- 1Department of Oceanography, Federal University of Pernambuco, Recife, Brazil
- 2Department of Earth Sciences, ETH Zürich, Zurich, Switzerland
- 3Department of Geology, University of Oviedo, Oviedo, Spain
- 4Institute of Geosciences, Christian-Albrechts-Universität zu Kiel, Kiel, Germany
- 5Bazaruto Center for Scientific Studies, Inhambane, Mozambique
Coccolithophores are one of the major contributors to the pelagic production of calcium carbonate and their fossilized remains are a key component of the biogeochemical cycles of calcium (Ca), magnesium (Mg), and other divalent cations present in the intracellular precipitated calcitic structures (coccoliths). The geochemical signature of coccoliths (e.g., Sr/Ca and Mg/Ca ratios) is used as paleoproxy to reconstruct past environmental conditions and to understand the underlying physiological precipitation kinetics. Here, we present the elemental fractionation of Sr and Mg in calcite of the coccolithophore Gephyrocapsa oceanica from controlled laboratory experiments applying an extended temperature gradient (12 to 27°C). The physiological reaction norm of G. oceanica, in terms of growth rate, exhibited optimum behavior while the partition coefficient of Sr (DSr) was linearly correlated with temperature and DMg indicated no specific trend. Our results indicate: (1) a presumably secondary physiological control of DSr, and (2) the importance of calibrating coccolithophore-based proxies using experiments that include the full physiological reaction norms (i.e., a possible non-linear response) to environmental drivers (e.g., temperature, salinity, and pH, etc.). The presented results contribute to an improved understanding of the underlying physiological kinetics involved in regulating coccolith elemental fractionation and give additional implications for designing future laboratory experiments to calibrate and apply coccolithophore based paleoproxies on the fossil sediment record.
Introduction
The pelagic production of calcium carbonate (CaCO3) in the ocean is mainly dominated by coccolithophores and foraminifera. Coccolithophores are distinguishable from other phytoplankton functional groups by the presence of a cell cover of elaborated calcitic platelets, named coccoliths, contributing to the calcareous sediment record since the Late Triassic (Bown et al., 2004). The abundance, diversity, geometry, and geochemical composition of coccoliths have been the subject of paleoceanographic studies in order to reconstruct past environmental settings and changes (Stoll and Ziveri, 2004; Erba, 2006; Beaufort et al., 2011; Ziveri et al., 2012). The incorporation and fractionation of divalent cations (e.g., Ca, Sr, and Mg) into the calcite lattice of coccoliths depends on a diverse set of physicochemical and physiological parameters. Disentangling the environmental from the physiological factors of elemental fractionation is an important step in understanding and applying coccolithophore based paleoproxies. The coccolith Sr/Ca ratio has been predominately associated with coccolithophore productivity and growth dynamics (Stoll and Schrag, 2000; Stoll and Ziveri, 2004; Stoll et al., 2007) while the application of coccolith Mg/Ca ratio as paleoproxy is under discussion (Ries, 2010; Müller et al., 2014; Li et al., 2016). A careful calibration of these paleoproxies needs to be realized via controlled laboratory experiments with different coccolithophore species, testing individual and combined environmental parameters (e.g., Gibbs et al., 2013; Hermoso et al., 2014; Müller et al., 2018). The experimental testing of an extended pCO2/pH gradient revealed that physiological reaction norms of coccolithophores (in terms of growth rate) cannot be matched with coccolith Sr/Ca ratios when the gradient passes the optimum growth point of the species (Müller et al., 2014). While this has been tested for changes in seawater carbonate chemistry, where several parameters (e.g., CO2, HCO3– availability and H+ concentration) can be responsible for the physiological response of coccolithophore species (Bach et al., 2011), it remains to be tested for single ambient parameters, such as temperature. Here, we tested if the observed divergence between coccolithophore physiology and DSr, observed under an extended pCO2/pH gradient, is also valid under an extended temperature gradient using the cosmopolitan species Gephyrocapsa oceanica. Experimental temperature gradient was generated to observe physiological (i.e., growth rate) and geochemical (coccolith Sr/Ca and Mg/Ca) responses above and below the species optimum growth temperature.
Materials and Methods
Coccolithophore Culture Conditions and Experimental Set-Up
Artificial seawater was prepared according to Kester et al. (1967) as basis for coccolithophore culture media (salinity of 35 psu). Macronutrients (nitrogen and phosphorus) were added and verified according to Hansen and Koroleff (1999), resulting in final average concentrations of 55.3 ± 6.7 μmol NO3 kg–1 and 3.0 ± 0.6 μmol PO4 kg–1 for all replicate treatments (±1 sd, n = 18). Trace metals and vitamins were added according to f/8 culture media (Guillard, 1975). Culture media was sterilized using a Whatman® PolycapTM 75 AS filter with a pore size of 0.2 μm and adjusted to ambient pCO2 conditions of ∼400 μatm through appropriate additions of HCl and Na2CO3 stock solutions, according to the EPOCA Guide for Best Practices for ocean acidification research (Riebesell et al., 2010). A monoculture of the coccolithophore G. oceanica RCC1303 was obtained from the Roscoff Culture Collection. The strain was cultured and maintained in diluted batch mode, keeping cell densities below 100,000 cells ml–1. Cultures and growth media were acclimated to the experimental temperatures (12, 15, 18, 21, 24, and 27°C) for at least eight generations before experimentation. All incubations were conducted at the Helmholtz Centre for Ocean Research Kiel (GEOMAR) in temperature controlled climate chambers (RUMED Rubarth Apparate GmbH), providing a temperature accuracy of ±0.5°C. Each temperature experiment was conducted in triplicate 4 L acid cleaned and autoclaved polycarbonate bottles (NalgeneTM) with a minimal headspace and growth media adjusted to the experimental temperatures. Cultures received a photosynthetic active radiation of 135 μmol photon m–2 s–1 under a light:dark cycle of 16:8 h. In all experiments, cultures were inoculated at low cell densities and allowed to grow exponentially for 7 to 10 generations, corresponding to a maximum dissolved inorganic carbon (CT) consumption of 10%. Initial growth media was sampled for CT, total alkalinity (AT), elemental composition and macronutrient concentrations. In order to ensure homogenous cell distribution, incubation bottles were manually rotated once a day. At the termination of the experiment (1 to 4 h after the onset of the light phase) samples were collected for CT, AT, cell number, total particulate carbon (TPC), particulate organic carbon and nitrogen (POC and PON, respectively), chlorophyll-a (Chl-a) and coccolith elemental composition. Experiments were terminated at an average cell density of 63,967 ± 27,314 cells ml–1 (±1 sd, n = 18) to ensure nutrient replete conditions at the time of sampling. Samples for AT and CT were filtrated through GF/F filters (Whatmann, pre-combusted at 500°C for 6 h), poisoned with HgCl2 to avoid biological activity and kept till analysis at 6°C in 500 ml borosilicate flasks. Total alkalinity and CT were analyzed by the Service National d’Analyze des paramètres Océaniques du CO2 (SNAPO-CO2; Edmond, 1970) and corrected to Certified Reference Materials (provided by Scripps Institution of Oceanography, batch 111) with a reproducibility (1σ) of better than 3 μmol kg–1 for both CT and AT (detailed analytical report is provided in the Supplementary Material). One single measurement of AT and CT was performed per biological replicate (=per incubation bottle) before starting incubation and at the end of incubation (n = 6). Carbonate system parameters were calculated from temperature, salinity, phosphate concentrations, CT and AT (means calculated from start and end values of the incubation period) using CO2SYS (v.2.1 by E. Lewis and D. W. R. Wallace), with the stoichiometric equilibrium constants for carbonic acid given in Roy et al. (1993). Total averaged values of AT and CT were 2169 ± 148 and 1920 ± 130 μmol kg–1 (±1 sd, n = 6) with the corresponding calculated pCO2, pH, and Ωcalcite values of 404 ± 71 μatm, 8.03 ± 0.07, and 4.2 ± 0.4, respectively (Supplementary Table 1).
