- 1Museum of Nature South Tyrol, Bozen/Bolzano, Italy
- 2Department of Earth Sciences, University of Geneva, Geneva, Switzerland
- 3Department für Geo- und Umweltwissenschaften, Paläontologie und Geobiologie, Ludwig-Maximilians-Universität, München, Germany
- 4Bayerische Staatssammlung für Paläontologie und Geobiologie, München, Germany
It has long been recognized that terrestrial floras underwent major and long-lasting changes during the Permian and Triassic, some of which have been attributed to the end-Permian mass extinction. However, these changes are still poorly understood with regard to the late Permian and Early Triassic. In particular, the impact that ecological disturbances around the Permian–Triassic boundary had on the composition and palaeogeographical distribution of land plant communities needs to be scrutinized. We analyse this impact based on fossil floras from across the world, covering the Wuchiapingian to Ladinian time interval. The plant assemblages are assigned to biomes representing particular environmentally controlled community types. Variations in the distribution of biomes between stages indicate shifts in the environmental parameters affecting terrestrial floras, and provide insights into population turnover dynamics. A substantial shift towards increasing seasonality and a reduction of biome diversity occurs in the earliest Triassic and stabilised throughout the Middle Triassic. However, results also show that the stratigraphically and (palaeo-) geographically unequal distribution of sampled localities constitutes an important limitation for this kind of analysis.
Introduction
The Permian–Triassic transition was marked by drastic disturbances in marine and terrestrial ecosystems. Most importantly, the end-Permian mass extinction constituted the most severe extinction event for marine animals (e.g., Benton and Twitchett, 2003; Stanley, 2016; Delfino et al., 2020). The timing and impact of this event on the biodiversity of terrestrial animals and land plants is not yet settled (e.g., Benton and Newell, 2014; Cascales-Miñana and Cleal, 2014, Cascales-Miñana et al., 2016; Fielding et al., 2019; Nowak et al., 2019; Gastaldo et al., 2020; Mays et al., 2020). However, it is generally accepted that the community structure of terrestrial floras underwent important changes, and there is a growing consensus that these changes should primarily be understood as a matter of successive ecological disturbances, rather than a question of abrupt biodiversity loss (Hochuli et al., 2016; Mishra et al., 2017; Fielding et al., 2019; Nowak et al., 2019; Vajda et al., 2020).
Palaeophytogeographical aspects of these changes on a global scale have mainly been studied through the recognition of different phytochoria (or phytoprovinces) – phytogeographical units that are defined on the presence of common taxa, and are consequently stratigraphically constrained by the ranges of these taxa (e.g., Vakhrameev et al., 1978). While the geographical spread of the defining plants was certainly dependent on environmental conditions, phytochoria from different times do not necessarily reflect equivalent environments. By contrast, biomes are defined as areas with comparable, climatically controlled plant and animal assemblages (Walter, 1985). The biome concept has successfully been applied to plant fossil together with climate-sensitive sediment data from ancient times as well, including the Sakmarian (Cisuralian/early Permian; Rees et al., 2002), Wordian/Kungurian (Guadalupian/middle Permian; Ziegler, 1990; Rees et al., 1999a; Rees et al., 1999b; Rees et al., 2002; Willis and McElwain, 2014) and the Jurassic (Rees and Ziegler, 1996; Rees et al., 2000; Willis and McElwain, 2014). Biomes for the Triassic and Jurassic in Eurasia were presented by Ziegler et al. (1993). Global biome maps for the Early to Middle Triassic are not yet available. Climate models have been used to estimate the distribution of biomes in the Sakmarian (Rees et al., 2002), the Wordian/Kungurian (Kutzbach and Ziegler, 1993; Rees et al., 1999a; Rees et al., 1999b; Rees et al., 2002), the Changhsingian (Lopingian/late Permian; Fluteau et al., 2001; Roscher et al., 2011) and the Late Triassic (Sellwood and Valdes, 2006). Spore-pollen data have been used for palaeophytogeography as well, including considerations of climate conditions as indicated by palynological assemblages in the Early Triassic (Yaroshenko, 1997) and the late Middle–early Late Triassic (Visscher and Van der Zwan, 1981). Chumakov and Zharkov (2002) and Chumakov and Zharkov (2003) used palyno- and macrofloras together with lithological, palaeogeography and palaeoclimate data to reconstruct climatic belts for the early and late Permian and Early Triassic. The palaeophytogeographical distribution and climatic implications of macrofloras from southern Gondwana were also explored by Cúneo (1996) for the Permian and by Artabe et al. (2003) and Spalletti et al. (2003) for the Triassic.
We present here a stage-level analysis of the distribution of biomes based on land plant macrofossil assemblages from the Wuchiapingian to Ladinian, in an attempt to track global-scale climate shifts with an effect on plant communities. This study also shows how the composition of biomes changes through time and how the patchiness of the plant fossil record affects the uncertainty regarding the boundaries between biomes.
Materials and Methods
Data Sources and Selection
This study is based on a subset of the data of Nowak et al. (2019), most of which were taken from earlier publications (Supplementary Data; see also supplementary data 5 and supplementary references of Nowak et al., 2019). Our subset represents 8,892 land plant macrofossil occurrences from the Wuchiapingian to Ladinian stages dated with reasonable confidence and with sufficiently precise information on the geographical position of the studied outcrops (Supplementary Table S1). Compared to the initial dataset, we made numerous corrections with regards to taxonomic synonyms and locality data. Occurrences with uncertain identifications at genus-level were not taken into considerations. In addition, latitude/longitude coordinates were assigned to the localities.
The palaeogeographic maps shown herein correspond to the conversion of global plate tectonic reconstructions into 3D topography surfaces (palaeotopography) or paleo-DEMs (digital elevation models). The conversion stems from the work of Vérard et al. (2015a) and Vérard et al. (2015b). The plate tectonic model corresponds to the first version of the Panalesis model (still under development), which is described in Vérard (2019a) and Vérard (2019b). While superseding the UNIL model developed at the University of Lausanne (e.g., Stampfli and Borel, 2002; Hochard, 2008), the Panalesis model is created from scratch but is based on the same techniques and know-how. The definition of synthetic isochrones, in particular, helps reconstructing not only continental areas but also oceanic realms, including those having disappeared with subduction processes, such as the Panthalassic Ocean. The global reconstructions are the results of iterative processes where tectonic plates (with closed plate boundaries and 100% of the earth surface covered) are moved according to geological data of broad type, plate geometry, plate kinematics, and geodynamical scenarios defined in three dimensions and time (see for instance Fig 2.5.11-13 in Hochard, 2008).
Climate-indicative sediment data from the late Permian to Middle Triassic were extracted from Boucot et al. (2013); see Supplementary Figures S1–S3.
Calculations and Interpretations
Present-day coordinates of plant fossil localities and sediment data were translated into late Permian to Middle Triassic palaeocoordinates according to the Panalesis model (Vérard, 2019a; Vérard, 2019b). Regional taxonomic diversity is calculated per stage for biomes (Table 1), 10° palaeolatitudinal slices (Table 2) and major palaeogeographic regions (Table 3; Figure 1) as sampled-in-bin diversity at the genus level, i.e., the number of genera that have been reported from each stage (not counting uncertain identifications). Endemism (Table 4) is calculated as the ratio of plant genera in only one major palaeogeographic region within a specific stage. Cases where the same genus occurs in a different region in another stage are ignored.
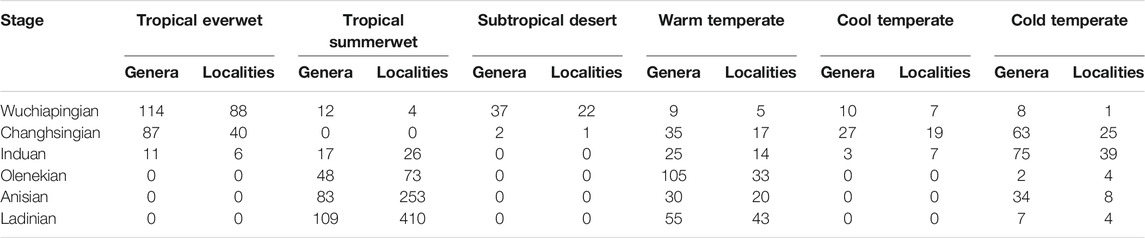
TABLE 1. Number of plant fossil localities and taxonomic diversity at genus level per stage and biome.
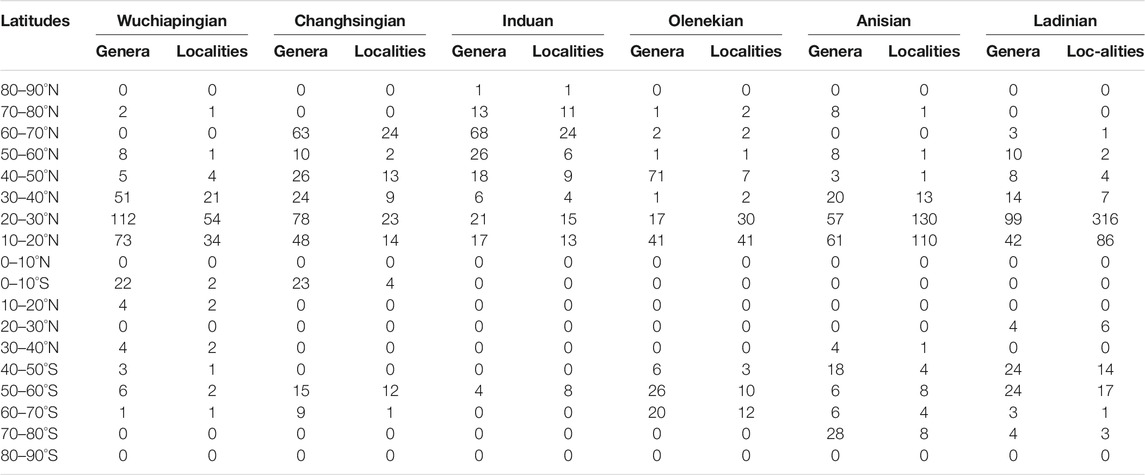
TABLE 2. Number of plant fossil localities and taxonomic diversity at genus level per stage and 10° latitudinal slice.
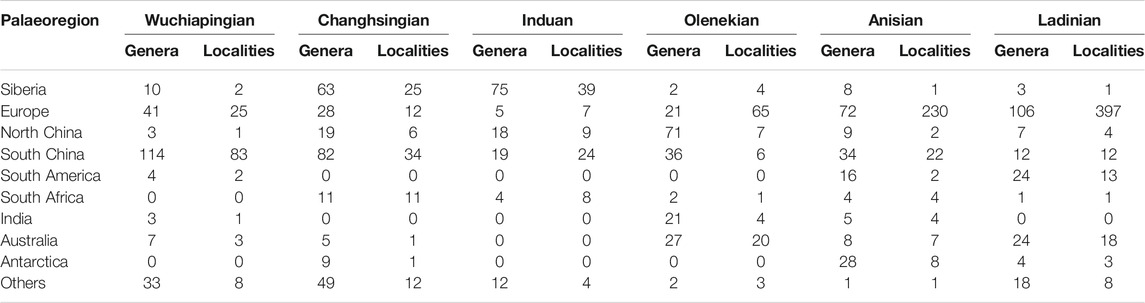
TABLE 3. Number of plant fossil localities and taxonomic diversity at genus level per stage and palaeogeographic region.
