, M., Paciello, R., et al. (2016). Long-term RST analysis of anomalous TIR sequences in relation with earthquakes occurred in Greece in the period 2004–2013. Pure Appl. Geophys. 173, 285–303. doi:10.1111/j.1365-3121.1991.tb00172.x
Festa, G., Picozzi, M., Caruso, A., Colombelli, S., Cattaneo, M., Chiaraluce, L., et al. (2017). Performance of earthquake early warning systems during the 2016–2017 Mw 5–6.5 Central Italy sequence. Seismol. Res. Lett. 89, 1–12. doi:10.1785/0220170150
Filizzola, C., Pergola, N., Pietrapertosa, C., and Tramutoli, V. (2004). Robust satellite techniques for seismically active areas monitoring: a sensitivity analysis on September 7, 1999 Athens’s earthquake. Phys. Chem. Earth 29, 517–527. doi:10.1016/j.pce.2003.11.019
Frezzotti, M. L., Peccerillo, A., and Panza, G. (2010). “Earth’s degassing in Italy. Journal of the virtual explorer,” in The geology of Italy: Tectonics and life along plate margins. Editors C. Beltrando, C. Peccerillo, C. Mattei, C. Conticelli and Doglioni, Vol. 36, paper 21. http://virtualexplorer.com.au/article/2010/227/earth-co-degassing.
Gentili, S., Di Giovambattista, R., and Peresan, A. (2017). Seismic quiescence preceding the 2016 central Italy earthquakes. Phys. Earth Planet. In. 272, 27–33. doi:10.1016/j.pepi.2017.09.004
Genzano, N., Aliano, C., Corrado, R., Filizzola, C., Lisi, M., Mazzeo, G., et al. (2009). RST analysis of MSG-SEVIRI TIR radiances at the time of the Abruzzo 6 April 2009 earthquake. Nat. Hazard. Earth Sys. 9 (6), 2073–2084. doi:10.5194/nhess-9-2073-2009
Genzano, N., Filizzola, C., Paciello, R., Pergola, N., and Tramutoli, V. (2015). Robust Satellite Techniques (RST) for monitoring earthquake prone areas by satellite TIR observations: the case of 1999 Chi-Chi earthquake (Taiwan). J. Asian Earth Sci. 114, 289–298. doi:10.1016/j.jseaes.2015.02.010
Gherardi, F., and Pierotti, L. (2018). The suitability of the Pieve Fosciana hydrothermal system (Italy) as a detection site for geochemical seismic precursors. Appl. Geochem. 92, 166–179. doi:10.1016/j.apgeochem.2018.03.009
Heinicke, J., Martinelli, G., and Telesca, L. (2011). Geodynamically induced variations in the emissions of CO2 gas at San Faustino (Central Apennines, Italy). Geofluids 12, 123–132. doi:10.1111/j.1468-2011.00345.x
Irwin, W. P., and Barnes, I. (1980). Tectonic relations of carbon dioxide discharges and earthquake. J. Geophys. Res. 85 (B6), 3115–3121. doi:10.1029/jb085ib06p03115
Italiano, F., Martinelli, G., and Rizzo, A. (2004). Geochemical evidence of seismogenic-induced anomalies in the dissolved gases of thermal waters: a case study of Umbria (Central Apennines, Italy) both during and after the 1997-1998 seismic swarm. Geochem. Geophys. 5, Q11001. doi:10.1029/2004GC000720
King, C.-Y., Evans, W. C., Presser, T., and Husk, R. (1981). Anomalous chemical changes in well water and possible relation to earthquakes. Geophys. Res. Lett. 8, 425–428. doi:10.1029/GL008i005p00425
King, C.-Y., Zhang, W., and Zhang, Z. (2006). Earthquake-induced groundwater and gas changes. Pageoph special issue hiroshi Wakita vol. I “Terrestrial fluids, earthquakes and volcanoes” 163, 633–645. doi:10.1007/s00024-006-0049-7
Kumpel, H.-J. (1992). About the potential of wells to reflect stress variations within inhomogeneous crust. Tectonophysics 211, 317–336. doi:10.1016/0040-1951(92)90068-H
MAGA Project 2013-2018 (2018). Mapping gas emissions. http://www.magadb.net/ (Accessed July 16 2020).
Marchetti, D., De Santis, A., D’Arcangelo, S., Poggio, F., Piscini, A., Campuzano, S. A., et al. (2019). Pre-earthquake chain processes detected from ground to satellite altitude in preparation of the 2016–2017 seismic sequence in Central Italy. Remote Sens. Environ. 229, 93–99. doi:10.1016/j.rse.2019.04.033
Martinelli, G., and Albarello, D. (1997). Main constraints for siting monitoring networks devoted to the study of earthquake related phenomena in Italy. Ann. Geophys. 40, 1505–1522. doi:10.4401/ag-3827
Martinelli, G., and Dadomo, A. (2018). “Geochemical and fluid-related precursors of earthquakes: previous and ongoing research trends” in Pre-earthquake processes, a multidisciplinary approach to earthquake studies. Editors S. Ouzounov, K. Pulinets, P. Hattori Taylor (Washington D.C: AGU-Wiley), .219–228.