Physiological Parameters
Samples for cell numbers were analyzed directly after sampling using a Beckman Z2 Coulter®Particle Counter, and growth rate (μ) was calculated according to
where c0 and c1 are the cell concentrations at the beginning (t0) and end of the incubation period (t1), expressed in days. For particulate matter measurements (TPC, PON, POC, and Chl-a), duplicate samples were filtered onto GF/F filters (Whatmann, pre-combusted at 500°C for 6 h) and stored in glass petri dishes (pre-combusted at 500°C for 6 h) at −20°C. Chl-a filters were placed into plastic vials, containing 90% acetone and glass beads, and the mixture was homogenized using a cell mill followed by centrifugation at 4°C (10 min. at 800 g). Subsequently, the supernatant was analyzed for with a Turner 10-AU fluorometer (Welschmeyer, 1994). Calibration of the fluorometer was performed using spinach Chl-a extract following the procedures of Strickland and Parsons (1972). Prior to analysis, filters for TPC and PON were dried overnight at 60°C while filters for POC were placed in a desiccator above a 37% HCl solution for 2 hours to remove all inorganic carbon and dried overnight at 60°C. Subsequently, carbon and nitrogen content of the filters were analyzed with a Euro EA Elemental Analyzer according to Hedges and Stern (1984). Particulate inorganic carbon was calculated from the difference between TPC and POC. Cell quota of particulate matter (PM = PIC, POC, PON, and Chl-a) was calculated as
where PM(filter) is the mass (pg) of particulate matter per filter, V is the volume (ml) filtrated and N is the number of cells per ml. Production rates (PIC pr., POC pr., and PON pr.) were calculated by multiplying the cell quota with the growth rate (μ). All values are given as the mean of triplicate treatments with associated standard deviations (±1 sd, n = 3).
Coccolith and Seawater Geochemistry
After sampling for physiological and seawater chemistry parameters, the remaining culture media of each treatment was centrifuged and concentrated using a Beckman AVANTITM J-25 Centrifuge. Aliquots of 500 and 50 ml were consecutively centrifuged in flat-bottom tubes (22,000 × g, 10 min at 4°C) until a single pellet for each replicate treatment was obtained. Pellets were dried 48 h at 60°C and preserved at room temperature. Prior to elemental analysis, sample pellets were cleaned following an established organic removal protocol involving a reduction with hydroxylamine-hydrochloride prior an oxidation with H2O2 (Blanco-Ameijeiras et al., 2012). Mg/Ca and Sr/Ca ratios of the coccoliths and the artificial growth media were analyzed using matrix-matched standards on a simultaneous dual ICP-AES (Thermo ICAP DUO 6300 at the University of Oviedo, Spain). Standards were prepared from single element ICP standards (CPI Corporation) diluted in the same acid matrix as the samples. The calibration standards covered the Mg/Ca and Sr/Ca range of the calcite samples. Elemental ratios are reported from measurements made in radial detection mode for Sr at 421 nm, Mg at 279.5 nm, Fe at 259 nm, Ca at 315 nm, and P at 177 nm. Calibration was conducted offline using the intensity ratio method described by de Villiers et al. (2002). In this case, three standards were prepared with constant Ca concentrations and variable Sr/Ca and Mg/Ca ratios of 0.75 to 4 mmol mol–1. Aliquots from this set of standards were uniformly diluted to provide curves for various Ca concentrations to match sample concentrations. Sr/Ca internal reproducibility was better than 0.02 mmol mol–1, based on replicate analyses of the same sample dilutions. Mg/Ca internal reproducibility was better than 0.8% of the measured ratio. Coccolith elemental ratios of each replicate treatment were analyzed in duplicate measurements. Coccolith P/Ca and Fe/Ca ratios were used as an indicator for Mg cleaning efficiency and contamination. Outlier detection, using the interquartile range, was performed on P/Ca and Fe/Ca ratios to determine non-sufficient cleaning and the corresponding Mg/Ca and Sr/Ca ratios were removed from the data set. Average values for P/Ca and Fe/Ca ratios (excluding outliers) were 1.41 ± 1.16 and 1.22 ± 1.10 mmol mol–1 (±1 sd; n = 28), respectively, which is in line with previous results on P/Ca and Fe/Ca as contamination index (Müller et al., 2014). Artificial seawater media analysis resulted in Sr/Ca and Mg/Ca ratios of 9.735 ± 0.037 mmol mol–1 and 5.65 ± 0.022 mol mol–1 (±1 sd, n = 6), respectively.