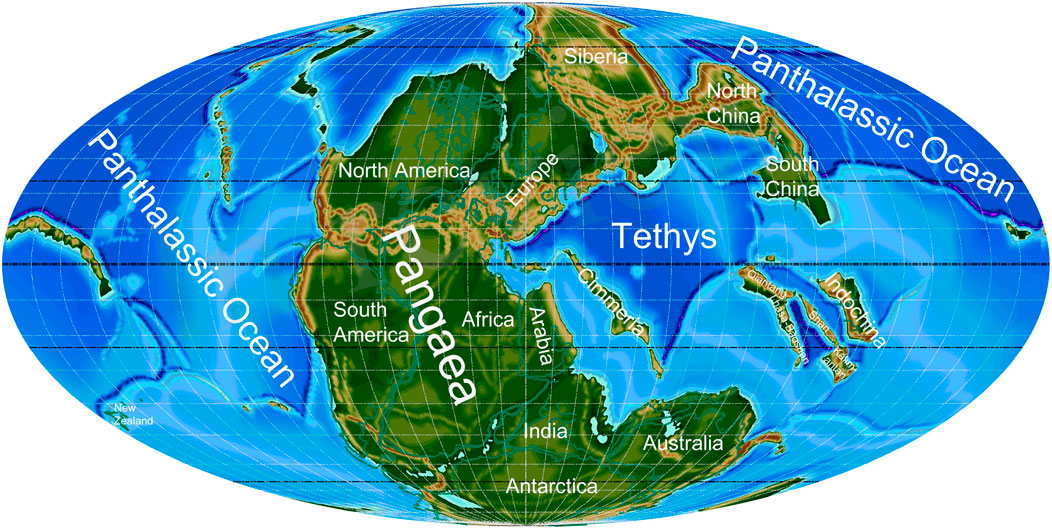
FIGURE 1. Palaeogeographic map for the late Permian–Early Triassic (250 Ma) indicating major regions.
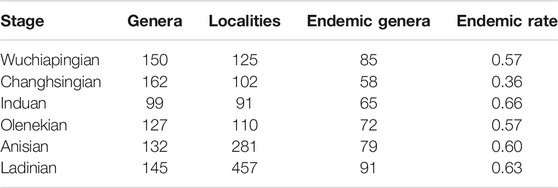
TABLE 4. Number of plant fossil localities and taxonomic diversity at genus level per stage, including number and rate of endemic genera.
Based on known ecological constraints and the distribution of plant taxa during the time interval in question, we determined in which biomes individual plant genera would be expected to occur commonly or exclusively (Supplementary Table S2). The biomes used herein correspond to the ten biomes of Ziegler (1990), although not all were applicable (see below). For each locality-stage combination in the dataset, we calculated the most likely biome by first pooling occurrences from coeval localities within a moving window with a 100 km radius (calculated from the palaeogeographical coordinates under the assumption of a spherical earth with a mean radius of 6371.009 km) in order to take into account short-range variation, incompletely reported assemblages and effectively equivalent localities that were reported with different levels of geographical precision. Based on the pooled occurrences and the aforementioned biome assignments, a weighted vote was created: Each genus occurrence in the pool is counted with a weight equal to the reciprocal of the number of biomes it is associated with. A biome assignment is considered likely if it is indicated by >50% of the weighted vote for a locality and stage. If at least one genus is present that is considered to be an exclusive element of a particular biome, then the respective collection is assigned to that biome with certainty as well. In the case of collections where the calculation does not indicate any biome with sufficient confidence, a biome is assigned manually based on the taxonomic composition, geographic position and similarities with neighbouring assemblages. Microenvironments originating locally due to the effect of the nearby coastal line, deltaic settings and/or sea-level changes (e.g., Kustatscher et al., 2017) are not taken into account. The assigned biomes were projected on palaeogeographic maps for each stage, and we added a tentative extrapolation of the biome areas, taking into account the palaeotopography and climate-indicative sediment data (Figures 2–7).
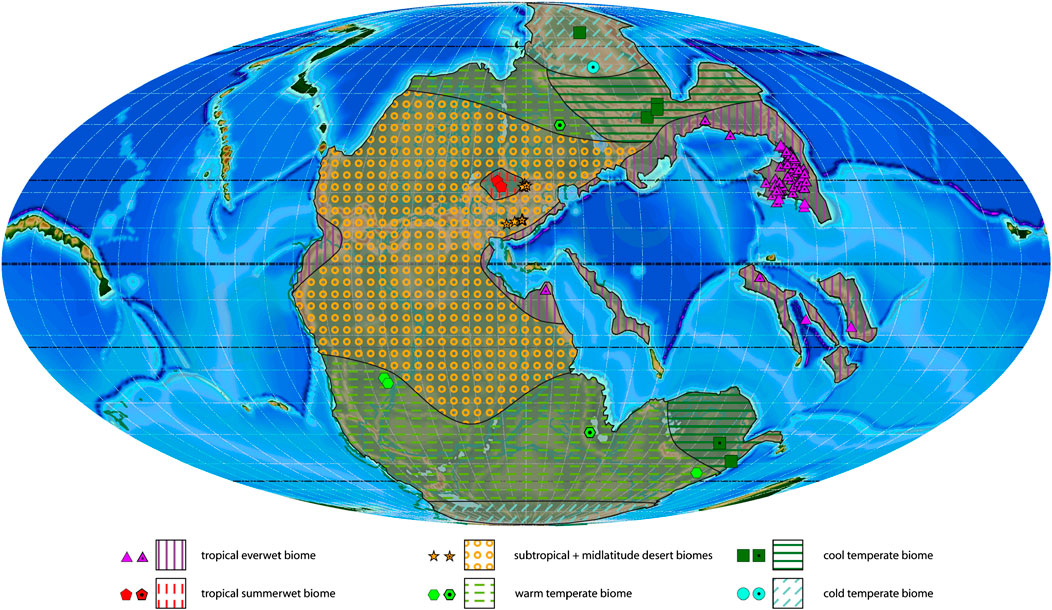
FIGURE 2. Wuchiapingian plant macrofossil localities with associated biomes and estimated biome extension areas projected on a palaeogeographic reconstruction for the late Permian–Early Triassic (250 Ma). Dotted symbols indicate calculated biomes, empty symbols indicate manual biomes.
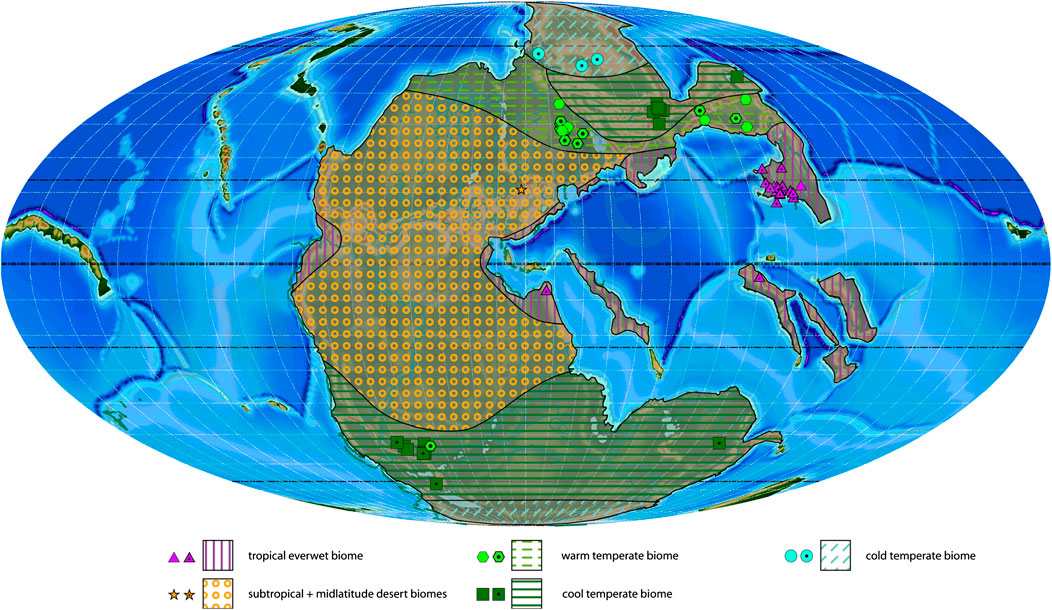
FIGURE 3. Changhsingian plant macrofossil localities with associated biomes and estimated biome extension areas projected on a palaeogeographic reconstruction for the late Permian–Early Triassic (250 Ma). Dotted symbols indicate calculated biomes, empty symbols indicate manual biomes.
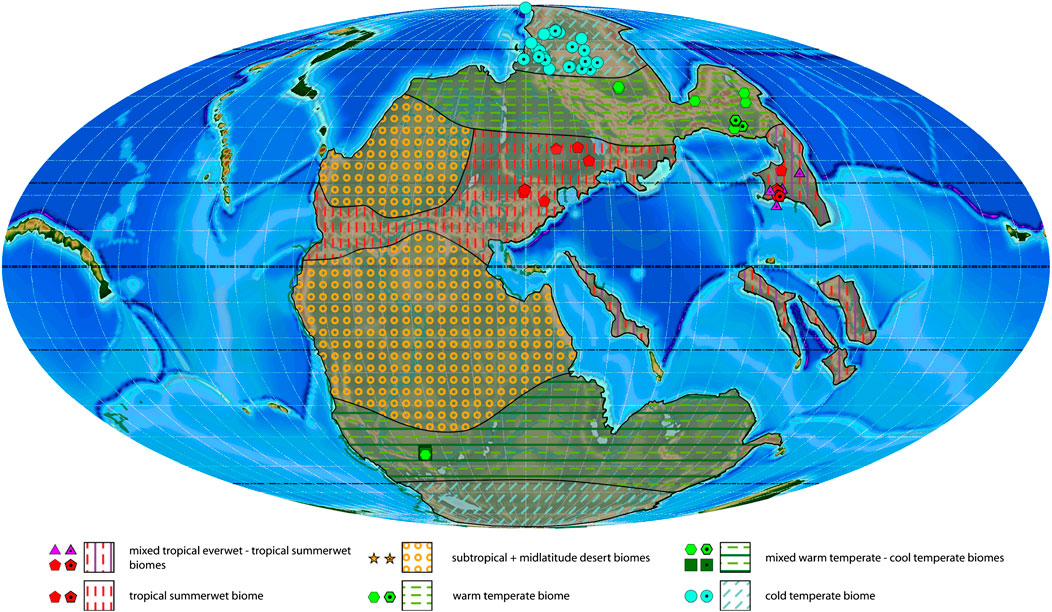
FIGURE 4. Induan plant macrofossil localities with associated biomes and estimated biome extension areas projected on a palaeogeographic reconstruction for the late Permian–Early Triassic (250 Ma). Dotted symbols indicate calculated biomes, empty symbols indicate manual biomes.