Martini, C. (2016). Signals in water- the deep originated CO2 in the Peschiera-Capone aqueduct in relation to monitoring of seismic activity in central Italy. It. J. Groundwater, 5, 7–20. doi:10.7343/as-2016-246
Minissale, A. (1991). Thermal springs in Italy and their relation to recent tectonics. Appl. Geochem. 6, 201–212. doi:10.1016/j.earscirev.2003.09.001
Minissale, A. (2004). Origin, transport and discharge of CO2 in central Italy. Earth Sci. Rev. 66, 89–141.
Montone, P., and Mariucci, M. T. (2016). The new release of the Italian contemporary stress map. Geophys. J. Int. 205, 1525–1531. doi:10.1093/gji/ggw100
Montuori, C., Murru, M., and Falcone, G. (2016). Spatial variation of the b-value observed for the periods preceding and following the 24 August 2016, Amatrice earthquake (ML 6.0) (Central Italy). Ann. Geophys. 59, doi:10.4401/ag-7273.
Moro, M., Saroli, M., Stramondo, S., Bignami, C., Albano, M., Falcucci, E., et al. (2017). New insights into earthquake precursors from InSAR. Sci. Rep. 7, 12035. doi:10.1038/s41598-017-12058-3.
Nardò, S., Ascione, A., Mazzoli, S., Terranova, C., and Vilardo, G. (2020). InSAR data analysis: pre-seismic ground deformation in the 2009 L’Aquila earthquake region. Boll. Geofis. Teor. Appl., 61, 41–56. doi:10.4430/bgta0251
Pace, B., Peruzza, L., Lavecchia, G., and Boncio, P. (2006). Layered seismogenic source model and probabilistic seismic hazard analyses in central Italy. Bull. Seism. Soc. Am. 96, 107–132.
Panza, G. F., Peresan, A., Sansò, F., Crespi, M., Mazzoni, M., and Nascetti, A. (2018). How geodesy can contribute to the understanding and prediction of earthquakes. Rend. Lincei Sci. Fis. Nat. 29, 81–93. doi:10.1007/s12210-017-0626-y
Parkhurst, D. L., and Appelo, C. A. J. (2013). “Description of input and examples for PHREEQC version 3–a computer program for speciation, batch-reaction, one-dimensional transport, and inverse geochemical calculations.” (Denver, CO: U.S. Geological Survey techniques and methods), Book 6, Chapter A43.
Patacca, E., Scandone, P., Di Luzio, E., Cavinato, G. P., and Parotto, M. (2008). Structural architecture of the central Apennines: interpretation of the CROP 11 seismic profile from the Adriatic coast to the orographic divide: CROP 11 seismic profile. Tectonics 27, doi:10.1029/2005TC001917
Patacca, E., and Scandone, P. (2001). “Late thrust propagation and sedimentary response in the thrust-belt—foredeep system of the Southern Apennines (Pliocene-Pleistocene),” in Anatomy of an orogen: the Apennines and adjacent Mediterranean basins. Editors G. B. Vai and I. P. Martini, 401–440.
Petitta, M., Mastrorillo, L., Preziosi, E., Banzato, F., Barberio, M. D., Billi, A., et al. (2018). Water-table and discharge changes associated with the 2016-2017 seismic sequence in central Italy: hydrogeological data and a conceptual model for fractured carbonate aquifers. Hydrogeol. J. 26, 1009–1026. doi:https://doi.org/10.1007/s10040-017-1717-7
Pierotti, L., Botti, F., D’Intinosante, V., Facca, G., and Gherardi, F. (2015). Anomalous CO2 content in the Gallicano thermo-mineral spring (Serchio valley, Italy) before the 21 June 2013, Alpi Apuane earthquake (M = 5.2). Phys. Chem. Earth 85–86, 131–140. doi:10.1016/j.pce.2015.02.007
Pierotti, L., Gherardi, F., Facca, G., Piccardi, L., and Moratti, G. (2017). Detecting CO2 anomalies in a spring on Mt.Amiata volcano (Italy). Phys. Chem. Earth 98, 161–172. doi:10.1016/j.pce.2017.01.008
Roeloffs, E. A., Burford, S. S., Riley, F. S., and Records, A. W. (1989). Hydrologic effects on water level changes associated with episodic fault creep near Parkfield, California. J. Geophys. Res., 94, 12387–12402. doi:10.1029/JB094iB09p12387
Roeloffs, E. A. (1988). Hydrologic precursors to earthquakes: a review. Pure Appl. Geophys. 126, 177–209. doi:10.1007/BF00878996
Rojstaczer, S., Wolf, S., and Michel, R. (1995). Permeability enhancement in the shallow crust as a cause of earthquake-induced hydrological changes. Nature 373, 237–239. doi:10.1038/373237a0
Sato, M., Sutton, A. J., McGee, K. A., and Russel-Robinson, S. (1986). Monitoring of hydrogen along the san andreas and calaveras faults in central California in 1980–1984. J. Geophys. Res. 91, 12315–12326. doi:10.1029/JB091iB12p12315