The partition coefficient of the trace metal (Sr and Mg), DTr, between calcite (c), and seawater (s) was calculated as:
Results
The growth rate of G. oceanica exhibited an optimum response to the applied temperature gradient with the highest rate of 1.09 ± 0.01 d–1 and the lowest rate of 0.23 ± 0.00 d–1 at 21°C and 12°C, respectively (Figure 1 and Table 1). The low growth rate at 12°C resulted in low biomass production over time, and as a consequence all available biomass from this treatment was harvested for elemental analysis of biogenic CaCO3, with no samples being taken for TPC, POC, PON, and Chl-a. No specific trends in cellular quota of POC, PIC, and PON were detected in regard to the temperature range from 15 to 27°C. Similar results were obtained for the cellular ratios of PIC:POC and POC:PON and the production rates of POC, PIC and PON (see Supplementary Figure 1). Chl-a content, on the other hand, indicated a clear temperature relation similar to the growth rate with a parabolic behavior peaking at 21°C. This trend was enhanced for Chl-a production rate due to multiplying Chl-a content per cell with the growth rate (Supplementary Figure 1j). The ratios of POC:Chl-a and PON:Chl-a demonstrated lowest values at 21°C with increasing values diverging from this point, mainly driven by the temperature relation of Chl-a cellular content.
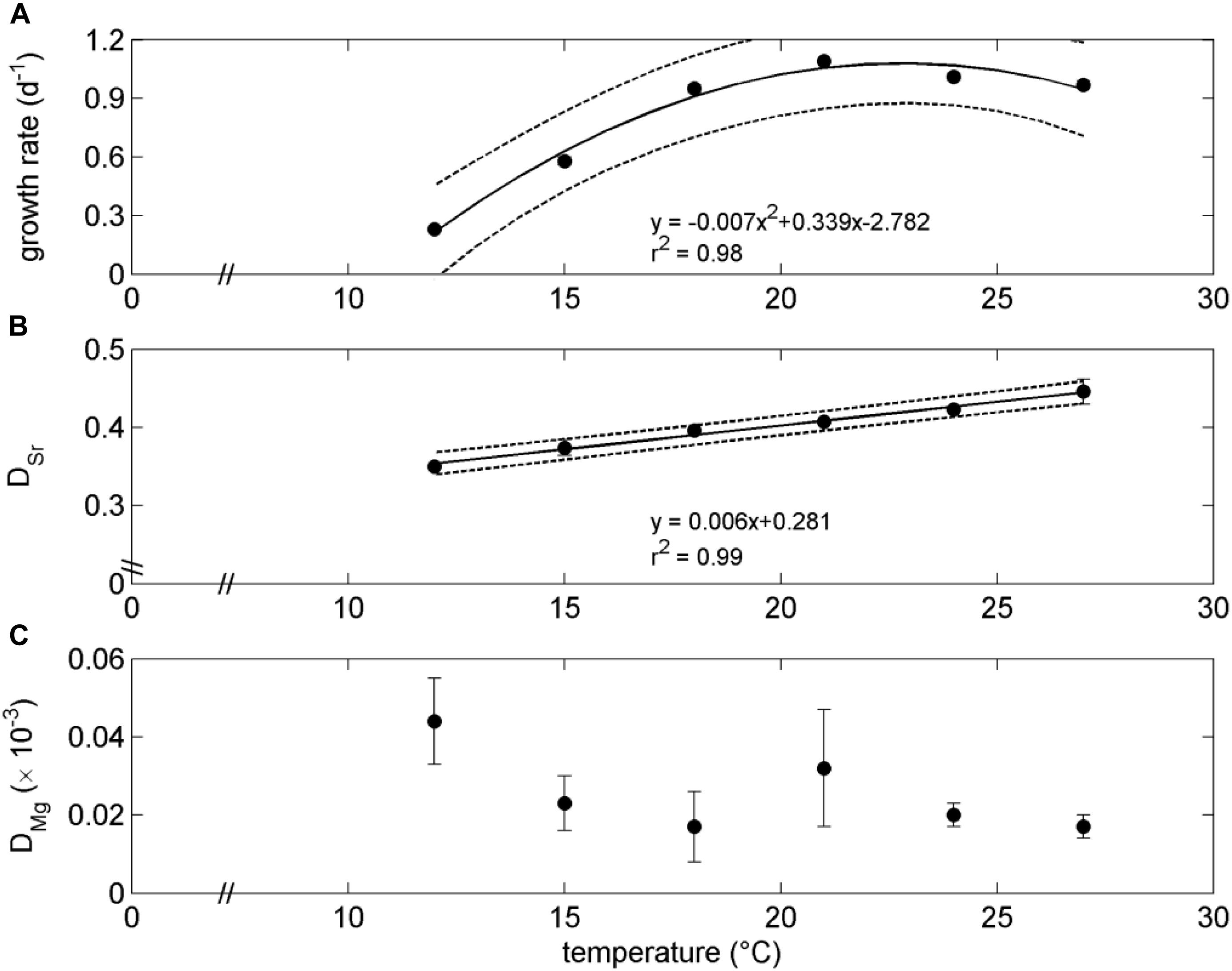
Figure 1. Growth rate (A) of G. oceanica and associated values of DSr (B) and DMg (C) for biogenic produced calcite in relation to the experimental applied temperature gradient.
Analyzed coccolith Sr/Ca and Mg/Ca ranged from 3.40 ± 0.01 to 4.35 ± 0.16 mol mol–1 and from 0.095 ± 0.015 to 0.250 ± 0.064 mmol mol–1, respectively (Table 1), which resulted in DSr and DMg ranging from 0.350 ± 0.001 to 0.446 ± 0.016 and from 0.017 ± 0.003 × 10–3 to 0.044 ± 0.011 × 10–3, respectively. A clear significant linear relation between DSr and temperature emerged whereas no trend was detected regarding DMg and temperature (Figures 1B,C). Population growth rate was also significantly correlated with DSr over the applied temperature gradient from 12 to 27°C (Figure 2B). However, this correlation was mainly driven by data points from low temperatures (below the optimum growth temperature) which became evident when individually correlating DSr with temperature and population growth at low temperatures (12–21°C), high temperatures (18–27°C), and the total applied temperature range (12–27°C). Only the correlation between DSr and temperature resulted in significant linear regressions at each temperature range (Figure 2).