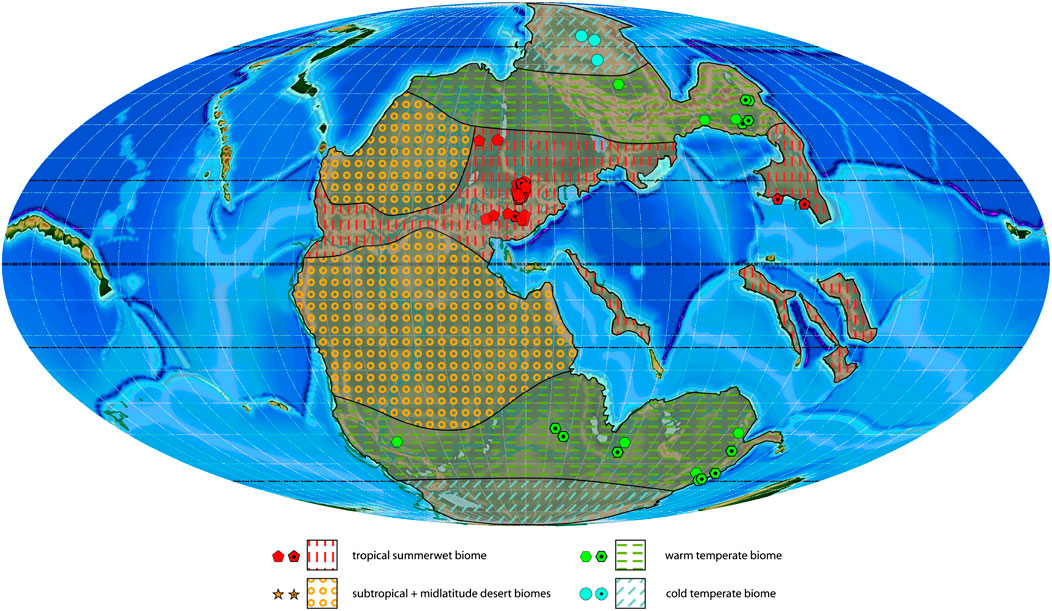
FIGURE 5. Olenekian plant macrofossil localities with associated biomes and estimated biome extension areas projected on a palaeogeographic reconstruction for the late Permian–Early Triassic (250 Ma). Dotted symbols indicate calculated biomes, empty symbols indicate manual biomes.
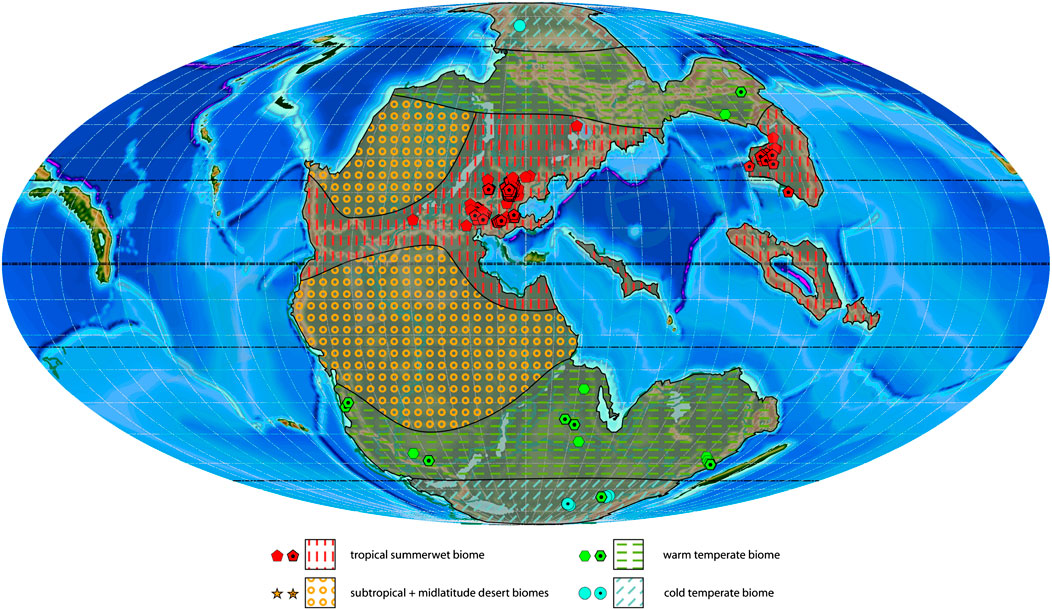
FIGURE 6. Anisian plant macrofossil localities with associated biomes and estimated biome extension areas projected on a palaeogeographic reconstruction for the Middle Triassic (240 Ma). Dotted symbols indicate calculated biomes, empty symbols indicate manual biomes.
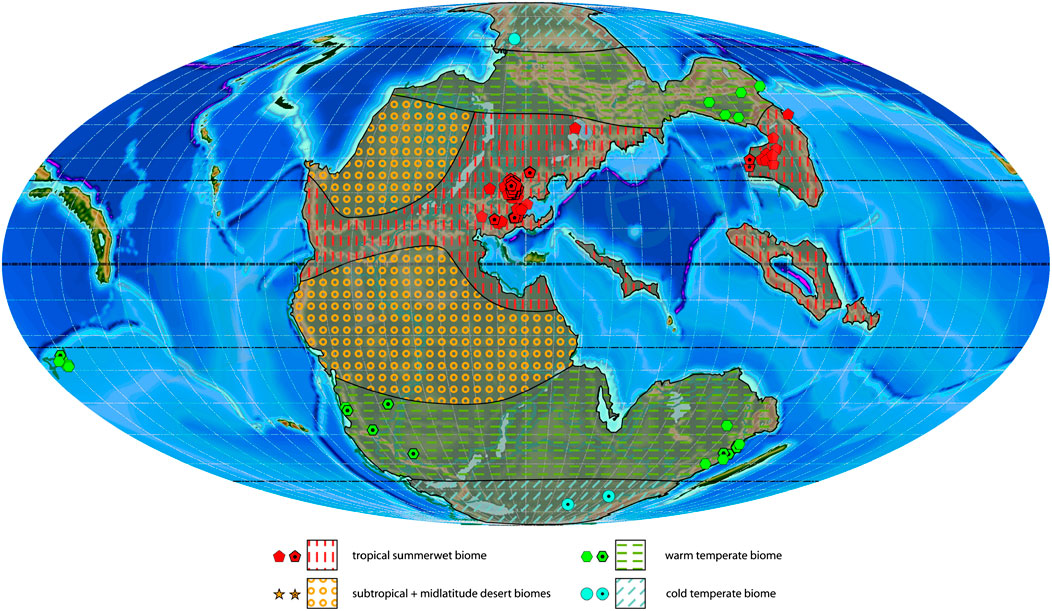
FIGURE 7. Ladinian plant macrofossil localities with associated biomes and estimated biome extension areas projected on a palaeogeographic reconstruction for the Middle Triassic (240 Ma). Dotted symbols indicate calculated biomes, empty symbols indicate manual biomes.
Biomes
The following ten biomes were defined by Ziegler (1990) and provide the basis for our analysis:
The tropical everwet biome (biome 1: equatorial and tropical everwet biome of Ziegler, 1990) represents evergreen rainforests in the tropics that develop under constantly hot (mean T = 26–29°C) and humid conditions (10–20 mm/month) and tends to be the most taxonomically diverse biome. Typical for this biome are abundant multistoried forests with high trees and lianas. The forests increase the constant conditions by trapping air, retaining moisture and creating moisture due to continuous transpiration. The biome also includes peat soils/coal swamps and their characteristic plant communities. It is most common close to the equator but can also occur in middle latitudes. The plant communities growing under these environmental conditions have a high preservation potential.
The tropical summerwet biome (biome 2: tropical and subtropical summerwet biome of Ziegler, 1990) comprises a variety of plant communities ranging from deciduous forests to savannas with varying degrees of wood- and grassland, all of which become productive during the wet summer months in low to middle latitudes with strong seasonality. They are transitional between the tropical everwet and the subtropical desert biomes. Although these floras are productive and differentiated, their preservation potential is limited due to the potential oxidation of the soils during dry seasons.
The subtropical desert biome (biome 3: coastal and inland tropical desert biome of Ziegler, 1990) is characterized by a water deficit during every month of the year. The vegetation is generally scarce and the plants that do occur are adapted for survival rather than competition. The vegetation is dominated by xerophytes and succulents that can persist in a continuously dry environment. Large flat root systems are specialised to gather moisture; leaf sizes are reduced to avoid desiccation. The preservation potential of these plants is exceptionally low. Sedimentary indicators are evaporites and aeolian sands.
The winterwet biome (biome 4 of Ziegler, 1990) relates to sclerophyllous evergreen and typically low trees with leathery leaves with high diversity. The winterwet aspect of this biome is unique and related today to the development of the polar front and associated extratropical cyclones. The productivity in biomass and the preservation potential of this vegetation is low. This biome is subsumed under the warm temperate biome for this study, because we found it impossible to differentiate the two in terms of taxonomic composition.
The warm temperate biome (biome 5: western and eastern warm temperate biome of Ziegler, 1990) refers to temperate evergreen forests that are affected by seasonally changing climates. The rainfall is well-distributed today throughout the year due to the alternating winter frontal systems and summer convective systems. The daily temperature minimum of the coldest months is above freezing (in contrast to the cool temperate biome), but the vegetation spends the winter in a resting state. The productivity is half of the tropical everwet biome, but the diversity is generally very high. Peat swamps can develop locally.
The cool temperate biome (biome 6: western and eastern cool temperate biome of Ziegler, 1990) marks deciduous forests that are subjected to hard frosts and cold winters. Deciduous trees require a growing season of at least four months with an average daily temperature above 10°C in order to have enough time to generate leaves. This biome may experience an annual change in the community due to the dense canopy in the summer and leafless trees during the other seasons.
The midlatitude desert biome (biome 7 of Ziegler, 1990) comprises deserts and steppes with cold winters. Today this biome is dominated by tall grass or short grass prairies due to the desertic environments and annual water deficiency. The productivity of biomass is moderate to low, the preservation potential is very low. Floras indicative of such steppes could not be distinguished in our dataset, but it can be assumed that some of the wide areas without plant fossil assemblages within the temperate belt corresponded to this biome. In the figures presented herein, this biome was merged with the subtropical desert biome, since boundaries between these two would be purely speculative.