Serpelloni, E., Anderlini, L., and Belardinelli, M. E. (2012). Fault geometry, coseismic-slip distribution and Coulomb stress change associated with the 2009 April 6, Mw 6.3, L’Aquila earthquake from inversion of GPS displacements. Geophys. J. Int. 188, 473–489. doi:10.1111/j.1365-246X.2011.05279.x
Sgrigna, V., and Malvezzi, V. (2003). Preseismic creep strains revealed by ground tilt measurements in central Italy on the occasion of the 1997 Umbria-Marche Apennines earthquake sequence. Pure Appl. Geophys. 160 (8), 1493–1515. doi:10.1007/s00024-003-2357-5
Tamburello, G., Pondrelli, S., Chiodini, G., and Rouwet, D. (2018). Global-scale control of extensional tectonics on CO2 Earth degassing. Nat. Commun. 9, 4608. doi:10.1038/s41467-018-07087-z
Terakawa, T., Zoporowski, A., Galvan, B., and Miller, S. M. (2017). High-pressure fluid at hypocentral depths in the L’Aquila region inferred from earthquake focal mechanisms. Geology 38, 995–998. doi:10.1130/G31457.1
Toutain, J. P., and Baubron, J. C. (1999). Gas geochemistry and seismotectonics: a review. Tectonophysics 304 (1–2), 1–27. doi:10.1016/S0040-1951(98)00295-9
Tramutoli, V., Aliano, C., Corrado, R., Filizzola, C., Genzano, N., Lisi, M., et al. (2013). On the possible origin of thermal infrared radiation (TIR) anomalies in earthquake-prone areas observed using robust satellite techniques (RST). Chem. Geol. 339, 157–168. doi:10.1016/j.chemgeo.2012.10.042
Tramutoli, V., Corrado, R., Filizzola, C., Genzano, N., Lisi, M., and Pergola, N. (2015). From visual comparison to Robust satellite techniques: 30 years of thermal infrared satellite data analyses for the study of earthquake preparation phases. Boll. Geof. Teor. Appl. 56, 167–202. doi:10.4430/bgta0149
Tramutoli, V., Cuomo, V., Filizzola, C., Pergola, N., and Pietrapertosa, C. (2005). Assessing the potential of thermal infrared satellite surveys for monitoring seismically active areas. The case of Kocaeli (Izmit) earthquake, August 17th, 1999. Remote Sens. Environ. 96, 409–426. doi:10.1016/j.rse.2005.04.006
Tramutoli, V. (1998). “Robust AVHRR techniques (RAT) for environmental monitoring: theory and applications,” in Earth surface remote sensing II: SPIE. Editors E. Cecchi and Zilioli, Vol. 3496, 101–113.
Tramutoli, V. (2005). “Robust satellite techniques (RST) for natural and environmental hazards monitoring and mitigation: ten years of successful applications,” in Proceeding of the 9th international symposium on physical measurements and signatures. In remote sensing XXXVI, 792–795.
Tramutoli, V. (2007). “Robust satellite techniques (RST) for natural and environmental hazards monitoring and mitigation: theory and applications,” in 2007 international workshop on the analysis of multi-temporal remote sensing images (IEEE), 1–6.
Vai, G. B., and Martini, I. P. (2001). Anatomy of an orogen: the Apennines and adjacent Mediterranean basins. Dordrecht: Springer-Science+Business Media, 633.
Valoroso, L., Chiaraluce, L., Piccinini, D., Di Stefano, R., Schaff, D., and Waldhauser, F. (2013). Radiography of a normal fault system by 64,000 high-precision earthquake locations: the 2009 L’Aquila (central Italy) case study. J. Geophys. Res. 118, 1–21. doi:10.1002/jgrb.50130
Keywords: groundwater chemistry, CO2 degassing, CO2 anomaly, satellite observation, thermal infrared anomaly, crustal deformation, earthquake forecasting, central Italy
Citation: Martinelli G, Facca G, Genzano N, Gherardi F, Lisi M, Pierotti L and Tramutoli V (2020) Earthquake-Related Signals in Central Italy Detected by Hydrogeochemical and Satellite Techniques. Front. Earth Sci. 8:584716. doi: 10.3389/feart.2020.584716
Received: 21 July 2020; Accepted: 16 October 2020;
Published: 12 November 2020.
Edited by:
Frédéric Frappart, UMR5566 Laboratoire d'études en géophysique et océanographie spatiales (LEGOS), FranceCopyright © 2020 Martinelli, Facca, Genzano, Gherardi, Lisi, Pierotti and Tramutoli. This is an open-access article distributed under the terms of the Creative Commons Attribution License (CC BY). The use, distribution or reproduction in other forums is permitted, provided the original author(s) and the copyright owner(s) are credited and that the original publication in this journal is cited, in accordance with accepted academic practice. No use, distribution or reproduction is permitted which does not comply with these terms.
*Correspondence: Fabrizio Gherardi, Zi5naGVyYXJkaUBpZ2cuY25yLml0