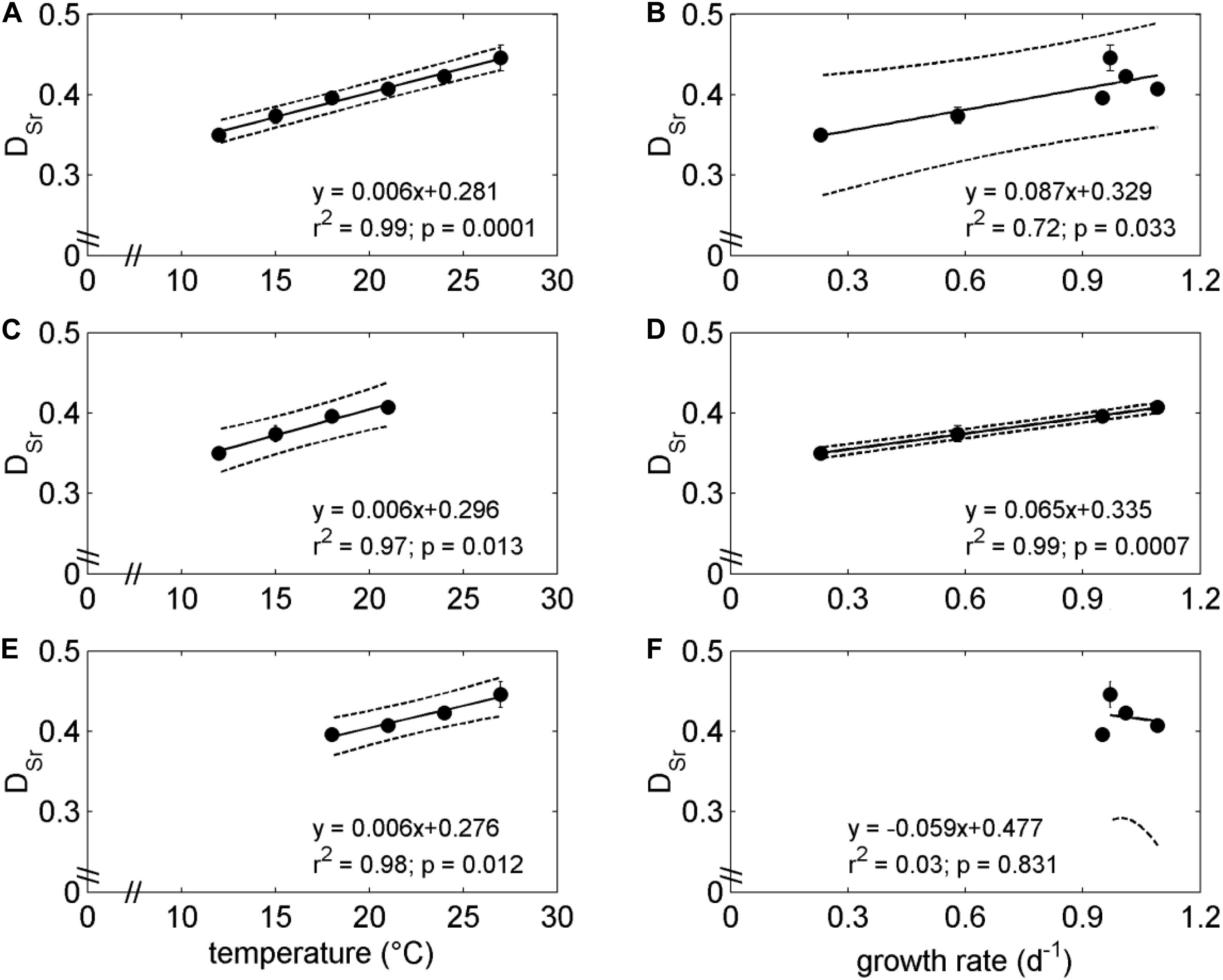
Figure 2. Regression analysis investigating the relation of DSr with temperature (A,C,E) and population growth rate (B,D,F). (B,D,F) represent data points at temperatures from 12 to 27, 12 to 21, and 18 to 27°C, respectively.
Discussion
The presented experiment was conducted over a temperature gradient from 12 to 27°C. Temperature has a direct influence on the equilibrium constants of the carbonate system and the applied temperature gradient has the potential to induce indirect carbonate chemistry effects on cellular physiological and calcite precipitation kinetics. However, this temperature effect on carbonate chemistry was masked by biological activity and experimental handling procedures. Consequently, no relations between temperature and carbonate chemistry parameters were observed (Supplementary Table 1). Seawater pCO2 and pH varied around average values of 404 ± 71 μatm and 8.03 ± 0.07, respectively. While changes in carbonate chemistry are known to influence coccolithophore physiology and coccolith geochemistry (Hoppe et al., 2011; Müller et al., 2014), the here registered carbonate chemistry variation can be neglected in comparison to the temperature effects as no significant changes in physiology and geochemistry have been observed within the applied carbonate chemistry range (Müller et al., 2014, 2018; Gafar et al., 2019). Therefore, the here observed changes in physiology and coccolith Sr/Ca and Mg/Ca ratios are related to the applied temperature gradient. However, a possible effect of temperature induced carbonate chemistry variation on physiology and coccolith geochemistry cannot be excluded. Experimental designs to disentangle carbonate chemistry from temperature effects should be considered for future investigations.
The applied temperature gradient resulted in optimum growth behavior of G. oceanica. The fitted regression model indicated an optimum growth temperature at ∼24°C, which is similar to results from previous studies indicating highest growth rates of G. oceanica at 25°C (Buitenhuis et al., 2008; Sett et al., 2014). The optimum point and skewness of coccolithophore growth curves, however, can be influenced by the applied experimental conditions (e.g., light, salinity, and growth media), and by strain specific physiological reaction norms (Müller et al., 2015; Gafar et al., 2018, 2019). Cell quotas of POC, PIC, and PON did not indicate specific trends in regard to the applied temperature gradient, which was additionally reflected in the absence of a trend in the corresponding ratios of PIC:POC and POC:PON (Supplementary Figure 1), confirming the previously observed temperature independence of cellular carbon quota in G. oceanica (Sett et al., 2014) which is different than the observed relation of temperature and cell quota or cell volume in Emiliania huxleyi (Rosas-Navarro et al., 2016; Müller et al., 2021), stressing the importance of species specific response-mechanisms and the importance of understanding the regulating mechanisms for coccolithophore cell quota and allometry (Aloisi, 2015). It is, on the other hand, intriguing that cellular Chl-a demonstrated a similar temperature dependency as observed for the cellular growth rate (Figure 1A and Supplementary Figure 1d). This indicates that G. oceanica is able to maintain cellular organic and inorganic carbon quota at a relatively constant level over the applied temperature gradient while adjusting cellular Chl-a content, associated carbon fixation and ATP production inside the chloroplast. Disentangling the allocation of POC into cellular lipid, protein and carbohydrate compounds might give additional information on the underlying physiological mechanisms controlling relatively constant cellular POC and PON quota (Aveiro et al., 2020). The feature of G. oceanica exhibiting optimum curve behavior in growth rate and in cellular Chl-a content in response to temperature while keeping relatively constant cellular quota of PIC, POC and PON, provides an interesting opportunity to link physiological reaction norms to the coccolith geochemical signature (i.e., DSr and DMg).