The cold temperate biome (biome 8 of Ziegler, 1990) is characterized nowadays by boreal conifer forests and taigas (widely spaced trees with a ground cover of mosses and lichens). Today it is missing on the Southern Hemisphere but well-developed on the Northern Hemisphere. Precipitation is low to moderate, but water is not a limiting factor due to the low evaporation rates and short growing seasons. A defining feature is the short growing season, which is controlled by the temperature and sunshine rather than by precipitation.
The arctic biome (biome 9 of Ziegler, 1990) represents a tree-less tundra with a diminutive flora of herbs and small shrubs because of the abridged growing season (can also be only one month in modern equivalents). Modern tundras are composed of grasses, mosses, lichens, and some dwarf shrubs. This biome could not be identified in our dataset due to a lack of characteristic taxa.
The glacial biome (biome 10 of Ziegler, 1990) is essentially abiotic and can be indicated by sediments such as tillites and dropstones. As it is devoid of plants, it is ignored in our analysis, but it should be noted that glacial sediments (dropstones and glendonites) have been reported from the late Permian in Antarctica and Australia (Boucot et al., 2013, and references therein). These occur in the vicinity of or at even lower latitudes than late Permian plant assemblages and consequently do not serve to indicate a large-scale glaciation.
Results
Wuchiapingian
The Wuchiapingian is represented in this study by 125 studied localities yielding a total of 150 plant genera (Table 4). It is the only stage in the studied interval for which six different biomes are distinguished by the available palaeofloral data (Figure 2). It is marked by a wide spread of the tropical everwet biome, which is present in North and South China, Turkey, and on several Tethyan terranes (Indochina, Shan, Qiangtian). Based on this distribution and considering similarities with previously described biome models for the middle Permian (Ziegler, 1990; Rees et al., 1999a; Rees et al., 1999b, Rees et al., 2002), as well as climate models for the latest Permian (Roscher et al., 2011), it seems likely that the tropical everwet biome also covered other areas along the equatorial and northern Tethys coast, as well as the equatorial eastern coast of Pangaea. This is the most diverse biome in the Wuchiapingian with 114 genera (17 orders) in the Northern Hemisphere and 24 genera (11 orders) in the Southern Hemisphere (Table 1; Supplementary Table S3). Common elements of the tropical everwet biome are the Lepidodendrales (6 genera) among the lycophytes, Equisetales (11 genera) and Sphenophyllales (4 genera) among the sphenophytes, Marattiales (11 genera) and Osmundales (6 genera) among the ferns, Medullosales (1 genus) and Glossopteridales (1 genus) among the seed ferns and Gigantopteridales (10 genera), Cordaitales (4 genera) and Noeggerathiales (4 genera) representing gymnosperms of uncertain affinity (Supplementary Table S3). Several taxa are typical elements of the Carboniferous swamps that survived in the smaller swampy basins of the late Permian, especially on the North China and South China blocks (e.g., Sun et al., 1995; Broutin et al., 2020). These acted as a refugium for Carboniferous-type peat-forming plant assemblages (such as lycophytes, cordaites and tree ferns) and as a centre for the appearance of new peat-forming swamp communities (e.g., gigantopterids) and gymnosperm taxa (e.g., conifers; Broutin et al., 2020).
The tropical summerwet biome has a very limited appearance in the Zechstein Basin in Europe, where it is however diverse with a wide range of spore- and pollen-producing taxa (12 genera; Figure 2; Table 1). It is surrounded by the subtropical desert biome, which – in terms of documented plant communities – is likewise limited to Europe. The dominant plant group are the conifers (especially Voltziales; 14 genera), followed by various gymnosperms including Cordaitales (5 genera) and seed ferns (Peltaspermales; 2 genera), as well as ferns (3 genera). With 37 genera, the subtropical desert biome is the second-most diverse in the Wuchiapingian (Table 1). Sediment data indicating aridity (Boucot et al., 2013) and a general lack of plant fossil records suggest that deserts corresponding to the subtropical desert biome and presumably partly to the midlatitude desert biome were probably much more extensive, extending up to ca. 50°N/S.
To the south and north of the tropical wet and subtropical desert biomes lie the warm temperate (7 genera) and cool temperate biomes (10 genera; Supplementary Table 3). The warm temperate and cool temperate biomes occur on similar latitudes within the temperate belt in the Northern and Southern Hemispheres, with one outlier in the form of an assemblage in the northern polar region with a cool temperate character. In general, assemblages of the cool temperate biome are clustered in the northeastern and southeastern corners of Pangaea (Kazakhstan and North China in the North; Northern Australia in the South; Figure 2), whereas the warm temperate biome is found in the more central and western parts of the continent (European Russia, Brazil, Tibet, New South Wales). Both the warm temperate and cool temperate biomes are represented by plant fossil assemblages with a relatively low diversity (respectively 9 and 10 genera, belonging to five and six orders) and characterized by a mixture of spore- and pollen-producing plants. The cold temperate biome is only recorded in the Northern Hemisphere at one locality in Siberia, with a relatively low diversity (eight genera, five orders). Its presence in the southern polar region can only be inferred.
Looking at the diversity at different latitudes (Table 2), the most diverse latitudinal slice is the one between 20°N and 30°N (112 genera out of 150), which accounts for many assemblages of the tropical everwet biome. The second-most diverse latitudinal slice lies between 10°N and 20°N (73 genera out of 150) and includes the assemblages of the subtropical desert biome.
Changhsingian
More than 160 genera have been described so far from 102 localities for this stage (Table 4). During the Changhsingian (Figure 3), the tropical everwet biome (with occurrences of 87 genera) reduces its extension southwards on the North China block, but covers still South China, Turkey and the Qiantang terrane (Tibet). Climate models furthermore indicate its presence along the northern Tethys coast and near the equator at the eastern and western margin of Pangaea (Roscher et al., 2011). Like in the Wuchiapingian this biome includes Lepidodendrales (8 genera), Equisetales (5 genera), Sphenophyllales (3 genera), Marattiales (8 genera), Osmundales (6 genera), Cycadales (4 genera), Medullosales (4 genera), Gigantopteridales (2 genera), Cordaitales (3 genera) and Noeggerathiales (1 genus; Supplementary Table S3). The tropical everwet biome still includes survivors of the Carboniferous swamps, although the diversity in this biome is distinctively reduced at genus level (87 against 115) but not in its orders (19 against 17 in the Wuchiapingian).
In Europe, a subtropical desert biome persists with representatives of the Voltziales (2 genera), but the tropical summerwet biome of the Zechstein basin is no longer evident (Figure 3). The extent of the subtropical and midlatitude desert biomes is inferred by comparison with the older and younger biome distributions and coeval sediment indicators.
The warm temperate biome (35 genera) expands in the Northern Hemisphere to somewhat lower latitudes of the Russian platform and eastwards to North China. It comprises lycophytes (Isoetales, Pleuromeiales), sphenophytes (Equisetales), ferns (Osmundales, Marattiales), Ginkgoales, Cycadales, seed ferns (Peltaspermales) and conifers (Coniferales, Voltziales). The same biome is recognized in the Southern Hemisphere at only one locality in South Africa and characterized by seed ferns of the orders Glossopteridales and Peltaspermales. The temperate belt in the Southern Hemisphere is dominated by the cool temperate biome (Australia, South Africa, Antarctica; Figure 3), with abundant sphenophytes (Equisetales, Sphenophyllales), rare conifers (Coniferales) and Cordaitales, but dominated by the Glossopteridales (Supplementary Table S3). The Glossopteridales are absent in the Northern Hemisphere but rare Medullosales are recorded.
The cold temperate biome is well-represented in the Northern Hemisphere at several localities in Siberia, where its diversity is dominated by Equisetales (8 genera), Osmundales (6 genera), Marattiales (4 genera), Sphenophyllales (2 genera) and several fern-like and seed fern leaf taxa, including Peltaspermales (4 genera; Supplementary Table S3). In the Southern Hemisphere, this biome is only inferred.
The highest diversity lies during the Changhsingian in the tropical everwet biome (87 genera), while the second biome in order of diversity is the cold temperate biome (63 genera; Table 1). Also the warm and cool temperate biomes become more diverse. The latitudinal gradient between the plant diversity and the number of studied outcrops does not correlate anymore (Table 2). Although the diversity is still highest between 10°N and 30°N, the latitudinal slice between 60°N and 70°N is also highly diverse.
Induan
This stage is represented by 99 genera collected from 91 localities (Table 4). The tropical everwet biome (11 genera, 7 order) is reduced to a few localities in South China characterized by Lepidodendrales, Equisetales (3 genera), Sphenophyllales (2 genera), Osmundales, Peltaspermales, Cordaitales and Gigantopteridales (2 genera; Table 1; Supplementary Table S3). The tropical summerwet biome, rich in Gigantopteridales (2 genera), occurs nearby, so that individual areas for the two biomes are not readily distinguishable (Figure 4). Thus, we propose a transitional state between the tropical everwet and tropical summerwet biomes for this region. A clear tropical summerwet biome occurs in Euramerica. These plant assemblages are characterized by Pleuromeiales (3 genera) and Lepidodendrales (2 genera), Equisetales (3 genera), Peltaspermales (3 genera) and rare Voltziales. Sediment and climate modelling data support an extension of this biome for the Tethys Realm and a low latitudinal band of southern Euramerica. Consequently, the subtropical and midlatitude desert biomes are distinctly reduced during the Induan in the Northern Hemisphere, whereas arid areas on the Southern Hemisphere are similar to the Wuchiapingian, although possibly also slightly restricted in the higher latitudes. There are no floras that are assignable to the subtropical desert biome in the Induan.
The cool temperate biome disappears in the Northern Hemisphere in favour of the extension of the warm temperate biome. The latter is characterized by Pleuromeiales, Equisetales (6 genera), Osmundales (3 genera), Ginkgoales, Corystospermales, Peltaspermales, Coniferales (3 genera), Voltziales (2 genera) and Cordaitales (2 genera). The Southern Hemisphere is represented exclusively by a few localities in South Africa. These yielded a mixture of cool temperate (Equisetales, Sphenophyllales) and warm temperate plant assemblages (Coniferales), suggesting a mixed or transitional warm to cool temperate biome for the middle-high latitudes. The cold temperate biome is represented by numerous localities across Siberia, but is only inferred on the Southern Hemisphere. This biome is very diverse (75 genera, 15 order), with a dominance (in the number of taxa) of ferns (18 genera; Osmundales, Marattiales), followed by sphenophytes (10 genera; Equisetales, Sphenophyllales), conifers (10 taxa; Coniferales, Voltziales) and seed ferns (8 genera; Peltaspermales).