The coccolith DSr indicated a clear linear relationship with temperature (Figure 1B), with values comparable to previous culture studies on coccolithophores (Rickaby et al., 2002; Stoll et al., 2002; Langer et al., 2006; Müller et al., 2014). It is intriguing that the temperature relationship of DSr is linear over the tested temperature range, while no physiological parameter exhibited linear relationship to temperature (Supplementary Figure 1). Previous laboratory temperature experiments investigated solely temperature values below the species specific optimal growth temperature (e.g., Stoll et al., 2002; Müller et al., 2014), which resulted in a significant linear relationship between species growth rate and coccolith DSr (see Figure 2D). Here, however, we demonstrate that this relationship is challenged when investigating temperatures above the optimal growth temperature (Figures 1, 2).
The difficulties of relating coccolith DSr to physiological responses (when manifested in the form of optimum curves) has been previously observed using extended experimental gradients of seawater pCO2/pH (Müller et al., 2014). However, natural coccolith samples from surface waters, sediment traps and sediments are likely produced at temperatures below the optimum growth temperature because evolutionary adaptation strategies will favor ecological niches below or at temperature optima. This is related to a common feature of organismal fitness curves being unimodal with a sharper decline above the optimum temperature than below. Organisms thriving at optimum temperatures are more sensitive to warming than to cooling (Eppley, 1972; Kingsolver, 2009) resulting in a general distribution of phytoplankton at habitats below or at their optimal growth temperature (Thomas et al., 2012). Therefore, we postulate that the previous observed relation between coccolith DSr (or Sr/Ca ratio) and growth or production rates (Stoll et al., 2007; Auliaherliaty et al., 2009) remains applicable as proxy but only partly describes the underlying physiological and/or thermodynamic reasons. It is interesting that the temperature-DSr correlation is maintained above the temperature optimum for coccolithophore growth (Figure 1) and, additionally, that elemental Sr fractionation in inorganic precipitated calcite follows an opposite negative trend to temperature (Tang et al., 2008). This divergence between inorganic and coccolithophore calcite precipitation is certainly related to cellular physiology and associated transmembrane transport mechanisms. However, the question that arises is whether this physiological influence is an active and selected control/transport of Sr ions to the site of calcification, or if Sr is passively transported and “hitchhikes” ion transport ways established for other divalent cations, such as, for example, the Ca-flux from seawater to the site of calcification, the coccolith vesicle. Our observations give an important insight on the physiological control on Sr transport and fractionation into coccolith calcite. First, it is essential to remember the underlying physiological processes that induce an optimum physiological response curve in regard to (a) carbonate chemistry (Müller et al., 2015; Gafar et al., 2018) and (b) temperature. In the first case, seawater carbonation supplies carbon fixation for the enzyme Rubisco resulting in an increase of energy availability and growth rate. Further elevation of CO2 increases the seawater H+ concentration (or acidity), which interferes with cellular pH levels and enzymatic activity resulting in a general decrease of growth (Bach et al., 2011; Suffrian et al., 2011). In the case of temperature, the optimal reaction norms of microbial growth are governed by enzymatic activity. In principle, the increase in cellular chemical and enzymatic reaction rates with temperature can be associated to the Arrhenius relationship and increased kinetic energy availability. The reaction rate of enzymes, however, does not uniformly follow temperature increases and depends on enzymatic stability, denaturation and evolutionary history. Therefore, elevated temperatures as well as other environmental drivers can result in enzymatic activity loss. Additionally, biomembrane permeability/fluidity and resulting transmembrane ion transport depend on the ambient temperature (i.e., kinetic energy available) and the membrane composition of polar lipids to maintain homeoviscous stability control (Valentine and Valentine, 2004; Kellermann et al., 2016). Therefore, cellular physiology and the current state of the cell can be quite different below and above the growth optimum, having to regulate different essential cellular functions and maintaining an energetic balance between costs and benefits. Thus, if a primarily physiological control exists on DSr, it is intriguing that this control is not disturbed when the cell switches from the physiological states below to the state above the temperature optimum. This is even more surprising when remembering that the Sr incorporation into coccolith calcite is not a vital process. Interestingly, no trend was detected in DMg in regard to temperature, which is consistent with previous results (Müller et al., 2014). This indicates that DMg of coccolithophore calcite seems to be inappropriate as a paleoproxy for seawater temperature as it has been applied for foraminifera (e.g., Mawbey et al., 2020). This difference is presumably related to the diverging calcification mechanism and associated ion transport from seawater to the site of calcification (vacuolization vs transmembrane ion transport; Nehrke et al., 2013). On the other hand, species-specific trends were identified in DMg with regards to increasing pCO2 levels (Müller et al., 2014; Li et al., 2016). Contrary to Müller et al. (2014), here, we could not identify a relation between Chl-a production and coccolith DMg. In comparison to Sr analysis of coccolith calcite, the determination of Mg encounters the necessity of sufficient cleaning and removing of organic Mg from the sample material. Mg is a crucial biological element and involved in important physiological functions (e.g., cell signaling, RNA/DNA synthesis, cofactor for protein activity, and membrane structure) and highly abundant in the cellular cytosol and compartments. Sr, on the other hand, is considered a non-essential element for plant growth (Comar, 1955) and has a relatively low abundance in phytoplankton cells compared to other elements (Ho et al., 2003). Therefore, efficient cleaning methods had been developed to minimize a possible contamination of organic Mg during coccolith Mg/Ca analysis (Blanco-Ameijeiras et al., 2012; Li et al., 2016). However, the associated error of the cleaning procedure applied here and Mg analysis is not necessarily satisfied when compared to the associated error for Sr analysis. The calculated range of the relative standard deviation of DSr and DMg resulted in 0.2–3.6% and 15–53%, respectively. The DMg values are associated with an error of a magnitude higher than DSr values and points toward the need of adjusting and developing improved protocols and methods for coccolith Mg analysis. For example, the replacement of organic bound Mg in coccolithophore sample material by divalent cations with a higher ion exchange affinity (e.g., Cu) and subsequent analysis of Mg via high performance chelation ion chromatography resulted in a relative standard deviation of DMg ranging from 2.7 to 11.4% (Li et al., 2016). The successful removal of organic Mg when analyzing coccolithophore calcite is essential due to the fact that coccolithophores precipitate low-Mg calcite (<0.1% mol), and that Mg has an essential role in cellular biochemical reactions and has a 20,000 times higher cytosol concentration than Ca (Brownlee et al., 1995; Bose et al., 2011). It might be possible that the lack of a visible trend in DMg in regard to temperature (Figure 1C) is superimposed by the relatively high associated error of the here conducted cleaning procedure and analysis.
Transferring results from single species laboratory experiments to the sediment record is not trivial because single strain responses have to be disentangled from shifts in population strain diversity, especially in regard to the high phenotypic variability observed in coccolithophore species (Blanco-Ameijeiras et al., 2016). Addressing this issue will give us important notions on the question if physicochemical parameters alter/control changes in Sr/Ca and Mg/Ca of calcareous sediments and/or if this change can be biological buffered or enhanced. Additionally, future experiments will greatly benefit from exploring distinct physiological states of coccolithophores, such as comparing controlled nutrient limitation (using chemostat cultures) and nutrient starvation (using batch cultures) with additional investigation of elemental fractionation, coccolithophore allometry, and the content of the cellular organic material (Aloisi, 2015; Müller et al., 2018, 2021).
The presented results clearly indicate the importance of investigating the full reaction norms (i.e., non-linear physiological responses) of coccolithophores to understand the underlying physiological and thermokinetics of elemental Sr fractionation in coccolithophore calcite. We postulate that investigations and experimentations above the temperature growth optimum have the potential to give new and useful insights on the physiological influence on coccolith geochemistry. The application of coccolith Sr/Ca ratio as proxy for population growth or productivity is not necessarily influenced by our findings because natural populations of coccolithophores predominantly occur below or at their species specific temperature optimum.
Data Availability Statement
All relevant data is contained within the article: The original contributions presented in the study are included in the article/Supplementary Material, further inquiries can be directed to the corresponding author/s.
Author Contributions
SB-A and ML conceived and conducted the experiment. HS, SB-A, and AM-V conducted geochemical analysis. SB-A and MM analyzed the data and wrote the manuscript with essential contributions from all authors.
Funding
This work was funded by the “European Project on Ocean Acidification” [EPOCA; which received funding from the European Community’s Seventh Framework Program (FP7/2007-2013) under grant agreement No. 211384] for the Ph.D. of ML, Abbey-Santander Internationalization Fund to SB-A, and ERC-STG-240222PACE for funding HS and AM-V. The National Council for Scientific and Technological Development – CNPq funds MM (305467/2020-4).
Conflict of Interest
The authors declare that the research was conducted in the absence of any commercial or financial relationships that could be construed as a potential conflict of interest.
Acknowledgments
We are grateful to Tania Klüver for laboratory assistance and Julie LaRoche for allowing the use of their laboratory facilities at Helmholtz Centre for Ocean Research Kiel (GEOMAR).
Supplementary Material
The Supplementary Material for this article can be found online at: https://www.frontiersin.org/articles/10.3389/feart.2021.582521/full#supplementary-material
References
Aloisi, G. (2015). Covariation of metabolic rates and cell size in coccolithophores. Biogeosciences 12, 4665–4692. doi: 10.5194/bg-12-4665-2015
Auliaherliaty, L., Stoll, H. M., Ziveri, P., Malinverno, E., Triantaphyllou, M., Stravrakakis, S., et al. (2009). Coccolith Sr/Ca ratios in the eastern Mediterranean: production versus export processes. Mar. Micropaleontol. 73, 196–206. doi: 10.1016/j.marmicro.2009.10.001
Aveiro, S. S., Melo, T., Figueiredo, A., Domingues, P., Pereira, H., Maia, I. B., et al. (2020). The polar lipidome of cultured Emiliania huxleyi: a source of bioactive lipids with relevance for biotechnological applications. Biomolecules 10:1434. doi: 10.3390/biom10101434
Bach, L. T., Riebesell, U., and Schulz, K. G. (2011). Distinguishing between the effects of ocean acidification and ocean carbonation in the coccolithophore Emiliania huxleyi. Limnol. Oceanogr. 56, 2040–2050. doi: 10.4319/lo.2011.56.6.2040
Beaufort, L., Probert, I., de Garidel-Thoron, T., Bendif, E. M., Ruiz-Pino, D., Metzl, N., et al. (2011). Sensitivity of coccolithophores to carbonate chemistry and ocean acidification. Nature 476, 80–83. doi: 10.1038/nature10295
Blanco-Ameijeiras, S., Lebrato, M., Stoll, H. M., Iglesias-Rodriguez, D., Müller, M. N., Méndez-Vicente, A., et al. (2016). Phenotypic variability in the coccolithophore Emiliania huxleyi. PLoS One 11:e0157697. doi: 10.1371/journal.pone.0157697
Blanco-Ameijeiras, S., Lebrato, M., Stoll, H. M., Iglesias-Rodriguez, M. D., Mendez-Vicente, A., Sett, S., et al. (2012). Removal of organic magnesium in coccolithophore carbonates. Geochim. Cosmochim. Acta 89, 226–239. doi: 10.1016/j.gca.2012.04.043
Bose, J., Babourina, O., and Rengel, Z. (2011). Role of magnesium in alleviation of aluminium toxicity in plants. J. Exp. Biol. 62, 2251–2264. doi: 10.1093/jxb/erq456
Bown, P. R., Lees, J. A., and Young, J. R. (2004). “Calcareous nannoplankton evolution and diversity through time,” in Coccolithophores – from Molecular Processes to Global Impact, eds H. R. Thierstein and J. R. Young (New York, NY: Springer).