During the Induan a general decrease in the sampled diversity is observed (from 162 genera during the Wuchiapingian to 99 genera during the Induan; Table 4). The cold temperate biome is now the most diverse biome (75 genera against 63 during the Wuchiapingian). The wet or seasonal biomes of the lower latitudes reduce noticeably in diversity. Most important is the decrease from 87 genera in the Changhsingian to 11 genera in the Induan in the tropical everwet biome. The warm temperate biome reduces its diversity much less (25 against 35 during the Wuchiapingian), whereas the subtropical desert biome no longer has any plant records. The highest diversity and also number of localities is observed during the Induan at the high latitudes of the Northern Hemisphere, between 59 and 75°N (Table 2). Still diverse and represented by a relatively high number of outcrops is the latitudinal slice between 20 and 30°N in the Northern Hemisphere.
Olenekian
The Olenekian mostly resembles the Induan, but it shows an increase in observed taxa (127 genera) and sampled localities (110; Table 4), and the mixed/transitional states between the tropical everwet and tropical summerwet biomes in South China and between the cool temperate and warm temperate biomes in Gondwana have ended, as the tropical everwet and cool temperate biomes disappear (Figure 5). From the Olenekian onward, only three biomes can be distinguished through floral assemblages: 1) the tropical summerwet biome; 2) the warm temperate biome, 3) the cold temperate biome. The subtropical and midlatitude desert biomes are inferred based on sediment data and on comparisons with the older and younger stages. It is evident that the climate is becoming more and more dry both at the low, middle and high latitudes. No clear everwet biomes are present anymore.
The tropical summerwet biome substitutes the mixed tropical everwet-tropical summerwet biome area on the North China block and characterizes big parts of Europe and the northern tropical-subtropical belt. The plant assemblage composing it is diverse with a high generic richness among the conifers (14 genera, Coniferales, Voltziales), followed by ferns (9 genera, Osmundales, Marattiales, Filicales) and sphenophytes (6 genera, Equisetales; Table 1; Supplementary Table S3). The mixed cool temperate-warm temperate biome area of the Induan middle-high latitudes of the Southern Hemisphere corresponds now to the warm temperate biome. This biome is documented from East to West in localities in South Africa, India and Australia. In the Northern Hemisphere it is assignable to Northern China and parts of Russia. The warm temperate biome is characterized by a very diverse flora (105 genera belonging to 18 orders) with all major groups from the bryophytes to the conifers well represented. The main difference between the biome expressions of the Northern and Southern Hemispheres is the presence of Isoetales, ginkgophytes, Coniferales and Cordaitales in the Northern Hemisphere (16 orders) and Lycopodiales and Noeggerathiales in the Southern Hemisphere (12 orders in total). The cold temperate biome is represented by few plant assemblages in Siberia and is only inferred in the Southern Hemisphere. The few plant remains found so far in Siberia belong to the lycophytes (Pleuromeiales) and ferns (Osmundales).
During the Olenekian the number of studied outcrops increases by about 20% (110 instead of 91 during the Induan) whereas the number of genera increases by almost 30% (127 against 99 in the Induan; Table 4). The diversity decreases again at the poles and the warm temperate biome becomes the most diverse biome (105 genera, 17 orders), followed by the tropical summerwet biome (48 genera, 14 order; Table 1). The diversity in the Northern Hemisphere starts to resemble again that of the Permian stages, but the diversity increases noticeably in the Southern Hemisphere. The most diverse latitudinal slices are found at the middle latitudes of the Northern Hemisphere between 40 and 50°N (71 genera from 7 outcrops), and at the low latitudes between 10 and 20°N (41 genera from 41 outcrops; Table 2).
Anisian
The number and types of biomes identified from the Anisian is identical to the Olenekian; tropical summerwet biome, subtropical and midlatitude desert biomes, warm temperate biome, cold temperate biome (Figure 6). The desert biomes are again only inferred by sediments and not confirmed by plant remains. The Anisian differs from the Olenekian mostly in the amount sampled localities (281 against 110 during the Olenekian; Table 4) and somewhat in the spatial distribution of the biomes. Data from sediments support a noticeable extension of the tropical summerwet biome towards the south along the eastern coast of Pangea. This conversely restricts slightly the subtropical desert biome in the Southern Hemisphere (Figure 5).
The tropical summerwet biome, well-represented in South China and Europe is the most diverse biome of this stage (83 genera, 15 order) with a wide range of plant groups, with the most diverse plant group being the conifers (26 genera, Coniferales, Voltziales), followed by the ferns (17 genera, Marattiales, Osmundales) and the Cycadales (6 genera; Table 1; Supplementary Table S3). Interesting is also the first appearance of the Caytoniales among the seed ferns (Kustatscher et al., 2007). The warm temperate biome resembles in spatial distribution closely that of the Olenekian, with the difference that its presence at lower latitudes at the coasts in the South is evidenced by several plant assemblages in India and Argentina. The warm temperate biome is less diverse (globally 30 genera, 11 orders), with a higher diversity in the Southern Hemisphere (29 genera, 11 orders; Australia, India, South Africa, Argentina, Antarctica) than in the Northern Hemisphere (9 genera, 7 orders; North China). The main difference is the presence of Marattiales, Cycadales, Peltaspermales and Voltziales in the Southern Hemisphere. A similar situation is observed in the cold temperate biome (globally 34 genera, 13 orders), which is more diverse in the Southern Hemisphere (27 genera, 9 orders against 8 genera, six orders), with the presence of the characteristic Petriellales and Corystospermales. The cold temperate biome has its first clear record in Antarctica, in the southern polar region. There is in the same region a flora with a warm temperate composition, which is considered an outlier.
Latitudinally, most sampled localities and the highest generic diversity are located between 10 and 30°N. A relatively high diversity (28 genera) is also indicated at high southern latitudes between 70 and 80°S. In contrast to the South and to preceding stages, only few localities and low diversity are recorded northwards of 40°N.
Ladinian
The number of studied outcrops for the Ladinian almost doubles in comparison with the Anisian (457 against 281), but the taxonomic diversity increases only slightly (145 genera against 132 during the Anisian). The biomes in the Ladinian (Figure 7) are very similar to the Anisian (Figure 6), both in the number of distinguishable biomes and their spatial distribution. However, several assemblages from New Zealand become available, which indicate a warm temperate biome. Moreover, a warm temperate flora from Brazil suggests that the extension of the subtropical desert biome towards the high latitudes on the Southern Hemisphere may have slightly decreased.
As in the Anisian, the most diverse biome in the Ladinian is the tropical summerwet biome (109 genera, 18 orders; Table 1; Supplementary Table S3) which yielded plants only in the Northern Hemisphere (Europe, South China). The composition of the biome resembles closely that of the Anisian as well. The most diverse groups are the conifers (23 genera, Coniferales, Voltziales), followed by the cycads (18 genera, Cycadales, putative Bennettitales) and ferns (20 genera, Filicales, Marattiales, Osmundales). The warm temperate biome is less diverse (55 genera, 11 orders) with a higher diversity in the Southern Hemisphere (11 orders; Australia, South Africa, Brazil, Argentina, New Zealand) compared to the Northern Hemisphere (5 orders; North China, Russia). The main difference is the presence of Marattiales, Ginkgoales, Corystospermales and Cordaitales in the Southern Hemisphere. Apart from the Corystospermales, which are rare in the Northern Hemisphere but are one of the most characteristic plant groups of the Southern Hemisphere during the Triassic, all other orders are missing, although they are well-represented in the older and younger sediments of the same area (Supplementary Table S3). The cold temperate biome (7 genera, three orders) has a low diversity. In the Southern Hemisphere (Antarctica), this plant assemblage is only composed of ferns (Filicales, Marattiales, Osmundales), while in the Northern Hemisphere (Siberia) there are also Peltaspermales among the plant remains.
The most diverse and highly studied outcrops lie at the low latitudes of the Northern Hemisphere (between 10 and 30°N; Table 2). In the Southern Hemisphere, the most diverse latitudinal slices are those between 40 and 60°S, showing a general increase in diversity and sampling effort in the South (24 genera from 14 outcrops against 18 genera from 4 outcrops during the Anisian).
Discussion
Diversity Change Across the Biomes and Latitudes
Today the most diverse biome is the tropical everwet biome, followed by the warm temperate biome. Looking at the different stages, the tropical everwet biome is the most diverse biome during the Wuchiapingian (114 genera) but the second biome in order of diversity is the subtropical desert biome (37 genera; Table 1). This is also shown by the diversity gradient, where the highest diversity lies between 10 and 40°N, in correspondence with the spatial distribution of the tropical everwet biome in South China and the tropical summerwet and subtropical desert biomes in Europe (Table 2). These are also the regions with the highest concentration of documented floras (Table 3). There seems to exist an apparently direct correlation between the taxonomical diversity and the number of studied localities (Tables 1–3) suggesting an effect of the collecting effort on certain areas. At the very least, the dearth of assemblages from outside of Europe and China poses a limit on the possible coverage of the actual diversity, although this limit is not easily quantifiable.
The Changhsingian is represented by noticeably less localities yielding plant fossils compared to the Wuchiapingian (125 in the Wuchiapingian against 102 in the Changhsingian; Table 4), especially with regards to the end of this stage. The diversity (number of genera), on the other hand does not decrease, but rather increases for the time bin (162 against 150 in the Wuchiapingian). This can be explained by a higher number of well-sampled areas representing different biomes. Whereas documented assemblages in the Wuchiapingian overwhelmingly represent the tropical everwet and subtropical desert biomes, the Changhsingian has numerous localities with plant fossils from the tropical everwet, warm temperate, cool temperate and cold temperate biomes. The cold temperate biome becomes the second-most diverse biome during the Changhsingian, after the tropical everwet biome. However, also the warm and cool temperate biomes become more diverse in genera during this stage. Also the diversity gradient changes (Table 2). Although the diversity is still highest between 20 and 30°N (accounting for most of the tropical everwet assemblages), the latitudinal slice between 60 and 70°N, which contains the cold temperate biome in Siberia, is also highly diverse. This could be linked to the fact that a high number of plant-bearing Changhsingian outcrops is concentrated in this region (Table 3). It could, however, also be related to the fact that the increasing aridity and temperature favours a higher diversity in high latitudes, due to the extension of the growing seasons and increase in mean annual temperature in these latitudes. The water availability enabled a proliferation of hygrophytic plant groups such as sphenophytes and ferns (Supplementary Table S3).
During the Induan, a general decrease in diversity is observed, the number of identified genera decreases by about 40% (Table 4). At the same time, the number of studied outcrops yielding plant fossils does not decrease significantly (91 against 102 in the Changhsingian), but they are again more unevenly distributed. Most data come from the Northern Hemisphere (Table 2); the lack of data from the Southern Hemisphere is mostly due to a collecting and/or publishing artefact. The fact that the cold temperate biome became the most diverse biome, whereas the biomes of the low latitudes and the wet environments decrease noticeably in diversity are clearly not only due to collecting biases but related to the climate change across the Permian–Triassic boundary, with an increase in temperature and a decrease in precipitation (Preto et al., 2010). The highest diversity and also number of localities in the high latitudes of the Northern Hemisphere (Tables 2,3) could be linked to the special attention the Siberian area has received due to the existence of the Siberian Traps or represents an early recovery of the vegetation and floral cover after the eruptions of the Siberian Large Igneous Province.