Brownlee, C., Davies, M., Nimer, N., Dong, L. F., and Merrett, M. J. (1995). Calcification, photosynthesis and intracellular regulation in Emiliania huxleyi. Bull. Inst. Oceanogr. Monaco 14, 19–35.
Buitenhuis, E. T., Pangerc, T., Franklin, D. J., Le Quere, C., and Malin, G. (2008). Growth rates of six coccolithophorid strains as a function of temperature. Limnol. Oceanogr. 53, 1181–1185. doi: 10.4319/lo.2008.53.3.1181
Comar, C. L. (1955). Radioisotopes in Biology and Agriculture (p. 302). New York, NY: McGraw-Hill Book Co.
de Villiers, S., Greaves, M., and Elderfield, H. (2002). An intensity ratio calibration method for the accurate determination of Mg/Ca and Sr/Ca of marine carbonates by ICP-AES. Geochem. Geophys. Geosyst. 3:2001GC000169. doi: 10.1029/2001GC000169
Edmond, J. M. (1970). High precision determination of titration alkalinity and total carbon dioxide content of sea water by potentiometric titration. Deep Sea Res. 17, 737–750. doi: 10.1016/0011-7471(70)90038-0
Erba, E. (2006). The first 150 million years history of calcareous nannoplankton: biosphere–geosphere interactions. Palaeogeogr. Palaeocl. 232, 237–250. doi: 10.1016/j.palaeo.2005.09.013
Gafar, N. A., Eyre, B. D., and Schulz, K. G. (2018). A conceptual model for projecting coccolithophorid growth, calcification and photosynthetic carbon fixation rates in response to global ocean change. Front. Mar. Sci. 4:433. doi: 10.3389/fmars.2017.00433
Gafar, N. A., Eyre, B. D., and Schulz, K. G. (2019). A comparison of species specific sensitivities to changing light and carbonate chemistry in calcifying marine phytoplankton. Sci. Rep. 9:2486. doi: 10.1038/s41598-019-38661-0
Gibbs, S., Poulton, A., Bown, P., Daniels, C. J., Hopkins, J., Young, J. R., et al. (2013). Species-specific growth response of coccolithophores to Palaeocene–Eocene environmental change. Nat. Geosci. 6, 218–222. doi: 10.1038/ngeo1719
Guillard, R. (1975). “Culture of phytoplankton for feeding marine invertebrates,” in Culture of Marine Invertebrate Animals, eds W. Smith and M. H. Chanley (New York, NY: Springer), 29–60. doi: 10.1007/978-1-4615-8714-9_3
Hansen, H. P., and Koroleff, F. (1999). “Determination of nutrients,” in Methods of Seawater Analysis, 3rd Edn, eds K. Grasshoff, K. Kremling, and M. Erhardt (Weinheim: Wiley-VCH), 159–226.
Hedges, J. I., and Stern, J. H. (1984). Carbon and nitrogen determinations of carbonate-containing solids. Limnol. Oceanogr. 29, 657–663. doi: 10.4319/lo.1984.29.3.0657
Hermoso, M., Horner, T. J., Minoletti, F., and Rickaby, R. E. M. (2014). Constraints on the vital effect in coccolithophore and dinoflagellate calcite by oxygen isotopic modification of seawater. Geochim. Cosmochim. Acta 141, 612–627. doi: 10.1016/j.gca.2014.05.002
Ho, T.-Y., Quigg, A., Finkel, Z. V., Milligan, A. J., Wyman, K., Falkowski, P. G., et al. (2003). The elemental composition of some marine phytoplankton. J. Phycol. 39, 1145–1159. doi: 10.1111/j.0022-3646.2003.03-090.x
Hoppe, C. J. M., Langer, G., and Rost, B. (2011). Emiliania huxleyi shows identical responses to elevated pCO2 in TA and DIC manipulations. J. Exp. Mar. Biol. 406, 54–62. doi: 10.1016/j.jembe.2011.06.008
Kellermann, M. Y., Yoshinaga, M. Y., Valentine, R. C., Wörmer, L., and Valentine, D. L. (2016). Important roles for membrane lipids in haloarchaeal bioenergetics. Biochim. Biophys. Acta Biomembr. 1858, 2940–2956. doi: 10.1016/j.bbamem.2016.08.010
Kester, D. R., Duedall, I. W., Conners, D. N., and Pytkowicz, R. M. (1967). Preparation of artificial seawater. Limnol. Oceanogr. 12, 176–179.
Kingsolver, J. G. (2009). The well-temperatured biologist. Am. Nat. 174, 755–768. doi: 10.1086/648310
Langer, G., Nehrke, G., Riebesell, U., Eisenhauer, A., Kuhnert, H., Rost, B., et al. (2006). Coccolith strontium to calcium ratios in Emiliania huxleyi: the dependence on seawater strontium and calcium concentrations. Limnol. Oceanogr. 51, 310–320. doi: 10.4319/lo.2006.51.1.0310
Li, Y., Müller, M. N., Paull, B., and Nesterenko, P. N. (2016). Precise and fast determination of inorganic magnesium in coccolithophore calcite. Chem. Geol. 437, 1–6. doi: 10.1016/j.chemgeo.2016.05.010
Mawbey, E. M., Hendry, K. R., Greaves, M. J., Hillenbrand, C.-D., Kuhn, G., Spencer-Jones, C. L., et al. (2020). Mg/Ca-temperature calibration of polar Benthic foraminifera species for reconstruction of bottom water temperatures on the Antarctic shelf. Geochim. Cosmochim. Acta 283, 54–66. doi: 10.1016/j.gca.2020.05.027
Müller, M. N., Brandini, F. P., Trull, T. W., and Hallegraeff, G. M. (2021). Coccolith volume of the Southern Ocean coccolithophore Emiliania huxleyi as a possible indicator for palaeo−cell volume. Geobiology 19, 63–74. doi: 10.1111/gbi.12414
Müller, M. N., Krabbenhöft, A., Vollstaedt, H., Brandini, F. P., and Eisenhauer, A. (2018). Stable isotope fractionation of strontium in coccolithophore calcite: influence of temperature and carbonate chemistry. Geobiology 16, 297–306. doi: 10.1111/gbi.12276
Müller, M. N., Lebrato, M., Riebesell, U., Barcelos e Ramos, J., Schulz, K. G., Blanco-Ameijeiras, S., et al. (2014). Influence of temperature and CO2 on the strontium and magnesium composition of coccolithophore calcite. Biogeosciences 11, 1065–1075. doi: 10.5194/bg-11-1065-2014
Müller, M. N., Trull, T. W., and Hallegraeff, G. M. (2015). Differing responses of three Southern Ocean Emiliania huxleyi ecotypes to changing seawater carbonate chemistry. Mar. Ecol. Prog. Ser. 531, 81–90. doi: 10.3354/meps11309
Nehrke, G., Keul, N., Langer, G., de Nooijer, L. J., Bijma, J., and Meibom, A. (2013). A new model for biomineralization and trace element signatures of Foraminifera tests. Biogeosciences 10, 6759–6767. doi: 10.5194/bg-10-6759-2013
Rickaby, R. E. M., Schrag, D. P., Zondervan, I., and Riebesell, U. (2002). Growth rate dependence of Sr incorporation during calcification of Emiliania huxleyi. Glob. Biogeochem. Cycles 16:1006. doi: 10.1029/2001GB001408
Riebesell, U., Fabry, V. J., Hansson, L., and Gattuso, J.-P. (2010). Guide to Best Practices for Ocean Acidification Research and Data Reporting. Luxembourg: Publications Office of the European Union.
Ries, J. B. (2010). Review: geological and experimental evidence for secular variation in seawater Mg/Ca (calcite-aragonite seas) and its effects on marine biological calcification. Biogeosciences 7, 2795–2849. doi: 10.5194/bg-7-2795-2010
Rosas-Navarro, A., Langer, G., and Ziveri, P. (2016). Temperature affects the morphology and calcification of Emiliania huxleyi strains. Biogeosciences 13, 2913–2926. doi: 10.5194/bg-13-2913-2016
Roy, R. N., Roy, L. N., Vogel, K. M., Porter-Moore, C., Pearsona, T., Good, C. E., et al. (1993). The dissociation constants of carbonic acid in seawater at salinities 5 to 45 and temperatures 0 to 45°C. Mar. Chem. 44, 249–267. doi: 10.1016/0304-4203(93)90207-5
Sett, S., Bach, L. T., Schulz, K. G., Koch-Klavsen, S., Lebrato, M., and Riebesell, U. (2014). Temperature modulates coccolithophorid sensitivity of growth, photosynthesis and calcification to increasing seawater pCO2. PLoS One 9:e88308. doi: 10.1371/journal.pone.0088308
Stoll, H. M., Klaas, C. M., Probert, I., Ruiz Encinar, J., and Garcia Alonso, J. I. (2002). Calcification rate and temperature effects on Sr partitioning in coccoliths of multiple species of coccolithophorids in culture. Glob. Planet. Change 34, 153–171. doi: 10.1016/s0921-8181(02)00112-1
Stoll, H. M., and Schrag, D. P. (2000). Coccolith Sr / Ca as a new indicator of coccolithophorid calcification and growth rate. Geochem. Geophys. Geosyst. 1, 1–24.
Stoll, H. M., and Ziveri, P. (2004). “Coccolithophorid-based geochemical paleoproxies,” in Coccolithophores – from Molecular Processes to Global Impact, eds H. R. Thierstein and J. R. Young (New York, NY: Springer).
Stoll, H. M., Ziveri, P., Shimizu, N., Conte, M., and Theroux, S. (2007). Relationship between coccolith Sr / Ca ratios and coccolithophore production and export in the Arabian Sea and Sargasso Sea. Deep Sea Res. II 54, 581–600. doi: 10.1016/j.dsr2.2007.01.003
Strickland, J. D. H., and Parsons, T. R. (1972). A Practical Handbook of Seawater Analysis, ed. J. C. Stevenson Ottawa, ON: Fisheries research board of Canada.
Suffrian, K., Schulz, K. G., Gutowska, M. A., Riebesell, U., and Bleich, M. (2011). Cellular pH measurements in Emiliania huxleyi reveal pronounced membrane proton permeability. New Phytol. 190, 595–608. doi: 10.1111/j.1469-8137.2010.03633.x
Tang, J., Köhler, S. J., and Dietzel, M. (2008). Sr2+/Ca2+ and 44Ca/40Ca fractionation during inorganic calcite formation: I. Sr incorporation. Geochim. Cosmochim. Acta 72, 3718–3732. doi: 10.1016/j.gca.2008.05.031
Thomas, M. K., Kremer, C. T., Klausmeier, C. A., and Litchman, E. (2012). A global pattern of thermal adaptation in marine phytoplankton. Science 338, 1085–1088. doi: 10.1126/science.1224836
Valentine, R. C., and Valentine, D. L. (2004). Omega-3 fatty acids in cellular membranes: a unified concept. Prog. Lipid Res. 43, 383–402. doi: 10.1016/j.plipres.2004.05.004
Welschmeyer, N. (1994). Fluorometric analysis of chlorophyll a in the presence of chlorophyll b and pheopigments. Limnol. Oceanogr. 39, 1985–1992. doi: 10.4319/lo.1994.39.8
Keywords: paleoproxy, geochemistry, calibration, coccolith elemental fractionation, coccolithophore
Citation: Müller MN, Blanco-Ameijeiras S, Stoll HM, Mendez-Vicente A and Lebrato M (2021) Temperature Induced Physiological Reaction Norms of the Coccolithophore Gephyrocapsa oceanica and Resulting Coccolith Sr/Ca and Mg/Ca Ratios. Front. Earth Sci. 9:582521. doi: 10.3389/feart.2021.582521
Received: 12 July 2020; Accepted: 30 March 2021;
Published: 20 April 2021.
Edited by:
Oscar Branson, University of Cambridge, United KingdomReviewed by:
Yong Zhang, Fujian Normal University, ChinaDavid Evans, Goethe University Frankfurt, Germany
Copyright © 2021 Müller, Blanco-Ameijeiras, Stoll, Mendez-Vicente and Lebrato. This is an open-access article distributed under the terms of the Creative Commons Attribution License (CC BY). The use, distribution or reproduction in other forums is permitted, provided the original author(s) and the copyright owner(s) are credited and that the original publication in this journal is cited, in accordance with accepted academic practice. No use, distribution or reproduction is permitted which does not comply with these terms.
*Correspondence: Marius N. Müller, bWFyaXVzbm11bGxlckBnbWFpbC5jb20=