The diversity gradient in the Northern Hemisphere becomes more centred on the middle latitudes during the Olenekian, contemporaneously with an increase in diversity in the Southern Hemisphere. The high diversity observed at the middle latitudes can mostly be linked to the detailed studies carried out in North China and Australia (Table 3). The apparently low diversity of the cold temperate biome compared to the two preceding stages and the Anisian is probably a collecting artefact considering that very few Olenekian assemblages are available from this biome.
During the Middle Triassic, the climatic and environmental conditions become more and more stable. The tropical summerwet biome becomes the most diverse biome both during the Ansian (83 genera) and the Ladinian (109 genera; Table 1). The most diverse latitudinal slice lies between 10 and 30°N (Table 2). Most data come from Europe, including the Vosges in France, various localities in Germany and Spain, and the Dolomites in Italy, as well as China (Table 3). The high diversity at high latitudes of the Southern Hemisphere during the Anisian, between 70 and 80°S, is dependent on the first records of plant assemblages from Antarctica within the studied interval, but may also correspond to a more favourable climate at high southern latitudes during this time slice. Interestingly, the number of studied outcrops in total more than doubles (281 against 110) whereas the number of taxa increases only slightly (132 against 127 in the Olenekian), which can be explained by the clustering of the vast majority of localities within the tropical summerwet biome in Europe and South China, creating a large overlap in sampled taxa (Tables 3, 4).
The Ladinian does not differ much from the Anisian in biome distribution and composition, although the number of studied outcrops increases distinctively at a worldwide scale (457 against 281 during the Anisian; Table 4). This increase is almost entirely limited to Central and South Europe (Germany and the Dolomites in Italy in particular; Table 3). The overall similarity between the Olenekian and Anisian could suggest that biotic recovery after the end-Permian mass extinction was reached already during the Olenekian, and not during the late Anisian as hypothesized previously (Grauvogel-Stamm and Ash, 2005). Another interpretation could be that the ongoing stabilisation of the various biomes did not increase anymore substantially the number of taxa but rather created an evolutionary pressure for the appearance of new taxa. However, there are still a lot of areas, especially at the low and middle latitudes of the Southern Hemisphere as well as North America and Russia, that are missing data and would need a detailed study.
Biome and Climate Shifts
The biome snapshots of the six stages (Wuchiapingian to Ladinian) show how the spatial distribution of the biomes changed trough time and how some biomes, especially the “wet” ones, well-represented during the late Permian, disappear in the Early and Middle Triassic. These shifts in biome distribution between successive stages are interpreted primarily as the results of a change in the global climate at large scale. The end-Permian mass extinction is considered to have caused a short-term cooling, followed by a severe warming of 6–8°C (or locally more) and generally hot and dry conditions in the Early Triassic (Preto et al., 2010; Joachimski et al., 2012; Sun et al., 2012; Schobben et al., 2014; Rey et al., 2016). This can account for the reduction of the tropical everwet and cool temperate biomes in the Induan and the appearance of the tropical summerwet biome in central Pangaea at latitudes that were previously occupied by the warm temperate biome. The climatic shift associated with the end-Permian mass extinction has also been linked to an increase in the ratio of spores (mostly from lycophytes) compared to gymnosperm pollen and to the dominance and widespread occurrence of lycophytes such as Pleuromeia in Early Triassic macrofloras (e.g., Looy et al., 1999; Grauvogel-Stamm and Ash, 2005). However, high-resolution palynological sampling shows that terrestrial floras were subject to repeated environmental disturbances during the Early Triassic (Hochuli et al., 2010; Hermann et al., 2011; Hermann et al., 2012; Hochuli et al., 2016). This aligns with our results showing a transitional biome distribution in the Induan.
Within the studied interval, both the tropical everwet and cool temperate biomes have their last appearances in the Induan. Here, both occur in the same regions as the tropical summerwet (South China) and warm temperate biomes (South Africa), respectively, which indicates an ongoing shift towards increasingly warm and seasonally dry conditions. The seasonal character of the tropical summerwet and the warm temperate biomes is reflected by a wide range of plant groups that characterize these two biomes, which are generally diverse including ferns, cycads, seed ferns and conifers, but also with representatives of all other major plant groups. An increase in seasonality matches the theory of a strengthened megamonsoon in the Triassic (Parrish, 1993). The Olenekian, Anisian and Ladinian floras are subsequently dominated by four types of biomes: 1) the tropical summerwet biome; 2) the warm temperate biome, 3) the subtropical/midlatitude desert biome(s) and the 4) cold temperate biome. By contrast, Ziegler et al. (1993) considered the cold temperate biome to be absent during the early Mesozoic in Eurasia, but recognized a cool temperate biome, while also assigning numerous Triassic floras in high palaeolatitudes Siberia to the warm temperate biome. Other authors have assigned the northern Siberian floras to a warm temperate climate as well (e.g., Yaroshenko, 1997). We found that floras in the higher palaeolatitudes of Siberia showed a typical character of the cold temperate biome due to the predominance of small, adaptable plants (lycophytes, sphenophytes, ferns), making them distinct from the warm temperate biome. A cool temperate biome in the Triassic could only be identified in the Induan of South Africa in our analysis, but it is conceivable that it persisted elsewhere in high altitude areas that were not conducive to preservation. The warm temperate biome that dominates the southern floral record in the Olenekian, Anisian and Ladinian corresponds to the seasonal subtropical evergreen forests described by Spalletti et al. (2003).
The tropical everwet and cool temperate biomes include the main (but not sole) areas for peat production. Their reduction in the Induan and subsequent absence in the Olenekian, Anisian and Ladinian is thus consistent with the so-called “coal gap,” referring to the almost complete absence of coal measures in the Early to Middle Triassic (Retallack et al., 1996). Although discrete swamps and peats are still missing at a worldwide scale in the Ladinian, small coal horizons start to appear such as the “Lettenkohle” in the Erfurt Formation of the Central European Basin (e.g., Kelber, 2015).
The subtropical desert biome was evidenced in our analysis in several European localities from the Wuchiapingian and Changhsingian, but in the Triassic this biome is missing. A subtropical dry biome was indicated by Ziegler et al. (1993) for the Triassic in Europe, where our results would place the tropical summerwet biome. In fact, the Induan–Olenekian Buntsandstein contains an alternating succession of floras from both subtropical desert and tropical summerwet biomes (Grauvogel-Stamm and Kustatscher, in press), as well as sedimentary evidence of arid and semi-arid to humid conditions (Voigt, 2017; Paul, in press). However, this is obscured by the stratigraphic resolution of our analysis. Limitations due to the chronostratigraphic resolution of the available data and the necessity to bin the various outcrops into defined time intervals (in our case stages) do not permit to show short-term changes in climate within the stages. Even if such changes would lead to shifts in the palaeofloras, these may be obscured after binning roughly coeval localities and result in ambiguities. It can be expected that only relatively stable, long-term conditions will produce a clear signal. Furthermore, because sampling is limited to mostly isolated areas, the boundaries between biomes can only be approximated. This is related to the fact that not all biomes provide favourable conditions for the preservation of plant fossils, due to the fact that the plants themselves do not have equally high preservation potentials and the sedimentation rates and fossilisation potential of the various sediments may vary noticeably (Ziegler, 1990).
As far as the resolution of our data can show, the biomes appear to have been largely similar between the Olenekian and the Ladinian, which would suggest relatively stable climate conditions. There is evidence (based on spore-pollen assemblages, ammonoids and geochemistry) for an abrupt climate shift in the Olenekian, at the Smithian–Spathian boundary, which is supposed to be linked to an important change in plant communities from lycophyte-dominated to a recovery of gymnosperms (Galfetti et al., 2007; Romano et al., 2013; Saito et al., 2013), but this is not evident in our biome maps. In this context, it is important to consider that biomes can persist even when the composition of floras changes.
Outlook
Most of the palaeogeographical regions considered in this study do not have a continuous plant fossil record at the stage level, and individual stages with records vary strongly in the amount of available data (Table 3). The Induan in particular is underrepresented in all regions except for Siberia. This limits our ability to accurately trace changes in phytogeography across the Permian–Triassic boundary. The effect of the amount and distribution of plant fossil localities on apparent biodiversity has also been noted by Rees (2002), who considered variations in sampling as a factor for explaining global and regional diversity patterns, alongside geography and climate. While our dataset is different, it shows similar patterns and evidence that the apparent biodiversity of land plants in the Induan at a global scale is influenced by a lack of data (Nowak et al., 2019).
The gaps in regional records will necessarily exclude all endemic taxa from global biodiversity estimates. Conversely, studies on endemism/cosmopolitanism and migration also need to take such gaps into account. A relatively simple estimate of the number of endemic genera indicates a high degree of endemism (Table 4). The expected impact of the record gaps – and to a smaller degree of undersampled stages – is therefore also high. The stratigraphic ranges of affected taxa will be truncated as well, which means that the timing and rates of originations and extinctions will be distorted. This is particularly relevant in the case of the Permian–Triassic transition, as the plant fossil record would seem to indicate a drastic loss of diversity if taken at face value (Nowak et al., 2019).
While this analysis was based on the taxonomic composition of land plant macrofossil assemblages and took into account climate-sensitive sediment data and palaeotopography, there are other lines of evidence that could potentially enhance the results. Quantitative data on the dominance structure of the plant communities were disregarded in this analysis because this sort of information is not always reported, and not in a consistent way when it is. It is also subject to taphonomic and sampling bias to an even greater extent than mere presence/absence data and its reliability therefore questionable. Leaf sizes and shapes (Royer et al., 2005) as well as cuticle thickness (Ziegler, 1990) and stomatal indices (Retallack, 2001) are other ways in which plant fossils can serve as environmental proxies. Spore/pollen data are available from many areas where no macrofloras have been documented (Nowak et al., 2019) and can provide information about the surrounding plant communities, but the source area can be vast and environmental interpretations of spore/pollen assemblages are dependant on reliable associations between dispersed sporomorph taxa and biological plant groups. Such associations are not available for many dispersed taxa, and in many more cases they are ambiguous (e.g., Balme, 1995). Still, this may improve in the future, as we understand better the connections between plant macro- and microfossils. Furthermore, soils are specifically relevant for the identification of biomes (Walter, 1985; Ziegler, 1990), and thus a compilation of palaeosoil data would be useful. Another useful tool are climate simulations, which in the studied interval are only available with sufficient detail for the Changhsingian so far (Roscher et al., 2011). Climate models can indicate where the conditions for certain biomes were met, while conversely, plant fossils can provide means to calibrate the models. In addition, tetrapods have been shown to have potential as climate indicators (Rey et al., 2016; Bernardi et al., 2018), and their distribution can be linked to plants as well (Rees et al., 2004; Bernardi et al., 2017). Ideally, all types of information would be used to construct a coherent biome model. Our current study will hopefully be a step in that direction.
Data Availability Statement
The original contributions presented in the study are included in the article/Supplementary Material, further inquiries can be directed to the corresponding author.
Author Contributions
HN and EK conceived the study. EK determined plant-biome associations. EK and HN assigned localities to coordinates. CV calculated palaeogeographic coordinates. HN performed statistical analyses. CV and HN produced the figures. HN and EK wrote the manuscript. All authors contributed to the interpretation of results, edited and revised the text.
Funding
This work was funded under the Euregio Science Fund (call 2014, IPN16: “The end-Permian mass extinction in the Southern and Eastern Alps: extinction rates vs taphonomic biases in different depositional environments”) of the Europaregion/Euregio Tirol-Südtirol-Trentino/Tirolo-Alto Adige-Trentino and by the Forschungsfonds des Betriebs Landesmuseen as part of the research project “MAMPFT – Mikrosporen an MakroPflanzen-Fossilien der Trias”. EK received support for the study of the unedited material included in our raw data at European natural history collections through the SYNTHESYS and SYNTHESYS+ projects (funded by the European Commission) “Biodiversity of the Buntsandstein and Muschelkalk (Early and Middle Triassic) floras in the Central European Basin” (DE-TAF-2463) and “Palaeozoic-relict and ”modern” Mesozoic taxa in the Ladinian and Carnian floras of Europe” (DE-TAF-5898).
Conflict of Interest
The authors declare that the research was conducted in the absence of any commercial or financial relationships that could be construed as a potential conflict of interest.
Acknowledgments
Elke Schneebeli-Hermann (University of Zurich, Switzerland) is thanked for suggesting the cooperation that made this study possible.
Supplementary Material
The Supplementary Material for this article can be found online at: https://www.frontiersin.org/articles/10.3389/feart.2020.613350/full#supplementary-material.
References
Artabe, A. E., Morel, E. M., and Spalletti, L. A. (2003). Caracterización de las provincias fitogeográficas triásicas del Gondwana extratropical. Ameghiniana 40, 387–405.
Balme, B. E. (1995). Fossil in situ spores and pollen grains: an annotated catalogue. Rev. Palaeobot. Palynol. 87, 81–323. doi:10.1016/0034-6667(95)93235-X
Benton, M. J., and Newell, A. J. (2014). Impacts of global warming on Permo-Triassic terrestrial ecosystems. Gondwana Res. 25, 1308–1337. doi:10.1016/j.gr.2012.12.010
Benton, M. J., and Twitchett, R. J. (2003). How to kill (almost) all life: the end-Permian extinction event. Trends Ecol. Evol. 18, 358–365. doi:10.1016/S0169-5347(03)00093-4
Bernardi, M., Petti, F. M., Kustatscher, E., Franz, M., Hartkopf-Fröder, C., Labandeira, C. C., et al. (2017). Late Permian (Lopingian) terrestrial ecosystems: a global comparison with new data from the low-latitude Bletterbach Biota. Earth Sci. Rev. 175, 18–43. doi:10.1016/j.earscirev.2017.10.002
Bernardi, M., Petti, F. M., and Benton, M. J. (2018). Tetrapod distribution and temperature rise during the Permian-Triassic mass extinction. Proc. R. Soc. B. 285, 20172331. doi:10.1098/rspb.2017.2331
Boucot, A. J., Xu, C., Scotese, C. R., and Morley, R. J. (2013). Phanerozoic paleoclimate: an atlas of lithologic indicators of climate. Tulsa, Oklahoma, USA: SEPM (Society for Sedimentary Geology). doi:10.2110/sepmcsp.11
Broutin, J., Yu, J., Shi, X., Shu, W., and Qing, X. (2020). Terrestrial palaeofloral succession across the Permian-Triassic Boundary in the North and South China blocks: a brief review. PalZ 94, 633. doi:10.1007/s12542-020-00511-0
Cascales-Miñana, B., and Cleal, C. J. (2014). The plant fossil record reflects just two great extinction events. Terra. Nova 26, 195–200. doi:10.1111/ter.12086
Cascales-Miñana, B., Diez, J. B., Gerrienne, P., and Cleal, C. J. (2016). A palaeobotanical perspective on the great end-Permian biotic crisis. Hist. Biol. 28, 1066–1074. doi:10.1080/08912963.2015.1103237
Chumakov, N. M., and Zharkov, M. A. (2002). Climate during Permian–Triassic biosphere reorganizations, article 1: climate of the early Permian. Stratigr. Geol. Correl. 10, 586–602.
Chumakov, N. M., and Zharkov, M. A. (2003). Climate during the Permian–Triassic biosphere reorganizations. Article 2. Climate of the late Permian and Early Triassic: general inferences. Stratigr. Geol. Correl. 11, 361–375.
Cúneo, N. R. (1996). Permian phytogeography in Gondwana. Palaeogeogr. Palaeoclimatol. Palaeoecol. 125, 75–104. doi:10.1016/S0031-0182(96)00025-9
Delfino, M., Kustatscher, E., Lavezzi, F., and Bernardi, M. (2020). “The end-Permian mass extinction: nature’s revolution,” in Nature through time: Virtual field trips through the Nature of the past springer textbooks in earth sciences, geography and environment. Editors E., Martinetto, E., Tschopp, and R. A., Gastaldo (Cham, Switzerland: Springer Nature Switzerland AG), 253–267. doi:10.1007/978-3-030-35058-1_10
Fielding, C. R., Frank, T. D., McLoughlin, S., Vajda, V., Mays, C., Tevyaw, A. P., et al. (2019). Age and pattern of the southern high-latitude continental end-Permian extinction constrained by multiproxy analysis. Nat. Commun., 10, 385. doi:10.1038/s41467-018-07934-z
Fluteau, F., Besse, J., Broutin, J., and Ramstein, G. (2001). The Late Permian climate. What can be inferred from climate modelling concerning Pangea scenarios and Hercynian range altitude?. Palaeogeogr. Palaeoclimatol. Palaeoecol. 167, 39–71. doi:10.1016/s0031-0182(00)00230-3
Galfetti, T., Hochuli, P. A., Brayard, A., Bucher, H., Weissert, H., and Vigran, J. O. (2007). Smithian-Spathian boundary event: evidence for global climatic change in the wake of the end-Permian biotic crisis. Geol. 35, 291–294. doi:10.1130/G23117A.1
Gastaldo, R. A., Kamo, S. L., Neveling, J., Geissman, J. W., Looy, C. V., and Martini, A. M. (2020). The base of the Lystrosaurus Assemblage Zone, Karoo Basin, predates the end-Permian marine extinction. Nat. Commun. 11, 1428. doi:10.1038/s41467-020-15243-7
Grauvogel-Stamm, L., and Ash, S. R. (2005). Recovery of the Triassic land flora from the end-Permian life crisis. Comptes Rendus Palevol 4, 593–608. doi:10.1016/j.crpv.2005.07.002
Grauvogel-Stamm, L., and Kustatscher, E. (in press) “Makrofloren der Germanischen Trias: Buntsandstein und Muschelkalk,” in Trias – Aufbruch ins Erdmittelalter. Editors N. Hauschke, G. H. Bachmann, and M. Franz (München, Germany: Verlag Dr. Pfeil), 218–227.
Hermann, E., Hochuli, P. A., Bucher, H., Brühwiler, T., Hautmann, M., Ware, D., et al. (2011). Terrestrial ecosystems on North Gondwana following the end-Permian mass extinction. Gondwana Res. 20, 630–637. doi:10.1016/j.gr.2011.01.008
Hermann, E., Hochuli, P. A., Bucher, H., Brühwiler, T., Hautmann, M., Ware, D., et al. (2012). Climatic oscillations at the onset of the Mesozoic inferred from palynological records from the North Indian Margin. J. Geol. Soc. 169, 227–237. doi:10.1144/0016-76492010-130
Hochard, C. (2008). GIS and geodatabases application to global scale plate tectonic modelling. Lausanne, Switzerland: DissertationUniversity of Lausanne.
Hochuli, P. A., Sanson-Barrera, A., Schneebeli-Hermann, E., and Bucher, H. (2016). Severest crisis overlooked-Worst disruption of terrestrial environments postdates the Permian-Triassic mass extinction. Sci. Rep. 6, 28372. doi:10.1038/srep28372
Hochuli, P. A., Vigran, J. O., Hermann, E., and Bucher, H. (2010). Multiple climatic changes around the Permian-Triassic boundary event revealed by an expanded palynological record from mid-Norway. Geol. Soc. Am. Bull. 122, 884–896. doi:10.1130/B26551.1
Joachimski, M. M., Lai, X., Shen, S., Jiang, H., Luo, G., Chen, B., et al. (2012). Climate warming in the latest Permian and the Permian-Triassic mass extinction. Geology 40, 195–198. doi:10.1130/G32707.1
Kelber, K.-P. (2015). “Die Makroflora des Lettenkeupers,,”. Der lettenkeuper-ein fenster in die zeit vor den dinosauriern.-palaeodiversity supplement. Editors H., Hagdorn, R., Schoch, and G., Schweigert (Stuttgart, Germany: Staatliches Museum für Naturkunde), 51–100.
Kustatscher, E., Bernardi, M., Petti, F. M., Franz, M., Van Konijnenburg-van Cittert, J. H. A., and Kerp, H. (2017). Sea-level changes in the Lopingian (late Permian) of the northwestern Tethys and their effects on the terrestrial palaeoenvironments, biota and fossil preservation. Global Planet. Change 148, 166–180. doi:10.1016/j.gloplacha.2016.12.006
Kustatscher, E., Wachtler, M., and Van Konijnenburg-Van Cittert, J. H. A. (2007). Horsetails and seed ferns from the Middle Triassic (Anisian) locality Kühwiesenkopf (Monte Prà della Vacca), Dolomites, northern Italy. Palaeontology 50, 1277–1298. doi:10.1111/j.1475-4983.2007.00707.x
Kutzbach, J. E., and Ziegler, A. M. (1993). Simulation of Late Permian climate and biomes with an atmosphere-ocean model: comparisons with observations. Philos. Trans. R. Soc. B 341, 327–340.
Looy, C. V., Brugman, W. A., Dilcher, D. L., and Visscher, H. (1999). The delayed resurgence of equatorial forests after the Permian-Triassic ecologic crisis. Proc. Natl. Acad. Sci. Unit. States Am. 96, 13857–13862. doi:10.1073/pnas.96.24.13857
Mays, C., Vajda, V., Frank, T. D., Fielding, C. R., Nicoll, R. S., Tevyaw, A. P., et al. (2020). Refined Permian -Triassic floristic timeline reveals early collapse and delayed recovery of south polar terrestrial ecosystems. GSA Bulletin, 132, 1489–1513. doi:10.1130/B35355.1
Mishra, S., Aggarwal, N., and Jha, N. (2017). Palaeoenvironmental change across the Permian-Triassic boundary inferred from palynomorph assemblages (Godavari Graben, south India). Palaeobio. Palaeoenv. 98, 177–204. doi:10.1007/s12549-017-0302-3
Nowak, H., Schneebeli-Hermann, E., and Kustatscher, E. (2019). No mass extinction for land plants at the Permian-Triassic transition. Nat. Commun. 10, 1–8. doi:10.1038/s41467-018-07945-w
Parrish, J. T. (1993). Climate of the supercontinent Pangea. J. Geol. 101, 215–233. doi:10.1086/648217
Paul, J. (in press) “Klima der Trias im Germanischen Becken,” in Trias – Aufbruch ins Erdmittelalter. Editors N. Hauschke, G. H. Bachmann, and M. Franz (München, Germany: Verlag Dr. Pfeil), 152 –157.
Preto, N., Kustatscher, E., and Wignall, P. B. (2010). Triassic climates-state of the art and perspectives. Palaeogeogr. Palaeoclimatol. Palaeoecol. 290, 1–10. doi:10.1016/j.palaeo.2010.03.015
Rees, P. M., Ziegler, A. M., and Valdes, P. J. (1999b). “Jurassic phytogeography and climates: new data and model comparisons,” in In warm climates in earth history. Editors B. T., Huber, K. G., Macleod, and S. L., Wing (Cambridge, UK: Cambridge University Press), 297–318. doi:10.1017/CBO9780511564512.011
Rees, P. M., Gibbs, M. T., Ziegler, A. M., Kutzbach, J. E., and Behling, P. J. (1999a). Permian climates: Evaluating model predictions using global paleobotanical data. Geology 27, 891. doi:10.1130/0091-7613(1999)027<0891:PCEMPU>2.310.1130/0091-7613(1999)027<0891:pcempu>2.3.co;2
Rees, P. M. (2002). Land-plant diversity and the end-Permian mass extinction. Geology. 30, 827–830. doi:10.1130/0091-7613(2002)030<0827
Rees, P. M., Noto, C. R., Parrish, J. M., and Parrish, J. T. (2004). Late Jurassic climates, vegetation, and dinosaur distributions. J. Geol. 112, 643–653. doi:10.1086/424577
Rees, P. M., Ziegler, A. M., Gibbs, M. T., Kutzbach, J. E., Behling, P. J., and Rowley, D. B. (2002). Permian phytogeographic patterns and climate data/model comparisons. J. Geol. 110, 1–31. doi:10.1086/324203
Rees, P. M., and Ziegler, A. M. (1996). Jurassic floral gradients and climates. Spec. publ. (Paleontol. Soc.) 8, 319. doi:10.1017/S247526220000321X
Rees, P. M., Ziegler, A. M., and Valdes, P. J. (2000). “Jurassic phytogeography and climates: new data and model comparisons,” in Warm Climates in Earth History. Editors B. T. Huber, K. G. Macleod, and S. L. Wing (Cambridge, UK: Cambridge University Press), 297–318.
Retallack, G. J. (2001). A 300-million-year record of atmospheric carbon dioxide from fossil plant cuticles. Nature 411, 287–290. doi:10.1038/35077041
Retallack, G. J., Veevers, J. J., and Morante, R. (1996). Global coal gap between Permian-Triassic extinction and Middle Triassic recovery of peat-forming plants. Geol. Soc. Am. Bull. 108, 195–207. doi:10.1130/0016-7606(1996)108<0195:GCGBPT>2.310.1130/0016-7606(1996)108<0195:gcgbpt>2.3.co;2
Rey, K., Amiot, R., Fourel, F., Rigaudier, T., Abdala, F., Day, M. O., et al. (2016). Global climate perturbations during the Permo-Triassic mass extinctions recorded by continental tetrapods from South Africa. Gondwana Res. 37, 384–396. doi:10.1016/j.gr.2015.09.008
Romano, C., Goudemand, N., Vennemann, T. W., Ware, D., Schneebeli-Hermann, E., Hochuli, P. A., et al. (2013). Climatic and biotic upheavals following the end-Permian mass extinction. Nature Geosci 6, 57–60. doi:10.1038/ngeo1667
Roscher, M., Stordal, F., and Svensen, H. (2011). The effect of global warming and global cooling on the distribution of the latest Permian climate zones. Palaeogeogr. Palaeoclimatol. Palaeoecol. 309, 186–200. doi:10.1016/j.palaeo.2011.05.042
Royer, D. L., Wilf, P., Janesko, D. A., Kowalski, E. A., and Dilcher, D. L. (2005). Correlations of climate and plant ecology to leaf size and shape: potential proxies for the fossil record. Am. J. Bot. 92, 1141–1151.doi:10.3732/ajb.92.7.1141
Saito, R., Kaiho, K., Oba, M., Takahashi, S., Chen, Z.-Q., and Tong, J. (2013). A terrestrial vegetation turnover in the middle of the Early Triassic. Global Planet. Change 105, 152–159. doi:10.1016/j.gloplacha.2012.07.008
Schobben, M., Joachimski, M. M., Korn, D., Leda, L., and Korte, C. (2014). Palaeotethys seawater temperature rise and an intensified hydrological cycle following the end-Permian mass extinction. Gondwana Res. 26, 675–683. doi:10.1016/j.gr.2013.07.019
Sellwood, B. W., and Valdes, P. J. (2006). Mesozoic climates: general circulation models and the rock record. Sediment. Geol. 190, 269–287. doi:10.1016/j.sedgeo.2006.05.013
Spalletti, L. A., Artabe, A. E., and Morel, E. M. (2003). Geological factors and evolution of southwestern Gondwana Triassic plants. Gondwana Res. 6, 119–134. doi:10.1016/S1342-937X(05)70648-1
Stampfli, G. M., and Borel, G. D. (2002). A plate tectonic model for the Paleozoic and Mesozoic constrained by dynamic plate boundaries and restored synthetic oceanic isochrons. Earth Planet Sci. Lett. 196, 17–33. doi:10.1016/S0012-821X(01)00588-X
Stanley, S. M. (2016). Estimates of the magnitudes of major marine mass extinctions in Earth history. Proc. Natl. Acad. Sci. USA 113, E6325–E6334. doi:10.1073/pnas.1613094113
Sun, G., Meng, F., Qian, L., and Ouyang, S. (1995). “Triassic Floras,” in Fossil floras of China through the geological ages. Editors X., Li, Z., Zhou, C., Cai, G., Sun, S., Ouyang, and L., Deng (Guangzhou, China: Guangzhou Science and Technology Press), 305–342.
Sun, Y., Joachimski, M. M., Wignall, P. B., Yan, C., Chen, Y., Jiang, H., et al. (2012). Lethally hot temperatures during the Early Triassic greenhouse. Science 338, 366–370. doi:10.1126/science.1224126
Vajda, V., McLoughlin, S., Mays, C., Frank, T. D., Fielding, C. R., Tevyaw, A., et al. (2020). End-Permian (252 Mya) deforestation, wildfires and flooding --An ancient biotic crisis with lessons for the present. Earth Planet. Sci. Lett., 529, 115875. doi:10.1016/j.epsl.2019.115875
Vakhrameev, V. A., Dobruskina, I. A., Meyen, S. V., and Zaklinskaja, E. D. (1978). Paläozoische und Mesozoische Floren Eurasiens und die Phytogeographie dieser Zeit. Stuttgart, Jena, NY, USA: VEB Gustav Fischer Verlag
Vérard, C., Hochard, C., Baumgartner, P. O., Stampfli, G. M., and Liu, M. (2015a). 3D palaeogeographic reconstructions of the Phanerozoic versus sea-level and Sr-ratio variations. J. Palaeogeogr. 4, 64–84. doi:10.3724/SP.J.1261.2015.00068
Vérard, C., Hochard, C., Baumgartner, P. O., Stampfli, G. M., and Liu, M. (2015b). Geodynamic evolution of the Earth over the Phanerozoic: plate tectonic activity and palaeoclimatic indicators. J. Palaeogeogr. 4, 167–188. doi:10.3724/SP.J.1261.2015.00072
Vérard, C. (2019b). Panalesis: towards global synthetic palaeogeographies using integration and coupling of manifold models. Geol. Mag. 156, 320–330. doi:10.1017/S0016756817001042
Vérard, C. (2019a). Plate tectonic modelling: review and perspectives. Geol. Mag. 156, 208–241. doi:10.1017/S0016756817001030
Visscher, H., and Van der Zwan, C. J. (1981). Palynology of the circum-Mediterranean Triassic: phytogeographical and palaeoclimatological implications. Geol. Rundsch. 70, 625–634. doi:10.1007/bf01822140
Voigt, T. (2017). Die Ablagerungssysteme des Unteren und Mittleren Buntsandsteins in Thüringen. Geowissenschaftliche Mitt. Thüringen 14, 39–104.
Walter, H. (1985). Vegetation of the earth and ecological systems of the geo-biosphere. 3rd Edn. Berlin Heidelberg: Springer-Verlag
Yaroshenko, O. P. (1997). Palynology and phytogeography of the Early Triassic. Palaeontol. J. 31, 168–177
Ziegler, A. M., Parrish, M., Jiping, Y., Gyllenhaal, E. D., Rowley, D. B., Parrish, J. T., et al. (1993). Early Mesozoic phytogeography and climate. Philos. Trans. R. Soc. 297–305. doi:10.1098/rstb.1993.0115
Keywords: palaeophytogeography, biomes, land plants, Permian, Triassic, end-Permian mass extinction
Citation: Nowak H, Vérard C and Kustatscher E (2020) Palaeophytogeographical Patterns Across the Permian–Triassic Boundary. Front. Earth Sci. 8:613350. doi: 10.3389/feart.2020.613350
Received: 02 October 2020; Accepted: 12 November 2020;
Published: 08 December 2020.
Edited by:
Martin Daniel Ezcurra, Museo Argentino de Ciencias Naturales Bernardino Rivadavia, ArgentinaReviewed by:
Silvia Césari, Consejo Nacional de Investigaciones Científicas y Técnicas (CONICET), ArgentinaShreya Mishra, Birbal Sahni Institute of Palaeosciences (BSIP), India
Copyright © 2020 Nowak, Vérard and Kustatscher. This is an open-access article distributed under the terms of the Creative Commons Attribution License (CC BY). The use, distribution or reproduction in other forums is permitted, provided the original author(s) and the copyright owner(s) are credited and that the original publication in this journal is cited, in accordance with accepted academic practice. No use, distribution or reproduction is permitted which does not comply with these terms.
*Correspondence: Hendrik Nowak, aGVuZHJpay5ub3dha0BuYXR1cm11c2V1bS5pdA==